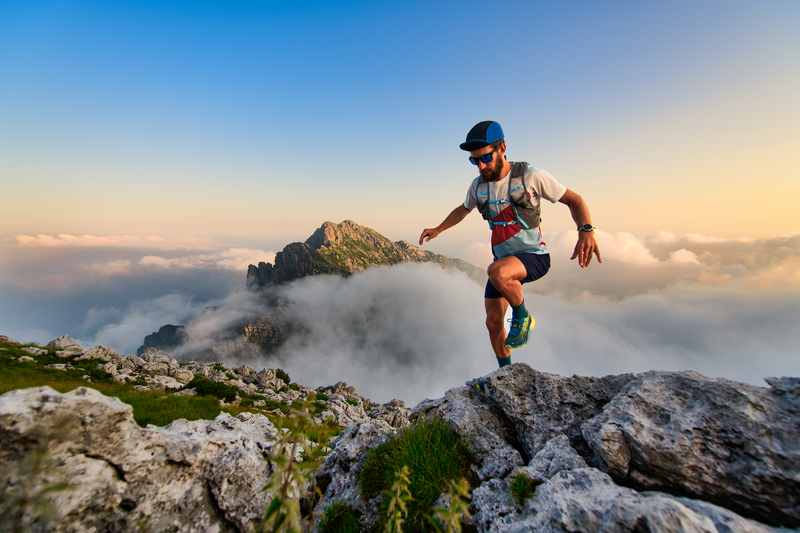
95% of researchers rate our articles as excellent or good
Learn more about the work of our research integrity team to safeguard the quality of each article we publish.
Find out more
ORIGINAL RESEARCH article
Front. Ecol. Evol. , 19 September 2023
Sec. Models in Ecology and Evolution
Volume 11 - 2023 | https://doi.org/10.3389/fevo.2023.1197757
This article is part of the Research Topic Modeling, Data Handling, and Analysis: Practical and Fundamental Consequences in Ecology and Evolution View all 9 articles
Introduction: Various herbivorous insects prefer toxic plants as their hosts, although this may appear paradoxical. They have evolved specific adaptations (called counter-defenses) against the toxins. For example, the two-component chemical defense system of plants of the Brassicaceae family against herbivores consists of glucosinolates (GLSs) and the activating enzyme myrosinase. GLS hydrolysis by myrosinase leads to isothiocyanates (ITCs), which are toxic and deterrent to many insect herbivores. Two different types of counter-defenses can be distinguished: a preemptive counter-defense that prevents the GLSs from being hydrolyzed to ITCs due to metabolic redirection and direct counter-defense, where the ITCs are formed but then metabolized to non-toxic conjugates. In general, preemptive counter-defense is only a possibility if the plant stores a precursor of the toxin, which is activated upon attack. Preemptive counter-defense is believed to be more efficient due to the lower exposure to ITCs, but this has not been satisfactorily demonstrated experimentally.
Methods: Here, we derive on theoretical grounds the conditions under which preemptive counter-defense reduces exposure to ITCs compared to direct counter-defense by studying the dynamics of GLS defense and counter-defense with two separate ordinary differential equation models. We model how herbivory transfers the GLSs to the insect gut with the leaf material. Thereafter, we describe the insects’ exposure to toxins by deriving the dynamics of ITCs in the gut during feeding with the two types of counter-defenses.
Results and discussion: By calculating the area under the curve (AUC) of the ITC concentrations, we show, based on empirical data, that herbivores with a preemptive detoxification system are usually less exposed to ITCs. In addition, our models explain how the decline in the level of ITCs is achieved by both counter-defenses, which helps to understand the overall mechanisms and benefits of these techniques. Our results may also apply to plant defenses by inactive toxin precursors other than GLSs as well as to insects that sequester such precursors for their own defense.
One of the best-studied plant chemical defenses is based on the glucosinolates (GLSs), found principally in the Brassicaceae and related families. GLSs are accompanied by a glucohydrolase called myrosinase that upon herbivory converts GLSs into active forms, which are toxic and deterrent to herbivores (Wittstock et al., 2003; Halkier and Gershenzon, 2006). The most widespread active forms of GLSs are isothiocyanates (ITCs), which have been demonstrated to be toxic to many insect herbivores (Wittstock and Burow, 2010; Sun et al., 2019) (Figure 1). Despite the GLS–myrosinase defense system, some insects are observed to feed on GLS-containing plants. In several cases, these insects have been demonstrated to possess different types of detoxification enzymes (Schramm et al., 2012; Jeschke et al., 2016; Zou et al., 2016) (Figure 1).
Figure 1 Schematic diagram for glucosinolate (GLS) defense of plants, together with preemptive and direct counter-defense in insect herbivores.
Specialist insects that feed on GLS-containing plants often prevent the GLSs from being hydrolyzed to ITCs by metabolic redirection. This detoxification scheme can be referred to as a preemptive counter-defense because it avoids the formation of toxic ITCs. For example, larvae of the large cabbage white (Pieris rapae) redirect GLS hydrolysis to form less toxic nitriles by using a nitrile-specifier protein (NSP) (Wittstock et al., 2004). Another example is provided by the larvae of the diamondback moth (Plutella xylostella) that desulfate GLSs before they can be hydrolyzed (Ratzka et al., 2002). However, a portion of GLSs can escape being metabolized by these preemptive mechanisms and produce ITC products via myrosinase-catalyzed hydrolysis (Jeschke et al., 2017).
Another adaption of some specialists is to absorb or accumulate GLSs in their bodies for their own defense (Petschenka and Agrawal, 2016; Beran et al., 2019; Yang et al., 2020; Sporer et al., 2021). For example, larvae of the turnip sawfly (Athalia rosae L.) and horseradish flea beetles (Phyllotreta armoraciae) absorb ingested GLSs from their gut (Müller et al., 2001; Sporer et al., 2021). Hydrolysis of GLSs by myrosinase is avoided by rapid absorption after ingestion and by partial inhibition of myrosinase activity (Sporer et al., 2021). This adaptation can also be considered a type of preemptive counter-defense. However, a portion of GLSs can escape the sequestration process and produce ITCs through myrosinase-catalyzed hydrolysis (Yang et al., 2020; Sporer et al., 2021).
In contrast to specialists, generalists feed only occasionally on GLS-containing plants and typically do not possess preemptive detoxification systems. Once ITCs have been formed, parts of these are detoxified directly via conjugation to the tripeptide glutathione (GSH) (Yu, 1987; Wadleigh and Yu, 1988; Schramm et al., 2012). Therefore, we call this adaptation direct counter-defense. Experimental studies have reported that lepidopteran generalists (e.g., Spodoptera littoralis, Spodoptera exigua, Trichoplusia ni, Mamestra brassicae, and Helicoverpa armigera) employ this detoxification strategy. In this case, a major portion of the ITCs is not conjugated to GSH but is released in the feces (Schramm et al., 2012; Jeschke et al., 2017).
Experimental studies show that specialist insects generally perform significantly better on GLS-containing plants than generalists (Li et al., 2000; Hopkins et al., 2009; Sarosh et al., 2010; Rohr et al., 2011) presumably due to lower exposure to ITCs. For example, when the preemptive desulfation detoxification system of P. xylostella was knocked down by RNA interference, the level of ITCs present in the gut increased by over 10-fold (Sun et al., 2019). Also, the published data (Jeschke et al., 2017) shown in Table 1 point to an advantage of preemptive counter-defense over direct counter-defense.
Table 1 Mean percentage of toxic and non-toxic products of GLSs excreted by various insects as measured by radioactivity (Jeschke et al., 2017).
However, it remains unclear if this is a general phenomenon or under which conditions this might change, all the more as ITC concentrations are difficult to measure experimentally in a time course. Therefore, it is very helpful to establish a mathematical model to compare the two different counter-defense strategies (preemptive and direct) against GLSs (Figure 1) and to derive a relation among the parameters under which one strategy is superior to the other one.
Here, we attempt to model the metabolism of GLSs in insect herbivores to determine the theoretical exposure to ITCs during preemptive vs. direct detoxification. Mathematical modeling using ordinary differential equations (ODEs) helps to understand the change in substrate concentration (plant defense compounds in our case) over time (Knoke et al., 2009; Johnson and Goody, 2011; Schuster et al., 2019; Srinivasan, 2022). Moreover, food intake by organisms can also be modeled by ODEs (Dawes and Souza, 2013). Systems of ODEs are widely used in mathematical biology because they are well-suited to describe dynamical processes provided that the variables are (approximately) continuous (Kot, 2001; Murray, 2002). By developing two different ODE models for the two different types of counter-defenses, we simulate the dynamics of ITC concentrations in the insect gut. Using the concept of the area under the curve (Wagner et al., 1985; Schuster et al., 2019), we quantify the total exposure to ITCs. We analyze under which conditions preemptive counter-defense is superior to direct counter-defense. Then, we check, based on empirical data, whether these conditions are fulfilled. Our models also help to explain how both counter-defenses may entirely degrade the host plant defense.
We develop two different deterministic models, one for preemptive counter-defense and the other for direct counter-defense. For the model formulation, we assume ingestion and degradation of plant GLSs (by insects) are simultaneous processes because free GLSs are usually not found in the insect gut (Schramm et al., 2012; Jeschke et al., 2016; Jeschke et al., 2017).
In the case of insects with a preemptive detoxification system, let α be the rate constant of plant GLS degradation by the preemptive detoxification enzyme and β the rate constant of ITC formation by the hydrolysis of plant GLSs that escape preemptive detoxification. Further, the free ITCs in the insect gut are released in the feces with a rate constant, γ. Based on mass-action kinetics, the rate equations are as follows:
where S is the plant GLS concentration and TP is the ITC concentration at time t for insects with preemptive counter-defense. Model Eq. 1 has an equilibrium point (S∗, TP∗) equal to (0, 0), which is asymptotically stable (a stable node). Therefore, without a doubt, the preemptive counter-defense can degrade the ITC concentration to 0. Since model Eq. 1 is a simple linear ODE system, the equations can be solved analytically:
The time-course of model Eq. 1 is shown in Figure 2A. It can be seen that there is an initial increase and then a decrease in the ITC concentration.
In the case of insects with a direct detoxification system, let δ be the rate constant at which plant GLSs are hydrolyzed to ITCs by myrosinase, µ the rate constant at which ITCs are reacting to produce ITC conjugates, and γ the rate constant of releasing ITCs in the feces. Eventually, the active portion of ITCs is decreased with an overall rate constant µ + γ. The rate equations are as follows:
where S has been defined before and TD refers to the ITC concentration at time t for direct counter-defense. The only equilibrium point of model Eq. 3 is (0, 0), which is also asymptotically stable. Similar to the preemptive counter-defense, direct counter-defense can also degrade the ITC concentration to 0. The time course of model Eq. 3 is shown in Figure 2B. Due to its simplicity, model Eq. 3 can also be solved analytically:
Figure 2 Degradation of plant glucosinolate (GLS) and isothiocyanate (ITC) exposure. Parameter values were chosen in accordance with Inequality 7. (A) In the case of preemptive counter-defense, as computed by model Eq. 1, the parameters are as follows: S0 = 100, α = 0.2, β = 0.1, and γ = 0.75. (B) In the case of direct counter-defense, obtained from model Eq. 3, the parameters are as follows: S0 = 100, δ = 0.3, µ = 0.1, and γ = 0.75.
We assumed that the excretion is independent of the type of counter-defense and similar for all insects. Therefore, the rate constant γ is the same for both preemptive and direct detoxification systems in model Eqs 1 and 3.
Note 1. Since the equilibrium points of both models (Eqs 1 and 3) are stable nodes (0, 0), they are reached for t → ∞. The proof is given below:
Note that both the exponential functions tend to zero as t∗ → ∞. A similar proof can be given for model Eq. 3 by equating (S(t∗), TD(t∗)) = (0, 0).
Using Haber’s rule in both model Eqs 1 and 3, we integrate TP and TD with respect to time (t) within the time range 0 to ∞. The reason for taking the upper limit of the integral ∞ is explained in Note 1. The definite integral gives the area enclosed by the ITC curves (TP and TD), called the area under the curve (AUC) (Wagner et al., 1985; Lappin et al., 2006; Connell et al., 2016; Schuster et al., 2019). Literally, AUC gives the entire amount of ITCs that the feeding insects are exposed to during the period of herbivory, as shown in Figure 3. The AUC is often used in pharmacology (Lappin et al., 2006) and toxicology (Connell et al., 2016) to quantify the total dose of a drug or toxin that an organism is exposed to over time.
Figure 3 Area enclosed by isothiocyanate (ITC) concentrations during the herbivory period for preemptive and direct counter-defenses, obtained from model Eq. 1 and model Eq. 3, respectively. Parameter values are the same as in Figure 2.
Let AUCP and AUCD be the ITC exposure of the insect population with preemptive and direct counter-defense, respectively. Integrating Eqs 2b and 4b, we obtain the following:
It is worth noting that the parameter δ does not appear in the formula for AUCD.
To make a comparison of the two types of counter-defenses under equal situations, we assumed that insects with preemptive and direct detoxification systems feed on plants or patches of plants that are identical in GLS concentration. That is why S0 and S are the same for both model Eqs 1 and 3. By comparing the ITC exposure, Eqs 5a and 5b, it is proved that AUCP < AUCD is enough to explain why the negative effects of ITCs are higher in insects with direct rather than preemptive detoxification. However, we have to investigate whether or not the inequality AUCP < AUCD is always true or conditional (i.e., depends on the parameters).
Hence, to verify,
By considering the reciprocals, we can simplify Inequality 6 to the following:
From the data shown in Table 1, we can establish relationships among the parameters in Inequality 7.
Property 1. For an insect with a preemptive detoxification system, only a small amount of GLSs escape to form ITCs, whereas most of the GLSs are detoxified preemptively, as shown in Table 1. The mean percentages of products were determined by gas chromatography–mass spectrometry (GC-MS) analysis (Wittstock et al., 2004), liquid chromatography–mass spectrometry (LC-MS) analysis, and direct radioactivity measurement (Jeschke et al., 2017). Thus, we obtain β < α.
Property 2. In direct detoxification, the major portion of free ITCs is excreted unmetabolized, whereas a minor portion is converted to non-toxic conjugates, as shown in Table 1. The mean percentages of products were measured by LC-MS analysis and flux measurements with radioactive labeling (Jeschke et al., 2016; Jeschke et al., 2017). Hence, we obtain µ < γ.
Statement 1. AUCP < AUCD or Inequality 6 is always true if the parameters fulfill Properties 1 and 2.
Proof. Since µ < γ (Prop. 2), the right-hand side (r.h.s.) of Inequality 7 is >1. However, the left-hand side (l.h.s.) of Inequality 7 is <1 because β < α (Prop. 1). Therefore, Inequality 6 is obvious for empirical studies, meaning preemptive detoxification performs always better; see Figure 3.
In Figure 2, the parameter values were chosen in accordance with Inequality 7. It can be seen that the ITC concentration is lower for preemptive counter-defense than for direct counter-defense in the entire time course.
Remark 1. If preemptive detoxification is too rapid, i.e., , then AUCP → 0; otherwise, not. Therefore, insects may not escape ITC exposure entirely by preemptive counter-defense.
It is of interest to find out under which conditions direct counter-defense would be superior. That is the case whenever the opposite order relation in Inequality 7 holds. Thus, inequality is the relevant criterion. For example, Inequality 7 is not satisfied if β > α (ITC formation is faster than preemptive GLS degradation) and µ > γ (ITC inactivation is faster than ITC excretion) because the l.h.s. of Inequality 7 then is >1, whereas the r.h.s. is <1; see Figure 4. Note that ITC excretion (rate constant γ) occurs in both types of counter-defenses but that low γ values are especially disadvantageous for the preemptive type.
Figure 4 Direct counter-defense performs better than preemptive counter-defense if β > α and µ > γ, with the following parameters: α = 0.05, β = 0.25, γ = 0.4, δ = 0.3, and µ = 0.45.
Remark 2. We did not make a direct comparison between the dynamic ITC concentrations TP and TD because to verify whether or not TD − TP > 0, we need to establish relations among the parameters α, β, and δ. It can be assumed that δ < α + β because insects with direct counter-defense feed slowly on toxic hosts (Jeschke et al., 2021; Zalucki et al., 2021). However, we have to be more specific to make such parameter comparisons. Fortunately, AUCD in Eq. 5b is free of δ. Therefore, we do not require a relation between α, β, and δ to compare the quantified toxin exposures AUCP and AUCD (Eq. 6).
By our model, we were able to generate time curves for the concentrations of activated toxins. It would be ideal to validate the model by comparing these curves with experimental data. However, no time-resolved data about ITC concentrations are available in the literature. Nevertheless, the model is useful for better understanding the different types of defense and counter-defense and for making testable predictions.
Insect herbivores employ two different strategies to detoxify activated plant defenses like GLSs: preemptive and direct detoxification. Our work shows that a preemptive counter-defense always outcompetes a direct counter-defense if Inequality 7 among the rate constants of plant GLS degradation by the preemptive detoxification enzyme (α), rate constant of ITC formation by the hydrolysis of plant GLSs (β), rate constant of releasing ITCs in the feces (γ), and rate constant of production of ITC conjugates (µ) is fulfilled. Empirical data suggest that Inequality 7 is always satisfied. Theoretically, if the order relation opposite to Inequality 7 holds, a direct counter-defense would outcompete preemptive detoxification, but we are unaware of such cases.
We built our model on the basis of experimental data concerning the concentrations of ITCs and products of direct and preemptive counter-defenses (Jeschke et al., 2017). This allowed us to make testable predictions on the time courses of the relevant substances, which can be checked in experimental studies in the future. For the purpose of this paper, notably the comparison between two types of counter-defenses, we do not need such exact, time-resolved data because the (qualitative) order relations are sufficient to reach conclusions about the preemptive vs. direct counter-defense. In future applications, it is desirable to use experimentally determined data such as transcript levels to assign values to the rate constants.
The superiority of preemptive counter-defense in Statement 1 (based on experimental studies) guarantees that herbivores possessing this strategy have an advantage over other herbivores on toxic host plants because they minimize contact with toxins. The toxic effects of ITCs on feeding insects exposed to this toxin (AUCP or AUCD) cause reductions in feeding rate, growth, and survival (Sun et al., 2019; Jeschke et al., 2021; Zalucki et al., 2021). Thus, low ITC exposure (AUCP) implies only minor effects on insect feeding behavior, growth, and mortality (Li et al., 2000; Hopkins et al., 2009; Rohr et al., 2011), whereas a high AUCD value leads to poor feeding behavior, slow growth, and a high mortality rate (Jeschke et al., 2021; Zalucki et al., 2021).
The effectiveness of preemptive detoxification does not necessarily mean that insects employing this strategy completely escape the adverse effects of ITCs. As described in Remark 1, negative effects occur as long as β < α does not hold. That could explain why some experimental studies report that insect species known to be preemptive detoxifiers of GLSs are affected by ITCs (Mewis et al., 2005; Mewis et al., 2006; Gols et al., 2007; Gols et al., 2008). For the preemptively detoxifying P. xylostella, larvae feeding on plants without any GLSs at all perform significantly better than those on GLS-containing plants, suggesting that some exposure to ITCs occurs despite an effective detoxification strategy (Sun et al., 2019). However, preemptive detoxification has also been documented to be very effective, with many studies reporting that species with this strategy are only marginally affected by the GLS–myrosinase defense system of their host plants (Slansky and Feeny, 1977; Blau et al., 1978; Broadway, 1995; Li et al., 2000; Sarosh et al., 2010; Rohr et al., 2011). In such cases, β is likely to be much less than α.
Our results may also apply to insects that sequester GLSs in their own defense, as these are also reported to avoid the negative effects of ITCs (Müller and Sieling, 2006; Müller, 2009; Beran et al., 2019; Sporer et al., 2021). This phenomenon is explainable from model Eq. 1 by assuming α to be the absorption or sequestration rate of GLSs, where β remains the rate of GLS hydrolysis. In fact, quick sequestration certainly leads to the situation β < α, a conclusion supported experimentally by the rapid absorption of GLSs measured in insect guts of sequestering herbivores (Abdalsamee et al., 2014; Petschenka and Agrawal, 2016; Sporer et al., 2021).
In natural systems, many plants of the Brassicaceae that produce GLSs constitutively have also been found to accumulate higher concentrations after herbivore damage (van Dam et al., 1993; Agrawal, 1998; Textor and Gershenzon, 2009). Experimental studies report that such GLS induction has noticeable adverse effects on insect herbivores (Agrawal, 2000; Agrawal and Kurashige, 2003) (van Dam et al., 2000). In our model Eq. 1 or 3, we considered an immediate induction of GLSs in plants, resulting in an increased initial plant GLS concentration (S0). This assumption is relevant because plants increase GLS levels to a certain high limit as soon as they recognize the attack by insect herbivores (Textor and Gershenzon, 2009). The immediate induction of GLSs causes a drastic increase in ITC exposure (i.e., AUCP and AUCD) due to high S0 value, which raises the toxic effect of ITCs on the herbivores.
The lower exposure to ITCs in preemptive detoxification (AUCP) versus direct detoxification (AUCD) may have an empirical basis due to the location of these reactions in the insect. The preemptive detoxification reactions of GLSs, such as desulfation, are known to occur extracellularly in the insect gut lumen by acting on GLSs in the plant tissue being digested (Sun et al., 2019). In contrast, once ITCs are formed by GLS breakdown in the gut, the direct detoxification reaction, conjugation with glutathione, occurs intracellularly. The ITCs formed thus need to cross through a membrane and enter a cell before being detoxified (Jeschke et al., 2016). This longer path to the site of detoxification in direct counter-defense allows more opportunities for the ITCs to react with target sites than in preemptive detoxification.
Our study adds to experimental results indicating that herbivore feeding on GLS-containing plants can be costly, even those with preemptive detoxification. Thus, it may seem puzzling that specialist herbivores with such detoxification systems use plant GLS or ITC content as a cue for their oviposition and feeding preference (Mewis et al., 2002; Renwick, 2002; Miles et al., 2005; Badenes-Perez et al., 2020) and thus prefer GLS-containing plants compared to plants without GLS despite the costs. A possible explanation is the reduced competition enjoyed on GLS-containing plants because of their generally toxic nature to most herbivores. From an evolutionary perspective, feeding on plants with GLSs or other toxins must benefit herbivores. Otherwise, the evolutionary origin of detoxification traits (Darwin, 1859; Dobzhansky, 1968) is hard to understand. Comparative fitness studies on toxic vs. non-toxic plants, both with and without competition, may help explain the shift to toxic plants.
Our major objective was to compare the two different counter-defenses of insect herbivores against chemical defenses in Brassicaceae plants. Employing a minimal, analytical model for better comprehension and tractability, we showed that preemptive counter-defense is more effective than direct counter-defense in light of the assumptions, based on Table 1. The model can be extended to accommodate more complexity in defense and counter-defense mechanisms. There is no a priori reason why herbivores could not possess both strategies, except for the potentially high metabolic costs. It is worth considering that case in future extensions of the model.
Although preemptive detoxification is only a useful strategy if the host plant(s) stores an inactive toxin precursor of the toxins that can be enzymatically activated, this situation is found not only for the GLSs of the Brassicaceae but also for cyanogenic glycosides, found commonly in the Rosaceae, Passifloraceae, Leguminosae, Sapindaceae, and Poaceae plant families, which can be hydrolyzed to toxic hydrogen cyanide (HCN) by enzyme catalysis, when the plant is damaged or under attack by herbivores (Vickery, 2010). In addition, various Poaceae families produce benzoxazinoid glucosides, which are also activated by hydrolysis upon plant damage (Vassão et al., 2018); the legume plant Medicago truncatula has a two-component defense system of β-glucosidase and triterpene saponins, which can be activated by damage or herbivory (Lacchini et al., 2023). Thus, our models may be valuable to understand and compare the counter-defense mechanisms used by insect herbivores of many plant species.
The original contributions presented in the study are included in the article. Further inquiries can be directed to the corresponding author.
SC established the mathematical model and performed the simulations. JG and SS coordinated and supervised the study. JG contributed expertise in chemical ecology. SS contributed expertise in mathematical modeling. All authors verified the results and wrote this manuscript. All authors contributed to the article and approved the submitted version.
We gratefully acknowledge support by the German Research Foundation (DFG) project no. 512648189 and SFB 1127 ChemBioSys project no. 239748522 as well as by the Open Access Publication Fund of the Thueringer Universitaetsund Landesbibliothek Jena.
We thank Jan Ewald, Christian Kost, and Daniel G. Vassão for the stimulating discussions.
The authors declare that the research was conducted in the absence of any commercial or financial relationships that could be construed as a potential conflict of interest.
All claims expressed in this article are solely those of the authors and do not necessarily represent those of their affiliated organizations, or those of the publisher, the editors and the reviewers. Any product that may be evaluated in this article, or claim that may be made by its manufacturer, is not guaranteed or endorsed by the publisher.
Abdalsamee M., Giampà M., Niehaus K., Müller C. (2014). Rapid incorporation of glucosinolates as a strategy used by a herbivore to prevent activation by myrosinases. Insect Biochem. Mol. Biol. 52, 115–123. doi: 10.1016/j.ibmb.2014.07.002
Agrawal A. (1998). Induced responses to herbivory and increased plant performance. Science 279, 1201–1202. doi: 10.1126/science.279.5354.1201
Agrawal A. (2000). Specificity of induced resistance in wild radish: causes and consequences for two specialist and two generalist caterpillars. Oikos 89, 493–500. doi: 10.1034/j.1600-0706.2000.890308.x
Agrawal A., Kurashige N. (2003). A role for isothiocyanates in plant resistance against the specialist herbivore Pieris rapae. J. Chem. Ecol. 29, 1403–1415. doi: 10.1023/A:1024265420375
Badenes-Perez F., Gershenzon J., Heckel D. (2020). Plant glucosinolate content increases susceptibility to diamondback moth (Lepidoptera: Plutellidae) regardless of its diet. J. Pest Sci. 93, 491–506. doi: 10.1007/s10340-019-01139-z
Beran F., Köllner T., Gershenzon J., Tholl D. (2019). Chemical convergence between plants and insects: biosynthetic origins and functions of common secondary metabolites. New Phytol. 223, 52–67. doi: 10.1111/nph.15718
Blau P., Feeny P., Contardo L., Robson D. (1978). Allylglucosinolate and herbivorous caterpillars: A contrast in toxicity and tolerance. Science 200, 1296–1298. doi: 10.1126/science.200.4347.1296
Broadway R. (1995). Are insects resistant to plant proteinase inhibitors? J. Insect Physiol. 41, 107–116. doi: 10.1016/0022-1910(94)00101-L
Connell D., Yu Q. J., Verma V. (2016). Influence of exposure time on toxicity-an overview. Toxicology 355-356, 49–53. doi: 10.1016/j.tox.2016.05.015
Dawes J., Souza M. (2013). A derivation of Holling’s type I, II and III functional responses in predator–prey systems. J. Theor. Biol. 327, 11–22. doi: 10.1016/j.jtbi.2013.02.017
Dobzhansky T. (1968). “On some fundamental concepts of darwinian biology,” in Evolutionary biology, vol. 2 . Eds. Dobzhansky T., Hecht M. K., Steere, W.C. (Boston, MA: Springer), 1–34. doi: 10.1007/978-1-4684-8094-81
Gols R., Raaijmakers C., van Dam N., Dicke M., Bukovinszky T., Harvey J. A. (2007). Temporal changes affect plant chemistry and tritrophic interactions. Basic Appl. Ecol. 8, 421–433. doi: 10.1016/j.baae.2006.09.005
Gols R., Wagenaar R., Bukovinszky T., van Dam N., Dicke M., Bullock J., et al. (2008). Genetic variation in defense chemistry in wild cabbages affects herbivores and their endoparasitoids. Ecology 89, 1616–1626. doi: 10.1890/07-0873.1
Halkier B., Gershenzon J. (2006). Biology and biochemistry of glucosinolates. Annu. Rev. Plant Biol. 57, 303–333. doi: 10.1146/annurev.arplant.57.032905.105228
Hopkins R., van Dam N., van Loon J. (2009). Role of glucosinolates in insect plant relationships and multitrophic interactions. Annu. Rev. Entomol 54, 57–83. doi: 10.1146/annurev.ento.54.110807.090623
Jeschke V., Gershenzon J., Vassão D. G. (2016). A mode of action of glucosinolate-derived isothiocyanates: Detoxification depletes glutathione and cysteine levels with ramifications on protein metabolism in Spodoptera littoralis. Insect Biochem. Mol. Biol. 71, 37–48. doi: 10.1016/j.ibmb.2016.02.002
Jeschke V., Kearney E. E., Schramm K., Kunert G., Shekhov A., Gershenzon J., et al. (2017). How glucosinolates affect generalist Lepidopteran larvae: Growth, development and glucosinolate metabolism. Front. Plant Sci. 8. doi: 10.3389/fpls.2017.01995
Jeschke V., Zalucki J., Raguschke B., Gershenzon J., Heckel D., Zalucki M., et al. (2021). So much for glucosinolates: A generalist does survive and develop on Brassicas, but at what cost? Plants 10, 962. doi: 10.3390/plants10050962
Johnson K., Goody R. (2011). The original Michaelis constant: Translation of the 1913 Michaelis–Menten paper. Biochemistry 50, 8264–8269. doi: 10.1021/bi201284u
Knoke B., Textor S., Gershenzon J., Schuster S. (2009). Mathematical modelling of aliphatic glucosinolate chain length distribution in Arabidopsis thaliana leaves. Phytochem. Rev. 8, 39–51. doi: 10.1007/s11101-008-9107-3
Kot M. (2001). Elements of mathematical ecology (Cambridge: Cambridge University Press). doi: 10.1017/CBO9780511608520
Lacchini E., Erffelinck M.-L., Mertens J., Marcou S., Molina-Hidalgo F. J., Tzfadia O., et al. (2023). The saponin bomb: a nucleolar-localized β-glucosidase hydrolyzes triterpene saponins in Medicago truncatula. New Phytol. 239, 705–719. doi: 10.1111/nph.18763
Lappin G., Rowland M., Garner R. C. (2006). The use of isotopes in the determination of absolute bioavailability of drugs in humans. Expert Opin. Drug Metab. Toxicol. 2, 419–427. doi: 10.1517/17425255.2.3.419
Li Q., Eigenbrode S., Stringam G., Thiagarajah M. (2000). Feeding and growth of Plutella xylostella and Spodoptera eridania on Brassica juncea with varying glucosinolate concentrations and myrosinase activities. J. Chem. Ecol. 26, 2401–2419. doi: 10.1023/A:1005535129399
Mewis I., Appel H., Hom A., Raina R., Schultz J. (2005). Major signaling pathways modulate Arabidopsis glucosinolate accumulation and response to both phloem-feeding and chewing insects. Plant Physiol. 138, 1149–1162. doi: 10.1104/pp.104.053389
Mewis I., Tokuhisa J., Schultz J., Appel H., Ulrichs C., Gershenzon J. (2006). Gene expression and glucosinolate accumulation in Arabidopsis thaliana in response to generalist and specialist herbivores of different feeding guilds and the role of defense signaling pathways. Phytochemistry 67, 2450–2462. doi: 10.1016/j.phytochem.2006.09.004
Mewis I., Ulrich C., Schnitzler W. (2002). The role of glucosinolates and their hydrolysis products in oviposition and host-plant finding by cabbage webworm, Hellula undalis. Entomol Exp. Appl. 105, 129–139. doi: 10.1046/j.1570-7458.2002.01041.x
Miles C., del Campo M., Renwick J. A. (2005). Behavioral and chemosensory responses to a host recognition cue by larvae of Pieris rapae. J. Comp. Physiol. A Neuroethol Sens Neural Behav. Physiol. 191, 147–155. doi: 10.1007/s00359-004-0580-x
Müller C. (2009). Interactions between glucosinolate- and myrosinase-containing plants and the sawfly Athalia rosae. Phytochem. Rev. 8, 121–134. doi: 10.1007/s11101-008-9115-3
Müller C., Agerbirk N., Olsen C., Boevé J.-L., Schaffner U., Brakefield P. (2001). Sequestration of host plant glucosinolates in the defensive hemolymph of the sawfly Athalia rosae. J. Chem. Ecol. 27, 2505–2516. doi: 10.1023/A:1013631616141
Müller C., Sieling N. (2006). Effects of glucosinolate and myrosinase levels in Brassica juncea on a glucosinolate-sequestering herbivore – and vice versa. Chemoecology 16, 191–201. doi: 10.1007/s00049-006-0347-7
Murray J. (2002). Mathematical biology I. An introduction. 3rd edition (New York: Interdisciplinary Applied Mathematics Springer). doi: 10.1007/b98868
Petschenka G., Agrawal A. (2016). How herbivores coopt plant defenses: natural selection, specialization, and sequestration. Curr. Opin. Insect Sci. 14, 17–24. doi: 10.1016/j
Ratzka A., Vogel H., Kliebenstein D., Mitchell-Olds T., Kroymann J. (2002). Disarming the mustard oil bomb. Proc. Natl. Acad. Sci. 99, 11223–11228. doi: 10.1073/pnas.172112899
Renwick J. (2002). The chemical world of crucivores: lures, treats and traps. Entomol Exp. Appl. 104, 35–42. doi: 10.1046/j.1570-7458.2002.00988.x
Rohr F., Ulrichs C., Schreiner M., Nguyen C., Mewis I. (2011). Impact of hydroxylated and non-hydroxylated aliphatic glucosinolates in Arabidopsis thaliana crosses on plant resistance against a generalist and a specialist herbivore. Chemoecology 21, 171–180. doi: 10.1007/s00049-011-0082-6
Sarosh B., Wittstock U., Halkier B., Ekbom B. (2010). The influence of metabolically engineered glucosinolates profiles in Arabidopsis thaliana on Plutella xylostella preference and performance. Chemoecology 20, 1–9. doi: 10.1007/s00049-009-0028-4
Schramm K., Vassão D. G., Reichelt M., Gershenzon J., Wittstock U. (2012). Metabolism of glucosinolate-derived isothiocyanates to glutathione conjugates in generalist Lepidopteran herbivores. Insect Biochem. Mol. Biol. 42, 174–182. doi: 10.1016/j.ibmb.2011.12.002
Schuster S., Ewald J., Dandekar T., Dühring S. (2019). Optimizing defence, counterdefence and counter-counter defence in parasitic and trophic interactions - a modelling study. arXiv:1907.04820. doi: 10.48550/arXiv.1907.04820
Slansky J. F., Feeny P. (1977). Stabilization of the rate of nitrogen accumulation by larvae of the cabbage butterfly on wild and cultivated food plants. Ecol. Monogr. 47, 209–228. doi: 10.2307/1942617
Sporer T., Körnig J., Wielsch N., Gebauer-Jung S., Reichelt M., Hupfer Y., et al. (2021). Hijacking the mustard-oil bomb: How a glucosinolate-sequestering flea beetle copes with plant myrosinases. Front. Plant Sci. 12. doi: 10.3389/fpls.2021.645030
Srinivasan B. (2022). A guide to the Michaelis–Menten equation: steady state and beyond. FEBS J. 289, 6086–6098. doi: 10.1111/febs.16124
Sun R., Jiang X., Reichelt M., Gershenzon J., Pandit S., Vassão D. (2019). Tritrophic metabolism of plant chemical defenses and its effects on herbivore and predator performance. eLife 8, e51029. doi: 10.7554/eLife.51029
Textor S., Gershenzon J. (2009). Herbivore induction of the glucosinolate–myrosinase defense system: major trends, biochemical bases and ecological significance. Phytochem. Rev. 8, 149–170. doi: 10.1007/s11101-008-9117-1
van Dam N., Hadwich K., Baldwin I. (2000). Induced responses in Nicotiana attenuata affect behavior and growth of the specialist herbivore Manduca sexta. Oecologia 122, 371–379. doi: 10.1007/s004420050043
van Dam N., van der Meijden E., Verpoorte R. (1993). Induced responses in three alkaloid-containing plant species. Oecologia 95, 425–430. doi: 10.1007/bf00320998
Vassão D., Wielsch N., Gomes A., Gebauer-Jung S., Hupfer Y., Svatos A., et al. (2018). Plant defensive β-glucosidases resist digestion and sustain activity in the gut of a Lepidopteran herbivore. Front. Plant Sci. 9. doi: 10.3389/fpls.2018.01389
Vickery M. (2010). Plant poisons: their occurrence, biochemistry and physiological properties. Sci. Prog. 93, 181–221. doi: 10.3184/003685010X12729948220326
Wadleigh R., Yu S. (1988). Detoxification of isothiocyanate allelochemicals by glutathione transferase in three Lepidopterous species. J. Chem. Ecol. 14, 1279–1288. doi: 10.1007/bf01019352
Wagner J., Szpunar G., Ferry J. (1985). Michaelis-Menten elimination kinetics: areas under curves, steady-state concentrations, and clearances for compartment models with different types of input. Biopharmaceut Drug disposition 6, 177–200. doi: 10.1002/bdd.2510060209
Wittstock U., Agerbirk N., Stauber E., Olsen C., Hippler M., Mitchell-Olds T., et al. (2004). Successful herbivore attack due to metabolic diversion of a plant chemical defense. Proc. Natl. Acad. Sci. 101, 4859–4864. doi: 10.1073/pnas.0308007101
Wittstock U., Burow M. (2010). Glucosinolate breakdown in Arabidopsis: mechanism, regulation and biological significance. Arabidopsis Book 8, e0134. doi: 10.1199/tab.0134
Wittstock U., Kliebenstein J., Lambrix V., Reichelt M., Gershenzon J. (2003). Glucosinolate hydrolysis and its impact on generalist and specialist insect herbivores. Recent Adv. Phytochem. 37, 101–125. doi: 10.1016/S0079-9920(03)80020-5
Yang Z., Kunert G., Sporer T., Körnig J., Beran F. (2020). Glucosinolate abundance and composition in brassicaceae influence sequestration in a specialist flea beetle. J. Chem. Ecol. 46, 186–197. doi: 10.1007/s10886-020-01144-y
Yu S. (1987). Microsomal oxidation of allelochemicals in generalist (Spodoptera frugiperda) and semispecialist (Anticarsia gemmatalis) insect. J. Chem. Ecol. 13, 423–436. doi: 10.1007/BF01880090
Zalucki J. M., Heckel D. G., Wang P., Kuwar S., Vassão D. G., Perkins L., et al. (2021). A generalist feeding on brassicaceae: It does not get any better with selection. Plants 10, 954. doi: 10.3390/plants10050954
Keywords: preemptive counter-defense, direct counter-defense, glucosinolates (GLSs), isothiocyanates (ITCs), mathematical model, defense chemicals, plant defense, area under the curve
Citation: Chakraborty S, Gershenzon J and Schuster S (2023) Comparing two strategies of counter-defense against plant toxins: a modeling study on plant–herbivore interactions. Front. Ecol. Evol. 11:1197757. doi: 10.3389/fevo.2023.1197757
Received: 31 March 2023; Accepted: 28 August 2023;
Published: 19 September 2023.
Edited by:
Bradley S. Case, Auckland University of Technology, New ZealandReviewed by:
Julian Chen, Chinese Academy of Agricultural Sciences, ChinaCopyright © 2023 Chakraborty, Gershenzon and Schuster. This is an open-access article distributed under the terms of the Creative Commons Attribution License (CC BY). The use, distribution or reproduction in other forums is permitted, provided the original author(s) and the copyright owner(s) are credited and that the original publication in this journal is cited, in accordance with accepted academic practice. No use, distribution or reproduction is permitted which does not comply with these terms.
*Correspondence: Suman Chakraborty, c3VtYW4uY2hha3JhYm9ydHlAdW5pLWplbmEuZGU=
Disclaimer: All claims expressed in this article are solely those of the authors and do not necessarily represent those of their affiliated organizations, or those of the publisher, the editors and the reviewers. Any product that may be evaluated in this article or claim that may be made by its manufacturer is not guaranteed or endorsed by the publisher.
Research integrity at Frontiers
Learn more about the work of our research integrity team to safeguard the quality of each article we publish.