- 1Co-Innovation Center for Sustainable Forestry in Southern China, Nanjing Forestry University, Nanjing, China
- 2College of Forestry, Nanjing Forestry University, Nanjing, China
- 3Jiangsu Academy of Forestry, Nanjing, China
Population density is an essential factor affecting the life history traits of insects and their trade-off relationships, as increasing density intensifies intraspecific competition. It decreases the average resources available to individuals within a population, affecting their morphology, physiology, behavior, and fitness. The fall webworm, Hyphantria cunea (Drury) (Lepidoptera: Arctiidae), has been an invasive pest of forest trees, ornamental plants, and fruit trees in China for many years. The larvae have a typical aggregation habit before the fourth instar and keep spitting silk to gather the damaged leaves into silk webs. However, the fitness of H. cunea in response to population density remains unclear. In this study, the critical biological parameters, food utilization, and population parameters of H. cunea in response to different rearing densities were investigated. The results showed that under high population density, H. cunea larvae showed better performance, with faster development, higher survival rates, and shorter generation time, but pupal weight and female fecundity decreased as population density increased. In contrast, for larvae raised in low density, the developmental period was prolonged, and mortality was increased, while higher food utilization, greater body size, and female fecundity were observed. Both males and females had similar development strategies in response to the density, but females may be more resistant to crowding than males. In conclusion, H. cunea could adopt different ecological strategies against the stress of density. High population densities result in shorter generation cycles and higher survival rates. Conversely, the low-density generation period becomes longer but with greater fecundity. The results may help determine the possible outbreak mechanism and develop effective population monitoring and forecasting measures for H. cunea.
1. Introduction
Population density impacts several eco-evolutionary processes, including as inter- and intra-specific competition, fitness, and population dynamics (Pepi et al., 2017). In holometabolous insects, the larval stage is especially vulnerable to density-dependent effects, as it is the stage that acquires resources (Dey and Joshi, 2018). Larval density-dependent effects can influence the expression of life-history features not only in the larval and adult stages but also downstream for population dynamics and evolution, altering evolutionary trajectories, species distribution ranges, and extinction risks (Ayali, 2019; Baleba et al., 2019). During the larval stage, population density influences resource availability, which can have positive and negative consequences on development and fitness (Ower and Juliano, 2019). Particularly in non-social holometabolous insects, it is recognized that competition for resources during the larval stage drives changes in larval development and adult morphology and fitness (Fantinou et al., 2008; Diamantidis et al., 2020). Because adults do not undergo molting, there is little opportunity for them to compensate for poor early growth, especially in traits like body size. As a result, the larval stage is crucial for acquiring resources, with impacts that depend on density being particularly noticeable. Interestingly, population density during the larval stage can contribute to faster adaptation to novel resources via increased intraspecific competition, a feature that can boost populations’ ability to adjust to shifting habitats and population range changes (Horgan et al., 2020; Than et al., 2020). Thus, density-dependent impacts during development have far-reaching ramifications.
Larval density has a well-reported effect on insect morphology and behavior, inducing the development of dispersal morphs (large, functional wings and flight muscles relative to body size) (Takeuchi and Hori, 2007; Tanaka et al., 2016; Baleba et al., 2019). In addition, larval density affects multiple sexually selected behaviors and morphological structures and further impacts insect ecological strategies (Applebaum and Heifetz, 1999). The general patterns of larval density that influence individuals’ size or weight are either directly reflected in the size or weight of resultant individuals or a lag between differences in population density and changes in size or weight (Peters and Barbosa, 1977). Competition for resources in the larval stage is crucial in regulating larval growth and development rates, adult lifespan traits, and fecundity (Fox and Messina, 2018). Lepidoptera is a typical holometabolan with limited ability to replenish nutrients in the adult stage. The energy required for adult reproduction depends on the energy reserves of the larval stage. In contrast, the food shortage in the larval stage will restrict the larvae’s foraging rate, food utilization, and food assignment (Altermatt and Pearse, 2011; Shin et al., 2012). Another way that larval density might benefit insects is by improving immunological function, which promotes resistance to insecticides as well as bacterial and viral infections (Grossman et al., 2018; Than et al., 2020). Several species have been reported to utilize early population density to estimate the risk of pathogen attack and to allocate resources appropriately to develop pathogen resistance, and the density-dependent effects on resistance might affect pest control in the field (Wilson et al., 2002; Martinez et al., 2018; Vanden Broecke et al., 2019). Moreover, density-dependent effects on population dynamics of pest can have a large impact on durability of pesticides (Martinez et al., 2018). Studies of the effect of negative density dependence in vital rates suggest variously that it can shorten or extend the durability of pesticides (Onstad and Flexner, 2023).
The fall webworm, Hyphantria cunea Drury (Lepidoptera: Arctiidae), is a polyphagous and severe pest of the forest, urban, and agricultural ecosystems around the world (Schowalter and Ring, 2017; Ge et al., 2019). In China, the H. cunea was first introduced in Dandong City, Liaoning Province, in 1979 and spread to Shandong, Shaanxi, Hebei, Tianjin, and Shanghai, causing significant damage to the agroforestry ecosystem (Yang et al., 2008; Tang et al., 2016). It forages on more than 400 plant species, covering nearly all deciduous trees, especially walnut, pecan, willow, mulberry, oak, sweetgum, and poplar (Edosa et al., 2019). As the name denotes, the fall webworm also is a remarkable web-maker. Larvae have an aggregation habit, 1 ~ 4 instars mainly gathered in the back of the host leaf by creating silk webs on tree branches to feed on the leaves, leaving the leaf veins and upper epidermis (Chen et al., 2020). They keep spitting silk to gather the damaged leaves into silk webs, and the increasing diameter of the silk webs expands with larval growth. After fourth instar, they break the net and disperse to feed and damage (Zalucki et al., 2002; Rezari et al., 2006; Wu et al., 2019). However, few reports have investigated density-mediated ecological adaptations of H. cunea. The biology of H. cunea in response to different rearing densities is also unknown. In this study, five populations with varying gradients of rearing density of H. cunea were established. The effects of population density on the growth, development, reproduction, and population parameters of the fall webworm were investigated to clarify the ecological response strategy of H. cunea to density factors.
2. Materials and methods
2.1. Insects
A total of 2,000–3,000 H. cunea eggs were collected from Prunus serrulata var. lannesiana (Carri.) Makino at Huaian county of Jiangsu Province (33.62°N, 119.02°E) in May, 2021. We obtained the newly hatched larvae reared on artificial diets [consisting of wheat germ (12%), casein (4.5%), Wesson’s salt (1.0%), ascorbic acid (0.5%), sugar (2%), agar (1.5%), choline chloride (0.05%), sorbic acid (0.4%), methyl p-hydroxybenzoate (0.25%), cholesterol (0.1%), Vanderzant’s vitamin mix (0.003%) and inositol (0.035%)] (Cao et al., 2014) on a fixed density (50 larvae/box (30 × 20 × 10 cm)) in a light incubator at 25 ± 1°C with a 16 h light/8 h dark cycle and 60 ± 5% humidity and fresh diets were supplied every 2 days until pupation. Pupae were sexed based on the morphology of a few terminal abdominal segments and maintained under the same conditions as larval rearing. Sixty newly emerged adults were placed at a ratio of 1:2 (female: male) in screen cages (32 × 22 × 15 cm) supplied with 10% honey water and corrugated paper at room temperature for mating and oviposition. The H. cunea colonies rearing at fixed density on artificial diets for one generation and the next generation was used for the experiments.
2.2. Larvae reared on different density
Since the neonate will die in large numbers in the following generation when they leave group rearing, post-molt second instar larvae were utilized in different density rearing trials. The newly hatched larvae are provided with sufficient diets and reared in groups at a fixed density, and after molting to the second instar, the larvae were reared at a gradient increasing density of 3, 6, 9, 12, and 15 larvae per 100 ml dish (4.5 cm × 5.7 cm × 7 cm). For each density treatment, at least 10 replicates were conducted per treatment. New artificial diets were supplemented every day. Rearing boxes were placed under the same conditions described above in a growth chamber. Growth and development parameters of the H. cunea were recorded every 24 h. The larvae were weighed at 2-day intervals until pupation, and the pupal was weighed 4–5 days after pupation by an electronic balance (ME204T, Switzerland, d = 0.0001 g). The gender of pupae was determined by the morphology of a few terminal abdominal segments (Loewy et al., 2013; Williams et al., 2015). Once the gender of the pupae was determined, the developmental duration of the male and female larvae was converted from the developmental time of each neonate to the male and female pupae. Newly emerged females and males from the same density treatment were paired and transferred into 450 ml (upper diameter 11.5 cm, bottom diameter 8 cm, and depth 6.3 cm) transparent plastic cups with a honey-water solution and corrugated paper for mating and oviposition. Honey water was replaced daily, and the oviposition period, lifetime fecundity, and adult longevity from the different density treatments were recorded. The number of eggs laid by each female on corrugated paper was counted under a binocular microscope (Leica EZ4, Germany) at the end of the oviposition.
Population parameters of the H. cunea reared on different larval densities were calculated according to age-stage, two-sex life table principle, and method (Chi, 2015; Chi et al., 2020).
Age-stage-specific survival rates (Sxj) were calculated according to Chi (Chi et al., 2020) with slight modifications:
where x = age in days and j = stage; n01 = number of second instar larvae, nxj = number of individuals at age x and stage j.
Age-specific fecundity (mx): the number of eggs per individual at age x:
Where m is the number of stages and fxj is the number of hatched eggs produced by female adults at age x.
Age-specific survival rate (lx) was calculated according to Chi (Chi et al., 2020) with slight modifications:
The intrinsic rate of increase (r) is estimated by using the iterative bisection method from the Euler-Lotka equation with age indexed from zero (Goodman, 1982):
Finite rate of increase (λ):
Net reproductive rate (R0):
Mean generation time (T):
2.3. Feeding experiments
Since significant differences in body size appeared between 14 and 17 days of larval development at different rearing densities, the fourth instar larvae were selected for feeding tests. We randomly selected 15 healthy larvae from each density treatment group. Then larvae were transferred to new Petri dishes (diameter 6 cm, depth 1.8 cm) with sufficient clearance between the lid and the bottom to ensure air circulation in the microenvironment. Before the feeding experiment started, the larvae were starved for 12 h to allow them to excrete excess feces. Subsequently, the larvae were weighed and supplied with a pre-weighed (approximately 1 g) diet for each larva. After 48 h, we weighed the remaining diet and feces. Five fresh diets were weighed and dried in an oven (at 60°C for 48 h), then re-weighed to determine the dry mass of the artificial diet. The dry mass of the artificial diets was calculated by the wet-dry regression formula for the linear regression was calculated (n = 5, dry mass = 0.00481 + 0.22539*wet mass, r2 = 0.996). The dry mass of larvae was regressed against the wet mass of larvae before feeding, and the wet-dry regression formula for the linear regression was calculated (n = 8, dry mass = −0.008 + 0.30416*wet mass, r2 = 0.993). We used the relative growth rate (RGR), relative consumption rate (RCR), the efficiency of converting ingested food into body matter (ECI), and the efficiency of conversion of digested food into body matter (ECD) to analyze the consumption and feeding efficiency. The formulas used to calculate these nutritional indices are as follows (Waldbauer, 1968):
Relative growth rate (RGR) (mg·mg -1·d -1) = (B – A)/ [((A + B)/2) × T];
Relative consumption rate (RCR) (mg·mg -1·d -1) = (D – C)/ [((A + B)/2) × T];
Efficiency of conversion of ingested food (ECI) % = (B – A)/F;
Efficiency of conversion of digested food (ECD)% = (B – A)/ [((D – C) – F) × T];
A is the dry mass of the larva before feeding; B is the dry mass of the larva after feeding; C is the dry mass of food before larvae feeding; D is the dry mass of food after larvae feeding; F is the dry mass of feces, and T is the duration of the feeding period.
2.4. Statistical analysis
One-way ANOVA was used for the comparative analysis of developmental periods of different stages, larval weight, pupal weight, and female fecundity of H. cunea under different rearing densities, followed by the Tukey’s test with α = 0.05 (Data are normally distributed). Kruskal-Wallis ANOVA test (p = 0.05) was used to compare the food consumption (RGR, RCR, ECI, and ECD) of larvae reared at different densities (Data does not conform to normal distribution). The sex ratios were compared using Chi-square tests. Yates’ correction was used when the expected frequency was below 5. Kaplan–Meier survival curves analyzed the survival of the larvae and pupae in each group, and the curves were compared using a log-rank test. The data above were analyzed using the statistical software package SPSS 22.0 (IBM Inc., New York, United States) and plotted using R 4.2.0 (R Core Team, 2022) within Rstudio (RStudio Team, 2022).
TWOSEX-MSChart 2022 software1 was used to calculate population parameters of H. cunea under different rearing densities, and the standard errors were determined by bootstrapping with 100,000 repetitions (Johnson, 2001; Tuan et al., 2014). TWOSEX-MSChart software was used to evaluate the statistical significance of the observed differences. Group differences were evaluated by paired bootstrapping, with p < 0.05 considered statistically significant.
3. Results
3.1. Development time of each stage of Hyphantria cunea
On different rearing densities, H. cunea could complete its whole life cycle. Varying larvae densities had a substantial effect on the developmental duration of the H. cunea larval and pupal stages (Male larvae: F4,191 = 21.7, p < 0.01; female larvae: F4,145 = 18.5, p < 0.01; female pupae: F4,105 = 5.3, p < 0.01), but not on adult lifespan (Male adults: F4,135 = 0.82, p = 0.51; female adults: F4,105 = 0.82, p = 0.51). Whether female or male larvae, the developmental period gradually shortened with increasing rearing density (Table 1). Crowding shortened the developmental period of larvae. Larvae develop more quickly in high-density rearing than in low-density rearing (Table 1). Larval periods reared at 15 larvae/dish compared to 3 larvae/dish, reducing by 1.6 days in males and by 0.9 days in females. Similarly, the female pupation period of rearing at 15 larvae/dish was also significantly shorter than that of 3 larvae/dish (Table 1). However, the difference in developmental duration was only in the preadult stage (larval and pupal stages, Table 1). Larval rearing density did not significantly affect adult longevity (Table 1; p > 0.05).
3.2. Survival rate
Larval survival rate was significantly affected by rearing density, and the survival rate of H. cunea larvae reared in high-density was significantly higher than rearing in low-density (log-rank test, χ2 = 24.93, p < 0.01). The increase in larval population density resulted in an increase in larval survival rate (Figure 1; Supplementary Table S1), and larval survival was greatest at a rearing density of 15 larvae/dish, which was much higher than the other rearing density (p < 0.01). Larval survival was lowest at 3 larvae/dish, which was not statistically different from 6 larvae/dish (χ2 = 0.5, p = 0.47) but considerably lower than the other treatment groups (Figure 1; Supplementary Table S1). There were no significant changes in pupal survival rates between different rearing densities (Supplementary Table S2; χ2 = 4.89, p = 0.29).
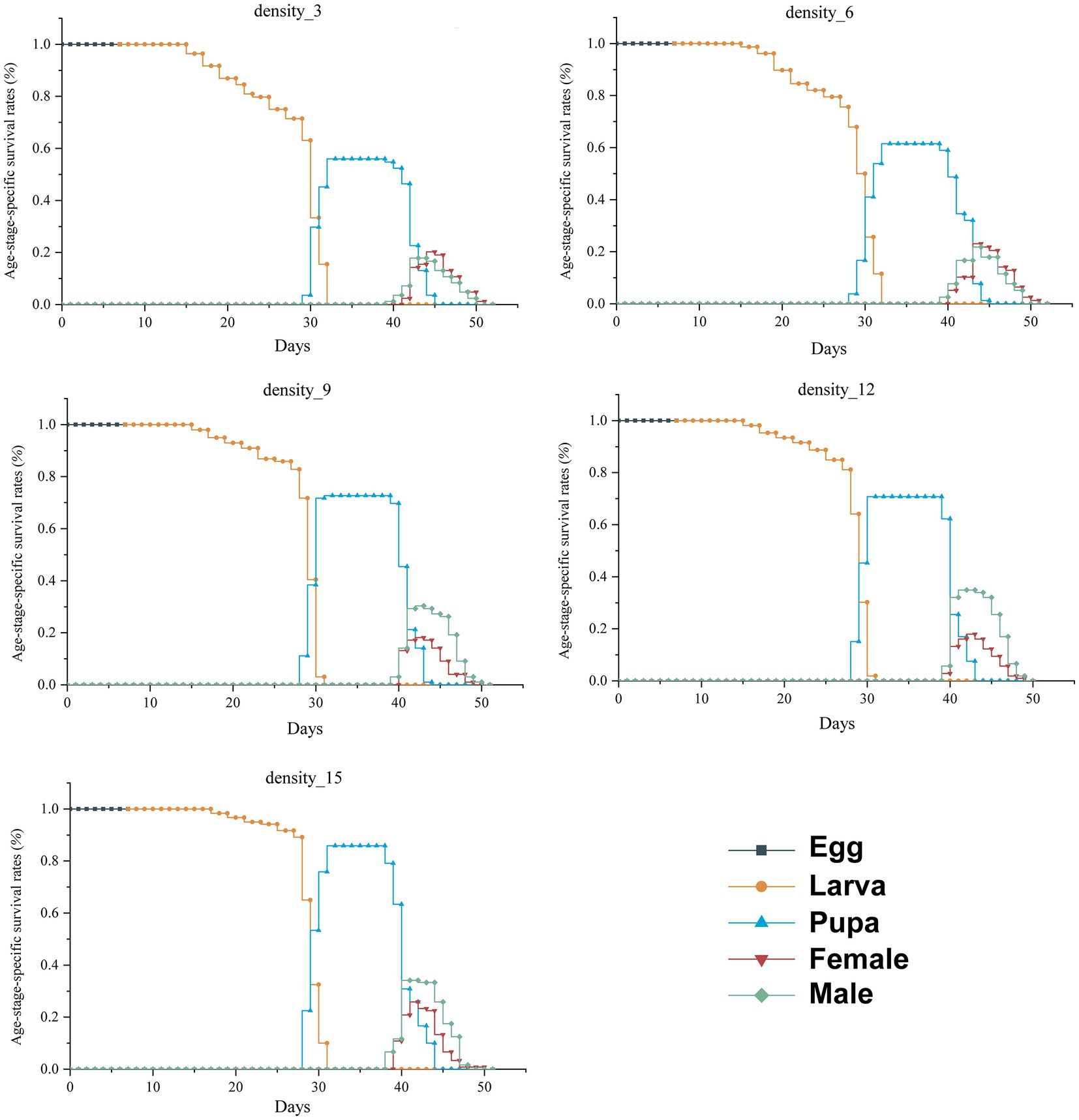
Figure 1. Survival rate of H. cunea under different rearing densities. Sxj: the probability that a newly laid egg will survive to age x and stage j.
3.3. Larval body size and food utilization
Larval body size, as well as food utilization, were significantly influenced by rearing density. The larvae under high-density rearing showed more rapid early development and larger size (Figure 2A). The larvae in the 3 larvae/dish rearing were consistently relatively more minor in size until 17 days of development (Figure 2A), and after 17 days of development, larvae in high-density rearing slowed down their body size development. Larvae reared at 15 larvae/dish were significantly smaller than those reared at 3 larvae/dish and 6 larvae/dish (Figure 2A; p < 0.05). Subsequently, in the feeding assay, it was found that there was no significant difference in the relative consumption rate (RCR) of 4–5 instar larvae at different density conditions (Figure 2B; Kruskal-Wallis test, p > 0.05). However, the efficiency of conversion of digested food (ECD) and the efficiency of conversion of ingested food (ECI) decreased significantly with increasing rearing density (Figure 2B; p < 0.05). The highest ECD and ECI were found for larvae reared at 3 and 6 larvae/dish (Figure 2B; p < 0.05). Furthermore, the relative growth rate (RGR) of larvae was consistent with the trend of the ECI, and the RGR reared at low density was comparatively higher.
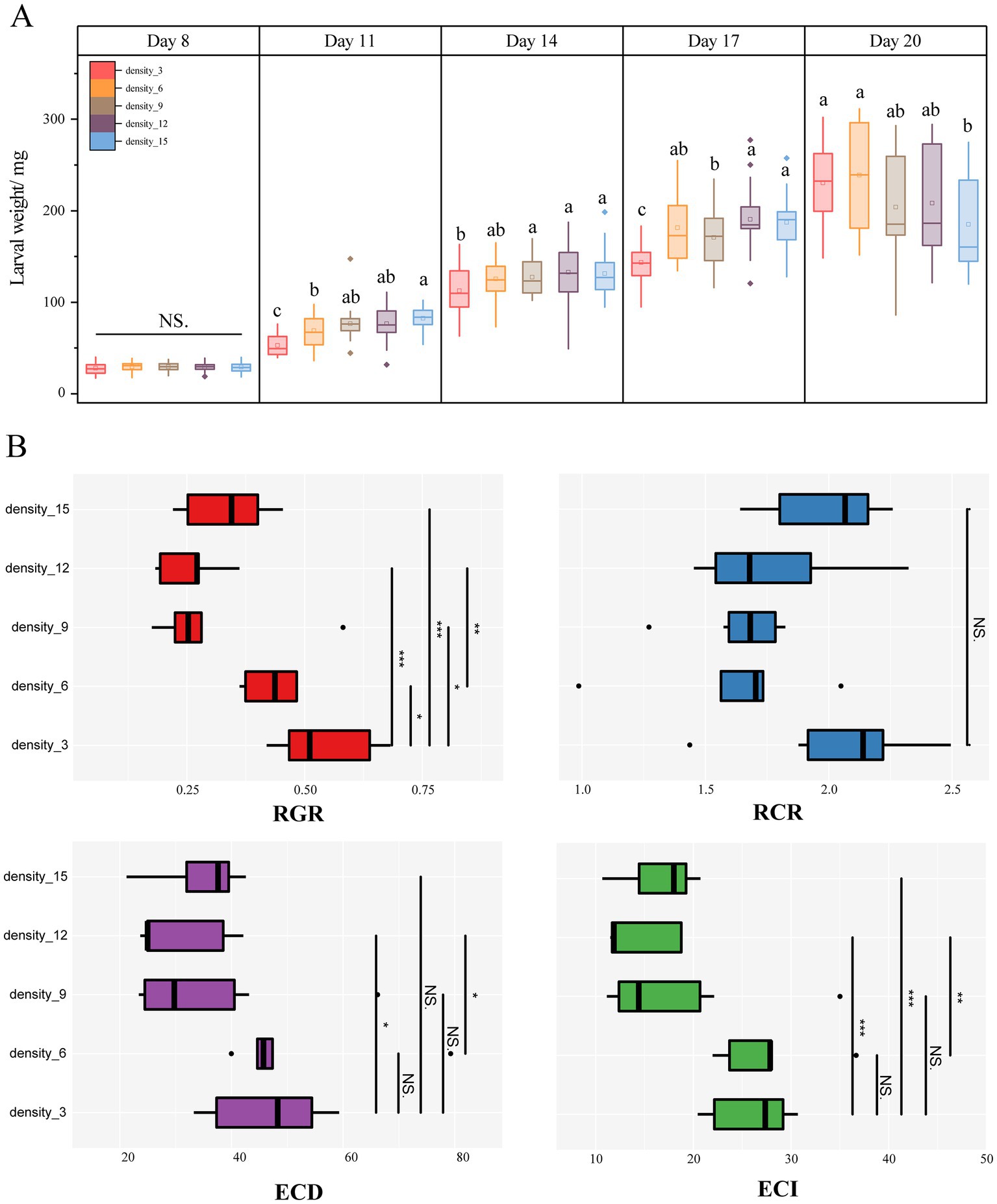
Figure 2. larval body weight (A) and food utilization (B) of H. cunea reared under different larval densities. Lowercase letters indicate significant differences in the body weight of H. cunea larvae reared at different densities, p < 0.05, Turkey test. The asterisks represent significant differences among larvae reared at different densities by the Kruskal-Wallis test. *p < 0.05, **p < 0.001, ***p < 0.0001, NS, not significant.
3.4. Pupal weight, sex ratio, and fertility
There was a significant effect of larval rearing density on the biological parameters of the post-pupae developmental stages of H. cunea larvae. In the statistics of the male-to-female sex ratio, we found that the male-to-female ratio under low-density rearing conditions was more significant than or close to 1:1. In contrast, as the rearing density increased, the proportion of females in the population was lower than that of males, and males were in the majority (Figure 3C; Chi-square test, χ2 = 11.91, p = 0.018). Pupal weights of both sexes were significantly affected by larval density and showed a similar trend, with an increase in rearing density leading to a decrease in pupal weight. Three and six larvae/dish densities resulted in the highest pupal weights for both sexes (Figures 3A,B). Moreover, with a similar tendency to pupae weight, female adults under 3 and 6 larvae/dish rearing exhibit higher fecundity for per female (Figure 3D).
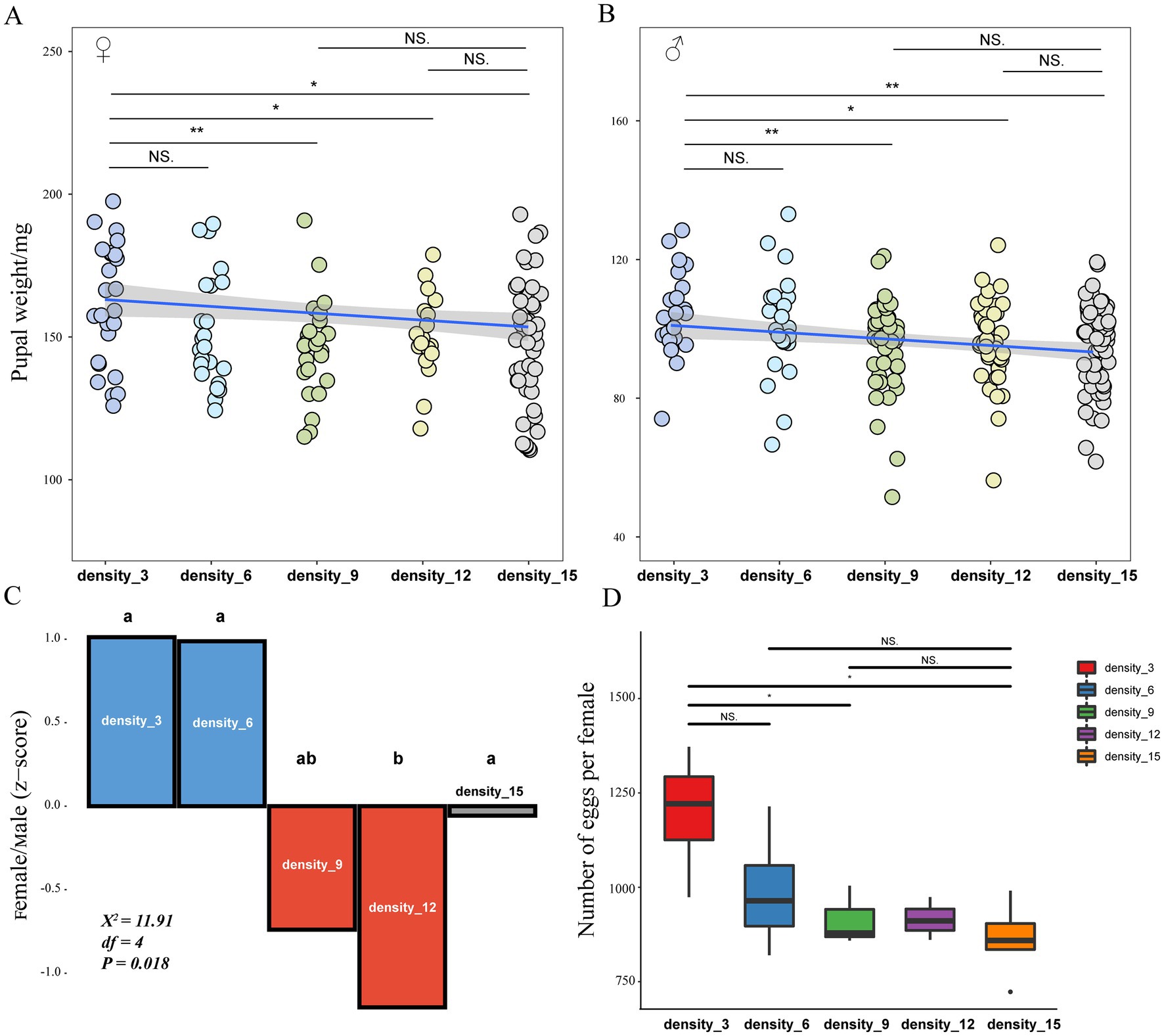
Figure 3. Pupal weight (A,B), sex ratio (C), and fertility (D) of H. cunea reared at different densities. Lowercase letters represent significant differences among larvae reared on different densities by Chi-square test, p < 0.05. The asterisks represent significant differences among larvae reared at different densities by the Turkey test, *p < 0.05, **p < 0.001, NS, not significant.
3.5. Population parameters
The r and λ values of H. cunea populations were > 0 and > 1, respectively, for all rearing density situations (Table 2), indicating that H. cunea could complete their life cycles under diverse population density circumstances. Nevertheless, no substantial differences in R0, r, and λ were identified across population densities. On the other hand, the mean generation time (T) of H. cunea populations raised in low density is longer than that of populations raised in high density.
4. Discussion
Intraspecific food resource competition is critical for organism growth and adult traits(Thiery et al., 2014). When population density grows, intraspecific competition intensifies, lowering the average resources individuals gain within the community (Horgan et al., 2020). At first glance, size, developmental rate, and fecundity seem intimately linked as reciprocal representations of rearing pressures’ impact on insects (Peters and Barbosa, 1977). Nevertheless, substantial evidence shows that these factors vary in their sensitivity to density but may be completely independent (Klepsatel et al., 2018).
For species with complicated life cycles, such as Lepidoptera, variations in larval density and food availability are always crucial in influencing adult fitness. The H. cunea is a polyphagous pest species in forest and agricultural ecosystems. Before the fourth instar, its larvae congregate by building silk webs on tree branches. This social behavior keeps them temperate and protects them from predators (Chen et al., 2020). In the forest, the growth and development of the H. cunea are hardly constrained by the population density due to sufficient space and food resources. In this study, the H. cunea exhibits population density-dependent effects on life-history traits with a gradient of rearing density in constant space. Crowding reduced larval development time substantially, and larvae developed faster in group raising than in low-density rearing in both females and males. Intraspecific competition caused by higher population density could evoke early pupation of larvae as a kind of biotic stress (Välimäki et al., 2013). On the other hand, a high larval density may signal to the larva that the resource is likely to become entirely exhausted in a shorter span of time due to intra- and inter-specific competition, which would accelerate the growth of the larva (Bauerfeind and Fischer, 2005; Yang et al., 2015). In Bicyclus anynana, a high larval rearing density had contrary to common wisdom, minimal impact on body size but reduced larval development time through increased growth rates (Bauerfeind and Fischer, 2005). Similar findings have been reported in the Lymantria dispar. The larval development time shortened with increasing population density and the same response in the European population and two Asian population colonies within a specific rearing density range (Wang et al., 2021).
We discovered that, in addition to developing time, H. cunea survival was highly impacted by population density and increased with rising rearing density. The primary reason for mortality during insect raising is infection by pathogenic microorganisms in the growing microenvironment. Pathogen-infected insects are common in the microenvironment (Anderson and May, 1981; Kong et al., 2018). Pathogen transmission is generally density-dependent; hence, the probability of insects getting infected with pathogens increases as population density increases. Yet, it has been shown that numerous insects use early population density to predict the potential of pathogen infection and allocate resources to develop pathogen resistance (Wilson and Reeson, 1998). Individuals raised in high-density conditions are expected to be more resistant to infections (or parasites) than those raised in low-density conditions. This phenomenon, termed “density-dependent prophylaxis” (DDP), has been observed in some phase-polyphenic insect species (Wilson et al., 2002; Wilson and Graham, 2015). The high population density could alter insect prophylactic immunity, and the immune system plays a vital role in insect prophylactic resistance to various microorganisms (Wilson et al., 2001; Wang et al., 2013). Therefore, the higher the rearing density, the greater the survival rate of larvae within a certain limited density. Similar findings were reported in Plutella xylostella. Larvae reared in groups showed a significantly higher survival rate than lower-density populations (Kong et al., 2020). In other Lepidoptera species, such as Spodoptera littoralis, Mythimna separata, the survival rate of larvae challenged by entomopathogenic fungi and viruses was significantly increased in larvae reared at higher densities (Wilson et al., 2001; Kong et al., 2018).
Furthermore, several ecologists and evolutionary biologists have proposed the slow growth-high mortality hypothesis for the evolution of sublethal effects to mitigate herbivory in response to low nutritional quality (Williams, 1999). The hypothesis states that herbivores with slower growth suffer more significant mortality by natural enemies due to an extended period of vulnerability (Chen and Chen, 2018). In this study, larval crowding shortens the developmental period, and greater survival rates are shown in populations with higher densities, supporting this hypothesis.
The performance and body composition of insect larvae depend on the quality and quantity of their diet and biotic factors such as larval density. The strong response of the larvae to overcrowding has been observed in immature stages of other insect species and, in many cases, has been attributed to competition for limited nutrients (Averill and Prokopy, 1987; Agnew et al., 2000; Reiskind et al., 2004). A restricted diet can directly impact mass gain. It may also influence the timing and pattern of gene expression, resulting in a lack of nutrient acquisition and reduced body size. The food consumption rate and utilization efficiency changes underlie fitness modifications (Kause et al., 1999). In this study, the larvae under high-density rearing showed more rapid in early development and larger size. As larvae mature, larvae raised in high densities possess slower growth rates; the food utilization parameters of H. cunea were significantly and negatively impacted by increasing larval densities, which negatively affected larval and pupal mass. Larvae rearing at lower density facilitated a higher relative growth rate, the efficiency of ingested food conversion, and the efficiency of digested food conversion. In Tenebrio molitor, larval weight gain, food consumption, ECI, ECD, and LWGpFC were negatively impacted by larval density (Morales-Ramos and Rojas, 2015). The Loxostege sticticalis larvae reared at 1 larva, 10 larvae, and 50 larvae per jar; the body weight and the efficiency of conversation of ingested food declined as larval density rose (Kong et al., 2012). The larval weight, approximate digestibility, and relative growth rate decreased with increasing larval density were observed in the Mythimna separata, indicating population density was a major factor affecting larval feeding behavior (Lizhi et al., 1995).
Larvae rearing in a crowded situation might develop more quickly, but rapid development was at the expense of larval and pupal size (Müller et al., 2016). The general patterns of population density influence on size or weight are either an immediate effect in which changes in population density are quickly reflected in the size or weight of consequent individuals or a lag between population density variations and modifications in size or weight (Peters and Barbosa, 1977; Applebaum and Heifetz, 1999). In this study, rearing density substantially affected the pupal weight of H. cunea, both male and female, with a significant decrease in pupal weight as rearing density increased, and the greater pupal weight usually implies higher fertility (Teimouri et al., 2015). In Lepidoptera, since the adult stage no longer molts, the adult stage has a very restricted capacity to compensate for life history characteristics, particularly those with decreased body size. Hence, competition for resources during larval influences adult morphological characteristics and fitness (Dmitriew and Rowe, 2011; Barbosa et al., 2016; Fox and Messina, 2018). Fecundity reduction with increasing larval density was observed in C. medinalis females even if the food supply was enough for larval growth (Yang et al., 2015). In Spodoptera exigua, pupal weight was significantly different and negatively correlated to larval rearing densities, and female fecundity was positively correlated with pupal weight (Wang et al., 2008). In Diabrotica virgifera LeConte, different density rearing conditions resulted in different fly-specified durations for adults, and female fecundity was significantly affected (Yu et al., 2019). In this study, higher fecundity was also observed in lower-density populations.
Furthermore, both sexes had similar development strategies, but females seemed more resistant to variable rearing density. We found that larvae have a higher proportion of females at lower rearing densities but also have a more extended larval developmental period and higher mortality. In lower-density populations, H. cunea takes more time to develop to obtain more nutrients. Meanwhile, the extended developmental period means more opportunities to be harmed by environmental microorganisms (Chen and Chen, 2018). The male is less resistant, resulting in a population reared at lower densities with more females. There are more female individuals in the population, which means that different rearing densities affect the sex ratio of the population by affecting the survival rate of the male individuals. Sex bias caused by abiotic factors is common in Lepidoptera. In Danaus chrysippus, sex ratio distortion is caused by the intracellular bacterium Spiroplasma which induces male killing (Jiggins et al., 2000b). In Acraea encedon, sex ratio bias was associated with a maternally inherited bacterium that kills males and could be cured by antibiotic treatment (Jiggins et al., 1998, 2000a). Sex ratio and sex-biased mortality were investigated in Eucheira socialis, and larval mortality was consistently disproportionately male, varying from 83 to 100% male, and pupal mortality tended to be female-biased (Underwood and Shapiro, 1999).
Larval density-dependent effects can modulate a species’ interactions with itself (e.g., intraspecific competition), other species (e.g., predators, preys), or both (May et al., 1981; Arditi et al., 2001). This will define both the ecological relevance of the species within the environment (density-dependent ecological impacts) and the evolutionary trajectory of the species across generations (Cappuccino, 1992; Than et al., 2020). In this study, the r and λ values of H. cunea populations were > 0 and > 1, respectively, for different rearing densities, suggesting that H. cunea can complete their life cycle in different population densities. However, the differences between r and λ were nonsignificant on different rearing densities, indicating no difference in the population growth rate of H. cunea under different population density conditions. However, the average generation period of H. cunea was shorter under high-density rearing than under low-density rearing.
In conclusion, this experiment investigated the variation of biological parameters and population parameters of H. cunea under different density conditions by assigning a gradient variation of rearing density. The results show that H. cunea could adopt a flexible ecological strategy in response to population density stress. Under high population density conditions, H. cunea would sacrifice body size and egg production to complete development faster and achieve higher survival rates. Under low population density conditions, H. cunea will possess a larger body size and more offspring at the expense of slower growth and higher mortality.
Data availability statement
The raw data supporting the conclusions of this article will be made available by the authors, without undue reservation.
Author contributions
TH: data curation. XZ and CX: formal analysis. ZZ and WX: investigation. MX and YG: project administration. XZ: writing—original draft. DH: writing—review and editing. All authors have read and agreed to the published version of the manuscript.
Funding
This research was supported by the National Key R&D Program of China (2022YFC2601002), the Science and Technology Project of Shanghai Landscaping and City Appearance Administrative Bureau (grant number G221207) and the Forestry Science and Technology Innovation and Promotion Project in Jiangsu Province [grant number LYKJ (2019)04].
Conflict of interest
The authors declare that the research was conducted in the absence of any commercial or financial relationships that could be construed as a potential conflict of interest.
Publisher’s note
All claims expressed in this article are solely those of the authors and do not necessarily represent those of their affiliated organizations, or those of the publisher, the editors and the reviewers. Any product that may be evaluated in this article, or claim that may be made by its manufacturer, is not guaranteed or endorsed by the publisher.
Supplementary material
The Supplementary material for this article can be found online at: https://www.frontiersin.org/articles/10.3389/fevo.2023.1177029/full#supplementary-material
Footnotes
References
Agnew, P., Haussy, C., and Michalakis, Y. (2000). Effects of density and larval competition on selected life history traits of Culex pipiens quinquefasciatus (Diptera: Culicidae). J. Med. Entomol. 37, 732–735. doi: 10.1603/0022-2585-37.5.732
Altermatt, F., and Pearse, I. S. (2011). Similarity and specialization of the larval versus adult diet of European butterflies and moths. Am. Nat. 178, 372–382. doi: 10.1086/661248
Anderson, R. M., and May, R. M. (1981). The population dynamics of microparasites and their invertebrate hosts. Philos. Trans. R. Soc. Lond. B 291, 451–524. doi: 10.1098/rstb.1981.0005
Applebaum, S. W., and Heifetz, Y. (1999). Density-dependent physiological phase in insects. Annu. Rev. Entomol. 44, 317–341. doi: 10.1146/annurev.ento.44.1.317
Arditi, R., Tyutyunov, Y., Morgulis, A., Govorukhin, V., and Senina, I. (2001). Directed movement of predators and the emergence of density-dependence in predator–prey models. Theor. Popul. Biol. 59, 207–221. doi: 10.1006/tpbi.2001.1513
Averill, A. L., and Prokopy, R. J. (1987). Intraspecific competition in the tephritid fruit fly Rhagoletis pomonella. Ecology 68, 878–886. doi: 10.2307/1938359
Ayali, A. (2019). The puzzle of locust density-dependent phase polyphenism. Curr. Opin. Insect Sci. 35, 41–47. doi: 10.1016/j.cois.2019.06.008
Baleba, S. B. S., Masiga, D., Torto, B., Weldon, C. W., and Getahun, M. N. (2019). Effect of larval density and substrate quality on the wing geometry of Stomoxys calcitrans L. (Diptera: Muscidae). Parasite Vector 12:222. doi: 10.1186/s13071-019-3483-y
Barbosa, T. A. N., Mendes, S. M., Rodrigues, G. T., De Aquino Ribeiro, P. E., Dos Santos, C. A., Valicente, F. H., et al. (2016). Comparison of biology between Helicoverpa zea and Helicoverpa armigera (Lepidoptera: Noctuidae) reared on artificial diets. Fla. Entomol. 99, 72–76. doi: 10.1653/024.099.0113
Bauerfeind, S. S., and Fischer, K. (2005). Effects of food stress and density in different life stages on reproduction in a butterfly. Oikos 111, 514–524. doi: 10.1111/j.0030-1299.2005.13888.x
Cao, L., Yang, F., Tang, S., and Chen, M. (2014). Development of an artificial diet for three lepidopteran insects. Chin. J. Appl. Entomol. 51, 1376–1386. doi: 10.7679/j.issn.2095-1353.2014.165
Cappuccino, N. (1992). Adjacent trophic-level effects on spatial density dependence in a herbivore–predator–parasitoid system. Ecol. Entomol. 17, 105–108. doi: 10.1111/j.1365-2311.1992.tb01165.x
Chen, K. W., and Chen, Y. (2018). Slow-growth high-mortality: a meta-analysis for insects. Insect Sci. 25, 337–351. doi: 10.1111/1744-7917.12399
Chen, Q., Zhao, H., Wen, M., Li, J., Zhou, H., Wang, J., et al. (2020). Genome of the webworm Hyphantria cunea unveils genetic adaptations supporting its rapid invasion and spread. BMC Genomics 21:242. doi: 10.1186/s12864-020-6629-6
Chi, H. (2015). TWOSEX-MSChart: a computer program for the age-stage, two-sex life table analysis. Available at: http://140.120.197.173/Ecology/.
Chi, H., You, M., Atlihan, R., Smith, C. L., Kavousi, A., Özgökçe, M. S., et al. (2020). Age-stage, two-sex life table: an introduction to theory, data analysis, and application. Entomol. Gen. 40, 103–124. doi: 10.1127/entomologia/2020/0936
Dey, S., and Joshi, A. (2018). “Two decades of drosophila population dynamics: modeling, experiments, and implications” in Handbook of statistics. eds. S. R. Srinivasa Rao and C. R. Rao (Amsterdam, Netherlands: Elsevier), 275–312.
Diamantidis, A. D., Ioannou, C. S., Nakas, C. T., Carey, J. R., and Papadopoulos, N. T. (2020). Differential response to larval crowding of a long-and a short-lived medfly biotype. J. Evol. Biol. 33, 329–341. doi: 10.1111/jeb.13569
Dmitriew, C., and Rowe, L. (2011). The effects of larval nutrition on reproductive performance in a food-limited adult environment. PLoS One 6:e17399. doi: 10.1371/journal.pone.0017399
Edosa, T. T., Jo, Y. H., Keshavarz, M., Anh, Y. S., Noh, M. Y., and Han, Y. S. (2019). Current status of the management of fall webworm, Hyphantria cunea: towards the integrated pest management development. J. Appl. Entomol. 143, 1–10. doi: 10.1111/jen.12562
Fantinou, A. A., Perdikis, D. C., and Stamogiannis, N. (2008). Effect of larval crowding on the life history traits of Sesamia nonagrioides (Lepidoptera: Noctuidae). Eur. J. Entomol. 105, 625–630. doi: 10.14411/eje.2008.084
Fox, C. W., and Messina, F. J. (2018). Evolution of larval competitiveness and associated life-history traits in response to host shifts in a seed beetle. J. Evol. Biol. 31, 302–313. doi: 10.1111/jeb.13222
Ge, X., He, S., Zhu, C., Wang, T., Xu, Z., and Zong, S. (2019). Projecting the current and future potential global distribution of Hyphantria cunea (Lepidoptera: Arctiidae) using CLIMEX. Pest Manag. Sci. 75, 160–169. doi: 10.1002/ps.5083
Goodman, D. (1982). Optimal life histories, optimal notation, and the value of reproductive value. Am. Nat. 119, 803–823. doi: 10.1086/283956
Grossman, M. K., Uc-Puc, V., Flores, A. E., Manrique-Saide, P. C., and Vazquez-Prokopec, G. M. (2018). Larval density mediates knockdown resistance to pyrethroid insecticides in adult Aedes aegypti. Parasit. Vectors 11, 1–6. doi: 10.1186/s13071-018-2865-x
Horgan, F. G., Arida, A., Ardestani, G., and Almazan, M. L. P. (2020). Intraspecific competition counters the effects of elevated and optimal temperatures on phloem-feeding insects in tropical and temperate rice. PLoS One 15:e0240130. doi: 10.1371/journal.pone.0240130
Jiggins, F., Hurst, G., Dolman, C., and Majerus, M. (2000a). High prevalence male-killing Wolbachia in the butterl y Acraea encedana. J. Evol. Biol. 13, 495–501. doi: 10.1046/j.1420-9101.2000.00180.x
Jiggins, F., Hurst, G., Jiggins, C., Vd Schulenburg, J., and Majerus, M. (2000b). The butterfly Danaus chrysippus is infected by a male-killing Spiroplasma bacterium. Parasitology 120, 439–446. doi: 10.1017/S0031182099005867
Jiggins, F. M., Hurst, G. D., and Majerus, M. E. (1998). Sex ratio distortion in Acraea encedon (Lepidoptera: Nymphalidae) is caused by a male-killing bacterium. Heredity 81, 87–91. doi: 10.1046/j.1365-2540.1998.00357.x
Johnson, R. W. (2001). An introduction to the bootstrap. Teach. Stat. 23, 49–54. doi: 10.1111/1467-9639.00050
Kause, A., Saloniemi, I., Haukioja, E., and Hanhimaki, S. (1999). How to become large quickly: quantitative genetics of growth and foraging in a flush feeding lepidopteran larva. J. Evol. Biol. 12, 471–482. doi: 10.1046/j.1420-9101.1999.00045.x
Klepsatel, P., Prochazka, E., and Galikova, M. (2018). Crowding of Drosophila larvae affects lifespan and other life-history traits via reduced availability of dietary yeast. Exp. Gerontol. 110, 298–308. doi: 10.1016/j.exger.2018.06.016
Kong, H., Dong, C., Tian, Z., Mao, N., Wang, C., Cheng, Y., et al. (2018). Altered immunity in crowded Mythimna separata is mediated by octopamine and dopamine. Sci. Rep. 8, 1–10. doi: 10.1038/s41598-018-20711-8
Kong, H., Liu, Z., Yang, P., Yuan, L., Jing, W., Dong, C., et al. (2020). Effects of larval density on Plutella xylostella resistance to Granulosis virus. Insects 11:857. doi: 10.3390/insects11120857
Kong, H., Luo, L., Jiang, X., Zhang, L., Yang, Z., and Hu, Y. (2012). Effects of larval density on food utilization efficiency and digestive enzyme activity of the beet webworm, Loxostege sticticalis (Lepidoptera: Pyralidae). Acta Entomol. Sin. 55, 361–366. doi: 10.16380/j.kcxb.2012.03.009
Lizhi, L., Haizhong, X., and Guangbo, L. (1995). Effects of rearing density on the food consumption and utilization of larval oriental armyworm Mythimna separata (Walker). Acta Entomol. Sin. 38, 429–435. doi: 10.16380/j.kcxb.1995.04.007
Loewy, K. J., Flansburg, A. L., Grenis, K., Kjeldgaard, M. K., Mccarty, J., Montesano, L., et al. (2013). Life history traits and rearing techniques for fall webworms (Hyphantria cunea Drury) in Colorado. J. Lepid Soc. 67, 196–205. doi: 10.18473/lepi.v67i3.a6
Martinez, J. C., Caprio, M. A., and Friedenberg, N. A. (2018). Density dependence and growth rate: evolutionary effects on resistance development to Bt (Bacillus thuringiensis). J. Econ. Entomol. 111, 382–390. doi: 10.1093/jee/tox323
May, R., Hassell, M., Anderson, R., and Tonkyn, D. (1981). Density dependence in host-parasitoid models. J. Anim. Ecol. 50, 855–865. doi: 10.2307/4142
Morales-Ramos, J. A., and Rojas, M. G. (2015). Effect of larval density on food utilization efficiency of Tenebrio molitor (Coleoptera: Tenebrionidae). J. Econ. Entomol. 108, 2259–2267. doi: 10.1093/jee/tov208
Müller, T., Küll, C. L., and Müller, C. (2016). Effects of larval versus adult density conditions on reproduction and behavior of a leaf beetle. Behav. Ecol. Sociobiol. 70, 2081–2091. doi: 10.1007/s00265-016-2212-1
Onstad, D. W., and Flexner, J. L. (2023). “Insect resistance, natural enemies, and density-dependent processes” in Insect resistance management. eds. David W. Onstad and Lisa M. Knolhoff (Amsterdam, Netherlands: Elsevier), 381–399.
Ower, G. D., and Juliano, S. A. (2019). Effects of larval density on a natural population of Culex restuans (Diptera: Culicidae): no evidence of compensatory mortality. Ecol. Entomol. 44, 197–205. doi: 10.1111/een.12689
Pepi, A. A., Broadley, H. J., and Elkinton, J. S. (2017). Erratum to: density-dependent effects of larval dispersal mediated by host plant quality on populations of an invasive insect. Oecologia 185, 533–535. doi: 10.1007/s00442-017-3947-8
Peters, T., and Barbosa, P. B. (1977). Influence of population density on size, fecundity, and developmental rate of insects in culture. Annu. Rev. Entomol. 22, 431–450. doi: 10.1146/annurev.en.22.010177.002243
Reiskind, M. H., Walton, E. T., and Wilson, M. L. (2004). Nutrient-dependent reduced growth and survival of larval Culex restuans (Diptera: Culicidae): laboratory and field experiments in Michigan. J. Med. Entomol. 41, 650–656. doi: 10.1603/0022-2585-41.4.650
Rezari, V., Moharamipour, S., Fathipour, Y., and Talebi, A. A. (2006). Nutritional indices and host preference of American white webworm, Hyphantria cunea (Lepidoptera: Arctiidae) on five host plants. J. Entomol. Soc. Iran 26, 57–72.
R Core Team (2022). R: a language and environment for statistical computing. R Foundation for Statistical Computing, Vienna, Austria. http://www.R-project.org/
Schowalter, T. D., and Ring, D. R. (2017). Biology and Management of the Fall Webworm, Hyphantria cunea (Lepidoptera: Erebidae). J. Integr. Pest. Manag. 8, 1–6. doi: 10.1093/jipm/pmw019
Shin, S. M., Akram, W., and Lee, J. J. (2012). Effect of body size on energy reserves in Culex pipiens pallens females (Diptera: Culicidae). Entomol. Res. 42, 163–167. doi: 10.1111/j.1748-5967.2012.00448.x
Takeuchi, Y., and Hori, M. (2007). Spatial density-dependent survival and development at different larval stages of the tiger beetle Cicindela japonica (Thunberg). Popul. Ecol. 49, 305–316. doi: 10.1007/s10144-007-0047-3
Tanaka, S., Harano, K. I., Nishide, Y., and Sugahara, R. (2016). The mechanism controlling phenotypic plasticity of body color in the desert locust: some recent progress. Curr. Opin. Insect Sci,. 17, 10–15. doi: 10.1016/j.cois.2016.05.011
Tang, R., Zhang, F., and Zhang, Z. N. (2016). Electrophysiological responses and reproductive behavior of fall webworm moths (Hyphantria cunea Drury) are influenced by volatile compounds from its mulberry host (Morus alba L.). Insects 7:19. doi: 10.3390/insects7020019
Teimouri, N., Sendi, J. J., Zibaee, A., and Khosravi, R. (2015). Feeding indices and enzymatic activities of carob moth Ectomyelois ceratoniae (Zeller) (Lepidoptera: pyrallidae) on two commercial pistachio cultivars and an artificial diet. J. Saudi Soc. Agric. Sci. 14, 76–82. doi: 10.1016/j.jssas.2013.08.003
Than, A. T., Ponton, F., and Morimoto, J. (2020). Integrative developmental ecology: a review of density-dependent effects on life-history traits and host-microbe interactions in non-social holometabolous insects. Evol. Ecol. 34, 659–680. doi: 10.1007/s10682-020-10073-x
Thiery, D., Monceau, K., and Moreau, J. (2014). Larval intraspecific competition for food in the European grapevine moth Lobesia botrana. Bull. Entomol. Res. 104, 517–524. doi: 10.1017/S0007485314000273
Tuan, S. J., Lee, C. C., and Chi, H. (2014). Population and damage projection of Spodoptera litura (F.) on peanuts (Arachis hypogaea L.) under different conditions using the age-stage, two-sex life table. Pest Manag. Sci. 70, 805–813. doi: 10.1002/ps.3618
Underwood, D. L., and Shapiro, A. M. (1999). A male-biased primary sex ratio and larval mortality in Eucheira socialis (Lepidoptera: Pieridae). Evol. Ecol. Res. 1, 703–717.
Välimäki, P., Kivelä, S. M., and Mäenpää, M. I. (2013). Temperature-and density-dependence of diapause induction and its life history correlates in the geometrid moth Chiasmia clathrata (Lepidoptera: Geometridae). Evol. Ecol. 27, 1217–1233. doi: 10.1007/s10682-013-9657-8
Vanden Broecke, B., Mariën, J., Sabuni, C. A., Mnyone, L., Massawe, A. W., Matthysen, E., et al. (2019). Relationship between population density and viral infection: a role for personality? Ecol. Evol. 9, 10213–10224. doi: 10.1002/ece3.5541
Waldbauer, G. (1968). “The consumption and utilization of food by insects” in Advances in insect physiology. eds. J. W. L. Beament and V. B. Wigglesworth (Amsterdam, Netherlands: Elsevier), 229–288.
Wang, Y., Harrison, R. L., and Shi, J. (2021). Effects of rearing density on developmental traits of two different biotypes of the gypsy moth, Lymantria dispar L., from China and the USA. Insects 12:175. doi: 10.3390/insects12020175
Wang, J., Jiang, X., Wu, D., and Luo, L. (2008). Effect of larval rearing density on development and fecundity of the beet armyworm, Spodoptera exigua (Lepidoptera: Noctuidae). Acta Entomol. Sin. 51, 889–894. doi: 10.16380/j.kcxb.2008.08.013
Wang, Y., Yang, P., Cui, F., and Kang, L. (2013). Altered immunity in crowded locust reduced fungal (Metarhizium anisopliae) pathogenesis. PLoS Pathog. 9:e1003102. doi: 10.1371/journal.ppat.1003102
Williams, I. S. (1999). Slow-growth, high-mortality–a general hypothesis, or is it? Ecol. Entomol. 24, 490–495. doi: 10.1046/j.1365-2311.1999.00217.x
Williams, C. M., Chick, W. D., and Sinclair, B. J. (2015). A cross-seasonal perspective on local adaptation: metabolic plasticity mediates responses to winter in a thermal-generalist moth. Funct. Ecol. 29, 549–561. doi: 10.1111/1365-2435.12360
Wilson, K., Cotter, S. C., Reeson, A. F., and Pell, J. K. (2001). Melanism and disease resistance in insects. Ecol. Lett. 4, 637–649. doi: 10.1046/j.1461-0248.2001.00279.x
Wilson, K., and Graham, R. I. (2015). Transgenerational effects modulate density-dependent prophylactic resistance to viral infection in a lepidopteran pest. Biol. Lett. 11:20150012. doi: 10.1098/rsbl.2015.0012
Wilson, K., and Reeson, A. F. (1998). Density-dependent prophylaxis: evidence from Lepidoptera-baculovirus interactions? Ecol. Entomol. 23, 100–101. doi: 10.1046/j.1365-2311.1998.00107.x
Wilson, K., Thomas, M. B., Blanford, S., Doggett, M., Simpson, S. J., and Moore, S. L. (2002). Coping with crowds: density-dependent disease resistance in desert locusts. Proc. Natl. Acad. Sci. 99, 5471–5475. doi: 10.1073/pnas.082461999
Wu, N., Zhang, S., Li, X., Cao, Y., Liu, X., Wang, Q., et al. (2019). Fall webworm genomes yield insights into rapid adaptation of invasive species. Nat. Ecol. Evol. 3, 105–115. doi: 10.1038/s41559-018-0746-5
Yang, F., Hu, G., Shi, J. J., and Zhai, B. P. (2015). Effects of larval density and food stress on life-history traits of Cnaphalocrocis medinalis (Lepidoptera: Pyralidae). J. Appl. Entomol. 139, 370–380. doi: 10.1111/jen.12179
Yang, Z. Q., Wang, X. Y., Wei, J. R., Qu, H. R., and Qiao, X. R. (2008). Survey of the native insect natural enemies of Hyphantria cunea (Drury) (Lepidoptera: Arctiidae) in China. Bull. Entomol. Res. 98, 293–302. doi: 10.1017/S0007485308005609
Yu, E. Y., Gassmann, A. J., and Sappington, T. W. (2019). Effects of larval density on dispersal and fecundity of western corn rootworm, Diabrotica virgifera virgifera LeConte (Coleoptera: Chrysomelidae). PLoS One 14:e0212696. doi: 10.1371/journal.pone.0212696
Keywords: Hyphantria cunea, development, rearing density, density-dependent effects, ecological strategies
Citation: Zhao X, Geng Y, Hu T, Xie C, Xu W, Zuo Z, Xue M and Hao D (2023) Ecological strategies of Hyphantria cunea (Lepidoptera: Arctiidae) response to different larval densities. Front. Ecol. Evol. 11:1177029. doi: 10.3389/fevo.2023.1177029
Edited by:
Chuanwang Cao, Northeast Forestry University, ChinaReviewed by:
Jian-Rong Wei, Hebei University, ChinaXiujun Wen, South China Agricultural University, China
Guanghong Liang, Fujian Agriculture and Forestry University, China
Copyright © 2023 Zhao, Geng, Hu, Xie, Xu, Zuo, Xue and Hao. This is an open-access article distributed under the terms of the Creative Commons Attribution License (CC BY). The use, distribution or reproduction in other forums is permitted, provided the original author(s) and the copyright owner(s) are credited and that the original publication in this journal is cited, in accordance with accepted academic practice. No use, distribution or reproduction is permitted which does not comply with these terms.
*Correspondence: DeJun Hao, ZGpoYW9AbmpmdS5lZHUuY24=