- 1College of Science, Shihezi University, Shihezi, Xinjiang, China
- 2College of Life Science, China West Normal University, Nanchong, Sichuan, China
Introduction: In the Qinghai–Tibet Plateau (QTP), alpine meadows are among the most noticeable reflection of global climate change. However, effects of global warming on soils hosting alpine meadows in the QTP, such as reduced moisture because of low precipitation, remain unclear.
Methods: Here, the soil moisture content (SMC), pH, dissolved organic carbon (DOC), ammonium nitrogen (NH4+–N), nitrate nitrogen (NO3−–N) and available phosphorus (AP) contents in the QTP were analyzed. The changes in and stoichiometries of total carbon, nitrogen, and phosphorus (TC, TN, and TP), microbial biomass carbon, nitrogen, and phosphorus (MBC, MBN, and MBP), β-1,4-glucosidase (BG), β-1,4-N-acetylglucoaminosidase (NAG), leucine aminopeptidase (LAP), and acid phosphatase (ACP) in the 0–30 cm layer of soils associated with warming in a greenhouse in the QTP from 2015 to 2020 were characterized.
Results: We found that warming in the greenhouse significantly decreased the SMC, NO3−–N, MBC, MBN, MBP, BG, LAP, ACP, and enzymatic C:N ratio. The warming increased the DOC, NH4+–N, AP, MBC:MBN, and enzymatic N:P ratios noticeably. The pH, TC, TN, TP, C:N, C:P, N:P, MBC:MBP, MBN:MBP, and enzymatic C:P ratios were minimally affected.
Conclusion: The results showed that warming and isolation from precipitation promoted mineralization of N and P in the soil but did not significantly alter the cycling of elements in soils in an alpine meadow.
1. Introduction
Worldwide, warming and precipitation variability is the most important manifestation of global climate change (Zhang W. X. et al., 2021), and these events will become more frequent in the future (IPCC, 2021). According to climate models, the global average temperature of the atmosphere will increase by 2–5°C relative to the pre-industrial revolution level before the end of the 21st century (Wieder et al., 2013; Meng et al., 2020). Temperature is a major driver of biogeochemical processes and thus warming alters the cycling of carbon (C), nitrogen (N), and phosphorus (P) in terrestrial ecosystems (Zhou et al., 2013a,b). Global warming increases the release of C from plants (leaves and roots) during photosynthesis (Zhao et al., 2016; Wang et al., 2021), the decomposition of soil organic C, and the flux of C from soils because of the enhanced activation of these processes (Fang et al., 2015). To improve the prediction of consequences linked to global warming, numerous simulation studies have been conducted (Fu et al., 2012; Wang et al., 2014; Meng et al., 2020). However, these studies have produced conflicting result. For example, the responses of microbial communities in soils to global warming differ according to the duration of experiments. Short-term (<3 years) warming studies show no significant impact on the microbial biomass in soils, whereas, warming that exceeds 3 years either increased or decreased the microbial biomass (Fu et al., 2012). Moreover, numerous studies have demonstrated that global warming is worsening phenomena such as droughts (Hu et al., 2020) because high temperatures stimulate evaporation and plant transpiration and increase soil water loss (Harte et al., 1995; Wan et al., 2002). Additionally, these studies commonly focused on one factor (Hu et al., 2020), such as warming (Fu et al., 2012; Wang et al., 2014; Meng et al., 2020), precipitation variability (Hu et al., 2020), and N deposition (Yang et al., 2021). Therefore, studies to evaluate the response of terrestrial ecosystems (grassland, forest, cropland) to climate change based on multiple components (Zhou et al., 2013a), such as vegetation and soil, as well as their interrelationships, are needed.
Soil is a crucial component of terrestrial ecosystems. It is the primary source of nutrients and water for plant growth, and thus, regulates the proliferation of plants (Yang et al., 2018; Tan et al., 2021). In soils, C, N, and P affect the metabolism and growth of plants, and therefore, a better understanding of relationships and the dynamics between elements can improve the management of terrestrial ecosystems (Tan et al., 2021). In addition to its utility for predicting the retention of nutrients and biomass production at varying scales (subcellular to ecosystem scales), the ecological stoichiometric approach enables the exploration of material circulation, energy balance, and the relationship between vegetation and the environment in terrestrial ecosystems (Sinsabaugh et al., 2009; Li et al., 2013; Yang et al., 2018). Thus, the ecological stoichiometric was used to reveal climate change (e.g., warming and precipitation variability) in the turnover of soil elements is very credible.
In soils, microorganisms cause decay of organic matter (Zhou et al., 2013a), maintain the balance between C, N, and P in terrestrial ecosystems (Gong et al., 2019), and ensure the availability of nutrients to plant through the provision of extracellular enzymes (Zhou et al., 2013a; Zhu et al., 2020). Therefore, the microbial biomass and elemental composition of secreted enzymes can be severely affected by the C:N:P ratio of the soil (Zhou et al., 2013a; Gong et al., 2019; Zhu et al., 2020). For, example, strong correlations were reported between the soil C:N and C:P ratios in high mountains, low mountains, and lowlands (Li et al., 2012; Gong et al., 2019). However, according to other studies, the stoichiometry of elements in soils and microbial biomass exhibit a weak or no correlation (Ehlers et al., 2010). In these studies, the ratios of elements in the microbial biomass were considered homeostatic and independent of the soil environment (Li et al., 2012). The relationships between elements in biomass and soils remain unclear. Therefore, to improve adaptation to climate change, further studies elucidating relationships between the stoichiometry of elements in soils and microbial biomass are needed.
The Qinghai–Tibet Plateau (QTP) is an area extremely sensitive to global climate change, and its ecosystems are quite fragile (Wang et al., 2014; Yang et al., 2018, 2021; Zhu et al., 2020). In particular, the average temperature rise in the region is ~40% higher than the global average (Luo et al., 2009; Zi et al., 2018). Therefore, it represents an ideal region for characterization of the response of terrestrial ecosystems to global climate change, such as warming (Luo et al., 2009; Fu et al., 2012; Zhao et al., 2016; Zi et al., 2018), warming and N fertilization (Zhao et al., 2014), increasing precipitation and warming (Niu et al., 2010). In addition, annual precipitation in the central part of the QTP exhibits an increasing trend, whereas a decreasing trend is displayed outside the region (Yang et al., 2011). Considering that vegetation covers more than 40% of the QTP, many studies on effects of global warming and other factor on plants and soils have been conducted in the region (Luo et al., 2009; Niu et al., 2010; Fu et al., 2012; Zhao et al., 2014, 2016; Zi et al., 2018). For example, warming can alter species of plant communities because of the enhanced availability of nutrients to competitive species, thereby inhibiting the growth of less competitive species (Zhao et al., 2016). Therefore, the growth and production of plants can be changed because of variations in the growing season and phenology of plants (Zi et al., 2018). Nevertheless, few studies on interactions between climatic factors and soils in alpine steppe ecosystems are available. Altitude, soil temperature and C/N ratio have the greatest impact on the diversity and composition of soil microbial communities in alpine ecosystems. Altitude is the most important environmental factor. It can not only adjust the availability of microclimate and nutrients, but also affect microbial communities by adjusting geographical distance (Cui et al., 2019). Due to the steep environmental gradient, different climatic zones may occur over a very short geographical distance. Therefore, we also need to carry out long-term multi-gradient warming research to explore the adaptation of the QTP to climate change.
Thus, here, a greenhouse experiment was conducted in an alpine meadow in the northeastern margin of the QTP, China. The hypothesis was that isolation from precipitation and warming can significantly alter the cycling of elements in soils in an alpine meadow. This study had three objectives: (1) determine if ecological stoichiometric characteristics, such as extracellular enzyme activities, were significantly altered, (2) determine changes in the cycling of elements in the soils, and (3) highlight mechanisms of the alterations caused by warming and decreased precipitation. We studied the relationship between soil physical and chemical properties and soil EEAs, which may provide insights on the mechanism of the interaction between climate factors and soil in alpine grassland ecosystem.
2. Materials and methods
2.1. Site description
The alpine meadow that was studied is in Hongyuan County, Sichuan Province, in the northeastern margin of the QTP, China (32°58′N, 102°37′E, 3475 m a.s.l.). The area is characterized by a cold plateau climate, with a mean annual temperature of 1.57°C and mean annual precipitation (1961–2015) of 752.21 mm (data from Chinese Meteorological Data Service Centre, http://data.cma.cn//). The coldest and hottest months are January and July, with mean temperatures of −9.66 and 11.12°C (Yang et al., 2021).
Soil in the study area is typical of an alpine meadow according to the soil classification standard of China. The meadow that was studied has served as a winter pasture (November–May) for yaks since 1996, and thus, it was fenced in July 2013. Vegetation in the region is dominated by the following species: Elymus nutans Griseb., Deschampisa caespitosa (L.) Beauv., Koeleria litwinowii Dom., Kobresia setchwanensis Hand.-Mazz., Festaca ovina L., Saussurea nigrescens Maxim., Trollius farreri stapf, Anemone rivularis Buch.-Ham. ex DC. var. flore-minore Maxim., and Astragalus polycladus Bur. et Franch (Yang et al., 2021).
2.2. Experimental design and sampling
One greenhouse (27 × 7.2 × 3.5 m) that was constructed in September 2015 was unperturbed prior to sampling. Temperature data loggers (WatchDog B101) were installed inside and outside the greenhouse on April 19, 2020, to collect data that were utilized for analyzing effects of warming. A data logger (A1) was installed at a height of 1.5 m in the center of the greenhouse to measure the air temperature, while another (A2) was buried at a depth of 10 cm at the same location as A1 to collect temperature data in the soil. Two data loggers (A3 and A4) were installed similar to A1 and A2, ~10 m from the edge of the greenhouse, to measure the air and soil temperature outside. The data loggers were retrieved and taken to the laboratory on May 11, 2021, and the collected data were downloaded.
In July 2020 (~5 years following the commencement of the greenhouse experiment), five 1 × 1 m quadrats (W, warming) along the principal axis of the greenhouse that were ~3 m apart were selected for sampling. In addition, five 1 m × 1 m quadrats (CK) ~10 m from the edges of the greenhouse were sampled. In each quadrant, the soil surface was cleaned and five cores (evenly distributed along a diagonal) were collected from the 0–30 cm (0–10, 10–20, and 20–30 cm intervals) layer using an auger (4 cm in diameter), and these were used for determination of properties of the soils.
2.3. Laboratory analysis
2.3.1. Sample preparation
Soil samples from each quadrant were mixed to produce a composite, which was sieved through a 2-mm mesh. Part of the sieved sample was used subsequently to determine the soil moisture content (SMC), pH, dissolved organic carbon (DOC), ammonium nitrogen (NH4+–N), nitrate nitrogen (NO3−–N), microbial biomass carbon (MBC), microbial biomass nitrogen (MBN), microbial biomass phosphorus (MBP), β-1,4-glucosidase (BG), β-1,4-N-acetylglucoaminosidase (NAG), leucine aminopeptidase (LAP), and acid phosphatase (ACP) contents. Another portion was air-dried, and a split was ground to <0.25 mm in diameter and used to determine the concentration of available phosphorus (AP), whereas another split was ground to <0.15 mm and utilized to measure the concentrations of TC, TN, and TP.
2.3.2. Determination of soil properties
Approximately 5 g of fresh soil was used to determine the pH of each sample using an acidimeter (PHS-3C, Shengke, China) following extraction for 30 min (200 r min−1) with distilled water (m:v = 1:5) at the ambient temperature. Relatedly, ~10 g of fresh soil was used to measure the SMC of each sample by drying to a constant weight at 105°C (Jiao et al., 2020). Further, another 5 g of fresh soil was extracted with 2 M KCl (m:v = 1:10) and filtered through a 0.45 μm filter membrane, and the DOC was determined from this sample using an automated total organic C analyzer (Shimadzu, TOC-Vwp, Japan; Zhang et al., 2018). A continuous flow analytical system (Auto Analyzer Bran + Luebbe, Germany) was used to determine the concentrations of NH4+–N and NO3−–N in the samples following the extraction of ~5 g of fresh soil with 2 M KCL (m:v = 1:10) on a shaker (200 r min−1) at the ambient temperature for l h. The concentrations of AP in the soil samples were determined using a spectrophotometer (Shimadzu, UV2450, Japan) at 880 nm after extraction with 0.2 M NaHCO3 (m:v = 1:10) on a shaker (200 r min−1) at the ambient temperature for l h, whereas those of TC and TN were measured using an elemental analyzer (Elementar Vario MACRO, Elementar, Germany). The concentration of TP was determined colorimetrically using a spectrophotometer (Shimadzu, UV2450, Japan) at 880 nm after the digestion of each sample with H2SO4 and H2O2 followed by H2SO4 and HClO4 (Yang et al., 2018). The stoichiometric ratios of C, N, and P are based on the mass of each element.
2.3.3. Measurement of the soil microbial biomass
The concentrations of MBC, MBN, and MBP were determined based on the chloroform-fumigation extraction method. Approximately 10 g of fresh soil was fumigated with chloroform in the dark for 24 h (fumigated soil), while another 10 g was cultivated without chloroform in the dark for 24 h (nonfumigated soil). Subsequently, ~5 g each of fumigated and nonfumigated soil samples were extracted using 0.5 M K2SO4 (m:v = 1:5) on a shaker at the ambient temperature for l h (200 r min−1). The concentrations of MBC and MBN were then determined correspondingly using an automated total organic C analyzer (Shimadzu, TOC-Vwp, Japan) and an auto analyzer (Bran + Luebbe, Germany). The other 5 g each of fumigated and nonfumigated soil samples were extracted using 0.5 M NaHCO3 (m:v = 1:10, pH = 8.5) on a shaker at the ambient temperature for 30 min (200 r min−1). The concentration of MBP in each soil sample was then determined using a spectrophotometer (Shimadzu, UV2450, Japan) at 880 nm. The soil microbial biomass element (MBE) was determined using the following expression:
where CF is the concentration of an element in the fumigated soil, CUF is the concentration of an element in the nonfumigated soil, and K is the correction factor. In the present study, the K values that were used for calculation of the MBC, MBN, and MBP were 0.45, 0.54, and 0.4, respectively (Zederer et al., 2017). The stoichiometric ratios of the MBC, MBN, and MBP are expressed based on the mass of each element.
2.3.4. Measurement of extracellular enzyme activities
A slightly modified 4-methyl-umbelliferyl substrate conjugate was used to determine extracellular enzyme activities (EEAs) (Saiya-Corka et al., 2002). Approximately 1 g of fresh soil (diameter < 0.15 mm) was mixed with 125 ml of an acetate buffer (50 mM, pH = 5.0) on a shaker (200 r min−1) at room temperature for 20 min to produce soil suspensions. Black 96-well microplates were then divided into six parts and eight replicate wells for each part. These included the following: sample assay (SA), sample control (SC), quench control (QC), reference standard (RS), negative control (NC), and blank (B) wells. First, 200 μL of the buffer was added to each of the B, RS, and NC wells. Second, 50 μL of the buffer was added into each of the B and SC wells to eliminate the natural fluorescence interference of the buffer and soil in the well plate. Third, 200 μL of the soil suspension was added to each of the SC, SA, and QC wells, and then 50 μL of a 10-μM MUB solution was poured into each of the RS and QC wells. Finally, 50 μL of a 200-μM MUB-linked substrate was added to each of the NC and SA wells, and the time was noted. The microplates were covered and incubated in the dark at 25°C for 4 h, and 10 μL of 1.0 M NaOH was added to each well to stop the reaction. The fluorescence intensities of samples were then measured at excitation wavelength of 365 nm and 450 nm emission filters. The substrates for the BG, NAG, LAP, and ACP were 4-methylumbelliferyl-β-D-glucopyranoside, 4-Methylumbelliferyl, N-acetyl-β-D-glucosaminide, L-Leucine-7-amino-4-methylcoumarin hydrochloride, and 4-Methylumbelliferyl phosphate, respectively. The EEAs were calculated according to the method described in a previous study (DeForest, 2009) and expressed in mmol h−1 g−1. The C:N, C:P, and N:P ratios of extracellular enzymes were correspondingly expressed as Ln(BG):Ln(LAP + NAG), Ln(BG):LN(ACP), and Ln(LAP + NAG):Ln(ACP) (Zhu et al., 2020).
2.4. Data analysis
The Statgraphics3.0 software (STN, St Louis, MO, United States) was used to perform all statistical analyses. The two-way ANOVA was used for data analysis following normality testing. The treatment (CK and W) and soil depth (0–10, 10–20, and 20–30 cm) served as independent variables. The Tukey-HSD was used to correct p-values associated with the multi-comparisons, and the difference between means was considered significant if the p-value of the ANOVA F-test was ≤0.05. All data are expressed as the mean ± the standard error (SE).
3. Results
3.1. Microclimate analysis
The results show that compared with the control, the greenhouse increased the air and soil temperatures by average values of 8.32 and 7.52°C, respectively (Figure 1; Supplementary Table S1, p < 0.001) between April 20, 2020 and October 10, 2021.
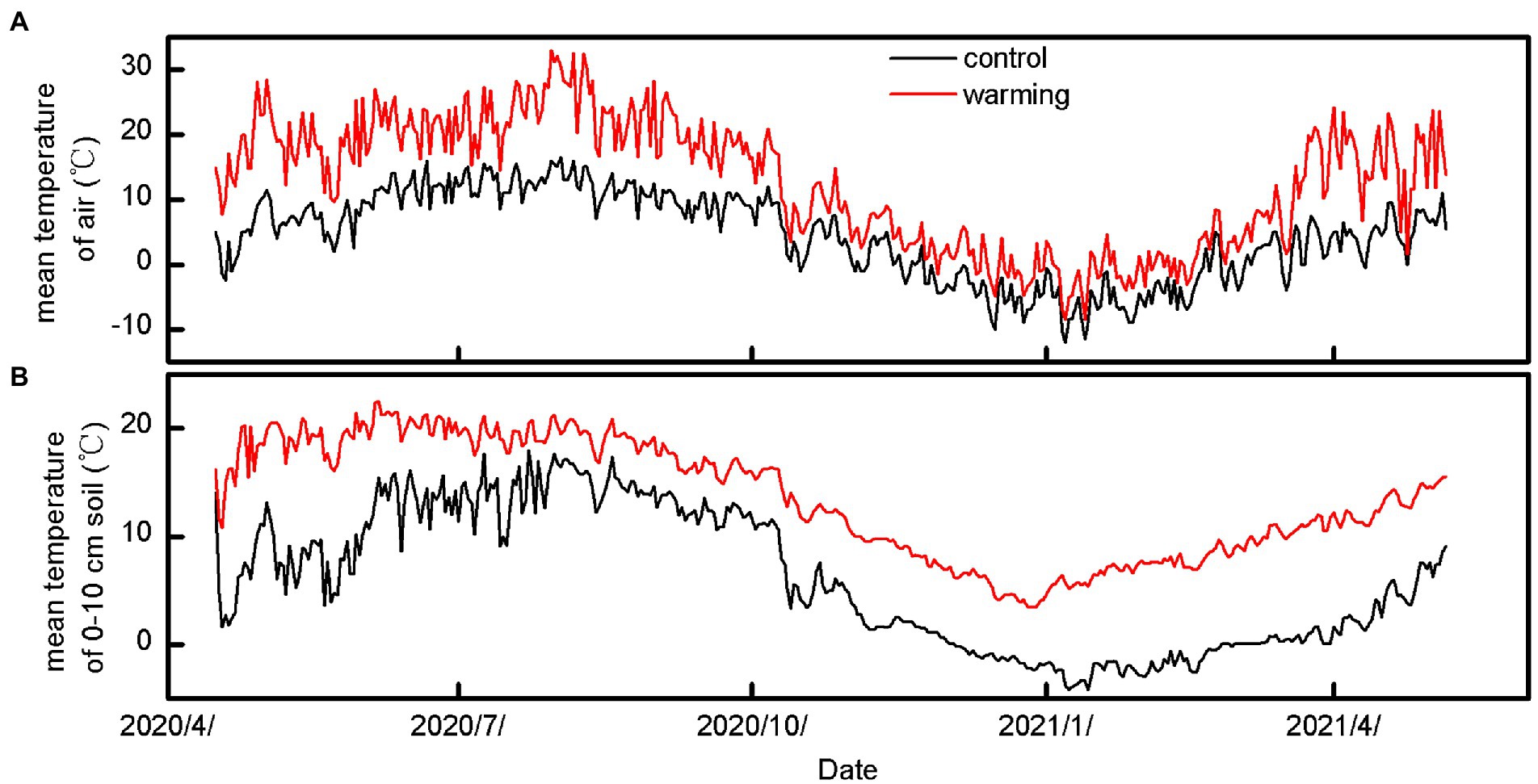
Figure 1. The mean temperature of air (A) and 10 cm soil (B) under control and warming during 20/4/2020–10/5/2021.
3.2. Variations in soil properties and the concentrations of carbon, nitrogen, and phosphorus
Compared with the control, the greenhouse significantly decreased the SMC (Figure 2A, p < 0.001) and concentration of NO3−–N (Figure 2E, p < 0.001) in the soil. In contrast, the greenhouse increased the concentrations of DOC (Figure 2C, p < 0.001), NH4+–N (Figure 2D, p < 0.001), and AP (Figure 2D, p < 0.001) in the soil. Interestingly, the greenhouse minimally affected the pH (Figure 2B, p = 0.410), and concentrations of TC (Figure 3A, p = 0.827), TN (Figure 3B, p = 0.713), and TP (Figure 3C, p = 0.860) in the soil. Moreover, the C:N (Figure 3D, p = 0.615), C:P (Figure 3E, p = 0.781), and N:P (Figure 3F, p = 0.605) ratios for samples from inside and outside the greenhouse were comparable.
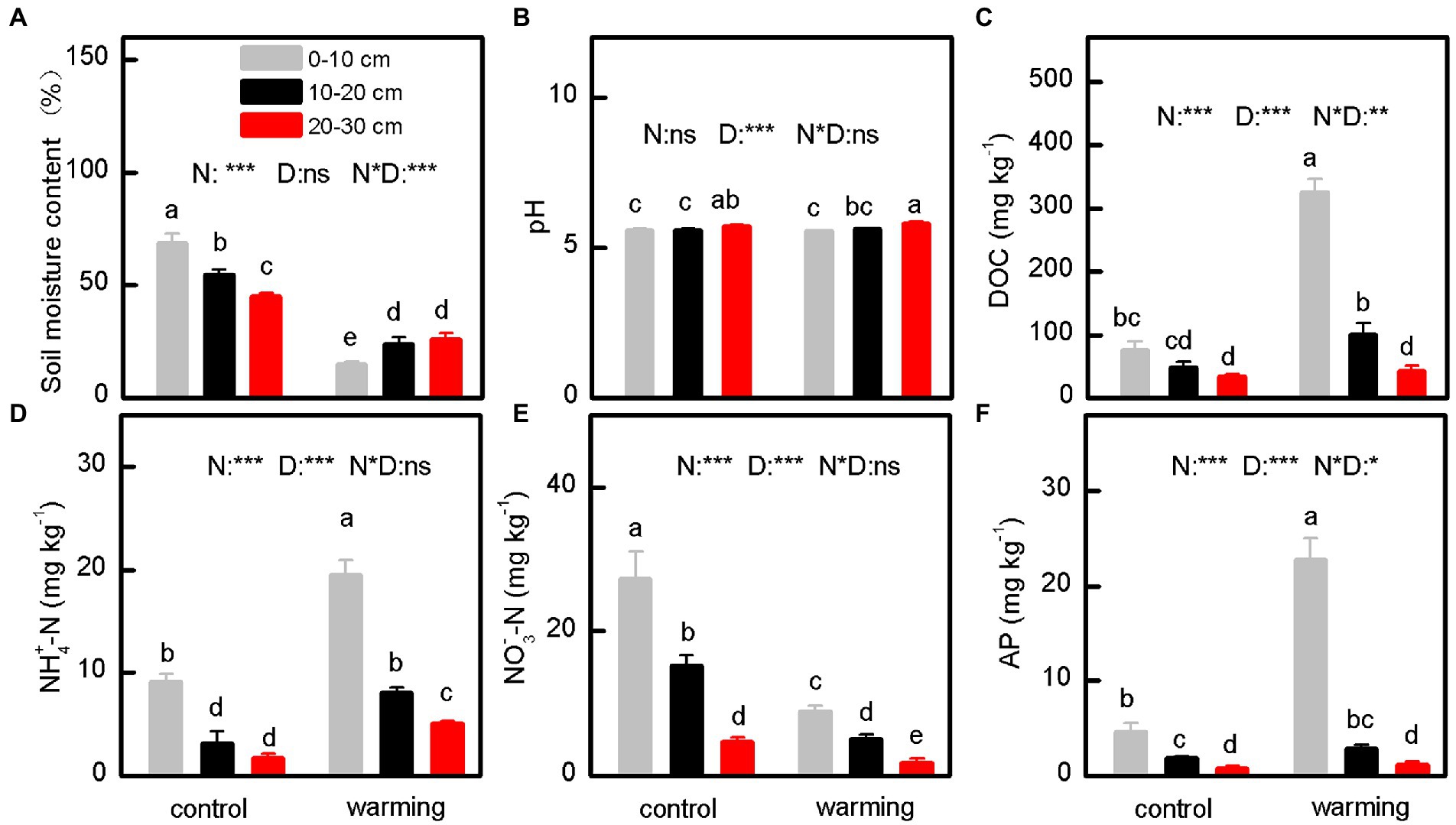
Figure 2. The SMC (soil moisture content) (A), pH (B), DOC (dissolved organic carbon) (C), NH4+-N (ammonium nitrogen) (D), NO3−-N (nitrate nitrogen) (E) and AP (available phosphorus) (F) of soil under control and warming. p-value of the ANOVA of treatments (control and warming, N), soil depths (0–10, 10–20 and 20–30 cm, D) and interactive of treatments and soil depths (N*D) is indicated: *p ≤ 0.05; **p ≤ 0.01; ***p ≤ 0.001; ns, not significant.
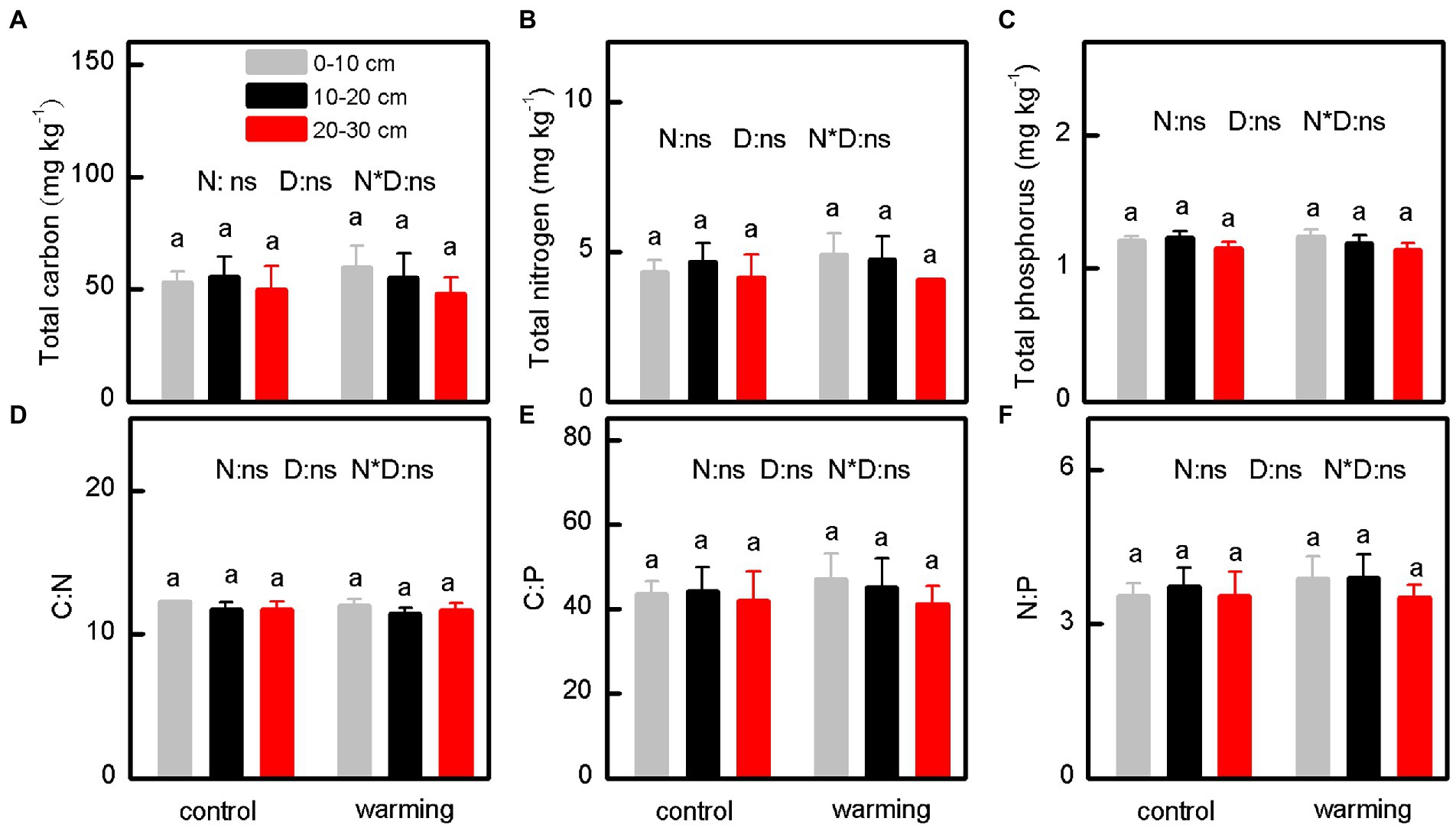
Figure 3. The concentration of total carbon (A), total nitrogen (B), total phosphorus (C), and the ratio of C:N (D), C:P (E) and N:P (F) of soil under control and warming. p-value of the ANOVA of treatments (control and warming, N), soil depths (0–10, 10–20 and 20–30 cm, D) and interactive of treatments and soil depths (N*D) is indicated: *p ≤ 0.05; **p ≤ 0.01; ***p ≤ 0.001; ns, not significant.
3.3. Variations in soil microbial biomass and enzymes
The concentrations of MBC (Figure 4A, p < 0.001), MBN (Figure 4B, p < 0.001), and MBP (Figure 4C, p < 0.001), and activities of BG (Figure 5C, p < 0.001), LAP (Figure 5C, p < 0.001), NAG (Figure 5C, p = 0.001), and ACP (Figure 5D, p < 0.001), as well as the enzymatic C:N ratio (Figure 5E, p < 0.001) for samples from the greenhouse were considerably lower than those for samples from outside. In contrast, compared with control samples, the greenhouse increased the MBC: MBN (Figure 4D, p < 0.001) and enzymatic N:P (Figure 5G, p < 0.001) ratios. Notably, the MBC:MBP (Figure 4E, p = 0.267), MBN:MBP (Figure 4F, p = 0.159), and enzymatic C:P (Figure 5F, p = 0.064) ratios for soils inside and outside the greenhouse were comparable.
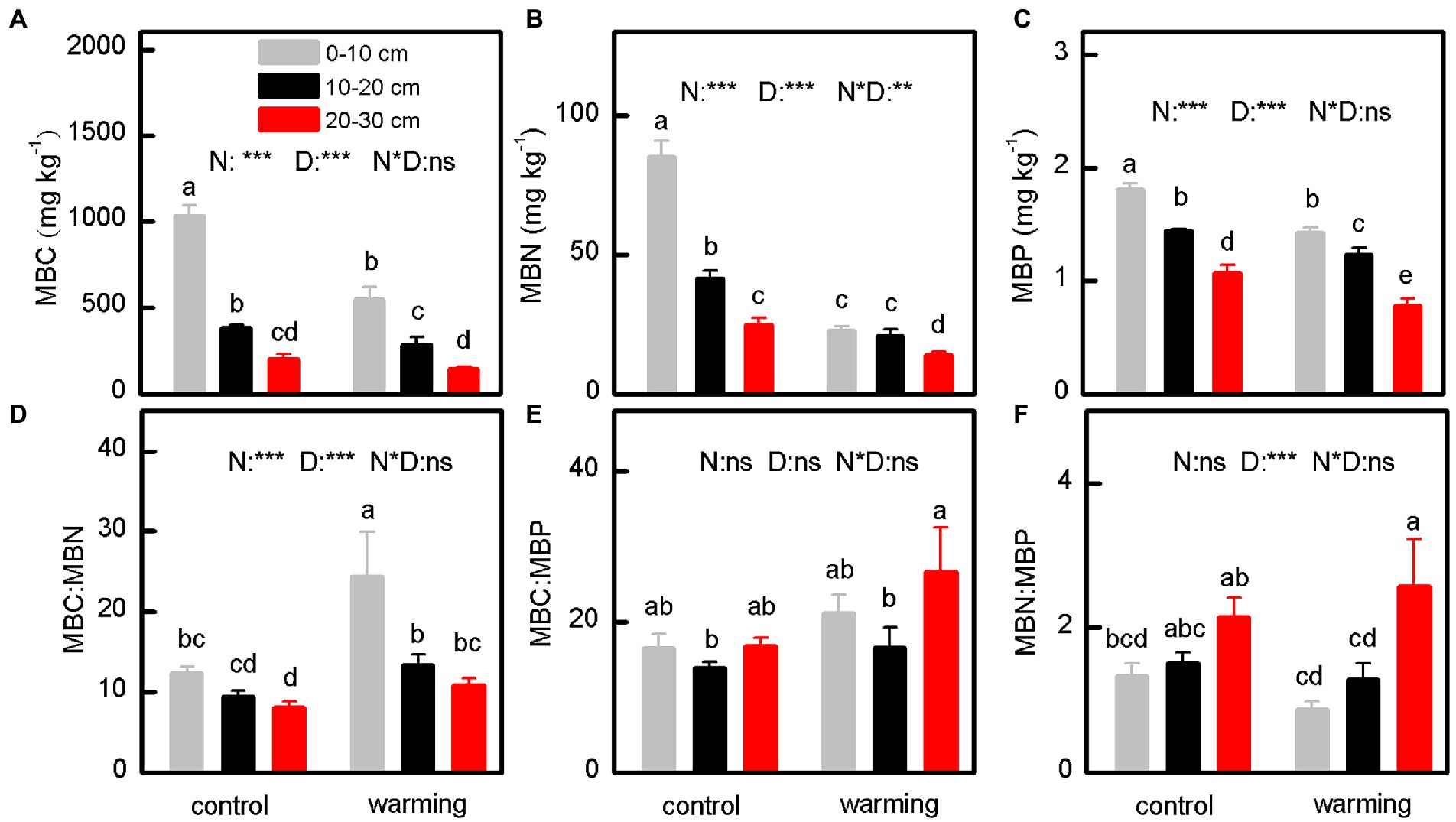
Figure 4. The microbial biomass carbon (A), microbial biomass nitrogen (B), microbial biomass phosphorus (C), and their stoichiometric ratios (D–F) of soil under control and warming. p-value of the ANOVA of treatments (control and warming, N), soil depths (0–10, 10–20 and 20–30 cm, D) and interactive of treatments and soil depths (N*D) is indicated: *p ≤ 0.05; **p ≤ 0.01; ***p ≤ 0.001; ns, not significant.
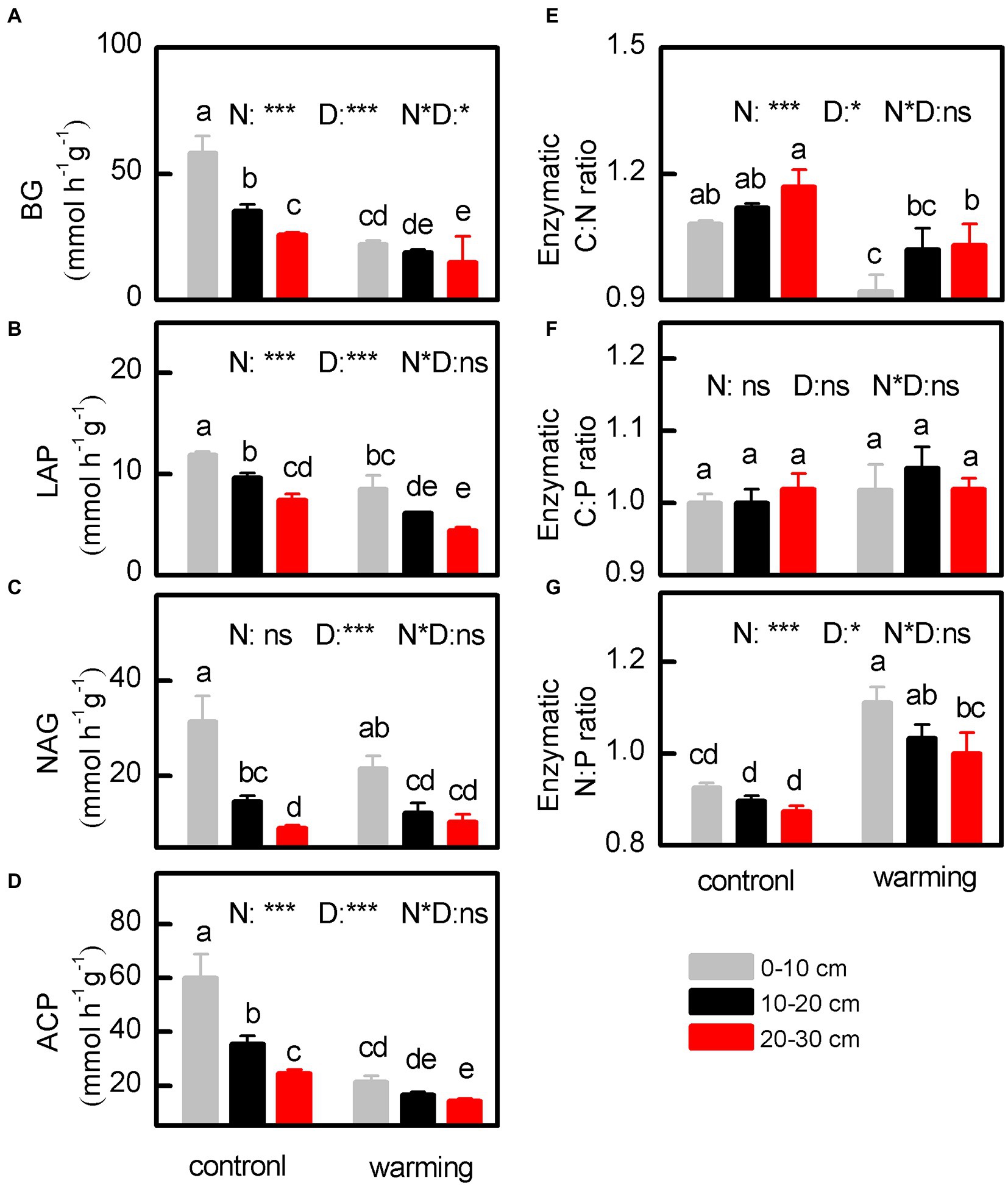
Figure 5. The activity of BG (β-1,4-glucosidase) (A), LAP (leucine aminopeptidase) (B), NAG (β-1,4-N-acetylglucoaminosidase) (C) and ACP (acid phosphatase) (D) and the stoichiometric ratios of C-, N- and P-degrading enzymes (E–G) of soil under control and warming. p-value of the ANOVA of treatments (control and warming, N), soil depths (0–10, 10–20 and 20–30 cm, D) and interactive of treatments and soil depths (N*D) is indicated: *p ≤ 0.05; **p ≤ 0.01; ***P ≤ 0.001; ns, not significant.
4. Discussion
4.1. Variations in soil properties
According to previous studies, warming can increase evaporation from soils and transpiration of plants, and these processes decrease the availability of water, and thus, the SMC (Zhou et al., 2013a,b). In the present study, the SMC was significantly decreased (Figure 2A) compared with the control. However, in addition to the warming, the greenhouse shielded the soil from precipitation during the period from September 2015 to July 2021 (prior to sampling). Therefore, the SMC was dependent on soil osmosis and capillary pore conditions in the greenhouse (Xiao et al., 2011), and the weakened soil capillary action caused by the increased evaporation exacerbated the decrease in the SMC (Wu et al., 2019).
In a previous study, warming was reported to exert no significant effect on the pH of soils in alpine meadows in the QTP (Zheng et al., 2012), and results in the present study are consistent with this observation (Figure 2B). However, the 5 years duration of warming was probably short to significantly influence the soil pH. According to another study on alpine meadows, warming starts to considerably impact the soil pH after at least 20 years (Zhang X. et al., 2021). Considering that fungi are tolerant to low pH conditions and high pH conditions are favorable for the growth of bacteria (Zhang et al., 2016). Therefore, the slight increase of the soil pH in the greenhouse (Figure 2B) probably increased the population of bacteria. However, a high MBC:MBN ratio (Figure 4D) is attributed to an increase in fungi (Yang et al., 2018), and thus, the impact of a high pH in the present study appears inconsistent with previous observations. Therefore, the effect of warming in alpine meadows on the soil pH requires further investigation.
The soil DOC exerts an important influence on the biochemical cycle. According to a previous study, warming significantly increased the DOC concentration in soil solutions (Luo et al., 2009), and this observation is consistent with results in the present study (Figure 2C). Warming enhances biological activity, including the decomposition of organic matter in soils (Luo et al., 2009). Moreover, warming and drying promote the production of dissolved organic matter (Luo et al., 2009). The decrease of soil moisture enhances the release of soil organic matter from roots (Kuzyakov and Domanski, 2000), and the amount of soil leached impacts the concentration of DOC (Zhang Q. Y. et al., 2019; Zhang S. S. et al., 2019). Owing to the lower leaching associated with drying, the released DOC was low, and thus, the soil DOC increased (Figure 2C).
The results in the present study reveal that warming and drying significantly increase the concentration of NH4+–N (Figure 2D) and decrease the concentration of NO3−–N (Figure 2E) in soils. These results show that even though the N mineralization rate increased, the nitrification rate in the soil decreased. In all ecosystems, a decrease in the availability of water limits the growth of plants (Hartmann et al., 2013; Schaeffer et al., 2017). This in turn reduces the production of roots and litter, which are the principal sources of N for plant growth (Hartmann et al., 2013; Schaeffer et al., 2017). According to previous studies, the accumulation of NH4+–N begins during a drought period, and the rate is higher than that of NO3−–N because of the lower nitrification in dry soils (Manzoni et al., 2014). Cells from dead microorganisms promote mineralization of N by drought-surviving microorganisms (Schaeffer et al., 2017), and this increased the concentration of NH4+–N in the soil (Figure 2D). In contrast, the concentration of NO3−–N in the soil decreased (Figure 2E). This is attributed to the low microbial activity in the soil that is associated with the loss of soil moisture (Figure 2A), which inhibited nitrification (Khan et al., 2016). Moreover, the N uptake preference of plants will also change at different growth stages (Cui et al., 2017). At the same time, a large amount of nitrate absorbed by plants may be assimilated into ammonium roots and amino acids in roots, resulting in the reduction of NO3−–N content (Liu et al., 2017). Alternatively, 16S rRNA gene abundance data suggest that the nitrifying activity of bacteria and archaea in the soil decreased under drought conditions, and the reduced nitrification rate accounts for the low NO3−–N concentration (Hartmann et al., 2013; Figure 2E). While, low MBN (Figure 4B), and high MBC/MBN ratio (Figure 4D) reflect a decrease in nitrifiers in the greenhouse soil (Khan et al., 2016). Therefore, as the SMC decreased (Figure 2A), the NO3−-N concentration also decreased (Figure 3E). Concurrently, the increasing temperature and decreasing water content promoted mineralization of organophosphorus in the soil (Zuccarini et al., 2020). The associated improved availability of P in the soil is manifested by the elevated concentration of AP (Figure 3F).
4.2. Variations in soil carbon, nitrogen, and phosphorus, and their stoichiometries
Warming alters the activities of enzymes in soils, and this subsequently affects the cycling of C and N (Gong et al., 2015). In the present study, the concentrations of TC (Figure 3A), TN (Figure 3B), and TP (Figure 3C) and the C:N (Figure 3D), C:P (Figure 3E), and N:P (Figure 3F) ratios of the soil were high. These results suggest that changes in the DOC, NH4+–N, NO3−–N, and AP were probably insufficient to alter the stability of their coupled relationships. This implies that the warming and isolation from precipitation in the present study minimally altered the cycling of C, N, and P in the alpine meadow.
In terrestrial ecosystems, the soil is an important pool for C and N, and their inputs (plant photosynthesis) and outputs (microbial decomposition, N absorption, and inter-cycling) control the balance of these elements (Tang et al., 2021). In the warming experiment, the net primary production and plant C absorption as total primary production increased (Deng et al., 2021; Tang et al., 2021). However, the increase was offset by the rise in C emissions to the atmosphere, and thus, no significant change occurred in the TC content of the soil (Figure 4A). Alternatively, the effect of warming on the soil respiration continued to decline, and thus, no significant change was observed as the duration of warming increased (Frey et al., 2008). This indirectly demonstrates that soil carbon minimally changed, and this was likely because the effect of decreased precipitation and warming in the meadow attained a stable state.
Relatedly, the net N mineralization rate increased because of the warming, whereas the soil TN was unaffected, and this is probably linked to the limited microbial carbon source (Tang et al., 2021). Even though the decomposition of organic matter can alter the soil TP, this change is limited, because it largely depends on the weathering of rocks (Yang et al., 2015; Jiao et al., 2016). Therefore, in the present study, the concentration of TP in the soil was unaffected by warming (Figure 4C). Moreover, the C:N:P stoichiometric ratio of soil directly influences the genetic resistance of the microbial population (Luo et al., 2020). In the present study, the C:N (Figure 4D), C:P (Figure 4E), and N:P (Figure 4F) ratios exhibited no significant change, and this is possibly because the microbial community can maintain a stoichiometric steady-state as an adaptation to changes in the status of nutrients.
4.3. Variation in soil microbial biomass
Microbes can decrease the production of enzymes, for example, by limiting substrates and other resources, including water (Nannipieri et al., 2012; Stark et al., 2014). This observation is consistent with results obtained in the present study (Figures 4A–C). The microbial biomass in soil is closely correlated to the type of vegetation (Baldrian et al., 2008). Deep-rooted species, such as grasses, can increase the storage of carbon via inputs of root material, microbial processing, and matrix stabilization, whereas forbs are associated with the opposite (Liu et al., 2018). In fact, if the proportion of grass decreases while that of forbs increases (unpublished data), soil root exudates and the MBC decrease (Figure 4A). In addition, bacteria need more N for assimilation than fungi (Högberg et al., 2007), and an increase in temperature is favorable for fungi in the soil microbial community (Zhao et al., 2014). This is consistent with the decrease in MBN that was obtained in the present study (Figure 4B). The increase in fungi explains the high MBC:MBN ratio (Figure 4D), considering that the C:N ratio of fungi (4.5–15) is significantly higher than that of bacteria (3–5) (Yang et al., 2018). The warming also increased the availability of N, which limited the phosphorus from microorganisms, which explains the decline in the concentration of MBP (Gong et al., 2019). In the present study, the MBC:MBN ratio (Figure 4D) in the soil increased, whereas the MBC:MBP (Figure 4E) and MBN:MBP (Figure 4F) ratios exhibited minor changes under the warming and decreased precipitation conditions. These changes mainly depended on the sampling period, and the level is the main factor that affects both the MBN and MBP concentrations (Li et al., 2016).
According to a previous simulation study, warming significantly increased the availability of nutrients, but the soil microbial biomass was essentially unchanged. These observations suggest that microorganisms in the soil did not directly benefit from higher availability of nutrients (Xu et al., 2010). While, in this study, warming and isolation from precipitation in the greenhouse significantly increased the concentrations of DOC (Figure 2C), NH4+–N (Figure 2D) and AP (Figure 2F), whereas the concentrations of MBC (Figure 4A), MBN (Figure 4B), and MBP (Figure 4C) decreased. These results also suggest that microorganisms in the soil failed to benefit directly from the higher availability of nutrients under the warming conditions.
4.4. Variations in activities of soil enzymes
The effects of warming on soil EEA that have been reported in previous studies vary (Zhou et al., 2013a; Wang et al., 2014). However, in this study, the BG (Figure 5A), LAP (Figure 5B), and ACP (Figure 5D) significantly decreased, whereas the NAG (Figure 5C) slightly decreased (mean = 15.32% for the 0–30 cm soil layer). In general, warming can enhance the efficiency of extracellular enzymes, thereby decreasing the production of such enzymes by microbes involved in the cycling of C and nutrients (Zhou et al., 2013a). This observation is supported by the strong positive correlations between the soil EEA and MBC (r = 0.693–0.809, p < 0.01), MBN (r = 0.717–0.907, p < 0.01), and MBP (r = 0.785–0.911, p < 0.01) (Supplementary Table S2). In addition, the strong positive correlation between the soil EEA and SWC (r = 0.439–0.869, p < 0.01; Supplementary Table S2) indicates that the soil EEA mainly depends on the soil moisture (Carlyle et al., 2011; Zhou et al., 2013a). Excluding the BG, the hydraulic isolation in soils caused by drought exerts direct physiological and indirect (reduction of substrate diffusion) pressures on the microbial community (Schaeffer et al., 2017). These pressures decrease microbial activities, and thus, accelerate the decomposition of organic carbon in soil, thereby decreasing the soil SOC (Schindlbacher et al., 2011; Schaeffer et al., 2017). The BG is closely related to the decomposition of cellulose, and its activity was also decreased by the warming (Zhou et al., 2013a; Figure 5A). The significant decrease in the N-acquisition enzyme LAP (Figure 5B) and slight decrease in the NAG (Figure 5C) under the warming conditions in this work may be linked to decreased oxidation of NH4+–N and an increase in the concentration of NH4+–N (Hartmann et al., 2013).
Therefore, the synthesis of N-acquisition enzymes (NAG and LAP) appears to be closely related to the fungal biomass, whereas some C-acquisition enzymes (e.g., BG) are more associated with bacteria (Fanin et al., 2016). Therefore, the decrease in the enzymatic C:N ratio (Figure 5E) was likely caused by an increase in fungi/bacteria in the microbial community owing to the warming. In the present study, the decrease in the enzymatic C:N ratio (Figure 5E) is primarily associated with the decrease in the BG (Figure 5A), which is more than those of the total LAP (Figure 5B) and NAG (Figure 5C). This is because the temperature sensitivities of N-acquisition enzymes are lower than those of C-acquisition enzymes (Steinweg et al., 2013). Therefore, the N-acquisition enzymes slightly decreased in response to the N limitation under warming conditions (Steinweg et al., 2013). However, considering that the activities of the BG and ACP decreased comparably, the enzymatic C:P ratio exhibits no significant change (Figure 5F). This is probably because the soil microbial community can attain a stoichiometric homeostasis in response to changes in the status of nutrients. To reach the elemental homeostasis, microorganisms can alternatingly adjust their production of extracellular enzymes by maximizing the mobilization of substrates that are rich in limiting elements (Mooshammer et al., 2014).
A field experiment in a temperate grassland region revealed significant differences in the response of soil EEA at different depths to climate change (Zhou et al., 2013a). In particular, changes in the 0–10 and 10–20 cm soil layers were mainly driven by the pH, NH4+–N, and NO3−–N, and NH4+–N, and the microbial biomass, respectively (Zhou et al., 2013a). However, results obtained in this study (Figure 5) differed from those of this previous study (Zhou et al., 2013a). The differences are mainly attributed to direct effects of warming on the soil temperature and SWC, instead of changes in the quantity of organic matter and the quality of nutrients in the soil (Sardans et al., 2008). Therefore, the SMC and activities of extracellular enzymes decrease as the depth of the soil layer increases (Figure 5A). Meanwhile, the strong positive correlations between the WSC and MBC (r = 0.537, p < 0.01), MBN (r = 0.801, p < 0.01), MBP (r = 0.681, p < 0.01), BG (r = 0.869, p < 0.01), LAP (r = 0.628, p < 0.01), NAG (r = 0.439, p < 0.01), and ACP (r = 0.845, p < 0.01; Supplementary Table S2) in this study are probably because of the isolation of soil in the greenhouse from precipitation for ~5 years, and thus, the soil in the greenhouse was severely short of water. Alternatively, the EEA data in the present study may be controlled primarily by the WSC in the 0–30 cm soil layer.
4.5. Effects of warming and isolation from precipitation on the availability of nitrogen
In alpine meadows, soil N is usually limited (Yang et al., 2021), and this can be accentuated as other components change because of warming and isolation from precipitation (Figure 6). First, the decrease in the concentration of NO3−–N surpassed the increase in the concentration of NH4+–N (Figures 2D,E). This was because the mineralization of N was promoted (Schaeffer et al., 2017), nitrification of N was inhibited (Hartmann et al., 2013; Khan et al., 2016), and a large amount of N absorbed by plants (Liu et al., 2017). Alternatively, the concentration of available N decreased (Supplementary Table S2). Second, the concentrations of MBC, MBN, and MBP significantly decreased because of the warming and drought, and the decrease in the concentration of MBN was higher than that of the MBC (Figures 3A–C), and thus, the MBC:MBN ratio increased (Figure 3E), which indicates a severe MBN deficiency. This may be because that soil microorganisms failed to benefit directly from the higher availability of nutrients under the warming conditions. Third, with the help of decreasing oxidation of NH4+–N and an increasing in the concentration of NH4+–N (Hartmann et al., 2013) under the warming conditions, the activity of the LAP (Figure 5B) and NAG (Figure 5C) were all decreased in response to the warming and isolation from precipitation. In conclusion, warming and isolation from precipitation enhance the limitation of N in alpine meadows.
4.6. Limitations
There were some limitations in this work. Firstly, the greenhouse increases the soil temperature, which is likely to be the realistic impact of future climate change (Carlyle et al., 2011). However, the atmospheric temperature was increased by 8.32°C in the greenhouse in this work (Figure 1A), which is higher than the predicted increase relative to the pre-industrial revolution level before the end of the 21st century (Wieder et al., 2013; Meng et al., 2020). Secondly, the high temperature can stimulate evaporation and plant transpiration and increase soil water loss (Harte et al., 1995; Wan et al., 2002) but the soil water loss caused by global warming is not as same as the isolation from precipitation studies in this work, and the SMC was dependent on soil osmosis and capillary pore conditions in the greenhouse (Xiao et al., 2011). Therefore, we are still far from explaining the impact of global climate change on the soil of the QTP. Additionally, warming and precipitation variability will become more frequent in the future (IPCC, 2021). Therefore, to consider the change of water grid under the influence of climate warming and drought in many aspects, it is necessary to carry out long-term continuous monitoring.
5. Conclusion
In the present study, a greenhouse experiment was conducted in an alpine meadow in the QTP to simulate the response of soils in the area to global warming. Data were generated for the SWC, pH, and changes in the DOC, NH4+–N, NO3−–N, and AP concentrations, as well as the stoichiometries of TC, TN, TP, MBC, MBN, MBP, BG, NAG, LAP, and ACP using samples that were collected from the 0–30 cm soil layer, over a duration of ~5 years (2015–2020). Evidently, the greenhouse warming significantly decreased the SMC, NO3−–N, MBC, MBN, MBP, BG, LAP, ACP, and enzymatic C:N ratio. Conversely, it considerably increased the DOC, NH4+–N, AP, MBC:MBN, and enzymatic N:P ratio. Interestingly, it minimally affected the soil pH, TC, TN, TP, C:N, C:P, N:P, MBC:MBP, MBN:MBP, and enzymatic C:P ratios. These results indicated that warming and isolation from precipitation promoted the mineralization of N and P in the soils. However, microorganisms in the soils minimally benefited directly from the higher availability of nutrients associated with the warming and isolation from precipitation. The warming and isolation from precipitation also significantly altered the microbial biomass, EEA, and their stoichiometric ratios, but the concentrations of C, N, and P in the soils exhibited limited changes. Therefore, to adequately understand the response of terrestrial ecosystems to the cycling of C and N under warming conditions, continuous monitoring over an extended duration is required.
Data availability statement
The datasets used and/or analyzed during the current study are available from the corresponding author upon reasonable request—ZY (eXphMjc2NUAxMjYuY29t).
Author contributions
YT: data curation and writing—original draft preparation. YL: methodology, investigation, and formal analysis. ZY: conceptualization, investigation, formal analysis, data curation, and writing—review and editing. All authors contributed to the article and approved the submitted version.
Acknowledgments
We would like to thank Editage (www.editage.cn) for English language editing.
Conflict of interest
The authors declare that the research was conducted in the absence of any commercial or financial relationships that could be construed as a potential conflict of interest.
Publisher’s note
All claims expressed in this article are solely those of the authors and do not necessarily represent those of their affiliated organizations, or those of the publisher, the editors and the reviewers. Any product that may be evaluated in this article, or claim that may be made by its manufacturer, is not guaranteed or endorsed by the publisher.
Supplementary material
The Supplementary material for this article can be found online at: https://www.frontiersin.org/articles/10.3389/fevo.2023.1149240/full#supplementary-material
References
Baldrian, P., Trögl, J., Frouz, J., Snajdr, J., Valàšková, V., Merhautvá, V., et al. (2008). Enzyme activities and microbial biomass in topsoil layer during spontaneous succesion in spoil heaps after brown coal mining. Soil Biol. Biochem. 40, 2107–2115. doi: 10.1016/j.soilbio.2008.02.019
Carlyle, C. N., Fraser, L. H., and Turkington, R. (2011). Tracking soil temperature and moisture in a multi-factor climate experiment in temperate grassland: do climate manipulation methods produce their intended effects? Ecosystems 14, 489–502. doi: 10.1007/s10021-011-9425-y
Cui, Y. X., Bing, H. J., Fang, L. C., Wu, Y. H., Yu, J. L., Shen, G. T., et al. (2019). Diversity patterns of the rhizosphere and bulk soil microbial communities along an altitudinal gradient in an alpine ecosystem of the eastern Tibetan plateau. Geoderma 338, 118–127. doi: 10.1016/j.geoderma.2018.11.047
Cui, J. H., Yu, C. Q., Qiao, N., Xu, X. L., Tian, Y. Q., and Ouyang, H. (2017). Plant preference for NH4+ versus NO3− at different growth stages in an alpine agroecosystem. Field Crop Res. 201, 192–199. doi: 10.1016/j.fcr.2016.11.009
DeForest, J. L. (2009). The influence of time, storage temperature, and substrate age on potential soil enzyme activity in acidic forest soils using MUB-linked substrates and L-DOPA. Soil Biol. Biochem. 41, 1180–1186. doi: 10.1016/j.soilbio.2009.02.029
Deng, L., Peng, C. H., Kim, D. G., Li, J. W., Liu, Y. L., Hai, X. Y., et al. (2021). Drought effects on soil carbon and nitrogen dynamics in global natural ecosystems. Earth-Sci. Rev. 214:103501. doi: 10.1016/j.earscirev.2020.103501
Ehlers, K., Bakken, L. R., Frostegård, Å., Frossard, E., and Bünemann, E. K. (2010). Phosphorus limitation in a Ferralsol: impact on microbial activity and cell internal P pools. Soil Biol. Biochem. 42, 558–566. doi: 10.1016/j.soilbio.2009.11.025
Fang, X., Zhou, G., Li, Y., Liu, S., Chu, G., Xu, Z., et al. (2015). Warming effects on biomass and composition of microbial communities and enzyme activities within soil aggregates in subtropical forest. Biol. Fert. Soils 52, 353–365. doi: 10.1007/s00374-015-1081-5
Fanin, N., Moorhead, D., and Bertrand, I. (2016). Eco-enzymatic stoichiometry and enzymatic vectors reveal differential C, N, P dynamics in decaying litter along a land-use gradient. Biogeochemistry 129, 21–36. doi: 10.1007/s10533-016-0217-5
Frey, S., Drijber, R., Smith, H., and Melillo, J. (2008). Microbial biomass, functional capacity, and community structure after 12 years of soil warming. Soil Biol. Biochem. 40, 2904–2907. doi: 10.1016/j.soilbio.2008.07.020
Fu, G., Shen, Z. X., Zhang, X. Z., and Zhou, Y. T. (2012). Response of soil microbial biomass to short-term experimental warming in alpine meadow on the Tibetan plateau. Appl. Soil Ecol. 61, 158–160. doi: 10.1016/j.apsoil.2012.05.002
Gong, S. W., Zhang, T., and Guo, J. X. (2019). Warming and nitrogen addition change the soil and soil microbial biomass C:N:P stoichiometry of a meadow steppe. Int. J. Env. Res. Pub. He. 16:2705. doi: 10.3390/ijerph16152705
Gong, S. W., Zhang, T., Guo, R., Cao, H. B., Shi, L. X., Guo, J. X., et al. (2015). Response of soil enzyme activity to warming and nitrogen addition in a meadow steppe. Soil Res. 53, 242–252. doi: 10.1071/SR14140
Harte, J., Torn, M. S., Chang, F. R., Feifarek, B., Kinzig, A. P., Shaw, R., et al. (1995). Climate warming and soil microclimate: results from a meadow-warming experiment. Eco. Appl 5, 132–150. doi: 10.2307/1942058
Hartmann, A. A., Barnard, R. L., Marhan, S., and Niklaus, P. A. (2013). Effects of drought and N-fertilization on N cycling in two grassland soils. Oecologia 171, 705–717. doi: 10.1007/s00442-012-2578
Högberg, M. N., Chen, Y., and Högberg, P. (2007). Gross nitrogen mineralisation and fungi-to-bacteria ratios are negatively correlated in boreal forests. Biol. Fertil. Soils 44, 363–366. doi: 10.1007/s00374-007-0215-9
Hu, Y. L., Wang, S., Niu, B., Chen, Q. Y., Wang, J., Zhao, J. X., et al. (2020). Effect of increasing precipitation and warming on microbial community in Tibetan alpine steppe. Environ. Res. 189:109917. doi: 10.1016/j.envres.2020.109917
IPCC. (2021). Climate change 2021: The physical science basis. Contribution of working group I to the sixth assessment report of the intergovernmental panel on climate change. Cambridge, United Kingdom and New York, NY: Cambridge University Press, 2021.
Jiao, Y. L., Chu, G. M., Yang, Z. A., Wang, Y., and Wang, M. (2020). Bacterial diversity in the rhizosphere of anabasis aphylla in the Gurbantunggut Desert, China. Curr. Microbiol. 77, 3750–3759. doi: 10.1007/s00284-020-02177-y
Jiao, F., Shi, X. R., Han, F. P., and Yuan, Z. Y. (2016). Increasing aridity, temperature and soil pH induce soil C-N-P imbalance in grasslands. Sci. Rep. 6:19601. doi: 10.1038/srep19601
Khan, K. S., Mike, R., Castillo, X., Kaiser, M., and Joergensen, R. G. (2016). Microbial biomology, fungi and bacteria residues and their relationship to the C/N/P/S rate of soil organics. Geoderma 271, 115–123. doi: 10.1016/j.geoderma.2016.02.019
Kuzyakov, Y., and Domanski, G. (2000). Carbon input by plants into the soil. Review. J. Plant Nutr. Soil Sci. 163, 421–431. doi: 10.1002/1522-2624(200008)163:4<421::AID-JPLN421>3.0.CO;2-R
Li, H., Li, J., He, Y. L., Li, S. J., Liang, Z. S., Peng, C. H., et al. (2013). Changes in carbon, nutrients and stoichiometric relations under different soil depths, plant tissues and ages in black locust plantations. Acta Physiol. Plant. 35, 2951–2964. doi: 10.1007/s11738-013-1326-6
Li, H. J., Liu, J. W., Yang, L., Zheng, H. F., Liu, Y., Yang, W. Q., et al. (2016). Effects of simulated climate warming on soil microbial biomass carbon, nitrogen and phosphorus of alpine forest. Chin. J. Appl. Environ. Biol. 22, 599–605. doi: 10.3724/SP.J.1145.2015.12022 (in Chinese with English Abstract)
Li, Y., Wu, J., Liu, S., Shen, J., Huang, D., Su, Y., et al. (2012). Is the C:N:P stoichiometry in soil and soil microbial biomass related to the landscape and landuse in southern subtropical China? Global Biogeochem. Cycle 26, 1–14. doi: 10.1029/2012GB004399
Liu, M., Li, C. C., Xu, X. L., Wanek, W. G., Jiang, N., Wang, H. M., et al. (2017). Organic and inorganic nitrogen uptake by 21 dominant tree species in temperate and tropical forests. Tree Physiol. 37, 1515–1526. doi: 10.1093/treephys/tpx046
Liu, H. Y., Mi, Z. R., Lin, L., Wang, Y. H., Zhang, Z. H., Zhang, F. W., et al. (2018). Shifting plant species composition in response to climate change stabilizes grassland primary production. Proc. Natl. Acad. Sci. U. S. A. 115, 4051–4056. doi: 10.1073/pnas.1700299114
Luo, C. Y., Xu, G. P., Wang, Y. F., Wang, S. P., Lin, X. W., Hu, Y. G., et al. (2009). Effects of grazing and experimental warming on DOC concentrations in the soil solution on the Qinghai-Tibet plateau. Soil Biol. Biochem. 41, 2493–2500. doi: 10.1016/j.soilbio.2009.09.006
Luo, G. W., Xue, C., Jiang, Q. H., Xiao, Y., Zhang, F. G., Guo, S. W., et al. (2020). Soil carbon, nitrogen, and phosphorus cycling microbial populations and their resistance to global change depend on soil C:N:P stoichiometry. mSystems. 5, e00162–e00120. doi: 10.1128/mSystems.00162-20
Manzoni, S., Schaeffer, S. M., Katul, G., Porporato, A., and Schimel, J. P. (2014). A theoretical analysis of microbial eco-physiological and diffusion limitations to carbon cycling in drying soils. Soil Biol. Biochem. 73, 69–83. doi: 10.1016/j.soilbio.2014.02.008
Meng, C., Tian, D. S., Zeng, H., Li, Z. L., Chen, H. Y. H., and Niu, S. L. (2020). Global meta-analysis on the responses of soil extracellular enzyme activities to warming. Sci. Total Environ. 705:135992. doi: 10.1016/j.scitotenv.2019.135992
Mooshammer, M., Wanek, W., Zechmeister-Boltenstern, S., and Richter, A. (2014). Stoichiometric imbalances between terrestrial decomposer communities and their resources: mechanisms and implications of microbial adaptations to their resources. Front. Microbiol. 5, 22–32. doi: 10.3389/fmicb.2014.00022
Nannipieri, P., Giagnoni, L., Renella, G., Puglisi, E., Ceccanti, B., Masciandaro, G., et al. (2012). Soil enzymology: classical and molecular approaches. Biol. Fertil. Soils 48, 743–762. doi: 10.1007/s00374-012-0723-0
Niu, S. L., Wu, M. Y., Han, Y., Xia, J., Li, L., and Wan, S. (2010). Water-mediated responses of ecosystem carbon fluxes to climatic change in a temperate steppe. New Phytol. 177, 209–219. doi: 10.1111/j.1469-8137.2007.02237.x
Saiya-Corka, K. R., Sinsabaugha, R. L., and Zakb, D. R. (2002). The effects of long term nitrogen deposition on extracellular enzyme activity in an Acer saccharum forest soil. Soil Biol. Biochem. 34, 1309–1315. doi: 10.1016/S0038-0717(02)00074-3
Sardans, J., Peñuelas, J., and Estiarte, M. (2008). Changes in soil enzymes related to C and N cycle and in soil C and N content under prolonged warming and drought in a Mediterranean shrubland. Appl. Soil Ecol. 39, 223–235. doi: 10.1016/j.apsoil.2007.12.011
Schaeffer, S. M., Homyak, P. M., Boot, C. M., Roux-Michollet, D., and Schimel, J. P. (2017). Soil carbon and nitrogen dynamics throughout the summer drought in a California annual grassland. Soil Biol. Biochem. 115, 54–62. doi: 10.1016/j.soilbio.2017.08.009
Schindlbacher, A., Rodler, A., Kuffner, M., Kitzler, B., Sessitsch, A., and Zechmeister-Boltenstern, S. (2011). Experimental warming effects on the microbial community of a temperate mountain forest soil. Soil Biol. Biochem. 43, 1417–1425. doi: 10.1016/j.soilbio.2011.03.005
Sinsabaugh, R. L., Hill, B. H., and Follstad, S. J. (2009). Ecoenzymatic stoichiometry of microbial organic nutrient acquisition in soil and sediment. Nature 462, 795–798. doi: 10.1038/nature08632
Stark, S., Männistö, M. K., and Eskelinen, A. (2014). Nutrient availability and pH jointly constrain microbial extracellular enzyme activities in nutrient-poor tundra soils. Plant Soil 383, 373–385. doi: 10.1007/s11104-014-2181-y
Steinweg, J. M., Dukes, J. S., Paul, E. A., and Wallenstein, M. D. (2013). Microbial responses to multi-factor climate change: effects on soil enzymes. Front. Microbiol. 4:146. doi: 10.3389/fmicb.2013.00146
Tan, Q. Q., Wang, G. A., Smith, M. D., Chen, Y. Z., and Yu, Q. (2021). Temperature patterns of soil carbon: nitrogen: phosphorus stoichiometry along the 400 mm isohyet in China. Catena 203:105338. doi: 10.1016/j.catena.2021.105338
Tang, S. R., Cheng, W. G., Hu, R. G., Guigue, J. L., Hattori, S., Tawaraya, K., et al. (2021). Five-year soil warming changes soil C and N dynamics in a single rice paddy field in Japan. Sci. Total Environ. 756:143845. doi: 10.1016/j.scitotenv.2020.143845
Wan, S. Q., Luo, Y. Q., and Wallace, L. L. (2002). Changes in microclimate induced by experimental warming and clipping in tallgrass prairie. Glob. Chang. Biol. 8, 754–768. doi: 10.1046/j.1365-2486.2002.00510.x
Wang, X. X., Dong, S. K., Gao, Q. Z., Zhou, H. K., Liu, S. L., Su, X. K., et al. (2014). Effects of short-term and long-term warming on soil nutrients, microbial biomass and enzyme activities in an alpine meadow on the Qinghai-Tibet plateau of China. Soil Biol. Biochem. 76, 140–142. doi: 10.1016/j.soilbio.2014.05.014
Wang, S., Quan, Q., Meng, C., Chen, W. N., Luo, Y. Q., and Niu, S. L. (2021). Experimental warming shifts coupling of carbon and nitrogen cycles in an alpine meadow. J. Plant Ecol. 14, 541–554. doi: 10.1093/jpe/rtab008
Wieder, W. R., Bonan, G. B., and Allison, S. D. (2013). Global soil carbon projections are improved by modelling microbial processes. Nat. Clim. Chang. 3, 909–912. doi: 10.1038/NCLIMATE1951
Wu, G. L., Huang, Z., Liu, Y. F., Cui, Z., Liu, Y., Chang, X. F., et al. (2019). Soil water response of plant functional groups along an artificial legume grassland succession under semi-arid conditions. Agric. For. Meteorol. 278:107670. doi: 10.1016/j.agrformet.2019.107670
Xiao, G. J., Zhang, Q., Li, Y., Zhang, F. J., and Luo, C. K. (2011). Effect of winter warming on soil moisture and salinization. Trans. CSAE 27, 46–51. doi: 10.3969/j.issn.1002-6819.2011.08.008, (in Chinese with English Abstract)
Xu, Z. F., Wan, C., Xiong, P., Tang, Z., Hu, R., Cao, X. K., et al. (2010). Initial responses of soil CO2 efflux and C, N pools to experimental warming in two contrasting forest ecosystems, Eastern Tibetan Plateau. Plant Soil, 336, 183–195. doi: 10.1007/s11104-010-0461-8
Yang, X. J., Huang, Z. Y., Zhang, K. L., and Cornelissen, J. H. C. (2015). C:N:P stoichiometry of Artemisia species and close relatives across northern China: unraveling effects of climate, soil and taxonomy. J. Ecol. 103, 1020–1031. doi: 10.1111/1365-2745.12409
Yang, K., Ye, B. S., Zhou, D. G., Wu, B. Y., Foken, T., Qin, J., et al. (2011). Response of hydrological cycle to recent climate changes in the Tibetan plateau. Clim. Chang. 109, 517–534. doi: 10.1007/s10584-011-0099-4
Yang, Z. A., Zhan, W., Jiang, L., and Chen, H. (2021). Effect of short-term low-nitrogen addition on carbon, nitrogen and phosphorus of vegetation-soil in alpine meadow. Int. J. Environ. Res. Public Health 18:10998. doi: 10.3390/ijerph182010998
Yang, Z. A., Zhu, Q. A., Zhan, W., Xu, Y. Y., Zhu, E. X., Gao, Y. H., et al. (2018). The linkage between vegetation and soil nutrients and their variation under different grazing intensities in an alpine meadow on the eastern Qinghai-Tibetan plateau. Ecol. Eng. 110, 128–136. doi: 10.1016/j.ecoleng.2017.11.001
Zederer, D. P., Talkner, U., Spohn, M., and Joergensen, R. G. (2017). Microbial biomass phosphorus and C/N/P stoichiometry in forest floor and a horizons as affected by tree species. Soil Biol. Biochem. 111, 166–175. doi: 10.1016/j.soilbio.2017.04.009
Zhang, W. X., Furtado, K., Wu, P. L., Zhou, T. J., Chadwick, R., Marzin, C., et al. (2021). Increasing precipitation variability on daily-to-multiyear time scales in a warmer world. Sci. Adv. 7:eabf8021. doi: 10.1126/sciadv.abf8021
Zhang, J. J., Peng, C. H., Xue, W., Yang, Z. A., Yang, B., Li, P., et al. (2018). Soil CH4 and CO2 dynamics and nitrogen transformation with incubation in mountain forest and meadow ecosystems. Catena 163, 24–32. doi: 10.1016/j.catena.2017.12.005
Zhang, X., Ren, H. Y., Kang, J., Zhu, Y., Li, D. H., and Han, G. D. (2021). Effects of warming and nitrogen addition on soil physical and chemical properties in desert steppe of Inner Mongolia. Chin. J. Grassl. 43, 17–24. doi: 10.16742/j.zgcdxb.20190225, (in Chinese with English Abstract)
Zhang, Q. Y., Shao, M. A., Jia, X. X., and Wei, X. R. (2019). Changes in soil physical and chemical properties after short drought stress in semi-humid forest. Geoderma 338, 170–177. doi: 10.1016/j.geoderma.2018.11.051
Zhang, Q., Wu, J. J., Yang, F., Lei, Y., Zhang, Q. F., and Cheng, X. L. (2016). Alterations in soil microbial commuity composition and biomass following agricultural land use change. Sci. Rep. 6:36587. doi: 10.1038/srep36587
Zhang, S. S., Zheng, Q., Noll, L., Hu, Y. T., and Wanek, W. (2019). Environmental effects on soil microbial nitrogen use efficiency are controlled by allocation of organic nitrogen to microbial growth and regulate gross N mineralization. Soil Biol. Biochem. 135, 304–315. doi: 10.1016/j.soilbio.2019.05.019
Zhao, Y. Y., Xu, L. H., Yao, B. Q., Ma, Z., Zhang, C. H., Wang, F. P., et al. (2016). Influence of simulated warming to the carbon, nitrogen and their stability isotope-(δ13C, δ15N) contents in alpine meadow plant leaves. Acta bot. Boreal.-Occident. Sin. 36, 777–783. doi: 10.7606/j.issn.1000-4025.2016.04.0777
Zhao, C. Z., Zhu, L. Y., Liang, J., Yin, H. J., Yin, C. Y., Li, D. D., et al. (2014). Effects of experimental warming and nitrogen fertilization on soil microbial communities and processes of two subalpine coniferous species in eastern Tibetan plateau. Plant Soil 382, 189–201. doi: 10.1007/s11104-014-2153-2
Zheng, Y., Yang, W., Sun, X., Wang, S. P., Rui, Y. C., Luo, C. Y., et al. (2012). Methanotrophic community structure and activity under warming and grazing of alpine meadow on the Tibetan plateau. Appl. Microbiol. Biotechnol. 93, 2193–2203. doi: 10.1007/s00253-011-3535-5
Zhou, X. Q., Chen, C. R., Wang, Y. F., Xu, Z. H., Duan, J. C., Hao, Y. B., et al. (2013b). Soil extractable carbon and nitrogen, microbial biomass and microbial metabolic activity in response to warming and increased precipitation in a semiarid inner Mongolian grassland. Geoderma 206, 24–31. doi: 10.1016/j.geoderma.2013.04.020
Zhou, X. Q., Chen, C. R., Wang, Y. F., Xu, Z. H., Han, H. Y., Li, L. H., et al. (2013a). Warming and increased precipitation have differential effects on soil extracellular enzyme activities in a temperate grassland. Sci. Total Environ. 444, 552–558. doi: 10.1016/j.scitotenv.2012.12.023
Zhu, X. M., Liu, M., Kou, Y. P., Liu, D. Y., Liu, Q., Zhang, Z. L., et al. (2020). Differential effects of N addition on the stoichiometry of microbes and extracellular enzymes in the rhizosphere and bulk soils of an alpine shrubland. Plant Soil 449, 285–301. doi: 10.1007/s11104-020-04468-6
Zi, H. B., Hua, L., Wang, C. T., Wang, G. X., Wu, P. F., Lerdauca, M., et al. (2018). Responses of soil bacterial community and enzyme activity to experimental warming of an alpine meadow. Eur. J. Soil Sci. 69, 429–438. doi: 10.1111/ejss.12547
Keywords: ecological stoichiometric characteristics, extracellular enzyme activities, warming, isolation from precipitation, soil moisture content
Citation: Tong Y, Long Y and Yang Z (2023) Effects of warming and isolation from precipitation on the soil carbon, nitrogen, and phosphorus, and their stoichiometries in an alpine meadow in the Qinghai–Tibet Plateau: A greenhouse warming study. Front. Ecol. Evol. 11:1149240. doi: 10.3389/fevo.2023.1149240
Edited by:
Zhongqing Yan, Chinese Academy of Forestry, ChinaReviewed by:
Lie Yang, Wuhan University of Technology, ChinaYongheng Gao, Chengdu Institute of Biology (CAS), China
Copyright © 2023 Tong, Long and Yang. This is an open-access article distributed under the terms of the Creative Commons Attribution License (CC BY). The use, distribution or reproduction in other forums is permitted, provided the original author(s) and the copyright owner(s) are credited and that the original publication in this journal is cited, in accordance with accepted academic practice. No use, distribution or reproduction is permitted which does not comply with these terms.
*Correspondence: Zhen’an Yang, eXphMjc2NUAxMjYuY29t