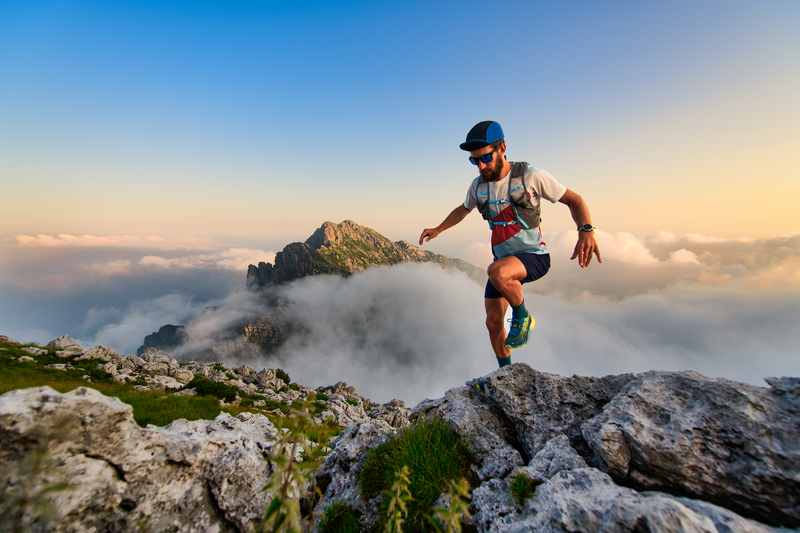
95% of researchers rate our articles as excellent or good
Learn more about the work of our research integrity team to safeguard the quality of each article we publish.
Find out more
ORIGINAL RESEARCH article
Front. Ecol. Evol. , 08 June 2023
Sec. Chemical Ecology
Volume 11 - 2023 | https://doi.org/10.3389/fevo.2023.1175205
This article is part of the Research Topic Chemical Ecology of Plant Arthropod Interactions: How do Herbivorous Arthropods Cope with Defence Chemicals of Their Host Plants? View all 7 articles
Milkweed–herbivore systems are characterized by cardenolide chemical defenses and specialized herbivore adaptations such as physiological target site insensitivity. Cardenolide defenses in milkweeds can vary in terms of the total concentration, differences in the polarity of individual cardenolides, and the substitution of the steroidal structures that can contribute to the molecule's reactivity. The variability in cardenolide defenses could represent the plant's response to natural selection and adaptation of resistant herbivores and is a characteristic of phenotype-matching between defensive and offensive traits resulting from coevolution. Here, we test the phenotypic match of the cardenolide composition of seeds of Asclepias curassavica and those sequestered by nymphs and adults of the specialized seed herbivore Oncopeltus fasciatus, combined with tests of the inhibitory capacity of a subset of seed cardenolides against the Na+/K+-ATPase of O. fasciatus and a non-adapted insect (Drosophila melanogaster). We compare this with the inhibitory capacity against the highly sensitive porcine Na+/K+-ATPase. Among the five most abundant cardenolides present in milkweed seeds, glucopyranosyl frugoside, glucopyranosyl gofruside, and glucopyranosyl calotropin were significantly more abundant in the seeds than in the adults and nymphs; the bugs contained higher concentrations of the deglucosylated compounds. The most abundant compound, glucopyranosyl frugoside, was also the most inhibitory for O. fasciatus, but O. fasciatus was significantly more tolerant to all compounds compared to D. melanogaster and the highly sensitive porcine enzyme. Our results add to the evidence that O. fasciatus sequesters specific individual cardenolides from its Asclepias host plants that are not directly linked to the concentration and inhibitory potency.
Insects can impose natural selection for chemical defenses in the plant tissues on which they feed, leading to phenotype-matching between defensive and offensive traits that may have resulted from coevolution (Futuyma and Agrawal, 2009). Such biochemical coevolutionary arms races (Ehrlich and Raven, 1964) can drive evolutionary innovation and diversification (Mauricio and Rausher, 1997; Berenbaum and Zangerl, 1998; Zangerl and Berenbaum, 2003; Agrawal, 2005; Benderoth et al., 2006; Prasad et al., 2012). For example, the evolution of novel genes for glucosinolate detoxification in Pierid butterflies has been accompanied by duplication and neofunctionalization of defensive glucosinolate genes in Brassicaceae host plant species (Wheat et al., 2007; Sønderby et al., 2010; Edger et al., 2015; BlaŽević et al., 2020). Explaining the diversity of plant secondary metabolites and insect herbivore tolerance mechanisms observed in nature is of interest to evolutionary and chemical ecologists who seek to understand the origins of phytochemical diversity and the scope for coevolution in a community context (Berenbaum et al., 1991; Jones and Firn, 1991; Berenbaum and Zangerl, 1996; Richards et al., 2015).
Many plants simultaneously produce multiple secondary metabolites of the same class (Dyer et al., 2018). One explanation for this is that across populations, different herbivores can select for specific defensive traits (Ayres et al., 1997; Züst et al., 2012), and individual plant compounds are targeted at distinct herbivores (i.e., compound selectivity). For example, multiple specialized herbivores of milkweed plants (Asclepias spp.) each have well-characterized genetically based physiological differences in tolerance to the overall concentration of the plant's toxic cardenolides (Dobler et al., 2012; Karageorgi et al., 2019). Cardenolides are a structurally diverse group of compounds that are found in at least 12 plant families (Agrawal et al., 2012). They consist of a steroid backbone, an unsaturated lactone, and glycoside moieties (Malcolm, 1991). Single species of Asclepias can contain up to 21 different cardenolides, and both compound diversity and concentration are variable among tissues eaten by insects (Abe et al., 1992; Warashina et al., 2008; Opitz and Müller, 2009; Zhang et al., 2014). These host plant cardenolides are selectively sequestered by insect herbivores. For example, the seed bug Oncopeltus fasciatus often sequesters intermediate and more polar cardenolides, even if reared on different host plants that have distinct chemical profiles (Moore and Scudder, 1985). The bug's cardenolide profiles often differ from the cardenolide profile of the host plants (Duffey and Scudder, 1974; Isman et al., 1977; Scudder et al., 1986), which can be explained by the metabolism of some cardenolides into distinct products (Agrawal et al., 2022). Studies that investigate the functional link between the specific defense compounds and their impacts on herbivores will advance our understanding of how the diversity of the same secondary metabolite compound arises, and why plants repeatedly derivatize the same class of compounds (Whitehead et al., 2022).
Recently, the interactions between particular toxic cardenolide heart poisons and insect target sites have begun to be identified (Petschenka et al., 2018; Agrawal et al., 2022; López-Goldar et al., 2022). Agrawal et al. (2022) found that the dominant seed cardenolide in Asclepias syriaca (glycosylated aspecioside) is more inhibitory to the target site of the seed herbivore than its sequestered conversion product (aspecioside A) and that the subdominant seed cardenolides—diglycosylated syriogenin and labriformin—were significantly more inhibitory than the more abundant compounds. The differential accumulation of the compounds could be evidence that they are under selection, especially if this is correlated to geographical areas that vary in insect diversity and herbivory. Further studies on the specificity in chemical defense toward particular resistant enzymes will increase our understanding of milkweed–herbivore coevolution and more broadly phytochemical diversity.
Here, we study the specific interaction of cardenolide toxins from Asclepias curassavica plants and insect Na+/K+-ATPases. We compare the cardenolide composition of seeds of Asclepias curassavica to those sequestered by nymphs and adults of the specialized seed herbivore Oncopeltus fasciatus. We combine this with tests of the inhibitory capacity of a subset of the seed cardenolides against the Na+/K+-ATPase of Oncopeltus and a non-adapted insect (Drosophila melanogaster). The cardenolides can be differentiated by the presence of a hydroxyl group (coroglaucigenin) or a carbonyl group (corotoxigenin/calotropagenin) at the C-19 position of the steroidal structure (see Figure 1 and Rubiano-Buitrago et al., 2023). The native range of A. curassavica is South America and Mexico, and it has spread to California, Florida, Tennessee, and Texas. A. curassavica is eaten by several specialist herbivores including true bugs, from the family Lygaeidae. A. curassavica is present in the migration range of milkweed bugs (Miller and Dingle, 1982; United States Department of Agriculture, 2020). The bugs feed on the seeds (Burdfield-Steel and Shuker, 2014) and sequester cardenolides present in the seeds for their own defense (Scudder and Meredith, 1982; Moore and Scudder, 1985). O. fasciatus is adapted to feed on Asclepias due to specific amino acid substitutions in the physiological target—the Na+/K+-ATPases—which reduce the insect's sensitivity (Bramer et al., 2015). We aimed to answer the following question in the context of milkweed-herbivore interactions: Do milkweed bugs sequester cardenolides according to the availability in the seeds or according to the inhibitory capacity of the cardenolides on the bugs' Na+/K+-ATPase?
Figure 1. Structures of the cardiac glycosides used in this study: glucopyranosyl frugoside, glucopyranosyl calotropin, frugoside, gofruside, and ouabain. Structural features that differentiate the three types of steroidal structure are drawn in red and include functional groups at C-19, a dioxane ring in C-2, C-3, and the ring B of the steroidal core.
Here, we compared the quantification of 10 seed cardenolides sequestered by nymphs and adults of the large milkweed bug Oncopeltus fasciatus (see methods in Rubiano-Buitrago et al., 2023). These cardenolides are: 3-O-β-allopyranosyl coroglaucigenin, 3-[4′-O-β-glucopyranosyl-β-allopyranosyl] coroglaucigenin, 3′-O-β-glucopyranosyl-15- β-hydroxycalotropin, 3-O-β-glucopyranosyl-12-β-hydroxyl coroglaucigenin, 4′-O-β- glucopyranosyl frugoside, 4′-O-β-glucopyranosyl gofruside, 3′-O-β-glucopyranosyl calotropin, frugoside, gofruside, and 16α-hydroxycalotropin.
To assess the sequestration behavior of O. fasciatus, we used bugs that originated from a long-term laboratory colony (originally from the United States) maintained on sunflower seeds. We reared the bugs in terrarium boxes (37 cm × 22 cm × 25 cm) lined with tissue paper and provided them with ad libitum water in Eppendorf tubes plugged with dental cotton, and ad libitum Asclepias curassavica seeds as a food source. The colonies of O. fasciatus were kept in an incubator (Polyklima PK 520-LED) at 70% humidity with a day/night cycle of (18:6) and temperatures of 28°C during the day and 18°C at night. O. fasciatus nymphs develop through five instars (N1–N5), after which they molt into adults. We collected three groups of adults and three groups of N5 nymphs from the experimental colonies and froze them at −80°C.
We extracted cardenolides from the three pools of adults and three pools of N5 nymphs. Each pool weighed ~700 mg. We ground the frozen material and exhaustively extracted cardenolides using a 1:1 MeOH/H2O three times (MeOH ROTISOLV 99.9%, Carl Roth GmbH, Karlsruhe, Germany, water was HPLC grade, deionized with a Merck Millipore Milli-Q A10, Merck KgA, Darmstadt, Germany), followed by two more times with 100% MeOH. The extracts were pooled and filtered through an MN HR-X 500 mg cartridge and washed with MeOH to remove lipophilic substances. The samples were dried using N2 gas at 36°C.
We obtained three nymph extracts of 85.2, 115.1, and 121.0 mg, and three adult extracts of 86.0, 92.0, and 81.9 mg. From each replicate, we prepared a 2.6 mg/mL solution for high-resolution mass spectrometry (HPLC-HRMS). We injected 5 μL of each replicate three times, resulting in nine data points per compound. The high-performance liquid chromatography coupled with high-resolution mass spectrometry (HPLC-HRMS) was achieved using an Agilent 1,260 Infinity, with a reversed-phase Agilent Poroshell 120 column (2.7 μm particle size, 4.6 × 50 mm). The mobile phase consisted of acetonitrile (ACN, supplied with 0.1% formic acid, FA, Carl Roth GmbH, Karlsruhe, Germany) and water (HPLC grade, 0.1% FA, deionized with a Merck Millipore Milli-Q A10, Merck KgA, Darmstadt, Germany). The elution gradient started with ACN/H2O (5:95) for 1 min, then to ACN/H2O (95:5) for 8 min, which was maintained for 2 min. Later, it was set back to ACN/H2O (5:95) for 1 min. High-resolution mass spectra were recorded on a Bruker Compact OTOF spectrometer (Bruker Daltonics GmbH, Bremen, Germany). Electrospray ionization (ESI) in positive ion mode was used for the analysis in full scan and auto MS/MS modes, scanning masses from m/z 50–1,300. Sodium formate adducts were used for internal calibration HPC mode. Bruker Compass ver.1.9 (OTOF Control ver.5.1.107 and HyStar 4.1.31.1) was used for data acquisition and instrument control, and Bruker DataAnalysis ver. 5.1.201 was used for data processing.
We processed the data by extracting the ion chromatogram per compound and measuring the corresponding peak area. The concentration per injection was calculated as mg of cardenolide per g of dry weight.
Na+/K+-ATPase extractions from the cardenolide-resistant seed bug (Oncopeltus fasciatus; Bramer et al., 2015; Agrawal et al., 2022; López-Goldar et al., 2022) and one non-adapted insect (Drosophila melanogaster wild type Canton S) (Dalla and Dobler, 2016; Dobler et al., 2019) were prepared according to the methods described in Petschenka et al. (2013, 2018, 2022). Fruit flies were reared under controlled conditions, and O. fasciatus were reared in terrarium boxes as described in section Cardenolides from Asclepias curassavica seeds and sequestration except that for this analysis, O. fasciatus adults were reared on sunflower seeds (Helianthus annuus).
Briefly, the brains and thoracic ganglia of individual O. fasciatus (killed and stored at −80°C) were dissected under deionized water. We pooled the brains of six individuals (following Bramer et al., 2015) and homogenized them in 500 μL of deionized water using an all glass 1 mL Wheaton grinder. For D. melanogaster, we dissected the heads of 90 individuals and homogenized them in 900 μL of deionized water and then split them into three 300 μL aliquots (following Taverner et al., 2019). All extracts were frozen, lyophilized, and stored at −80°C until use. Prior to the assays, the insect lyophilisates were reconstituted by adding 200 μL water (O. fasciatus) or 600 μL (D. melanogaster) followed by vortex and incubation for 10 min in a chilled ultrasonic bath. Porcine lyophilized Na+/K+-ATPase was dissolved in water to a concentration of 1 U/ml, stored at −80°C in single-use aliquots, and diluted with H2O to 0.05 U/ml for use in the in vitro assay (final concentration in the assay, 0.01 U/ml).
All cardiac glycosides (Figure 1) were tested with at least two biological replicates of Na+/K+-ATPase (i.e., Na+/K+-ATPase from genetically different specimens or pools of specimens). Reactions were performed in 96-well microplates to which the Na+/K+-ATPase protein was added in an eight-well row containing stabilizing buffers (see formulas in Petschenka et al., 2023). The first six wells in the eight-well row were exposed to exponentially decreasing concentrations of cardenolide (10−3, 10−4, 10−5, 10−6, 10−7, and 10−8 M). The seventh well was 2% DMSO (as an experimental control), and the eighth well was exposed to a combination of inhibition buffers lacking KCl but supplemented with 10−3 M cardenolide. This allows the measurement of background ATP hydrolysis. The proteins were incubated on a BioShake Iq microplate shaker (Quantifoil Instruments) at 200 rpm and 37°C for 10 min after which ATP (Sigma Aldrich, A9062-1G) was added to each well and then incubated at 200 rpm and 37°C for further 20 min. The Na+/K+-ATPase activity was measured as the amount of inorganic phosphate enzymatically released from ATP in the presence of K+ (Na+/K+-ATPase active) minus the amount of phosphate released in the absence of K+ (Na+/K+-ATPase inactive). The activity was quantified photometrically at 700 nm with a microplate reader (BMG Clariostar) following (Taussky and Shorr, 1953). Background phosphate absorbance levels from the reactions with inhibiting factors were used to calibrate phosphate absorbance in wells measuring cardenolide inhibition and in the control wells (Petschenka et al., 2013). For each cardenolide, we carried out two technical replicates, making each data point an average of four to six measurements (Petschenka et al., 2012, 2013; Taverner et al., 2019).
To compare the concentration of individual cardenolides available in the seeds to those sequestered by adults and nymphs we log-transformed the concentration data and analyzed the transformed data using analysis of variance. For glucopyranosyl frugoside and glucopyranosyl calotropin, the transformation did not improve the fit of the data for parametric tests, thus we compared them using the Wilcoxon rank test with a continuity correction. We did not analyze data where the bugs had not sequestered compounds (glucopyranosyl hydroxycalotropin and glucopyranosyl allopyranosyl coroglaucigenin) or where only the adult or the nymph (not both) had sequestered (allopyranosyl coroglaucigenin and glucopyranosyl gofruside).
For cardenolide sensitivity measurements, calibrated absorbance values were converted to the percentage of non-inhibited Na+/K+-ATPases activity based on measurements from the control wells. These data were plotted, and log10 of the IC50 values was calculated for each biological replicate from non-linear fitting using a four-parameter logistic curve, with the top and bottom asymptotes set to 100 and zero, respectively. We fitted the curves using the nlsLM function of the minipack.lm library in R (R Core Team, 2022) (Supplementary Figure 3 and Supplementary Table 1). We compared the log10 IC50 values of individual cardenolides for each Na+/K+-ATPase enzyme using the analysis of variance, with Tukey's HSD pairwise contrasts. We calculated the fold differences between the IC50 values of individual cardenolides for non-adapted and adapted insect Na+/K+-ATPase and the porcine Na+/K+-ATPase. To calculate the effects of individual cardiac glycosides on different forms of Na+/K+-ATPase (adapted or non-adapted), we compared the inhibition to a reference cardenolide by calculating the log10 of the ratio (IC50 reference compound)/(IC50 test compound). We used ouabain (Sigma Aldrich, O3125-1G) as a reference as it is the most widely used cardenolide in research on Na+/K+-ATPase (Petschenka et al., 2018). Here, negative values represent compounds that inhibit the Na+/K+-ATPase more weakly than ouabain, and positive values represent compounds that inhibit the Na+/K+-ATPase more strongly than ouabain. We also calculated this ratio using glucopyranosyl frugoside as the reference, because it is the most abundant cardenolide in the seeds (Rubiano-Buitrago et al., 2023).
All analyses were conducted in R (version 1.4.1717).
We found significant differences among the concentration of cardenolides available in the seeds of A. curassavica and those sequestered by nymphs and adults of O. fasciatus (see Supplementary Table 2). Among the five most abundant cardenolides present in milkweed seeds (accounting for 91% of the total), glucopyranosyl frugoside, glucopyranosyl gofruside, and glucopyranosyl calotropin were significantly more abundant in the seeds than the adults and nymphs. Frugoside and gofruside were significantly more dominant in the adults and nymphs than in the seeds (Figure 2 and Supplementary Figures 1, 2). Of the minor compounds, the adults and nymphs sequestered significantly more glucopyranosyl-12β-hydroxyl coroglaucigenin than was present in the seeds, but significantly less 16α-hydroxycalotropin. Neither adults nor nymphs sequestered glucopyranosyl-15β-hydroxycalotropin or glucopyranosyl-allopyranosyl coroglaucigenin that were present in the seeds. Adult bugs, but not nymphs, sequestered allopyranosyl coroglaucigenin and glucopyranosyl gofruside from the seeds. There were eight other cardenolides in the seeds that we did not identify and that the bugs did not sequester. There were also 14 cardenolides in the LC-HRMS data of O. fasciatus that did not have the retention time or the fragmentation pattern of our purified cardenolide reference obtained from the seeds, and that were also absent from the spectrometric data of the seeds. Of these 14 compounds, seven were present in both adults and nymphs, five were only found in the nymphs, and two were only found in the adults (see Supplementary Table 3).
Figure 2. Concentration of cardenolides in A. curassavica seeds (black) and sequestered by large milkweed bugs (O. fasciatus; whole adults extracted, orange; nymphs, blue; N = 9 per compound), shown as means ± SE quantified by LC-HRMS. The compounds are arranged from most polar on the left to non-polar on the right. The numbers in square brackets correspond with compound identification from Rubiano-Buitrago et al. (2023). Note the y-axis is on a log scale. Images: Oncopeltus nymph (Veit Grabe), Oncopeltus adult (Jena Johnson; The Scientist), seeds (Francesca Protti-Sánchez).
The compounds tested (glucopyranosyl frugoside, glucopyranosyl calotropin, frugoside, gofruside, and ouabain, Figure 3) had significantly different effects on the O. fasciatus Na+/K+-ATPase enzyme (ANOVA: F(4,17) = 9.70, p = 0.0003). Glucopyranosyl frugoside was four times more inhibitory than ouabain (Tukey's HSD: estimate = −0.68 ± 1.19, p = 0.002). Frugoside was 3.5 times more inhibitory than ouabain (Tukey's HSD: estimate = −0.64 ± 1.13, p = 0.007). Glucopyranosyl calotropin and gofruside did not significantly differ from ouabain in inhibitory capacity (Tukey's HSD: estimate = −0.165 ± 0.65, p = 0.84; estimate = −0.01 ± 0.51, p = 0.99).
Figure 3. Molar concentration of plant toxin necessary to cause 50% inhibition of the O. fasciatus enzyme (blue), non-adapted insect (D.melanogaster, green), and a vertebrate reference (S. domesticus, purple) Na+/K+-ATPases, or IC50 of the dominant seed cardenolide (glucopyranosyl frugoside) and the subdominant compounds, all of which are sequestered, shown as means ± SE, N = 4–6 per compound). Higher values on the y-axis indicate that the enzyme is more tolerant to the cardenolide.
For the sensitive invertebrate D. melanogaster, we also found a significant difference between the compounds on the IC50 values (ANOVA: F(4,23) = 8.04, p = 0.0003). Glucopyranosyl frugoside was 20 times more inhibitory than ouabain (Tukey's HSD: estimate = −1.21 ± 2.14, p = 0.007). Gofruside was 17 times more inhibitory than ouabain (Tukey's HSD: estimate = −1.43 ± 2.36, p = 0.001), and glucocalotropin was 12 times more inhibitory than ouabain (Tukey's HSD: estimate = −1.40 ± 2.33, p = 0.002). Frugoside did not differ significantly from ouabain (fold difference = 3; Tukey's HSD: estimate = −0.51 ± 1.46, p = 0.5). We also found a significant variation in the inhibitory capacity of the compounds on the highly sensitive porcine enzyme (ANOVA: F(4,25) = 49.63, p < 0.0001). Glucopyranosyl frugoside was five times less inhibitory than ouabain (Tukey's HSD: estimate = 0.72 ± 0.37, p = 0.00002), and glucopyranosyl calotropin was 1.5 times less inhibitory (Tukey's HSD: estimate = 0.27 ± 0.08, p = 0.18), whereas gofruside was 6.5 times more inhibitory (Tukey's HSD: estimate = −0.88 ± 1.23, p = 0.00007). Frugoside was not significantly different from ouabain in inhibitory activity (fold difference = 1.3, Tukey's HSD: estimate = −0.13 ± 0.48, p = 0.78).
Frugoside caused a unidirectional effect on the three enzymes compared to ouabain, whereas glucopyranosyl calotropin, glucopyranosyl frugoside, and gofruside produced countervailing effects (Figure 4). Glucopyranosyl calotropin and glucopyranosyl frugoside were stronger than ouabain on the insect enzymes but weaker than ouabain on the porcine Na+/K+-ATPases. Gofruside had almost equal to lower effect than ouabain for O. fasciatus but was more inhibitory for the sensitive species. Using glucopyranosyl frugoside as a reference in place of ouabain shifted the direction of the effects (Supplementary Figure 4).
Figure 4. Selective effects of individual cardiac glycosides on different forms of Na+/K+-ATPase (O. fasciatus, blue; non-adapted D. melanogaster, green, and S. domesticus, pink). Relative inhibition values are based on IC50 values compared with the standard ouabain (computed as the log10 of the ratio (IC50 reference compound)/(IC50 test compound). Inhibition weaker than ouabain is indicated by negative values, while inhibition greater than ouabain is indicated by positive values.
We found no significant relationship between concentration in the seeds and inhibitory effects of cardenolides across the insect enzymes (Pearson's correlations: D. melanogaster R = −0.32, p = 0.68; O. fasciatus R = −0.66, p = 0.34), but there was a trend toward a decrease in inhibitory capacity with increasing concentration for the porcine enzyme (R = 0.97, p = 0.026) (Supplementary Figure 5).
We quantified the cardenolides sequestered by the specialized milkweed herbivore Oncopeltus fasciatus across two stages of its life cycle when reared on seeds of Asclepias curassavica. We combined the identification and quantification of sequestered cardenolides with tests of their inhibitory capacity of the Na+/K+-ATPase. Among the five most abundant cardenolides present in milkweed seeds, frugoside and gofruside were more concentrated in the bugs than the seeds. These two compounds had contrasting potency toward the O. fasciatus enzyme–frugoside (and its glucosylated counterpart), which was the most inhibitory, whereas gofruside was among the weakest inhibitors. When comparing the inhibitory capacity of all the compounds to a non-adapted insect Drosophila melanogaster and the highly sensitive porcine Na+/K+-ATPase, we found that the compounds varied less in their inhibition of the O. fasciatus enzyme compared to the non-adapted insect enzyme. The finding that there is differential accumulation of A. curassavica seed cardenolides by O. fasciatus adds to the evidence that the bug sequesters particular, but not all, cardenolides from its Asclepias hostplants (Duffey et al., 1978; Scudder and Meredith, 1982; Meredith et al., 1984).
Milkweed bugs reared on seeds of A. curassavica sequestered two of the more non-polar cardenolides available in the seeds (frugoside and gofruside), which is in line with the preferential sequestration of the non-polar cardenolide digitoxin in the fat body of adult O. fasciatus reported by Duffey et al. (1978). The fat body often accumulates non-polar compounds owing to the lipophilic nature of such compounds (Kilby, 1963). O. fasciatus in our study did not sequester several compounds that are present in the seeds, which is also in line with the results of Moore and Scudder (1985) who showed that O. fasciatus sequestered most, but not all, of the cardenolides in seeds of A. speciosa (9 of 13 cardenolides sequestered) and A. syriaca (6 of 8 cardenolides sequestered). We found 14 compounds in the bugs that did not have the retention time or the fragmentation pattern of cardenolides from the seeds. A similar sequestration behavior has also been reported by Moore and Scudder (1985) who found that O. fasciatus contained 12 cardenolides that were not present in A. speciosa seeds, and 8 cardenolides that were not present in A. syriaca seeds. Our results could indicate that O. fasciatus concentrated some compounds that have such low abundance in the seeds that they were not detectable by spectrometric measurements or could indicate the metabolism of cardenolides, which is known to occur when O. fasciatus is reared on A. syriaca (Agrawal et al., 2022). The higher concentration of frugoside and gofruside in the bugs than in seeds could indicate that O. fasciatus cleaves the sugar from the glucosylated compounds during sequestration. To verify this, the insects could be reared on artificial diets containing these isolated cardenolides and the sequestration products could then be quantified (e.g., Pokharel et al., 2021; Agrawal et al., 2022).
Until recently the Na+/K+-ATPase of O. fasciatus has been studied almost exclusively with reference to ouabain (though see: Herbertz et al., 2020; Agrawal et al., 2022; López-Goldar et al., 2022). The main result from our tests of four compounds of A. curassavica is that glucopyranosyl frugoside and frugoside have potency against O. fasciatus, which has a highly adapted sodium pump characterized by gene duplications and substitutions (Bramer et al., 2015; Lohr et al., 2017). Phytochemical profiles of the aerial parts and roots of A. curassavica have no reports of glucopyranosyl frugoside being isolated, which could suggest it is a compound specific to the seeds and, given its inhibitory capacity, under selection by the seed bugs. Frugoside, on the other hand, is well distributed across the plant's tissues (Roy et al., 2005; Warashina and Noro, 2008; Warashina et al., 2008; Li et al., 2009; Al-Snafi, 2015; Ji et al., 2022). We did not test these compounds on other adapted herbivores, such as Monarch butterflies (Danaus plexippus), and so our data do not allow us to draw specific conclusions about Oncopeltus–milkweed coevolution (Berenbaum and Zangerl, 1996), but open up a path for future research on toxin–receptor interactions.
Our results are in contrast with those of Agrawal et al. (2022), who found that the dominant cardenolide in seeds of Asclepias syriaca was a less potent inhibitor of the O. fasciatus Na+/K+-ATPase than a minor compound, labriformin, that was the most potent inhibitor. Labriformin is characterized by nitrogen- and sulfur-containing thiazolidine ring moiety, which was not present in any of the major cardenolides isolated in A. curassavica seeds, but is known to be present in the leaves (Agrawal et al., 2022). Cardenolides with a thiazolidine ring are predicted to interact with the binding site of O. fasciatus Na+/K+-ATPase in in silico molecular docking simulations (Ramos et al., 2021), which likely explains why O. fasciatus sequesters the less toxic nitrogen-containing oxidized labriformin (Agrawal et al., 2022). Of our isolated compounds, we might have expected gofruside (which contains an aldehyde moiety as part of their corotoxigenin steroidal structure, Figure 1) and glucopyranosyl calotropin (which contains the same aldehyde group as gofruside and also has a dioxane ring bond in C-2 and C-3, Figure 1) to have been more inhibitory to the Oncopeltus enzyme (Zhang et al., 2014). For example, corotoxigenin cardenolides, like gofruside, are predicted to form a strong hydrophobic pi-sigma interaction between amino acid residue 783 and the steroidal ring B, which is hypothesized to assist the cardenolide-sensitive Na+/K+-ATPase complex (see the porcine model in Meneses-Sagrero et al., 2022). However, gofruside and glucopyranosyl calotropin did not differ in their inhibitory properties compared to ouabain to the O. fasciatus Na+/K+-ATPase. Both cardenolides were less inhibitory than the major compound in the seeds, which have hydroxyl moiety at C-19 (Figure 1). Gofruside and glucopyranosyl calotropin were, however, highly inhibitory to the D. melanogaster enzyme.
Different degrees of oxidation in the C-19 position are predicted to generate a key hydrogen bond between the cardenolide and the enzyme amino acid residue 111, a site that is strongly implicated in cardenolide resistance (Bramer et al., 2015). The hydrogen bonds between amino acid residue 111 and the aldehyde or hydroxyl moieties are predicted to influence the stability of the cardenolide-protein complex (Meneses-Sagrero et al., 2022). Further in silico molecular docking simulations of these compounds against both resistant and sensitive enzymes are needed to clarify the importance of the oxidation in the steroidal C-19 in relation to the amino acid residues that have been implicated in resistance.
Glycosylated cardenolides are generally considered to be more toxic than corresponding genins in whole organism vertebrate assays (Hoch, 1961). Petschenka et al. (2018) found that glycosides were on average 6-fold more potent in their inhibition of insect Na+/K+-ATPases, including the specialized monarch butterfly Na+/K+-ATPase, compared to genins. Agrawal et al. (2022) also found that diglycosylated compounds were highly inhibitory. We found similar inhibition of glucopyranosyl frugoside and frugoside against O. fasciatus, but the deglycosylated version was more inhibitory for the porcine enzyme. Given that we have only one comparison of a glycoside vs. its aglycone, we can tentatively suggest that the effect of the cardiac glycoside sugar moiety on inhibition depends on the specific biochemical properties of the target, the Na+/K+-ATPase enzyme. It is also suggested that the toxicity of cardenolides can increase from polar to non-polar compounds (Petschenka and Dobler, 2009; Rasmann and Agrawal, 2011; Agrawal et al., 2012; López-Goldar et al., 2022). In our analysis of five cardenolides with different polarities and Na+/K+-ATPase pumps from three species, we do not observe a clear trend where polarity is a key factor for the inhibitory response.
Our study, along with Agrawal et al. (2022) and Petschenka et al. (2018), focuses on individual compounds, rather than natural mixtures in which those compounds are encountered in nature (though see López-Goldar et al., 2022). Mixtures of cardenolides may be maintained because of synergistic or additive interactions among compounds (Richards et al., 2016; Whitehead et al., 2021). Mixtures may also be maintained because of antagonism, where the effects of active constituents are masked by other compounds in a complex mixture (Caesar and Cech, 2019). Comparison of the IC50 values for single cardenolides vs. mixtures of cardenolides would be useful for uncovering the combined effects of the compounds and could provide a functional explanation for the repeated derivatization of cardenolides in milkweeds (Whitehead et al., 2021) and the differences in the abundance and inhibitory properties of cardenolides in different species of Asclepias (Agrawal et al., 2022; López-Goldar et al., 2022). Such research could also shed more light on whether there are costs associated with the biosynthesis of different cardenolides (e.g., those with extra glycosylation, or further modifications to the sugar moiety), and the cost-benefit of producing specific cardenolides in relation to differences in insect diversity, herbivore specialization, and the constraints on those herbivores. Although Agrawal et al. (2022) found no significant effects of the most potent inhibitors on O. fasciatus growth and performance, we have recently shown that sequestration can be costly in terms of oxidative state (Blount et al., 2023; Heyworth et al., 2023) which is visible in the wing colors that milkweed herbivores use as anti-predator defenses. More tests on the costs of sequestration will also be important for understanding the evolutionary and ecological interactions between the plant and the herbivore.
The original contributions presented in the study are publicly available. The raw data and R scripts for statistical analysis and data visualisation can be found at: https://edmond.mpdl.mpg.de/dataset.xhtml?persistentId=doi:10.17617/3.SQGWHL.
Conceptualization: HMR, CP, and PR-B. Methodology: PR-B, SP, VG, CP, and HMR. Formal analysis and writing—original draft preparation: HMR and PR-B. Investigation: PR-B and SP. Resources and supervision: HMR and CP. Data curation: PR-B. Writing—review and editing: SP, CP, AA-A, and VG. Visualization: AA-A and PR-B. Project administration and funding acquisition: HMR. All authors have read and agreed to the published version of the manuscript.
PR-B was funded by an International Max Planck Research School Fellowship. SP, AA-A, HMR, and CP are funded by the Max Planck Society.
The authors thank Kati Barthold for caring for the bug colonies; Georg Petschenka, Shab Mohammadi, and Marlene Herbertz for advice on the NKA assays. The authors also thank Evelyn Claußen for administrative support.
The authors declare that the research was conducted in the absence of any commercial or financial relationships that could be construed as a potential conflict of interest.
All claims expressed in this article are solely those of the authors and do not necessarily represent those of their affiliated organizations, or those of the publisher, the editors and the reviewers. Any product that may be evaluated in this article, or claim that may be made by its manufacturer, is not guaranteed or endorsed by the publisher.
The Supplementary Material for this article can be found online at: https://www.frontiersin.org/articles/10.3389/fevo.2023.1175205/full#supplementary-material
Abe, F., Mori, Y., and Yamauchi, T. (1992). Cardenolide glycosides from the seeds of Asclepias curassavica. Chem. Pharm. Bull. 40, 2917–2920. doi: 10.1248/cpb.40.2917
Agrawal, A. A. (2005). Natural selection on common milkweed (Asclepias syriaca) by a community of specialized insect herbivores. Evol. Ecol. Res. 7, 651–667.
Agrawal, A. A., Espinosa del Alba, L., López-Goldar, X., Hastings, A. P., White, R. A., Halitschke, R., et al. (2022). Functional evidence supports adaptive plant chemical defense along a geographical cline. Proc. Natl. Acad. Sci. 119:e2205073119. doi: 10.1073/pnas.2205073119
Agrawal, A. A., Petschenka, G., Bingham, R. A., Weber, M. G., and Rasmann, S. (2012). Toxic cardenolides: chemical ecology and coevolution of specialized plant-herbivore interactions. New Phytol. 194, 28–45. doi: 10.1111/j.1469-8137.2011.04049.x
Al-Snafi, A. E. (2015). Chemical constituents and pharmacological effects of Asclepias Curassavica – a review. Asian J. Pharm. Res. 5, 83–87.
Ayres, M. P., Clausen, T. P., MacLean, S. F., Redman, A. M., and Reichardt, P. B. (1997). Diversity of structure and antiherbivore activity in condensed tannins. Ecology 78, 1696–1712. doi: 10.1890/0012-9658(1997)078[1696:DOSAAA]2.0.CO;2
Benderoth, M., Textor, S., Windsor, A. J., Mitchell-Olds, T., Gershenzon, J., and Kroymann, J. (2006). Positive selection driving diversification in plant secondary metabolism. Proc. Natl. Acad. Sci. USA. 103, 9118–9123. doi: 10.1073/pnas.0601738103
Berenbaum, M. R., Nitao, J. K., and Zangerl, A. R. (1991). Adaptive significance of furanocoumarin diversity in Pastinaca sativa (Apiaceae). J. Chem. Ecol. 17, 207–215. doi: 10.1007/BF00994434
Berenbaum, M. R., and Zangerl, A. R. (1996). “Phytochemical diversity: adaptation or random variation?,” in Phytochemical Diversity and Redundancy in Ecological Interactions, eds. J. T. Romeo, J. A. Saunders, and P. Barbosa (Boston, MA: Springer, Boston, MA), 1–24. doi: 10.1007/978-1-4899-1754-6_1
Berenbaum, M. R., and Zangerl, A. R. (1998). Chemical phenotype matching between a plant and its insect herbivore. Proc. Natl. Acad. Sci. USA. 95, 13743–13748. doi: 10.1073/pnas.95.23.13743
BlaŽević, I., Montaut, S., Burčul, F., Olsen, C. E., Burow, M., Rollin, P., et al. (2020). Glucosinolate structural diversity, identification, chemical synthesis and metabolism in plants. Phytochemistry 169:112100. doi: 10.1016/j.phytochem.2019.112100
Blount, J. D., Rowland, H. M., Mitchell, C., Speed, M. P., Ruxton, G. D., Endler, J. A., et al. (2023). The price of defence: toxins, visual signals and oxidative state in an aposematic butterfly. Proc. R. Soc. B Biol. Sci. 290:20222068. doi: 10.1098/rspb.2022.2068
Bramer, C., Dobler, S., Deckert, J., Stemmer, M., and Petschenka, G. (2015). Na+/K+-ATPase resistance and cardenolide sequestration: Basal adaptations to host plant toxins in the milkweed bugs (Hemiptera: Lygaeidae: Lygaeinae). Proc. R. Soc. B Biol. Sci. 282:20142346. doi: 10.1098/rspb.2014.2346
Burdfield-Steel, E. R., and Shuker, D. M. (2014). The evolutionary ecology of the Lygaeidae. Ecol. Evol. 4, 2278–2301. doi: 10.1002/ece3.1093
Caesar, L. K., and Cech, N. B. (2019). Synergy and antagonism in natural product extracts: when 1 + 1 does not equal 2. Nat. Prod. Rep. 36, 869–888. doi: 10.1039/C9NP00011A
Dalla, S., and Dobler, S. (2016). Gene duplications circumvent trade-offs in enzyme function: insect adaptation to toxic host plants. Evolution, 70, 2767–2777. doi: 10.1111/evo.13077
Dobler, S., Dalla, S., Wagschal, V., and Agrawal, A. A. (2012). Community-wide convergent evolution in insect adaptation to toxic cardenolides by substitutions in the Na+/K+-ATPase. Proc. Natl. Acad. Sci. USA. 109, 13040–13045. doi: 10.1073/pnas.1202111109
Dobler, S., Wagschal, V., Pietsch, N., Dahdouli, N., Meinzer, F., Romey-Glüsing, R., et al. (2019). New ways to acquire resistance: imperfect convergence in insect adaptations to a potent plant toxin. Proc. R. Soc. 286:20190883. doi: 10.1098/rspb.2019.0883
Duffey, S. S., Blum, M. S., Isman, M. B., and Scudder, G. G. E. (1978). Cardiac glycosides: a physical system for their sequestration by the milkweed bug. J. Insect Physiol. 24, 639–645. doi: 10.1016/0022-1910(78)90127-0
Duffey, S. S., and Scudder, G. G. E. (1974). Cardiac glycosides in Oncopeltus fasciatus (Dallas) (Hemiptera: Lygaeidae). I. the uptake and distribution of natural cardenolides in the body. Can. J. Zool. 52, 283–290. doi: 10.1139/z74-035
Dyer, L. A., Philbin, C. S., Ochsenrider, K. M., Richards, L. A., Massad, T. J., Smilanich, A. M., et al. (2018). Modern approaches to study plant–insect interactions in chemical ecology. Nat. Rev. Chem. 2, 50–64. doi: 10.1038/s41570-018-0009-7
Edger, P. P., Heidel-Fischer, H. M., Bekaert, M., Rota, J., Glöckner, G., Platts, A. E., et al. (2015). The butterfly plant arms-race escalated by gene and genome duplications. Proc. Natl. Acad. Sci. USA. 112, 8362–8366. doi: 10.1073/pnas.1503926112
Ehrlich, P. R., and Raven, P. H. (1964). Butterflies and plants: a study in coevolution. Evolution. 18, 586–608. doi: 10.1111/j.1558-5646.1964.tb01674.x
Futuyma, D. J., and Agrawal, A. A. (2009). Macroevolution and the biological diversity of plants and herbivores. Proc. Natl. Acad. Sci. USA. 106, 18054–18061. doi: 10.1073/pnas.0904106106
Herbertz, M., Dalla, S., Wagschal, V., Turjalei, R., Heiser, M., and Dobler, S. (2020). Different combinations of insect Na, K-ATPase α- and α-subunit paralogs enable fine tuning of toxin resistance and enzyme kinetics. bioRxiv [Preprint]. doi: 10.1101/2020.08.28.272054
Heyworth, H. C., Pokharel, P., Blount, J. D., Mitchell, C., Petschenka, G., and Rowland, H. M. (2023). Antioxidant availability trades off with warning signals and toxin sequestration in the large milkweed bug (Oncopeltus fasciatus). Ecol. Evol. 13:e9971. doi: 10.1002/ece3.9971
Hoch, J. H. (1961). A Survey of Cardiac Glycosides and Genins. 1st ed. Charleston, SC: University of South Carolina Press.
Isman, M. B., Duffey, S. S., and Scudder, G. G. E. (1977). Variation in cardenolide content of the lygaeid bugs, Oncopeltus fasciatus and Lygaeus kalmii kalmii and of their milkweed hosts (Asclepias spp.) in central California. J. Chem. Ecol. 3, 613–624. doi: 10.1007/BF00988061
Ji, A. -J., Ma, Q., Kong, M. -Y., Li, L. -Y., Chen, X. -L., Liu, Z. -Q., et al. (2022). Two cardenolide glycosides from the seed fairs of Asclepias curassavica and their cytotoxic activities. Chin. J. Nat. Med. 20, 202–209. doi: 10.1016/S1875-5364(21)60098-5
Jones, C. G., and Firn, R. D. (1991). On the evolution of plant secondary chemical diversity. Philos. Trans.-R. Soc. 333, 273–280. doi: 10.1098/rstb.1991.0077
Karageorgi, M., Groen, S. C., Sumbul, F., Pelaez, J. N., Verster, K. I., Aguilar, J. M., et al. (2019). Genome editing retraces the evolution of toxin resistance in the monarch butterfly. Nature 574, 409. doi: 10.1038/s41586-019-1610-8
Kilby, B. A. (1963). “The Biochemistry of the Insect Fat Body,” in Advances in Physiology, eds. J. W. L. Beament, J. E. Treherne, and V. B. Wigglesworth, (London: Academic Press), 111–174. doi: 10.1016/S0065-2806(08)60175-7
Li, J. -Z., Qing, C., Chen, C. -X., Hao, X. -J., and Liu, H. -Y. (2009). Cytotoxicity of cardenolides and cardenolide glycosides from Asclepias curassavica. Bioorg. Med. Chem. Lett. 19, 1956–1959. doi: 10.1016/j.bmcl.2009.02.045
Lohr, J. N., Meinzer, F., Dalla, S., Romey-Glüsing, R., and Dobler, S. (2017). The function and evolutionary significance of a triplicated Na+/K+-ATPase gene in a toxin-specialized insect. BMC Evol. Biol. 17, 256. doi: 10.1186/s12862-017-1097-6
López-Goldar, X., Hastings, A., Züst, T., and Agrawal, A. (2022). Evidence for tissue-specific defence-offence interactions between milkweed and its community of specialized herbivores. Mol. Ecol. 31, 3254–3265. doi: 10.1111/mec.16450
Malcolm, S. B. (1991). “Cardenolide-mediated interactions between plants and herbivores,” in Herbivores: Their Interactions With Secondary Plant Metabolites (Elsevier), 251–296. doi: 10.1016/B978-0-12-597183-6.50012-7
Mauricio, R., and Rausher, M. D. (1997). Experimental manipulation of putative selective agents provides evidence for the role of natural enemies in the evolution of plant defense. Evolution. 51, 1435–1444. doi: 10.1111/j.1558-5646.1997.tb01467.x
Meneses-Sagrero, S. E., Rascón-Valenzuela, L. A., García-Ramos, J. C., Vilegas, W., Arvizu-Flores, A. A., Sotelo-Mundo, R. R., et al. (2022). Calotropin and corotoxigenin 3-O-glucopyranoside from the desert milkweed Asclepias subulata inhibit the Na+/K+-ATPase activity. PeerJ. 10:e13524. doi: 10.7717/peerj.13524
Meredith, J., Moore, L., and Scudder, G. G. E. (1984). Excretion of ouabain by Malpighian tubules of Oncopeltus fasciatus. Am. J. Physiol. 15:R705–15. doi: 10.1152/ajpregu.1984.246.5.R705
Miller, E. R., and Dingle, H. (1982). The effect of host plant phenology on reproduction of the milkweed bug, Oncopeltus fasciatus, in tropical Florida. Oecologia 52, 97–103. doi: 10.1007/BF00349016
Moore, L. V., and Scudder, G. G. E. (1985). Selective sequestration of milkweed (Asclepias sp.) cardenolides in Oncopeltus fasciatus (Dallas) (Hemiptera: Lygaeidae). J. Chem. Ecol. 11, 667–687. doi: 10.1007/BF00988575
Opitz, S. E. W., and Müller, C. (2009). Plant chemistry and insect sequestration. Chemoecology 19, 117–154. doi: 10.1007/s00049-009-0018-6
Petschenka, G., and Dobler, S. (2009). Target-site sensitivity in a specialized herbivore towards major toxic compounds of its host plant: The Na+/K+-ATPase of the oleander hawk moth (Daphnis nerii) is highly susceptible to cardenolides. Chemoecology 19, 235–239. doi: 10.1007/s00049-009-0025-7
Petschenka, G., Fandrich, S., Sander, N., Wagschal, V., Boppré, M., and Dobler, S. (2013). Stepwise evolution of resistance to toxic cardenolides via genetic substitutions in the Na+/K+-ATPase of milkweed butterflies (lepidoptera: Danaini). Evolution. 67, 2753–2761. doi: 10.1111/evo.12152
Petschenka, G., Fei, C. S., Araya, J. J., Schröder, S., Timmermann, B. N., and Agrawal, A. A. (2018). Relative selectivity of plant cardenolides for Na+/K+-ATPases from the Monarch Butterfly and non-resistant insects. Front. Plant Sci. 9:1424. doi: 10.3389/fpls.2018.01424
Petschenka, G., Halitschke, R., Züst, T., Roth, A., Stiehler, S., Tenbusch, L., et al. (2022). Sequestration of defenses against predators drives specialized host plant associations in preadapted milkweed bugs (Heteroptera: Lygaeinae). Am. Nat. 199, E211–E228. doi: 10.1086/719196
Petschenka, G., Offe, J. K., and Dobler, S. (2012). Physiological screening for target site insensitivity and localization of Na+/K+-ATPase in cardenolide-adapted Lepidoptera. J. Insect Physiol. 58, 607–612. doi: 10.1016/j.jinsphys.2011.12.012
Petschenka, G., Züst, T., Hastings, A. P., Agrawal, A. A., and Jander, G. (2023). “Quantification of plant cardenolides by HPLC, measurement of Na+/K+-ATPase inhibition activity, and characterization of target enzymes,” in Methods in Enzymology (Cambridge, MA: Academic Press), 680, 275–302. doi: 10.1016/bs.mie.2022.08.003
Pokharel, P., Steppuhn, A., and Petschenka, G. (2021). Dietary cardenolides enhance growth and change the direction of the fecundity-longevity trade-off in Milkweed Bugs (Heteroptera: Lygaeinae). Ecol. Evol. 11, 18042–18054. doi: 10.1002/ece3.8402
Prasad, K. V. S. K., Song, B. H., Olson-Manning, C., Anderson, J. T., Lee, C. R., Schranz, M. E., et al. (2012). A gain of function polymorphism controlling complex traits and fitness in nature. Science. 337, 1081–1084. doi: 10.1126/science.0.1221636
R Core Team (2022). R: A Language and Environment for Statistical Computing. Vienna, Austria: R Foundation for Statistical Computing. Available online at: https://www.r-project.org/
Ramos, M. V., Freitas, L. B. N., Bezerra, E. A., Morais, F. S., Lima, J. P. M. S., Souza, P. F. N., et al. (2021). Structural Analysis Revealed the Interaction of Cardenolides from Calotropis procera with Na+/K+ ATPases from Herbivores. Protein Pept. Lett. 29, 89–101. doi: 10.2174/0929866528666211207111011
Rasmann, S., and Agrawal, A. A. (2011). Latitudinal patterns in plant defense: evolution of cardenolides, their toxicity and induction following herbivory. Ecol. Lett. 14, 476–483. doi: 10.1111/j.1461-0248.2011.01609.x
Richards, L. A., Dyer, L. A., Forister, M. L., Smilanich, A. M., Dodson, C. D., Leonard, M. D., et al. (2015). Phytochemical diversity drives plant-insect community diversity. Proc. Natl. Acad. Sci. USA. 112, 10973–10978. doi: 10.1073/pnas.1504977112
Richards, L. A., Glassmire, A. E., Ochsenrider, K. M., Smilanich, A. M., Dodson, C. D., Jeffrey, C. S., et al. (2016). Phytochemical diversity and synergistic effects on herbivores. Phytochem. Rev. 15, 1153–1166. doi: 10.1007/s11101-016-9479-8
Roy, M. C., Chang, F. R., Huang, H. C., Chiang, M. Y. N., and Wu, Y. C. (2005). Cytotoxic principles from the Formosan milkweed, Asclepias curassavica. J. Nat. Prod. 68, 1494–1499. doi: 10.1021/np0501740
Rubiano-Buitrago, P., Pradhan, S., Paetz, C., and Rowland, H. M. (2023). New Structures, spectrometric quantification, and inhibitory properties of cardenolides from Asclepias curassavica seeds. Molecules 28:105. doi: 10.3390/molecules28010105
Scudder, G. G. E., Moore, L. V., and Isman, M. B. (1986). Sequestration of cardenolides in Oncopeltus fasciatus: morphological and physiological adaptations. J. Chem. Ecol. 12, 1171–1187. doi: 10.1007/BF01639003
Scudder, G. G. E. E., and Meredith, J. (1982). Morphological basis of cardiac glycoside sequestration by Oncopeltus fasciatus (Dallas) (Hemiptera: Lygaeidae). Zoomorphology 99, 87–101. doi: 10.1007/BF00310302
Sønderby, I. E., Geu-Flores, F., and Halkier, B. A. (2010). Biosynthesis of glucosinolates - gene discovery and beyond. Trends Plant Sci. 15, 283–290. doi: 10.1016/j.tplants.2010.02.005
Taussky, H. H., and Shorr, E. (1953). A microcolorimetric method for the determination of inorganic phosphorus. J. boil. Chem. 202, 675–685.
Taverner, A. M., Yang, L., Barile, Z. J., Lin, B., Peng, J., Pinharanda, A. P., et al. (2019). Adaptive substitutions underlying cardiac glycoside insensitivity in insects exhibit epistasis in vivo. Elife 8, e48224. doi: 10.7554/eLife.48224
United States Department of Agriculture (2020). United States Department of Agriculture PLANTS Database. USDA PLANTS. Available online at: https://plants.sc.egov.usda.gov/java/ (accessed April 6, 2020).
Warashina, T., and Noro, T. (2008). Steroidal glycosides from the roots of Asclepias curassavica. Chem. Pharm. Bull. 56, 315–322. doi: 10.1248/cpb.56.315
Warashina, T., Shikata, K., Miyase, T., Fujii, S., and Noro, T. (2008). New cardenolide and acylated lignan glycosides from the aerial parts of Asclepias curassavica. Chem. Pharm. Bull 1, 1159–1163. doi: 10.1248/cpb.56.1159
Wheat, C. W., Vogel, H., Wittstock, U., Braby, M. F., Underwood, D., and Mitchell-Olds, T. (2007). The genetic basis of a plant-insect coevolutionary key innovation. Proc. Natl. Acad. Sci. USA. 104, 20427–20431. doi: 10.1073/pnas.0706229104
Whitehead, S. R., Bass, E., Corrigan, A., Kessler, A. E., and Poveda, K. (2021). Interaction diversity explains the maintenance of phytochemical diversity. Ecol. Lett. 24, 1205–1214. doi: 10.1111/ele.13736
Whitehead, S. R., Schneider, G. F., Dybzinski, R., Nelson, A. S., Gelambi, M., Jos, E., et al. (2022). Fruits, frugivores, and the evolution of phytochemical diversity. Oikos. 2022. doi: 10.1111/oik.08332
Zangerl, A. R., and Berenbaum, M. R. (2003). Phenotype matching in wild parsnip and parsnip webworms: causes and consequences. Evolution. 57, 806–815. doi: 10.1111/j.0014-3820.2003.tb00292.x
Zhang, R. R., Tian, H. Y., Tan, Y. F., Chung, T. Y., Sun, X. H., Xia, X., et al. (2014). Structures, chemotaxonomic significance, cytotoxic and Na+/K+-ATPase inhibitory activities of new cardenolides from Asclepias curassavica. Org. Biomol. Chem. 12, 8919–8929. doi: 10.1039/C4OB01545B
Keywords: Oncopeltus fasciatus, cardiac glycoside, phytochemical diversity, structure–activity relationship, toxin–receptor interactions, resistance
Citation: Rubiano-Buitrago P, Pradhan S, Grabe V, Aceves-Aparicio A, Paetz C and Rowland HM (2023) Differential accumulation of cardenolides from Asclepias curassavica by large milkweed bugs does not correspond to availability in seeds or biological activity on the bug Na+/K+-ATPase. Front. Ecol. Evol. 11:1175205. doi: 10.3389/fevo.2023.1175205
Received: 27 February 2023; Accepted: 15 May 2023;
Published: 08 June 2023.
Edited by:
Michael Wink, Heidelberg University, GermanyReviewed by:
Ute Wittstock, Technical University of Braunschweig, GermanyCopyright © 2023 Rubiano-Buitrago, Pradhan, Grabe, Aceves-Aparicio, Paetz and Rowland. This is an open-access article distributed under the terms of the Creative Commons Attribution License (CC BY). The use, distribution or reproduction in other forums is permitted, provided the original author(s) and the copyright owner(s) are credited and that the original publication in this journal is cited, in accordance with accepted academic practice. No use, distribution or reproduction is permitted which does not comply with these terms.
*Correspondence: Paola Rubiano-Buitrago, cGJ1aXRyYWdvQGljZS5tcGcuZGU=; Hannah M. Rowland, aHJvd2xhbmRAaWNlLm1wZy5kZQ==
†ORCID: Shrikant Pradhan orcid.org/0000-0002-5074-3633
Alfonso Aceves-Aparicio orcid.org/0000-0003-3043-7628
Disclaimer: All claims expressed in this article are solely those of the authors and do not necessarily represent those of their affiliated organizations, or those of the publisher, the editors and the reviewers. Any product that may be evaluated in this article or claim that may be made by its manufacturer is not guaranteed or endorsed by the publisher.
Research integrity at Frontiers
Learn more about the work of our research integrity team to safeguard the quality of each article we publish.