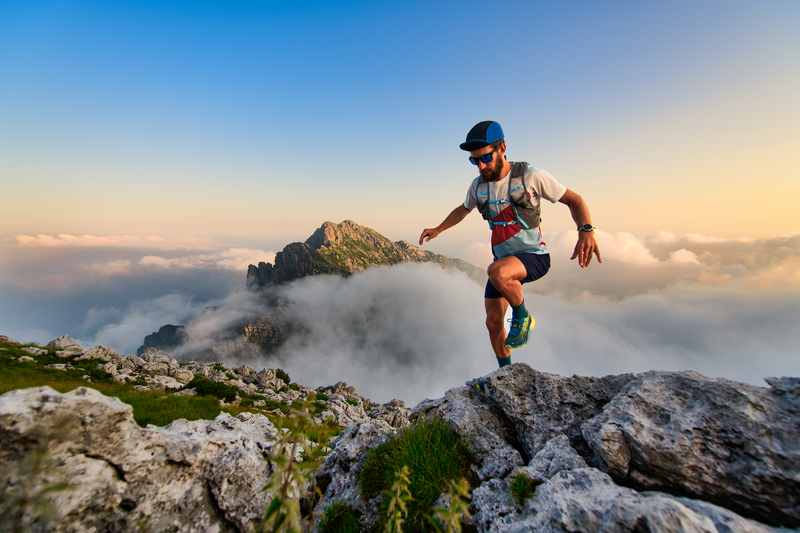
95% of researchers rate our articles as excellent or good
Learn more about the work of our research integrity team to safeguard the quality of each article we publish.
Find out more
REVIEW article
Front. Ecol. Evol. , 01 September 2023
Sec. Behavioral and Evolutionary Ecology
Volume 11 - 2023 | https://doi.org/10.3389/fevo.2023.1180506
This article is part of the Research Topic Adaptations to Subterranean Environments View all 14 articles
The adaptive-shift hypothesis for the evolution of cave-dwelling species posits that ancestor species in surface habitats had exaptations for subterranean life that were exploited when individuals invaded caves. Weakly electric Gymnotiform fishes, nocturnal South American teleost fishes, have features that appear to be likely exaptations for troglobitic life. These fishes have active electrosensory systems in which fish generate weak electric fields that are detected by specialized electroreceptors. Gymnotiform fishes use their electric fields for navigation, prey capture (scene analysis), and social communication. Although active electrosensory systems appear to be exaptations for troglobitic life, as fish use these systems to “see in the dark”, producing electric fields is energetically costly. Cave habitats, which often are low in resources, may not be able to support such high energetic demands. Eigenmannia vicentespelaea, a species of weakly electric fish that is endemic to the São Vicente II cave in central Brazil, surprisingly generates stronger electric fields than their surface relatives. The increase in strength of electric fields may result simply from differences in size between cave and surface populations, but may also be due to lack of predation pressure in the cave or increases in “sensory volumes” and acuity that improve prey localization and capture. Eigenmannia vicentespelaea exhibits the classical phenotypes of any troglobitic fish: these fish have small to nonexistent eyes and loss of pigmentation. The closest living surface relative, Eigenmannia trilineata, inhabits streams nearby and has eyes and pigmentation. The electrosensory and locomotor behavior of both species of fish were measured in their natural habitats using a grid recording system. Surface Eigenmannia exhibited dramatic circadian changes in social behavior, such as hiding under rocks during the day and foraging in groups at night, while cave Eigenmannia displayed territorial behavior with no apparent circadian modulations. The territorial behavior involved electrical and movement-based interactions that may be a form of boundary patrolling. Electrosocial behavior and scene analysis are mechanistically interlinked because both stem from active sensing tactics.
Darwin was once befuddled by the unusual phenotypes of cave organisms. He labeled them “wrecks of ancient life” and remarked “As it is difficult to imagine that eyes, though useless, could in any way be injurious to animals living in darkness, I attribute their loss solely to disuse” (Darwin, 1859). Absit omen, we disagree with Darwin – we would like to contend that cave animals are not a wreck, but simply elegant phenotypes that evolved to retain the most important aspects of life. Troglobites feature unusual and idiosyncratic morphologies that arise from a life history adapted to perpetually dark habitats. They brandish regressive features, such as the loss of eyes and pigmentation, as well as compensatory traits, such as a lower threshold for mechanosensation (Culver et al., 1995; Culver and Pipan, 2009; Haspel et al., 2012; Soares and Niemiller, 2013; Niemiller and Soares, 2015; Soares and Niemiller, 2020).
Cave organisms are recognized as unique model systems for testing evolutionary, ecological, and biogeographic hypotheses (Poulson and White, 1969; Jeffery, 2009; Juan and Emerson, 2010; Juan et al., 2010) and are promising systems to increase our understanding of evolutionary and historical processes that promote or constrain biodiversity (Juan et al., 2010). Despite increased interest and study of troglobites in recent years, subterranean biodiversity remains one of the most poorly understood faunas on the planet. Fishes are routinely prominent members of aquatic cave communities, and currently, over 300 species in 10 orders and 22 families (Proudlove, 2010: Soares and Niemiller, 2013) have been identified. Here we review intriguing electrosocial features of a species of weakly electric fish, Eigenmannia vicentespelaea, which evolved in a single cave system in central Brazil (Figure 1) and that is yet to be thoroughly studied (Bichuette and Trajano, 2006; Bichuette and Trajano, 2017; Fortune et al., 2020).
Figure 1 Habitats are composed of faster-moving rivers with quiet eddies and pools. (A) Surface habitat outside cave Rio de Lapa. Arrows point at the location where Eigenmannia triliniata were found and recorded in Fortune et al. (2020). (B) Cave Sao Vicente II where Eigenmannia vicentespelaea are endemic. Arrows show the recording rig: red floating inflatables maintained the position of the grid in the pool.
There are two contrasting hypotheses for the speciation of subterranean fishes: the climate-relict hypothesis (Holsinger, 1988; Peck and Finston, 1993; Holsinger, 2000) and the adaptive-shift hypothesis (Howarth, 1973; Howarth, 1981; Holsinger, 2000; Niemiller and Soares, 2015). The climate-relict hypothesis suggests that surface ancestors retreated into subterranean habitats in response to climate fluctuations. As surface conditions became more inhospitable, extirpation of surface populations promoted allopatric speciation. In contrast, the adaptive-shift hypothesis suggests that surface ancestors with exaptations to subterranean life (e.g., a nocturnal lifestyle) exploited new subterranean niches that offer reduced competition and quickly evolved in parapatry or sympatry with related surface populations (Juan et al., 2010; Niemiller and Soares, 2015). A key distinction between the climate relict and the adaptive shift hypotheses is whether divergence occurs in the absence (allopatric) or presence (parapatric or sympatric) of gene flow (Niemiller et al., 2008). In both hypotheses, cavefish species are derived from ancestral populations from the surface, whether the original population is extinct or not.
Weakly electric fishes have a suite of features that appear to be obvious exaptations for troglobitic life. Most species of weakly electric fishes are nocturnal, and many live in turbid waters. While in the absence of visual cues, weakly electric fishes use electrosensory feedback from self-generated electric fields in social communication, navigation, predator avoidance, and prey capture (Heiligenberg, 1991; Caputi and Budelli, 2006; Skeels et al., 2023). Such active electrosensory systems are considered to be adaptations for nocturnal lifestyles, and thus may be an exaptation for troglobitic habitats.
Specifically, weakly electric fishes have evolved specialized electric organs for the generation of electric fields in the water and have evolved corresponding electrosensory receptors that detect these fields (Hopkins, 1988; Heiligenberg, 1991; Caputi and Budelli, 2006; Crampton, 2019). In most species, the electric organ is composed of modified epaxial muscle cells, but some species rely on modified spinal axons for the generation of electric fields (Caputi and Budelli, 2006; Shifman et al., 2015; Crampton, 2019; LaPotin et al., 2022). These organs produce transient potential differences between the outside and inside of the fish (Babineau et al., 2006). These potential differences are detected by specialized electroreceptors that have lower resistances than the surrounding skin (Zakon, 1988; Gallant, 2019). Most of the electric current flows across these receptors, which are densely packed in the head of the fish (Heiligenberg and Bastian, 1984; Hopkins, 1988; Zakon, 1988; Nelson and Maciver, 1999). Two categories of signals modulate the flow of current across an individual’s electroreceptors: differences in the local resistance near the fish and the electric fields produced by nearby conspecifics and other organisms (Yu et al., 2012).
The electric fields generated by these fishes are affected by local differences in resistance in the surrounding water (Von der Emde, 1999). vzsavcwbcvSuch local differences can be caused by, for example, the presence of rocks (higher resistance) or other organisms (generally lower resistance) (Fotowat et al., 2013). These differences in resistance affect the flow of current through and voltages occurring at an individual’s electroreceptors, which are encoded by changes in neurophysiological activity (Assad, 1997; Rose, 2004; Fukutomi and Carlson, 2020). In this way, this active electric sense provides a sensory channel for the control of a wide range of behaviors in which the organism interacts with its surroundings (von der Emde, 2006). Indeed, an elegant set of experiments demonstrated how a species of weakly electric fish, Apteronotus albifrons, uses its electrosensory system to capture prey in the absence of visual cues (Bastian, 1981; Nelson and Maciver, 1999; MacIver et al., 2001).
Although these fishes use electric fields to detect nearby objects, evidence from catfish are nevertheless consistent with the hypothesis that the generation of weak electric fields evolved in the context of social signaling (Baron et al, 1994; Orlov et al, 2021). Weakly electric fishes detect the electric fields of nearby conspecifics (Heiligenberg, 1991) and the active electric sense is an important channel for social communication between conspecifics (Henninger, 2015; Caputi, 2017; Crampton, 2019). The ancestors of weakly electric fishes had a category of electroreceptors known as ampullary receptors, which detect low-frequency electric signals in the water. Ampullary receptors can detect, for example, the currents released by muscles that move the operculum (Bodznick and Montgomery, 2005; Grewe et al., 2011), or by the movements of prey items (MacIver et al., 2001).
Variations in muscle cell size (Zakon, 2012), morphology (Mills et al., 1992; Zakon, 1995; Gallant, 2011), and ion channels (Zakon et al., 2006; Gallant et al., 2014; Swapna et al., 2018) in the ancestors of weakly electric fishes may have resulted in increased leakage of current into the water. This increase in current leakage may have made those individuals easier to detect by electroreceptive conspecifics in dark or muddy water, therefore potentially leading to increased reproductive success. Findings from Clarias gariepinus catfish, which generate rudimentary electric fields during social encounters (Baron et al, 1994; Orlov et al, 2021), are consistent with this hypothesis.
This ability to “see in the dark” using self-generated electric fields, however, comes at a cost. The generation of electric fields in water is energetically expensive, consuming up to 30% of an individual’s energy budget (Salazar et al., 2013; Markham et al., 2016). This budget includes the cost of producing the electric field, which involves the cycling of sodium and potassium across cell membranes of electrolytes that comprise the electric organ and the activity of brain areas that both detect the electric fields and control behavioral responses to them.
Another potential cost of generating electric fields is the increased risk of predation by electroreceptive predators. Ampullary electroreceptors are found on many species of fishes that may be predators of weakly electric fishes, including catfish and electric eels. When weakly electric fish produce signals with power at low frequencies, e.g., below about 100 Hz, they may reveal themselves to fish with ampullary electroreceptors. Indeed, electric signals containing low-frequency components that are preferentially detected by ampullary receptors were shown to be more salient to an electric eel than electric signals without these low-frequency components (Electrophorus electricus; Stoddard, 1999; Stoddard, 2002).
Electroreceptive predators of weakly electric fishes appear to be overrepresented in the catfish group (Stoddard, 1999). Pimelodidae, a family of piscivorous catfishes, regularly prey upon weakly electric gymnotiform fishes (Reid, 1983; Duque and Winemiller, 2003; Petrere et al., 2004) in South America and the African sharptooth catfish, Clarias gariepinus has been described as a predator of mormyrid weakly electric fishes (Merron, 1993).
Eigenmannia vicentespelaea from central Brazil is the only known species of gymnotiform weakly electric fish that show complete troglomorphy. The closest epigean species, Eigenmannia trilineata, is found in the same watershed (Bichuette and Trajano, 2006). The cavefish show regression of eyes and loss of pigmentation (Bichuette and Trajano, 2006), while surface fish are pigmented and have functioning eyes (Figure 2). Nevertheless, the surface fish Eigenmannia trilineata and other species of Eigenmannia have been observed to be nocturnal (Tan et al., 2005). Eigenmannia hide in roots, rocks, and leaf litter during the day and actively forage at night (Hopkins, 1974; Hopkins, 1981; Deng and Tseng, 2000; Tan et al., 2005; Stamper et al., 2010; Fortune et al., 2020; Gouveia et al., 2022)
Figure 2 Anatomical differences between ancestral surface and derived cave Eigenmannia. (A) Eigenmannia trilineata collected in Rio da Lapa. (B) DICEuCT scan through the head and mid-body of Eigenmannia trilineata. Note the larger eyes of the surface fish. The arrow points to the electric organ. (C) Eigenmannia vicentespelaea specimen collected in the cave of Sao Vicente II. (D) DICEuCT scan through the head and mid-body of Eigenmannia vicentespelaea. Note small and vestigial eyes and larger electric organs (arrows). Scale bars 10 mm and 2 mm.
We traveled to the São Vicente II cave and measured the electrosensory and locomotor behavior of both surface Eigenmannia trilineata and cave Eigenmannia vicentespelaea (Fortune et al., 2020). We used a custom array of amplified electrodes, 50cm spacing, that both captured the electric behavior of nearby fish, and allowed us to calculate the positions of these fish (Madhav et al., 2018). These grid recording systems have allowed unprecedented insights into the behaviors of weakly electric fishes in their natural habitats (Raab et al., 2019; Henninger et al., 2020).
Generating electric fields is energetically costly, consuming up to 30% of an individual weakly electric fish’s energy budget (Salazar et al., 2013; Markham et al., 2016). Therefore, if a cave environment is poor in resources, one might expect that weakly electric cavefish might produce lower-amplitude electric fields. Indeed, non-electric species of cavefish have adaptations related to the scarcity of resources (Fišer, 2019). On the other hand, active electrosensation permits weakly electric fishes to image their environment (von der Emde, 2004) and capture prey in darkness (MacIver et al., 2001) and therefore might serve as an exaptation for increasing access to food in cave habitats.
We measured the amplitudes of the electric fields (technical details for these measurements are described in Madhav et al., 2018) produced by the weakly electric cavefish in the São Vicente II cave and of surface fish in the nearby Terra Ronca stream (Figure 1). We were surprised to find that the cavefish produced significantly higher amplitude electric fields (Fortune et al., 2020) compared to their closest living surface ancestors (Figure 3). These more powerful electric fields are likely to be energetically costly, but likely provide benefits to the fish. Stronger electric fields may increase the volume of water around the fish in which it can detect objects including prey – known as the “sensing volume” (MacIver et al., 2001; Snyder et al., 2007). A larger sensing volume increases the likelihood of detecting and capturing nearby prey items. Further, stronger electric fields could potentially result in greater spatial acuity and sensitivity.
Figure 3 A comparison of Eigenmannia EOD amplitudes from both habitats shows an increase in the median and mean amplitude in the cavefish (RED line). Also note greater numbers of the strongest EODs in cavefish.
We currently do not know the mechanism for the increase in amplitudes seen in the cavefish. The increase in amplitudes may simply result from size differences between the cave and surface populations of Eigenmannia: larger fish have larger electric organs, resulting in stronger electric fields. Our impression is that the population of adult cavefish we observed was generally larger than the population of adult surface fish. Other mechanisms that might contribute to increased electric field amplitudes of cave Eigenmannia include adaptations of the electric organ, including increases in the relative size of the electric organ in relation to body size, changes in the density or structure of electrocyte ion channels, and/or changes in other structural or resistive features in the skin.
Interestingly, pressure from potentially electroreceptive predators may limit the sizes of surface fish and may also lead to a reduction in the strength of their electric fields. We observed evidence of predation in the surface Eigenmannia population, with several adults having obvious morphological signs of regrowth of their tails (Figure 4). Loss of the tail and its regrowth has been considered evidence of predatory attacks (Dunlap et al., 2016). Our conclusion is that surface Eigenmannia may face significant predation pressure, supporting the adaptive-shift hypothesis for the emergence of cave populations. If so, the relative increase in the electric field amplitudes of the cavefish might result from a release from predation pressure.
Figure 4 Surface Eigenmannia showing predation and regrowth of tail. Notice the lack of black stripe and thickness change starting at the outlining box. Image was taken from a video recording in the field (Fortune et al., 2020).
Finally, the area in which Sao Vicente II cave is located has been classified as rupestrian grassland (Barbosa and Fernandes, 2016). This region was not frozen during the last glacial maximum (~21,000 years ago) and in the middle Holocene (~6,000 years ago), making the climate-relict hypothesis an unlikely explanation of the emergence of cave Eigenmannia.
Surface Eigenmannia exhibit overt diurnal behavioral cycles. During daylight hours, fish exhibit refuge-seeking behavior, hiding in crevices between rocks and root systems. While hiding during the day, surface Eigenmannia are most commonly found in aggregates of several fish (Tan et al., 2005; Fortune et al., 2020). During night hours, surface Eigenmannia emerge from their refugia to forage and perform other behaviors. Videos recorded at night of surface Eigenmannia in the streams outside the Terra Ronca cave show the fish foraging in groups (Supplemental material, Fortune et al., 2020). These video data corroborate the grid tracking data, which shows groups of several fish swimming near each other at night (Fortune et al., 2020). Most surface Eigenmannia were found in groups, and do not appear to maintain territories at any time during 24-hour diurnal cycles (Tan et al., 2005; Fortune et al., 2020).
Unlike surface Eigenmannia, the cave Eigenmannia appeared to occupy territories 24 hours a day. Cave Eigenmannia appeared to perform boundary patrolling behavior on the perimeter of an area of about a square meter (mean = 1.16 sq m, std = 0.76, n = 274). Videos of cave Eigenmannia in the São Vicente II karst show the fish engaging in mouth-to-mount conflicts in which both fish swim toward each other (Supplemental material, Fortune et al., 2020), which may be aggressive interactions between individuals defending their territories. We also observed chases between cavefish in the grid recordings in which the retreating fish produced a long frequency rise of its electric frequency (a rise of typically more than 5 Hz over a period on the order of 10s of seconds). In these interactions, the chasing fish did not change its electric frequency, but the retreating fish initiated a long frequency rise seconds before the fish were at their closest during the interaction (Figure 5). The chasing fish followed the retreating fish for about 10 seconds, at which time the retreating fish began to return its electric field to its normal frequency.
Figure 5 Potential boundary patrolling in Eigenmannia vicentespelaea (data from Fortune et al., 2020). (A) Top panel shows the mean difference (n = 208 events) in electric field frequencies (Hz) between pairs of fish, centered in time on the start of a long-frequency rise produced by one fish in the pair (grey vertical line). Bottom panel shows the average distance between the pairs of fish. Frequency rises were produced as fish approached each other, about 5 seconds before their closest distance. (B) Spatial map of the XY positions of two fish during a possible boundary patrolling event (blue fish). Colors of the lines correspond to (C), and the line is thinnest at the start of the epoch and thickest at the end. Numbers correspond to time points shown in (C) Black dots with arrows indicate the locations of both fish at the initiation of the frequency rise produced by the red fish. (C) Top panel shows the frequencies of both fish over time and bottom panel shows the distance between the fish. In this event, the red fish moved closer to the blue fish. At the moment of the frequency rise produced by the red fish, the blue fish changed direction and rapidly followed the red fish over a distance of well over 2 meters in a period of about 20 seconds. After the event, the blue fish returned to near its original position.
Eye loss is a trait that is associated with troglomorphy. In all animals studied so far, eyes develop normally in embryonic stages, but eventually the lens undergoes cell death which signals the eyes to arrest growth, leading to small eyes sunken into the orbits (Rétaux and Casane, 2013). Interestingly, this mechanism leads to the regression of both eyes. Eigenmannia vicentespelaea exhibits a diversity of eye sizes (Bichuette and Trajano, 2006). These include extreme individuals with a full eye on one side and a completely degenerated eye on the other. This suggests that there might be other evolutionary mechanisms at play in eye degeneration in Eigenmannia vicentespelaea.
The amplitudes of electric fields increase with the size of fish (Hopkins et al., 1990; Curtis and Stoddard, 2003; Gavassa et al., 2012) and can be used as a nonvisual proxy to estimate body size. However, Gavassa et al. (2013) have shown that social interactions can drive significant changes in the amplitude of electric fields. Further, hormones can increase the amplitudes of electric fields (Markham et al., 2009a; Migliaro and Silva, 2016). We already know that on average, the size of the EOD is larger in the cavefish, the next steps will include correlating them to the size of the fish. For example, we predict that the slope of the relationship between size and electric field amplitude will be steeper in the cavefish in comparison to the surface fish. Such differences would indicate active adaptations rather than simply size effects.
Cave Eigenmannia vicentespelaea show boundary patrolling, a form of territorial behavior absent in surface Eigenmannia. Territoriality can provide insights into how fish integrate social and environmental cues to produce behavioral responses. There is a cost-benefit in territorial defense, whether from breeding or resource defense (Kaufmann, 1983; Adams, 2001; Christensen and Radford, 2018). Territorial defense is usually associated with breeding males seasonally, but year-round territoriality can establish access to foraging areas or protection from predators (Silva et al., 2020).
A good example of weakly electric fish territorial behavior is shown by the Gymnotus omarorum. These fish have territory ranges that are independent of sex but are directly correlated to body size (Silva et al., 2020). During the breeding season, however, Silva et al., 2020 showed that Gymnotus omarorum establishes territories, which sizes are correlated to with gonadosomatic index in females and 11-ketotestosterone levels in males. We are interested in the roles of hormones in both regions of the body of the Eigenmannia vicentespelaea and trilineata populations. It will be interesting if expression differences are correlated with a cave habitat and what are the evolutionary forces driving it.
Vertebrate circadian behaviors are based on networks of endogenous clocks across tissues throughout the body of the fish that are synchronized to one another by a master circadian clock in the brain (Ripperger and Schibler, 2001). Master clocks guide physiology and behavior via neural and hormonal cues (Ripperger and Schibler, 2001; Patke et al., 2020).
We know that strongly defined cycles caused by inputs such as light, temperature, and other biological influences such as predation, can synchronize the intrinsic internal clock generated by cryptochrome gene expression. Ripperger and Schibler (2001) demonstrated that the endogenous timekeeping system created by the interaction of these genes can be synchronized by diverse types of zeitgebers (time-givers in German) (Takahashi, 1991; Wilsbacher and Takahashi, 1998).
Surface Eigenmannia modulates the amplitude of their wave-type electric organ discharges (EOD) in relation to the light/dark cycle, with greater EOD amplitude at night when these nocturnal animals are more active and a lower EOD amplitude during the day when fish are hidden within refugia (Deng and Tseng, 2000; Markham et al., 2009b; Sinnett and Markham, 2015). In Brachyhypopomus pinnicaudatus, a pulse-type weakly electric fish, pulse rate, pulse amplitude, and tauP2 – the time constant of recovery for the second phase of the pulse waveform, all increased during the subjective night. These circadian rhythms in electric field waveform were even more pronounced under breeding conditions, suggesting a social context (Silva et al., 2007). Similar to the results we report for surface Eigenmannia, B. pinnicaudatus EOD rhythms were stronger under photo entrained (12 hours of dark:12 hours of light) conditions than under free-running, or constant photic conditions (Stoddard et al., 2007).
Gymnotus omarorum is another pulse-type weakly electric fish with a circadian pattern in electric field pulse rate. These fish live in such dense vegetation in their natural habitat, that they experience no diurnal variation in photic cues, similar to the cave conditions. Because circadian changes in EOD pulse rate persist in G. omarorum when measured in the field along with daily fluctuations in temperature it is postulated that daily temperature fluctuations driven by the sun heating the water during the day, act as the primary zeitgeber in these animals (Migliaro et al., 2018). Indeed, temperature variation has been shown to induce changes in the EOD pulse rate in B. pinnicaudatus (Silva et al., 2007).
The data shown in this review were collected for a previous publication (Fortune et al., 2020). A detailed description of the materials and methods can be found there. In short, the procedures used for these observational studies of Gymnotiform fishes were reviewed and approved by the animal care and use committee of Rutgers University/New Jersey Institute of Technology (Protocol #999900774) and follow guidelines for the use of animals in field research established by the National Research Council. Field research permits in Brazil were granted by the ICMBio and SEMARH/SECIMA.
Both species of fish are endemic to the upper Tocantis river basin in the state of Goias, central Brazil (46° 10′–46° 30′ S, 13° 30′–13° 50′ W). The cavefish Eigenmannia vicentespelaea population is located in the cave of São Vicente II (13° 58′37″ S, 46° 40′04″ W), while Eigenmannia trilineata population is found in the Rio da Lapa (13° 38′44″ S, 46° 38′ 08″ W) approximately 8 km away. Both habitats have moderate water currents, clear water with a conductivity below 20 μS, and substrate composed of sand, rocks, and boulders (Fortune et al., 2020).
We chose to use Diffusible Iodine-based Contrast-enhanced Computed micro-Tomography (DICEuCT) because it permits visualization of soft tissue details without damage to the specimens. We imaged four previously collected specimens from Dr. Bichuette’s laboratory collection at the Universidade Federal de São Carlos, Brazil. Briefly, we submerged 70% ethanol fixed specimens in 11.25 Lugol’s iodine (I2KI) solution for up to 36 h before scanning. Stained specimens were washed in water to remove excess stain and sealed in rubber containers to prevent dehydration. Stained and unstained specimens were scanned at the Core Imaging Facility of the American Museum of Natural History (New York, NY), using a 2010 GE Phoenix v∣tome∣x s240CT high-resolution microfocus computed tomography system (General Electric, Fairfield, CT, USA) in 50 mL polypropylene centrifuge tubes. DScans were made at 125 kV, with an exposure time of 60 seconds. Voxel sizes were 20.0–25.9 μm and volume reconstruction of raw X-ray images was achieved using a GE Phoenix datos∣x.
We used a hand-held single-electrode probe attached to a custom amplifier and speaker to find the fish. When the probe was within about 1 meter of an Eigenmannia, its electric field was audible to the user. Also, we were also able to see the animals directly due to the clarity of the water. To record the electric behavior and movement of Eigenmannia, we deployed a grid (50 cm spacing) of active electrodes (Madhav et al., 2018; Fortune et al., 2020) at sites where we had previously observed fish. The surface fish were recorded along the edges of the Rio da Lapa outside of the Terra Ronca cave after sundown. The cavefish were recorded in eddies and side pools of the primary river within the São Vicente II cave.
We used an algorithm developed by Madhav et al. (2018; code available at doi:10.7281/T1/XTSKOW) to identify each fish via the time-varying fundamental frequency of its electric field. The locations and orientations of each individual were calculated in relation to the distribution of power at each electric field frequency across the electrodes. These recordings were made in shallow water of no more than 40 cm depth: position estimates were restricted to the XY plane. Continuous recording sessions using the grid were made both in the cave (N = 14) and surface (N = 5). Intervals between recording sessions ranged from 5 min to several hours and each recording was from 600 seconds to over 1,200 seconds in duration. The position and electric field frequency data were analyzed in 300 s duration non-overlapping epochs. Other details of the recording procedures and analysis can be found in Fortune et al. (2020).
Our future studies include the identification of the causal evolutionary interactions between categories of behavior: scene analysis and social behavior.
Written informed consent was obtained from the individuals for the publication of any identifiable images or data included in this article.
MB, DS, and EF contributed to the conception and design of the study. DS, KG, and EF wrote the first draft of the manuscript. All authors contributed to the writing and revision of the manuscript and have read and approved the submitted version.
The fieldwork was supported by startup funds provided by the New Jersey Institute of Technology to DS. MB received funding from the CNPq (Awards 308557/2014-0). This work includes materials from work supported by a National Science Foundation (NSF) Award to EF (1557858).
Field research permits were granted by the ICMBio and SEMARH/SECIMA. We would like to thank D. F. Torres, M. J. Rosendo, and C. C. P. de Paula for their help in the field. Thanks to Dr. Jessica Ware for the use of the CT scanner.
The authors declare that the research was conducted in the absence of any commercial or financial relationships that could be construed as a potential conflict of interest.
All claims expressed in this article are solely those of the authors and do not necessarily represent those of their affiliated organizations, or those of the publisher, the editors and the reviewers. Any product that may be evaluated in this article, or claim that may be made by its manufacturer, is not guaranteed or endorsed by the publisher.
Adams E. S. (2001). Approaches to the study of territory size and shape. Annu. Rev. Ecol. Syst 32 (1), 277–303. doi: 10.1146/annurev.ecolsys.32.081501.114034
Assad C. (1997). Electric field maps and boundary element simulations of electrolocation in weakly electric fish. California. Inst. Technol.
Babineau D., Longtin A., Lewis J. E. (2006). Modeling the electric field of weakly electric fish. J. Exp. Biol. 209 (18), 3636–3651. doi: 10.1242/jeb.02403
Barbosa N. P., Fernandes G. W. (2016). Rupestrian grassland: past, present and future distribution. In: Fernandes G. W. (ed) Ecology and conservation of mountaintop grasslands in Brazil. Switzerland: Springer, 531–544. doi: 10.1007/978-3-319-29808-5_22
Baron V. D., Orlov A. A., Golubtsov A. S. (1994). African Clarias catfish elicits long-lasting weak electric pulses. Experientia 50, 644–647. doi: 10.1007/BF01952864
Bastian J. (1981). Electrolocation: I. How the electroreceptors of Apteronotus albifrons code for moving objects and other electrical stimuli. J. Comp. Physiol. 144, 465–479. doi: 10.1007/BF01326832
Bichuette M. E., Trajano E. (2006). Morphology and distribution of the cave knifefish Eigenmannia vicentespelaea Triques 1996 (Gymnotiformes: Sternopygidae) from Central Brazil, with an expanded diagnosis and comments on subterranean evolution. Neotropical. Ichthyol. 4 (1), 99–105. doi: 10.1590/S1679-62252006000100011
Bichuette M. E., Trajano E. (2017). Biology and behavior of Eigenmannia vicentespelaea, a troglobitic electric fish from Brazil (Teleostei: Gymnotiformes: Sternopygidae): a comparison to the epigean species, E. trilineata, and the consequences of cave life. Trop. Zool. 30 2(2017), 68–82. doi: 10.1080/03946975.2017.1301141
Bodznick D., Montgomery J. C. (2005). The physiology of low-frequency electrosensory systems. In: Bullock T. H., Hopkins C. D., Popper A. N., Fay R. R. (eds) Electroreception. New York: Springer, 132–153. doi: 10.1007/0-387-28275-0_6
Caputi A. A. (2017). Active electroreception in weakly electric fish. Bioinspir Biomim. 12 (2), 025004.
Caputi A. A., Budelli R. (2006). Peripheral electrosensory imaging by weakly electric fish. J. Comp. Physiol. A. 192, 587–600. doi: 10.1007/s00359-006-0100-2
Christensen C., Radford A. N. (2018). Dear enemies or nasty neighbors? Causes and consequences of variation in the responses of group-living species to territorial intrusions. Behav. Ecol. 29 (5), 1004–1013. doi: 10.1093/beheco/ary010
Crampton W. G. (2019). Electroreception, electrogenesis and electric signal evolution. J. Fish. Biol. 95 (1), 92–134. doi: 10.1111/jfb.13922
Culver D. C., Kane T. C., Fong D. W. (1995). Adaptation and natural selection in caves: the evolution of Gammarus minus (Harvard University Press).
Culver D. C., Pipan T. (2009). The biology of caves and other subterranean habitats (Oxford: Oxford University Press).
Curtis C. C., Stoddard P. K. (2003). Mate preference in female electric fish, Brachyhypopomus pinnicaudatus. Anim. Behav. 66 (2), 329–336. doi: 10.1006/anbe.2003.2216
Darwin C. (1859). On the origin of species by means of natural selection, or, The preservation of favoured races in the struggle for life. London: J. Murray.
Deng T. S., Tseng T. C. (2000). Evidence of circadian rhythm of electric discharge in Eigenmannia virescens system. Chronobiol. Int. 17 (1), 43–48. doi: 10.1081/CBI-100101030
Dunlap K. D., Tran A., Ragazzi M. A., Krahe R., Salazar V. L. (2016). Predators inhibit brain cell proliferation in natural populations of electric fish, Brachyhypopomus occidentalis. Proc. R. Soc. B.: Biol. Sci. 283 (1824), 20152113.
Duque A. B., Winemiller K. O. (2003). Dietary segregation among large catfishes of the Apure and Arauca Rivers, Venezuela. J. Fish. Biol. 63, 410–427. doi: 10.1046/j.1095-8649.2003.00163.x
Fišer Ž. (2019). “Chapter 1 - Adaptation to low food,” in Encyclopedia of Caves (Third Edition). Eds. White W. B., Culver D. C., Pipan T. (Academic Press), 1–7.
Fortune E. S., Andanar N., Madhav M., Jayakumar R. P., Cowan N. J., Bichuette M. E., et al. (2020). Spooky interaction at a distance in cave and surface dwelling electric fishes. Front. Integr. Neurosci. 14, 561524. doi: 10.3389/fnint.2020.561524
Fotowat H., Harrison R. R., Krahe R. (2013). Statistics of the electrosensory input in the freely swimming weakly electric fish Apteronotus leptorhynchus. J. Neurosci. 33 (34), 13758–13772. doi: 10.1523/JNEUROSCI.0998-13.2013
Fukutomi M., Carlson B. A. (2020). A history of corollary discharge: contributions of mormyrid weakly electric fish. Front. Integr. Neurosci. 14, 42. doi: 10.3389/fnint.2020.00042
Gallant J. R. (2019). “The evolution and development of electric organs,” in Electroreception: fundamental insights from comparative approaches. (New York: Springer-Verlag), 91–123.
Gallant J (2011) Mechanisms of signal diversity in Mormyrid electric fish. PhD thesis, Cornell University.
Gallant J. R., Traeger L. L., Volkening J. D., Moffett H., Chen P. H., Novina C. D., et al. (2014). Genomic basis for the convergent evolution of electric organs. Science 344 (6191), 1522–1525.
Gavassa S., Silva A. C., Gonzalez E., Stoddard P. K. (2012). Signal modulation as a mechanism for handicap disposal. Anim. Behav. 83 (4), 935–944. doi: 10.1016/j.anbehav.2012.01.012
Gavassa S., Roach J. P., Stoddard P. K. (2013). Social regulation of electric signal plasticity in male Brachyhypopomus gauderio. J. Comp. Physiol. A 199, 375–384.
Gouveia É.J., Rondon P. L., Súarez Y. R. (2022). Feeding ecology of Eigenmannia desantanai (Gymnotiformes: Sternopygidae) in southern Pantanal, Brazil. Acta Limnol. Brasiliensia. 34. doi: 10.1590/s2179-975x9820
Grewe J., Walz H., Benda J. (2011). Coding of electrical signals in different subsystems of the electrosensory system of the weakly electric fish. In Front. Comput. Neurosci. Conference Abstract: BC11: Computational Neuroscience & Neurotechnology Bernstein Conference & Neurex Annual Meeting.
Haspel G., Schwartz A., Streets A., Camacho D. E. (2012). By the teeth of their skin, cavefish find their way. Current Biology 22 (16), R629–R630.
Heiligenberg W., Bastian J. (1984). The electric sense of weakly electric fish. Annu. Rev. Physiol. 46 (1), 561–583.
Henninger J. (2015). Social interactions in natural populations of weakly electric fish (Doctoral dissertation, Universität Tübingen).
Henninger J., Krahe R., Sinz F., Benda J. (2020). Tracking activity patterns of a multispecies community of gymnotiform weakly electric fish in their neotropical habitat without tagging. J. Exp. Biol. 223 (3), jeb206342.
Holsinger J. R. (1988). Troglobites: the evolution of cave-dwelling organisms. Am. Sci. 76 (2), 146–153.
Holsinger J. R. (2000). Ecological derivation, colonization, and speciation. in Wilkens H., Culver D. C., Humphreys W. F. eds. Ecosystems of the world. Oxford: Elsevier Science Ltd., vol. 30, 399–416.
Hopkins C. D. (1974). Electric communication: functions in the social behavior of Eigenmannia virescens. Behaviour 50 (3-4), 270–304. doi: 10.1163/156853974X00499
Hopkins C. D. (1981). On the diversity of electric signals in a community of mormyrid electric fish in West Africa. Am. Zool. 21 (1), 211–222. doi: 10.1093/icb/21.1.211
Hopkins C. D. (1988). Neuroethology of electric communication. Annu. Rev. Neurosci. 11 (1), 497–535. doi: 10.1146/annurev.ne.11.030188.002433
Hopkins C. D., Comfort N. C., Bastian J., Bass A. H. (1990). Functional Analysis of sexual dimorphism in an electric fish, hypopomus pinnicaudatus, order gymnotiformes (Part 1 of 2). Brain. Behav. Evol. 35 (6), 350–358. doi: 10.1159/000115880
Howarth F. G. (1973). The cavernicolous fauna of Hawaiian lava tubes, 1. Introduction. Pac. Insects. 15 (1), 139–151.
Howarth F. G. (1981). Community structure and niche differentiation in Hawaiian lava tubes. US/IBP synthesis series.
Jeffery W. R. (2009). Regressive evolution in Astyanax cavefish. Annu. Rev. Genet. 43, 25–47. doi: 10.1146/annurev-genet-102108-134216
Juan C., Emerson B. C. (2010). Evolution underground: shedding light on the diversification of subterranean insects. J. Biol. 9, 1–5. doi: 10.1186/jbiol227
Juan C., Guzik M. T., Jaume D., Cooper S. J. (2010). Evolution in caves: Darwin’s “wrecks of ancient life” in the molecular era. Mol. Ecol. 19 (18), 3865–3880. doi: 10.1111/j.1365-294X.2010.04759.x
Kaufmann J. H. (1983). On the definitions and functions of dominance and territoriality. Biol. Rev. 58 (1), 1–20. doi: 10.1111/j.1469-185X.1983.tb00379.x
LaPotin S., Swartz M. E., Luecke D. M., Constantinou S. J., Gallant J. R., Eberhart J. K., et al. (2022). Divergent cis-regulatory evolution underlies the convergent loss of sodium channel expression in electric fish. Sci. Adv. 8 (22), eabm2970.
MacIver M. A., Sharabash N., Nelson M. E. (2001). Quantitative analysis of prey capture behavior in the weakly electric fish Apteronotus albifrons. J. Exp. Biol. 204, 543–557. doi: 10.1242/jeb.204.3.543
Madhav M. S., Jayakumar R. P., Demir A., Stamper S. A., Fortune E. S., Cowan N. J. (2018). High-resolution behavioral mapping of electric fishes in Amazonian habitats. Sci. Rep. 8 (1), 5830.
Markham M. R., Allee S. J., Goldina A., Stoddard P. K. (2009a). Melanocortins regulate the electric waveforms of gymnotiform electric fish. Hormones. Behav. 55 (2), 306–313. doi: 10.1016/j.yhbeh.2008.11.002
Markham M. R., Ban Y., McCauley A. G., Maltby R. (2016). Energetics of sensing and communication in electric fish: A blessing and a curse in the anthropocene? Integr. Comp. Biol. 56 (5), 889–900. doi: 10.1093/icb/icw104
Markham M. R., McAnelly M. L., Stoddard P. K., Zakon H. H. (2009b). Circadian and social cues regulate ion channel trafficking. PloS Biol. 7 (9). doi: 10.1371/journal.pbio.1000203
Merron G. S. (1993). Pack-hunting in two species of catfish, Clarias gariepinus and C. ngamensis, in the Okavango Delta, Botswana. J. Fish. Biol. 43, 575–584. doi: 10.1006/jfbi.1993.1160
Migliaro A., Moreno V., Marchal P., Silva A. (2018). Daily changes in the electric behavior of weakly electric fish naturally persist in constant darkness and are socially synchronized. Biol. Open 7 (12).
Migliaro A., Silva A. (2016). Melatonin regulates daily variations in electric behavior arousal in two species of weakly electric fish with different social structures. Brain. Behav. Evol. 87 (4), 232–241. doi: 10.1159/000445494
Mills A., Zakon H. H., Marchaterre M. A., Bass A. H. (1992). Electric organ morphology of Sternopygus macrurus, a wave type, weakly electric fish with a sexually dimorphic EOD. J. Neurobiol. 23 (7), 920–932. doi: 10.1002/neu.480230712
Nelson M. E., Maciver M. A. (1999). Prey capture in the weakly electric fish Apteronotus albifrons: sensory acquisition strategies and electrosensory consequences. J. Exp. Biol. 202 (10), 1195–1203. doi: 10.1242/jeb.202.10.1195
Niemiller M. L., Fitzpatrick B. M., Miller B. T. (2008). Recent divergence with gene flow in Tennessee cave salamanders (Plethodontidae: Gyrinophilus) inferred from gene genealogies. Mol. Ecol. 17 (9), 2258–2275. doi: 10.1111/j.1365-294X.2008.03750.x
Niemiller M. L., Soares D. (2015). “Cave environments.” In: Riesch R., Tobler M., Plath M. (eds) Extremophile Fishes. Springer, 161–191. doi: 10.1007/978-3-319-13362-1_8
Orlov A. A., Olshanskiy V. M., Baron V. D. (2021). “Reconstruction of Electric Discharge Patterns and Electrogenesis Mechanism in African Sharptooth Catfish Clarias gariepinus (Clariidae, Siluriformes),” in Doklady Biological Sciences, vol. 500. (Pleiades Publishing), 145–148.
Patke A., Young M. W., Axelrod S. (2020). Molecular mechanisms and physiological importance of circadian rhythms. Nat. Rev. Mol. Cell Biol. 21 (2), 67–84. doi: 10.1038/s41580-019-0179-2
Peck S. B., Finston T. L. (1993). Galapagos islands troglobites: the questions of tropical troglobites, parapatric distributions with eyed-sister-species, and their origin by parapatric speciation. Mémoires. Biospéol. 20, 19–37.
Petrere M., Barthem R. B., Cordoba E. A., Gomez B. C. (2004). Review of the large catfish fisheries in the upper Amazon and the stock depletion of piraiba (Brachyplatystoma filamentosum Lichtenstein). Rev. Fish. Biol. Fish. 14, 403–414. doi: 10.1007/s11160-004-8362-7
Poulson T. L., White W. B. (1969). The Cave Environment: Limestone caves provide unique natural laboratories for studying biological and geological processes. Science 165 (3897), 971–981.
Proudlove G. S. (2010). Biodiversity and distribution of the subterranean fishes of the world. Biol. Subterranean. Fishes., 41–63. doi: 10.1201/EBK1578086702-c2
Raab T., Linhart L., Wurm A., Benda J. (2019). Dominance in habitat preference and diurnal explorative behavior of the weakly electric fish apteronotus leptorhynchus. Front. Integr. Neurosci. 13, 21.
Reid S. (1983). La biologia de los bagres rayados Pseudoplatystoma fasciatum y P. tigrinum en la cuenca del rio Apure - Venezuela. Rev. UNELLEZ. Ciencia. y. Tecnol. 1, 13–41.
Rétaux S., Casane D. (2013). Evolution of eye development in the darkness of caves: adaptation, drift, or both? EvoDevo 4 (1), 1–12. doi: 10.1186/2041-9139-4-26
Ripperger J. A., Schibler U. (2001). Circadian regulation of gene expression in animals. Curr. Opin. Cell Biol. 13 (3), 357–362. doi: 10.1016/S0955-0674(00)00220-9
Rose G. J. (2004). Insights into neural mechanisms and evolution of behaviour from electric fish. Nat. Rev. Neurosci. 5 (12), 943–951. doi: 10.1038/nrn1558
Salazar V. L., Krahe R., Lewis J. E. (2013). The energetics of electric organ discharge generation in gymnotiform weakly electric fish. J. Exp. Biol. 216 (13), 2459–2468. doi: 10.1242/jeb.082735
Shifman A. R., Longtin A., Lewis J. E. (2015). Ultrafast traveling wave dominates the electric organ discharge of Apteronotus leptorhynchus: an inverse modelling study. Sci. Rep. 5 (1), 15780.
Silva A., Perrone R., Macadar O. (2007). Environmental, seasonal, and social modulations of basal activity in a weakly electric fish. Physiol. Behav. 90 (2-3), 525–536. doi: 10.1016/j.physbeh.2006.11.003
Silva A. C., Zubizarreta L., Quintana L. (2020). A teleost fish model to understand hormonal mechanisms of non-breeding territorial behavior. Front. Endocrinol. 11, 468. doi: 10.3389/fendo.2020.00468
Sinnett P. M., Markham M. R. (2015). Food deprivation reduces and leptin increases the amplitude of an active sensory and communication signal in a weakly electric fish. Hormones. Behav. 71. doi: 10.1016/j.yhbeh.2015.03.010
Skeels S., von der Emde G., Burt de Perera T. (2023). Mormyrid fish as models for investigating sensory-motor integration: A behavioural perspective. J. Zool. 319 (4), 243–253. doi: 10.1111/jzo.13046
Snyder J. B., Nelson M. E., Burdick J. W., MacIver M. A. (2007). Omnidirectional sensory and motor volumes in electric fish. PloS Biol. 5 (11), e301. doi: 10.1371/journal.pbio.0050301
Soares D., Niemiller M. L. (2013). Sensory adaptations of fishes to subterranean environments. BioScience 63 (4), 274–283. doi: 10.1525/bio.2013.63.4.7
Soares D., Niemiller M. L. (2020). Extreme adaptation in caves. Anatomical. Rec. 303 (1), 15–23. doi: 10.1002/ar.24044
Stamper S. A., Carrera-G E., Tan E. W., Fugère V., Krahe R., Fortune E. S. (2010). Species differences in group size and electrosensory interference in weakly electric fishes: implications for electrosensory processing. Behav. Brain Res. 207 (2), 368–376. doi: 10.1016/j.bbr.2009.10.023
Stoddard P. K. (1999). Predation enhances complexity in the evolution of electric fish signals. Nature 400 (6741), 254–256.
Stoddard P. K. (2002). “Electric signals: predation, sex, and environmental constraints,” in Advances in the Study of Behavior, vol. 31. (Academic Press), 201–242.
Stoddard P. K., Markham M. R., Salazar V. L., Allee S. (2007). Circadian rhythms in electric waveform structure and rate in the electric fish Brachyhypopomus pinnicaudatus. Physiol. Behav. 90 (1), 11–20. doi: 10.1016/j.physbeh.2006.08.013
Swapna I., Ghezzi A., York J. M., Markham M. R., Halling D. B., Lu Y., et al. (2018). Electrostatic tuning of a potassium channel in electric fish. Curr. Biol. 28 (13), 2094–2102. doi: 10.1016/j.cub.2018.05.012
Takahashi J. S. (1991). Circadian rhythms: from gene expression to behavior. Curr. Opin. Neurobiol. 1 (4), 556–561. doi: 10.1016/S0959-4388(05)80028-5
Tan E. W., Nizar J. M., Carrera-G E., Fortune E. S. (2005). Electrosensory interference in naturally occurring aggregates of a species of weakly electric fish, Eigenmannia virescens. Behav. Brain Res. 164 (1), 83–92. doi: 10.1016/j.bbr.2005.06.014
Von der Emde G. (1999). Active electrolocation of objects in weakly electric fish. J. Exp. Biol. 2 (10), 1205–1215. doi: 10.1242/jeb.202.10.1205
von der Emde G. (2004). Distance and shape: perception of the 3-dimensional world by weakly electric fish. J. Physiology-Paris. 98 (1-3), 67–80. doi: 10.1016/j.jphysparis.2004.03.013
von der Emde G. (2006). Non-visual environmental imaging and object detection through active electrolocation in weakly electric fish. J. Comp. Physiol. A. 192, 601–612. doi: 10.1007/s00359-006-0096-7
Wilsbacher L. D., Takahashi J. S. (1998). Circadian rhythms: molecular basis of the clock. Curr. Opin. Genet. Dev. 8 (5), 595–602. doi: 10.1016/S0959-437X(98)80017-8
Yu N., Hupé G., Garfinkle C., Lewis J. E., Longtin A. (2012). Coding conspecific identity and motion in the electric sense. PloS Comput. Biol. 8 (7), e1002564. doi: 10.1371/journal.pcbi.1002564
Zakon H. H. (1995). “Electric organs: structure, physiology, hormone-sensitivity, and biochemistry,” in Biochemistry and Molecular Biology of Fishes, vol. 4. (Elsevier), 259–277.
Zakon H. H. (2012). Adaptive evolution of voltage-gated sodium channels: the first 800 million years. Proc. Natl. Acad. Sci. 109 (Supplement 1), 10619–10625. doi: 10.1073/pnas.1201884109
Zakon H. H., Lu Y., Zwickl D. J., Hillis D. M. (2006). Sodium channel genes and the evolution of diversity in communication signals of electric fishes: convergent molecular evolution. Proc. Natl. Acad. Sci. 103 (10), 3675–3680. doi: 10.1073/pnas.0600160103
Keywords: cave, weakly electric fish, evolution, EOD, territory, troglomorphism
Citation: Soares D, Gallman K, Bichuette ME and Fortune ES (2023) Adaptive shift of active electroreception in weakly electric fish for troglobitic life. Front. Ecol. Evol. 11:1180506. doi: 10.3389/fevo.2023.1180506
Received: 06 March 2023; Accepted: 06 July 2023;
Published: 01 September 2023.
Edited by:
Cristiano Bertolucci, University of Ferrara, ItalyReviewed by:
Bruce A. Carlson, Washington University in St. Louis, United StatesCopyright © 2023 Soares, Gallman, Bichuette and Fortune. This is an open-access article distributed under the terms of the Creative Commons Attribution License (CC BY). The use, distribution or reproduction in other forums is permitted, provided the original author(s) and the copyright owner(s) are credited and that the original publication in this journal is cited, in accordance with accepted academic practice. No use, distribution or reproduction is permitted which does not comply with these terms.
*Correspondence: Daphne Soares, c29hcmVzQE5KSVQuZWR1
Disclaimer: All claims expressed in this article are solely those of the authors and do not necessarily represent those of their affiliated organizations, or those of the publisher, the editors and the reviewers. Any product that may be evaluated in this article or claim that may be made by its manufacturer is not guaranteed or endorsed by the publisher.
Research integrity at Frontiers
Learn more about the work of our research integrity team to safeguard the quality of each article we publish.