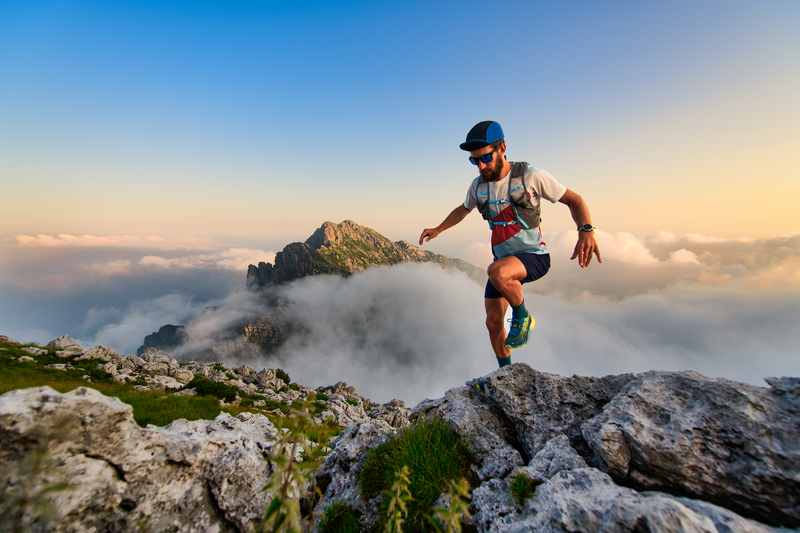
95% of researchers rate our articles as excellent or good
Learn more about the work of our research integrity team to safeguard the quality of each article we publish.
Find out more
ORIGINAL RESEARCH article
Front. Ecol. Evol. , 24 May 2023
Sec. Biogeography and Macroecology
Volume 11 - 2023 | https://doi.org/10.3389/fevo.2023.1144778
This article is part of the Research Topic Environmental Threats to the State of Florida—Climate Change and Beyond, volume II View all 10 articles
Introduction: Cultural eutrophication threatens numerous ecological and economical resources of Florida’s coastal ecosystems, such as beaches, mangroves, and seagrasses. In April 2021, an infrastructure failure at the retired Piney Point phosphorus mining retention reservoir garnered national attention, as 814 million liters of nutrient rich water were released into Tampa Bay, Florida over 10 days. The release of nitrogen and phosphorus-rich water into Tampa Bay – a region that had been known as a restoration success story since the 1990s – has highlighted the potential for unexpected challenges for coastal nutrient management.
Methods: For a year after the release, we sampled bi-weekly at four sites to monitor changes in nutrients, stable isotopes, and phytoplankton communities, complemented with continuous monitoring by multiparameter sondes. Our data complement the synthesis efforts of regional partners, the Tampa Bay and Sarasota Bay Estuary Programs, to better understand the effects of anthropogenic nutrients on estuarine health.
Results: Phytoplankton community structure indicated an initial diatom bloom that dissipated by the end of April 2021. In the summer, the bay was dominated by Karenia brevis, with conditions improving into the fall. To determine if there was a unique carbon (C) and nitrogen (N) signature of the discharge water, stable isotope values of carbon (δ13C) and nitrogen (δ15N) were analyzed in suspended particulate material (SPM). The δ15N values of the discharge SPM were −17.88‰ ± 0.76, which is exceptionally low and was unique relative to other nutrient sources in the region. In May and early June of 2021, all sites exhibited a decline in the δ15N values of SPM, suggesting that discharged N was incorporated into SPM after the event. The occurrence of very low δ15N values at the reference site, on the Gulf Coast outside of the Bay, indicates that some of the discharge was transported outside of Tampa Bay.
Discussion: This work illustrates the need for comprehensive nutrient management strategies to assess and manage the full range of consequences associated with anthropogenic nutrient inputs into coastal ecosystems. Ongoing and anticipated impacts of climate change – such as increasing tropical storm intensity, temperatures, rainfall, and sea level rise – will exacerbate this need.
Cultural eutrophication and climate change are two of the most important threats to the health and sustainability of coastal ecosystems around the world (Nixon, 1995; Hoegh-Guldberg and Bruno, 2010; Doney et al., 2012). From an algal perspective, cultural eutrophication has elevated the potential for harmful algal blooms (HABs; Cloern, 2001; Paerl et al., 2006; Heisler et al., 2008; Glibert, 2020; Gobler, 2020). Current trends in climatic conditions are exacerbating the challenges associated with eutrophication due to increases in temperature, changes in rainfall patterns, and increases in the intensity of tropical storms (Webster et al., 2005; Oneil et al., 2012; Wetz and Yoskowitz, 2013; Glibert et al., 2014; Griffith and Gobler, 2020; Phlips et al., 2020). One of the potential mechanisms for the combined impacts of eutrophication and climate change is the disruption of engineered structures associated with water treatment and retention (Lehner et al., 2011; Beusen et al., 2015; Grill et al., 2015; Maavara et al., 2015). Accidental or unavoidable discharges from compromised infrastructure can expose surrounding aquatic environments to excessive nutrient, algal and pollutant loads that negatively affect water quality, including elevated risks for HABs (Sin et al., 2013; Phlips et al., 2020; Herren et al., 2021; Metcalf et al., 2021). In this study, we examined an emergency release of water from a retired phosphorus mining reservoir into Tampa Bay, Florida and evaluated changes in water quality and algal populations in the Bay a year after the event.
Tampa Bay was designated as an impaired coastal waterbody in the 1980s, in part because of widespread losses of seagrasses. Subsequent restoration efforts and targeted nutrient management strategies resulted in successful restoration of seagrass habitats by the end of the century (Yates et al., 2011; Greening et al., 2014; Sherwood et al., 2017; Tomasko et al., 2018; Beck et al., 2019; DeAngelis et al., 2020; Tomasko et al., 2020). Despite these successes, nutrient management is an ongoing challenge due to increased development, reclaimed water usage, and septic and industrial activities adjacent to the Bay. Recently, higher shallow water temperatures and relatively high, sustained hydrologic inputs potentially linked to climate change drivers may also be confounding nutrient management efforts (Tampa Bay Nitrogen Management Consortium (TBNMC), 2022). During the 2016–2022 period, significant seagrass coverage was lost according to aerial photography estimates (>25% decline from 2016 peak coverage, or > 11,000 acres of seagrass coverage loss; SWFWMD, unpublished data). In addition to this recent bay-wide and regional seagrass loss, water quality declines in the northern portion of the bay (Old Tampa Bay) have occurred, and unexpected events, such as periodic releases of industrial process water, have caused further management challenges (Tampa Bay Estuary Program, 2022).
In late March 2021, an impaired liner at a decommissioned fertilizer facility (Piney Point) prompted the emergency release of 814 million liters of process water mixed with dredge water into the Bay, from March 30th to April 9th, 2021 (Nelson et al., 2021; Beck et al., 2022). The discharge water was high in inorganic nutrients, specifically ammonium and orthophosphate, prompting concerns that this pulse of nutrients might result in increased primary productivity, including phytoplankton, macroalgae and HABs, with adverse effects to seagrass meadows and other coastal habitats. Initial analyses soon after the event determined that there was a localized diatom bloom, and that the excess nutrients within the bay may have exacerbated the development of a red tide (Karenia brevis) bloom that was transported into the Bay (Beck et al., 2022).
While the initial effects of the discharge have been reported, the long-term effects on water quality and phytoplankton community structure have not yet been investigated. Here, we further investigate the fate of nutrients released from Piney Point and characterize the water quality conditions and phytoplankton community composition for the year following the event. We hypothesized that the initial pulse of inorganic nutrients was readily utilized by phytoplankton communities. This likely led to internal cycling of Piney Point-derived nutrients within the Bay, although other mechanisms, such as the deposition of nutrients into bay sediments and transport of nutrients outside of the bay were also likely important mechanisms influencing the fate of nutrients discharged from the facility. To evaluate this hypothesis, we monitored changes in water column nutrients, in situ water quality parameters, stable isotopes of carbon (C) and nitrogen (N) in suspended particulate material (SPM), chlorophyll a concentrations, and phytoplankton community structure over the course of a year after the event.
Our year-long monitoring campaign confirmed there was an initial diatom bloom adjacent to the location of the discharge that dissipated by the end of April 2021, as previously described in Beck et al. (2022), and revealed that elevated diatom biomass was more extensive than previously recognized as it extended to back bay regions. During the summer, the site adjacent to Piney Point had high K. brevis biomass (3 mg C L−1), which then declined into the fall. We found that the stable isotope values of C and N (δ13C and δ15N) of the discharge water SPM were-15.23‰ ± 0.53 and-17.88‰ ± 0.76, respectively. A δ15N value of-17.88‰ is exceptionally low and was unique relative to other nutrient sources in the region, likely due to isotopic fractionation associated with ammonium assimilation within the reservoir. In May and early June of 2021, all sites in the discharge region exhibited a sharp decline in the δ15N values of SPM, suggesting that discharge N was incorporated into SPM after the event, which may have been driven either by phytoplankton uptake of N and/or N sorption onto particulate material in the bay. This was further supported by concomitant declines in C:N values. After mid-June 2021, δ15N values generally returned to April 2021 values. This study found that phytoplankton communities and water quality were altered by the Piney Point event and that these dynamics can be influenced by tropical storms, highlighting the synergistic effects between disruptions of engineered structures and periodic events such as storms, which are predicted to increase in intensity due to climate change (Webster et al., 2005; Wetz and Yoskowitz, 2013).
Four sites were selected in consultation with the Tampa Bay Estuary Program (TBEP) and the University of South Florida’s Tampa Bay Coastal Ocean model (Chen et al., 2018, 2019). The sites included: one site proximal to Piney Point Creek, which is connected to the Piney Point facility via drainage canals (hereafter referred to as Piney Point), two back bay regions that were located south of the discharge location and forecast to have longer residence times (Bishop Harbor and Joe Bay), and one reference site outside of the Bay (St. Joseph Sound; Figure 1). All sites were located adjacent to TBEP seagrass monitoring transects (Beck et al., 2022) and had depths that varied with the tidal cycle, but generally ranged from 1–3 m. One of the back bay regions (Bishop Harbor) was the site of previous releases from the Piney Point facility (Garrett et al., 2011; Switzer et al., 2011), and the reference site was outside of the Bay, at a relatively pristine location with healthy seagrass meadows (Tomasko et al., 2020). For this event, emergency releases were conveyed directly into Tampa Bay in the vicinity of Port Manatee from March 30th to April 9th 2021, while an uncontrolled discharge to Piney Point creek occurred from March 30th to April 3rd, 2021 (Florida Department of Environmental Protection, 2021; Figure 1).
Figure 1. (A) Location of the Piney Point facility and study sites within and outside Tampa Bay. (B) Lower bay sites and their location in relationship to Piney Point and the discharge locations. Discharges occurred at Port Manatee from March 31st to April 9th, and at Piney Point Creek until April 3rd. Sites are marked with yellow points, locations of interest are marked with red points.
Water samples were collected on a bi-weekly basis from April 2021 until April 2022. At each site, water samples were collected from surface to near bottom (to avoid collection of bottom material) on a near bi-weekly basis using a depth-integrated pole sampling method (Phlips et al., 2010) to minimize any bias from water column stratification. Water samples were collected for total and dissolved nutrients, suspended particulate material (SPM), chlorophyll a, and phytoplankton community analyses, as described below. In addition to bi-weekly water samples, a discharge water sample was collected on April 7th, 2021, and processed as described for other water samples. An additional sample collection was conducted in October 2021 at the southern holding pond where the initial liner tear occurred to evaluate seasonal changes in reservoir characteristics and efforts to employ innovative treatment technologies within the remaining wastewater held at the facility following the emergency release. All samples were transported on ice and either refrigerated or frozen until analyzed.
Water samples were collected and filtered for chlorophyll a analysis in the field. Phytoplankton were filtered onto 0.7-μm Whatman glass fiber filter and stored in a dark container at −20°C. Chlorophyll a was solvent extracted (Sartory and Grobbelaar, 1984) and measured spectrophotometrically according to Standard Methods (American Public Health Association, 2005).
Integrated, whole water samples were preserved on-site with Lugol’s solution (American Public Health Association, 2005) and analyzed microscopically for phytoplankton abundance and species composition. General phytoplankton abundance and composition were determined using the Utermöhl method (Utermohl, 1958), as described in Badylak et al. (2014). Samples preserved in Lugol’s were settled in 19 mm diameter cylindrical chambers. Phytoplankton cells were identified and counted at 400× and 100× with a Leica phase contrast inverted microscope. At 400×, a minimum of 100 cells of a single taxon and 5 grids were counted. If 100 cells were not counted by 30 grids, up to a maximum of 100 grids were counted until 100 cells of a single taxon were reached. At 100×, a total bottom count was completed for taxa >30 μm in size.
Picocyanobacteria abundances were determined using a Zeiss Axio compound microscope, using green and blue light excitation (Fahnenstiel and Carrick, 1992; Phlips et al., 1999). Samples were preserved with buffered glutaraldehyde. Subsamples of water were filtered onto 0.2 μm Nucleopore filters and mounted between a microscope slide and cover slip with immersion oil and picoplankton counted at 1000x magnification.
Count data were converted to phytoplankton biovolume, using the closest geometric shape method (Smayda, 1978; Sun and Liu, 2003). Phytoplankton C values (as mg C L−1) were estimated by applying conversion factors for different taxonomic groups to biovolume estimates (expressed as 106 μm3 mL−1): i.e., 0.065 × biovolume of diatoms, 0.22 x biovolume for cyanobacteria, and 0.16 × biovolume for dinoflagellates or other taxa (Strathmann, 1967; Ahlgren, 1983; Sicko-Goad et al., 1984; Verity et al., 1992; Work et al., 2005).
A YSI EXO2 multiparameter sonde was deployed at each of the four study sites on April 16th, 2021, soon after the discharge ceased on April 9th. Deployment depth ranged from 1–3 m, depending on the site. Sondes continuously measured salinity, optical dissolved oxygen (DO), in situ chlorophyll, phycoerythrin (PE), fluorescent dissolved organic matter (fDOM), specific conductivity, temperature, pH, total dissolved solids, turbidity, and total suspended solids every 10 min. The average value for each day is reported here. Sondes were inspected on a bi-weekly basis, with maintenance and calibration occurring every ~3 weeks, or sooner if needed, according to the manufacturer’s instructions. Data were downloaded from sondes during bi-weekly sampling trips using KorEXO software. Data from April 16th, 2021 to May 5th, 2022 were aggregated and cleaned for this study using R version 4.1 (R Core Development Team, 2008). Values that were out of sensor range were flagged and removed from the dataset prior to analysis. For all parameters, approximately 1% or less of the values were out of range, except for in situ chlorophyll where 9.8% of the values were out of range.
Water samples collected for total phosphorus (TP) and total Kjeldahl nitrogen (TKN) were acidified to a pH of 2 in the field prior to analysis and analyzed within 28 days according to EPA Method 365.1 and 353.2, respectively. Water samples for ammonium-N (NH4-N) and nitrate + nitrite (NOx) analyses were 0.2 μm filtered and acidified to a pH of 2 in the field and analyzed within 28 days according to EPA Method 350.1. Water samples for total orthophosphate (orthoP) were not acidified and were analyzed within 48 h according to EPA Method 365.1. All nutrient analyses were certified and conducted at the University of Florida’s Analytical Research Laboratory, a National Environmental Laboratory Accreditation Program (NELAP) certified facility. Values below the minimum detection limit were set to NA prior to analysis.
Suspended particulate material was collected on pre-combusted, pre-weighed glass fiber filters (GF/F), frozen and then freeze dried. Filters were then placed into tin capsules for elemental (total carbon (TC) and total nitrogen (TN)) and stable isotope (δ13C, δ15N) analysis. Elemental and stable isotope analyses were conducted at the University of Florida’s Stable Isotope Laboratory, using a Carlo Erba 1500 CN elemental analyzer coupled to a Thermo Electron DeltaV Advantage isotope ratio mass spectrometer (Carlo Erba/ThermoFisher Scientific™, Waltham, MA, United States). Stable isotope ratios are reported for δ13C and δ15N in standard delta notation (‰) relative to Vienna Pee Dee Belemnite (VPDB) and atmospheric N2 standards, respectively. Total carbon and TN are reported on a percent mass basis. The C:N ratio is reported as the mass ratio, i.e., weight %TC ÷ weight %TN.
Data analysis was conducted in R version 4.1 (R Core Development Team, 2008). Grab sample values (i.e., chlorophyll a, phytoplankton, elemental analysis and isotope values) were averaged by location and month, while in situ sonde measurements were averaged by location and day. Trends in these values were then examined to elucidate the timing of maximum and minimum values relative to the emergency release and Tropical Storm Elsa, as well as variation between sites and relative to published values. Analysis scripts are available at the following GitHub repository.1
Chlorophyll a concentrations were used as one of the indicators of phytoplankton biomass. Overall temporal trends in chlorophyll a concentrations were similar at the three lower bay sampling sites, with concentrations mostly over 5 μg L−1 from April through August 2021 (Figure 2), exceeding an annual average lower bay management target of 4.6 μg L−1 (Tampa Bay Estuary Program, 2022). Peaks in chlorophyll a during the latter period of April–August reached values up to 20 μg L−1 at Piney Point. After summer, chlorophyll a declined to below 5 μg L−1 through the end of the study period, with a few exceptions. By contrast, chlorophyll a concentrations at the St. Joseph Sound reference site were consistently below 3 μg L−1 except for moderately elevated concentrations in July and August of 2021, coincident to red tide blooms that extended along the Southwest Florida coast during this time. Lower Tampa Bay values exceeded the 2006 to 2020 long-term median chlorophyll a value of lower Tampa Bay, which was 3.1 μg L−1 (min 2.3 μg L−1, max 3.5 μg L−1; Beck et al., 2022), but values of the reference site were similar to the long-term median value of the Lower Tampa Bay (3.1 μg L−1).
Figure 2. Chlorophyll a concentrations (μg L−1) at the three lower bay sampling sites (i.e., Bishop Harbor, Joe Bay, and Piney Point), and reference site (i.e., St. Joseph Sound).
On April 7th, 2021, emergency release water was dominated by a spherical single-celled green alga (Chlorophyta). Cell density of the green alga was 3.4 × 108 cells L−1, and biomass was 2.34 mg C L−1, almost an order of magnitude higher than the mean biomass for the study period in the Piney Point nearshore basin which was 0.36 mg C L−1. The discharge sample also contained several other species of nanophytoplankton, but the biomass contributions of these taxa were minor, i.e., < 0.02 mg C L−1.
The time-series of total phytoplankton biomass for the three lower bay sampling sites (i.e., Piney Point, Bishop Harbor, and Joe Bay) from April 9th, 2021, through May 2022 exhibited similar temporal patterns, with elevated levels in the late Spring of 2021, with peaks near, or over, 1.5 mg C L−1 (Figure 3). In mid-summer, biomass levels declined, and remained near, or below, 0.5 mg C L−1 through the end of the study period in May 2022. The reference site in St. Joseph Sound had modestly elevated total biomass in June and July, with peaks near 0.5 mg C L−1, then remained below 0.3 mg C L−1 for the rest of the study period. Mean total phytoplankton biomass values for the study period were similar across the three lower bay sampling sites, while the reference site exhibited the lowest value – although statistically it could not be differentiated from the Bishop Harbor or Piney Point sites (Table 1).
Figure 3. Time series of phytoplankton biomass (mg carbon L−1) for the four core sites, i.e., Bishop Harbor, Joe Bay, Piney Point, and St. Joseph Sound (reference site). Time series are divided into four phytoplankton groups, dinoflagellates (red), diatoms (yellow), cyanobacteria (blue), and all “other” taxa (green). Bottom panels for each site show relative (%) contribution of each group to total biomass.
Table 1. Mean biomass of four groups of phytoplankton (i.e., dinoflagellates, diatoms, cyanobacteria and all “other” taxa) at the four sampling sites (i.e., Bishop Harbor, Joe Bay, Piney Point and St. Joseph Sound) over the entire study period.
The initial peaks in phytoplankton biomass at the Bishop Harbor, Joe Bay and Piney Point sites in May 2021 were dominated by diatoms (Figure 3). Three diatom taxa, Leptocylindrus minimus, Leptocylindrus danicus, and Cerataulina pelagica, dominated the initial peak period in terms of biomass (Table 2). The subsequent major peak in biomass at the Piney Point site in June was dominated by the toxic dinoflagellate Karenia brevis and reached 3 mg C L−1. The more modest peaks in biomass at St. Joseph Sound in May and June of 2022 were dominated by dinoflagellates, including K. brevis in June. After July 2022, the Bishop Harbor, Joe Bay, and Piney Point sites showed variability in dominant taxa, with periods of supremacy by all four major phytoplankton groups, i.e., dinoflagellates, diatoms, cyanobacteria (primarily picocyanobacteria), and “other” taxa (most prominently nanophytoplankton, such as cryptophytes; Figure 3; Table 2).
Table 2. List of Top-50 individual biomass observations for individual taxa over the study period at the reference site, St. Joseph Sound (top panel), and Top-150 for the combined record for the three lower bay sites, i.e., Bishop Harbor, Joe Bay, and Piney Point (bottom panel).
Over the study period, mean biomass of diatoms were higher than dinoflagellates, cyanobacteria and “other” taxa at Bishop Harbor and Joe Bay (Table 1). At Piney Point, mean dinoflagellate and diatom biomass was higher than cyanobacteria and “other” taxa. At St. Joseph Sound, there were no significant differences in mean biomass of the four phytoplankton groups. In terms of regional differences within each phytoplankton group, mean dinoflagellate and cyanobacteria biomass levels across all the sites were not significantly different, despite the high mean value of dinoflagellates at the Piney Point site (Table 1). The apparent anomalously high annual mean at Piney Point reflects the effect of exceptionally high biomass of K. brevis in June on the mean. Joe Bay had a significantly higher mean diatom biomass than at St. Joseph Sound. For “other” taxa, Joe Bay had the highest mean biomass and St. Joseph Sound had the lowest mean biomass.
To examine more specific differences in taxonomic composition at the reference and lower study sites, a comparison was made of the individual taxa that accounted for the top 10% of biomass observations in the two regions, which roughly represented the Top-50 taxa observations at the St. Joseph Sound (reference site), and the Top-150 taxa observations for the combined results from the Bishop Harbor, Joe Bay and Piney Point sites (lower bay sites; Table 2). There were several key similarities, including the strong representation of cyanobacteria on both lists, and many similarities in the list of dinoflagellate species, including the prominence of the HAB species Karlodinium veneficum and K. brevis. These similarities fall in line with the lack of significant differences between sites for mean values of cyanobacteria and dinoflagellates biomass over the study period (Table 1). By contrast, for diatoms, there was a wider range of species and higher biomass values for the lower bay sites than the reference site (Table 2). The Top-150 list for the lower bay sites was led by spherical picoplanktonic cyanobacteria, other undefined small nanoplanktonic eukaryotes and the euryhaline cosmopolitan diatom species Skeletonema costatum, L. danicus and Rhizosolenia setigera, in terms of frequency on the list (Table 2). In terms of highest biomass observations, the dinoflagellate K. brevis, and the diatoms L. minimus, L. danicus, Guinardia delicatula, Skeletonema costatum, and C. pelagica led the Top-150 list, with peak biomass values greater than 0.40 mg C L−1.
The Top-50 list for the St. Joseph Sound reference site was led by spherical picoplanktonic cyanobacteria, other undefined small nanoplanktonic eukaryotes, and the dinoflagellate Karlodinium veneficum, in terms of frequency on the list (Table 2). for the three primary sampling sites. In terms of highest biomass observations in the Top-50 list for the St. Joseph Sound reference site, only K. brevis had a peak value similar to that encountered in the Top-150 list, i.e., 0.25 mg C L−1.
Another feature of the Top-50 and Top-150 lists of highest biomass observations was the presence of known HAB species, most of which are potential producers of toxins (Lassus et al., 2015; Table 2). The largest number of HAB species for both lists were dinoflagellates. K. veneficum, K. brevis, Takayama sp. and Akashiwo sanguinea were near the top of both lists in terms of HAB species. K. brevis had the highest peak biomass for HAB species in both lists, with a peak of 2.71 mg C L−1 (i.e., 3.9 million cells L−1) on the Top-150 list, which represents a major bloom. The potentially toxic filamentous nitrogen-fixing cyanobacterium Trichodesmium erythraeum and pennate diatom Pseudo-nitzschia sp. were on both lists. Karenia brevis was the only HAB species that reached major levels of concern in terms of harmful impact during this study period.
On April 6th, 2021, the water discharged from the facility was dominated by inorganic nutrients, namely ammonium-N (210 mg L−1) and orthophosphate (140 mg L−1), with NOx concentrations of 0.004 mg L−1 (Supplementary Table 1; Beck et al., 2022). All sites exhibited their greatest orthoP values in April 2021, with lower orthoP concentrations seen at sites further from the discharge site at that time (Supplementary Tables 1, 2). OrthoP values were greater in April 2021 when compared to April 2022 for all sites. All sites also exhibited their highest average total P values in April 2021 and had lower TP values in April 2022 when compared to April 2021, except for the reference site St. Joseph Sound. St. Joseph Sound had slightly lower total P values in April 2021 (11.38 μg L−1) when compared to April 2022 (13.71 μg L−1). For all sites, ammonium-N was highest in the first 5 months after the event, and in January 2022 with concentrations peaking in June and July of 2021 and in January 2022. Average monthly ammonium-N values in the lower Tampa Bay sites were from 0.14 mg L−1 (Piney Point and Bishop Harbor) to 0.17 mg L−1 (Joe Bay). Generally, NOx was not detectable at our sites (Supplementary Tables 1, 2).
For in situ measurements, there were distinct differences between the study sites. Salinity was consistently higher at the reference site, St. Joseph Sound (lowest 27.04 psu in mid-February 2022 and highest in early June 2021 34.96 psu) relative to the lower bay sites (0.66 to 34.94 psu). Salinity was highest at the beginning of the study period (Figure 4A), particularly for Piney Point and Bishop Harbor, which are further into the Bay. Joe Bay (closer to the mouth of Tampa Bay), and St. Joseph Sound (reference site) had increases in salinity through May and June 2021, then a decline in salinity in June and July 2021. Joe Bay’s salinity was lowest in mid-August 2021 (24.70 psu), and greatest in mid-June 2021 (34.94 psu). Piney Point’s salinity was lowest in early July 2021 (16.98 psu), likely due to Tropical storm Elsa, and greatest in early June 2021 (33.13 psu). Bishop Harbor’s salinity dipped in October 2021 to 0.66 psu, and its highest salinity was at the end of May 2021 (34.47 psu; Figure 4A).
Figure 4. In situ measurements for daily means of (A) salinity (psu), (B) dissolved oxygen (mg L−1), (C) in situ chlorophyll (μg L−1), and (D) phycoerythrin (relative fluorescence units, RFU) over the course of the study. The Piney Point event (March 30th – April 9th, 2021) is denoted by the green line, the period with maximum (> 105 cells L−1) Karenia brevis cell counts (Beck et al., 2022) is shown in the red box, and the date of tropical storm Elsa (July 5th, 2021) is shown with the vertical dashed black line. The smoothing line was generated using local polynomial regression fitting with a span of 0.175.
Dissolved oxygen (DO) was greatest at the reference site, St. Joseph Sound, and at Piney Point, while DO was lower in the back bay sites (Figure 4B). DO values tended to be lowest in mid- to late summer of 2021 and were highest from December 2021 to February 2022. All sites exhibited a slight decline in DO into the summer, and experienced increases in DO starting in October 2021, with a peak in DO in early 2022. The lowest daily mean DO at Bishop Harbor was at the end of September 2021 (1.52 mg L−1) and greatest in early February 2022 (8.98 mg L−1). The lowest DO at Joe Bay was in mid-June (3.45 mg L−1) and highest in December 2021 (8.63 mg L−1). The lowest DO at Piney Point was at the end of June (4.64 mg L−1) and greatest in early July 2021 (10.27 mg L−1; Figure 4B), at the time of peak K. brevis blooms in the Bay. The lowest DO at the reference site St. Joseph Sound was at the end of September 2021 (4.06 mg L−1) and greatest in February 2022 (9.90 mg L−1).
In situ chlorophyll was consistently higher when compared to extracted chlorophyll a (described above), and was greatest at Joe Bay and Piney Point, both of which exhibited an increase in June and July 2021. In general, sites exhibited the greatest in situ chlorophyll values in mid-summer 2021, during the period of peak K. brevis blooms, and lowest values in the late fall and winter (Figure 4C). At Bishop Harbor, in situ chlorophyll was greatest in early September 2021 (24.99 μg L−1), and lowest in late December 2021 (0.59 μg L−1). At Joe Bay, the greatest in situ chlorophyll values were in late June 2021 (36.96 μg L−1), and the lowest values were in early December 2021 (0.95 μg L−1). At the Piney Point site, the greatest in situ chlorophyll was in early July 2021 (30.21 μg L−1), and the lowest values were in mid-December 2021 (2.55 μg L−1). At St. Joseph Sound, the greatest in situ chlorophyll values were in mid-July 2021 (24.4 μg L−1), and the lowest values were in mid-October 2021 (0.03 μg L−1).
Phycoerythrin (PE) was greatest at Piney Point and Joe Bay, which had spikes in PE in June and July of 2021 (Figure 4D). Similar to in situ chlorophyll, PE was greatest in mid-summer 2021, corresponding with K. brevis blooms in the region, and lowest in the winter months. At Bishop Harbor, PE was greatest in early September 2021 (15.55 relative fluorescent units [RFU]) and lowest in late November 2021 (0.63 RFU). At Joe Bay, PE was greatest in mid-June 2021 (15.81 RFU) and lowest in early December (0.69 RFU). At Piney Point, PE was greatest in early July (14.74 RFU) and lowest in mid-May (1.32 RFU). At St. Joseph Sound, the greatest PE values were in mid-July (9.74 RFU), and lowest values were in mid-December (0.05 RFU).
Florescent dissolved organic matter (fDOM) was consistently higher in the back bay sites Bishop Harbor and Joe Bay, and lowest in the reference site, St. Joseph Sound. All lower bay sites exhibited an increase in fDOM in late July 2021 (Supplementary Figure 1). At Bishop Harbor the lowest value was early October 2021 (10.97 quinine sulfate units [QSU]) and greatest in mid-August 2021 (54.54 QSU). Joe bay was lowest in March 2022 (0.67 QSU) and highest in late July (42.84 QSU). Piney Point was lowest in mid-April 2022 (15.11 QSU) and greatest in mid-August 2021 (55.84 QSU). St. Joseph Sound was lowest in mid-December 2021 (5.15 QSU) and greatest in mid-October 2021 (22.34 QSU).
Patterns of turbidity varied by region (Supplementary Figure 1). The turbidity at Bishop Harbor was lowest in mid-August 2021 [0.31 formazin nephelometric units (FNU)] and greatest in early September 2021 (22.71 FNU), which coincided with higher in situ chlorophyll and PE values. The turbidity at Joe Bay was lowest in late January 2022 (0.70 FNU) and greatest in mid-September 2021 (42.59 FNU). Piney Point had the lowest turbidity in mid-August 2021 (0.41 FNU) and greatest in late November 2021 (68.12 FNU). St. Joseph Sound had the lowest turbidity in mid-October 2021 (0.29 FNU) and greatest in mid-July 2021 (82.76 FNU).
The discharge water SPM had an exceptionally depleted δ15N value of −17.88‰ ± 0.76, and a δ13C value of −15.23‰ ± 0.53. Stable isotope values were determined with a precision of 0.05‰ and 0.09‰ for δ15N and δ13C, respectively. The total nitrogen (TN) of the discharge water SPM was 2.8% ± 0.1, and total carbon (TC) was 11.9% ± 0.5, with a C:N ratio of 4.3 ± 0.2. The SPM of the reservoir water in October had similarly low δ15N and δ13C values as the discharge water from April, −19.15‰ ± 0.05 and −13.44 ‰ ± 0.03, respectively. Differences between the SPM from April discharge and October reservoir samples are likely due to the fact that on-site treatment technologies were being employed at the site since April 2021.
The greatest particulate TC values (2.88–3.77%) were seen in April 2021 for three of the four sites and then declined over the course of the study period (Figure 5A). The only exception was Piney Point, which exhibited its highest TC values (2.4% ± 1.3) in June 2021 coinciding with the K. brevis bloom in the bay and had its second highest TC in April 2021 (average 1.8% ± 0.5; max 2.6%), concurrent with the release from the Piney Point facility. The lowest TC values at Bishop Harbor and St. Joseph Sound site were seen in mid-July 2021 (0.2 ± 0.02%). A similar trend was seen in TN values, where Bishop Harbor and Joe Bay exhibited their greatest TN values in April 2021 (0.3–0.46%) Piney Point had its greatest particulate TN value in June 2021 (0.32% ± 0.10; Figure 5B). After early July 2021, sites exhibited a decline in particulate TN, with the exception of St. Joseph Sound, which exhibited an increase in particulate TN in August 2021, followed by a decline.
Figure 5. Bulk measures for suspended particulate material (SPM) for each location over the study period. Values for (A) total suspended particulate carbon, (B) total suspended particulate nitrogen, and (C) Carbon to Nitrogen (C:N) ratios are presented for each site. The Piney Point event (March 30th – April 9th, 2021) is denoted by the green line, the period with maximum (> 105 cells L−1) Karenia brevis cell counts (Beck et al., 2022) is shown in the red box, and the date of tropical storm Elsa (July 5th, 2021) is shown with the vertical dashed black line. The smoothing line was generated using local polynomial regression fitting with a span of 0.175.
Over the study period, the lower Tampa Bay sites showed similar trends in C:N values, with all sites exhibiting a higher C:N value in April 2021, with a subsequent decline in C:N to a value of ~5 in June 2021 (Figure 5C). After June 2021, C:N values of the bay sites increased and then stabilized from July 2021 to April 2022. Patterns in C:N were slightly different at the reference site, St. Joseph Sound, which also had high C:N values in April 2021, and a subsequent decrease in June 2021. However, in July 2021 there was a decline in C:N at St. Joseph Sound, likely driven by an influx of N derived from the surrounding watershed in association with Tropical Storm Elsa’s passing. Values then quickly increased and plateaued, until another decline in C:N was seen in January 2022 at St. Joseph Sound. The lower Tampa Bay sites also exhibited a slight decrease in C:N at this time, but to lesser degrees.
All sites exhibited similar trends from April to December 2021 for δ15N in SPM (Figure 6A). Specifically, δ15N values declined in all regions in May 2021, with values as low as −11.4‰ at Bishop Harbor, and −10.8‰ ± 0.02 at Piney Point, while the lowest average values at the reference site, St. Joseph Sound (−8.75‰ ± 10.8). and Joe Bay (−4.28‰ ± 7.7), were seen in June 2021. The decline in δ15N values may be related to mixing and/or uptake of N from the Piney Point discharge since the SPM δ15N value of the Piney Point discharge in April 2021 was −17.88‰ ± 0.76. Additionally, the Bishop Harbor and St. Joseph Sound sites had an increase in δ15N values in January 2022, which coincided with a drop in C:N values, possibly driven by terrestrial inputs of N, or remineralization by macroalgae in the region.
Figure 6. Stable isotope values for (A) nitrogen and (B) carbon in suspended particulate material (SPM). Values from the Piney Point discharge are shown with the horizontal green line. The Piney Point event (March 30th – April 9th, 2021) is denoted by the green line, the period with maximum (>105 cells L−1) Karenia brevis cell counts (Beck et al., 2022) is shown in the red box, and the date of tropical storm Elsa (July 5th, 2021) is shown with the vertical dashed black line.
In April 2021, δ13C values were consistently low at all sites ranging from −26.1 ± 3.3 at St. Joseph Sound to −22.6‰ ± 0.9 at Joe Bay. An increase in δ13C values was seen in May 2021 (Figure 6B), possibly driven by C input from SPM within the Piney Point discharge, which had δ13C values ranging from −14.01 to −15.82‰.
Temporal trends in phytoplankton composition and biomass offer insights into possible effects of the 2021 Piney Point discharge event on Tampa Bay proper. Chlorophyll a concentrations in the 3 months following the discharge period were significantly higher than the same period in the following year at all three Bay sites proximal to the source of the discharge (i.e., Bishop Harbor, Joe Bay, and Piney Point). At the reference site, St. Joseph Sound, outside of Tampa Bay proper, there was no major elevation of chlorophyll a concentrations during the 3 months directly after the discharge period, followed by moderately elevated concentrations in July and August of 2021, coincident with red tide blooms that extended along the Southwest Florida coast during this time. During the 3 months following the discharge, peak chlorophyll a values at our Tampa Bay sites fell within a range associated with meso-eutrophic coastal regions, i.e., > 10–20 μg L−1 (Hagy III et al., 2022). The latter chlorophyll levels (~20 μg L−1) are common in the northeast region of upper Tampa Bay, which is subject to autochthonous HAB events, including blooms of the toxic dinoflagellate Pyrodinium bahamense, but are rare in the outer regions of the bay (Badylak et al., 2007). As such, the chlorophyll a values seen in the three months following the discharge exceeded the long-term median and range of chlorophyll a values in lower Tampa Bay from 2006 to 2020, which was 3.1 μg L−1 and 2.3–3.5 μg L−1 (Beck et al., 2022), and exceeded the annual average lower bay management target of 4.6 μg L−1 (Tampa Bay Estuary Program, 2022). High chlorophyll a levels are encountered along the southwest coast of Florida during red tides of the toxic dinoflagellate Karenia brevis (Heil et al., 2014; Milbrandt et al., 2021; Phlips et al., 2023), which periodically intrude into Tampa Bay, as observed in this study (Beck et al., 2022).
Peaks in phytoplankton biomass observed at the lower bay sampling sites in the 2 months following the Piney Point discharge event were dominated by euryhaline diatom species (Eppley, 1977; Brand, 1984; Balzano et al., 2011; Karthik et al., 2017), most prominently Leptocylindrus minimus, Leptocylindrus danicus, and Cerataulina pelagica. The three species are commonly found in estuaries around the world (Reynolds, 2006), and in Florida (Badylak and Phlips, 2004; Quinlan and Phlips, 2007; Hart et al., 2015), including Tampa Bay (Badylak et al., 2007). The euryhaline characteristic of these species makes them competitive in estuaries like Tampa Bay, in which salinities can range from mesohaline (i.e., 5–18 psu) to euhaline (i.e., >30 psu), as observed in the three lower bay sites in this study. The three diatom species are also known to have high maximum growth rates, i.e., >1.5 dbl. day−1 (Stolte and Garcés, 2006; Ajani et al., 2016). The high growth rates allow such species to take advantage of pulses of nutrients (Litchman et al., 2007; Cermeño et al., 2011; Karthik et al., 2017; Anderson et al., 2022), such as those observed with the Piney Point discharge, compared to many bloom-forming dinoflagellates encountered in coastal ecosystems in Florida (Phlips et al., 2006, 2011) which have maximum growth rates less than 1 dbl. day−1 (Stolte and Garcés, 2006; Matsubara et al., 2007). This is particularly true during cooler months of the year in Florida when water temperatures fall within 20–25°C, which is well within the optimal temperature range for growth of these diatom species, as opposed to other regionally important bloom-forming cyanobacteria and dinoflagellate species which have growth temperature optima between 25 and 35°C (Phlips and Mitsui, 1982; Montagnes and Franklin, 2001; Phlips et al., 2006; Anderson and Rynearson, 2020). Another factor that can enhance the success of diatom taxa with spines, a characteristic of the three aforementioned species, is lower grazing loss rates (Irigoien et al., 2005). While most diatoms are not viewed as serious HAB species, with the noted exception of toxin-producing species of Pseudo-nitzschia (Lassus et al., 2015), major blooms of Leptocylindrus and Cerataulina have been implicated in mortalities, or other health issues, in fish and shellfish populations, in relationship to physical damage, production of congestive muscilage, or generation of hypoxic conditions (Taylor et al., 1985; Buschmann et al., 2006; Ianora et al., 2008; Martin and LeGresley, 2014). These observations highlight the importance of including these types of taxa in monitoring and management plans for impacted ecosystems such as Tampa Bay.
Another noteworthy aspect of the post Piney Point discharge period were peak biomass observations of the toxic red tide dinoflagellate, K. brevis at the Piney Point site in June 2021. Karenia brevis was also observed at Bishop Harbor and St. Joseph Sound during the same general time period, at lower biomass levels. This corroborates with Beck et al. (2022), who reported high concentrations of K. brevis in lower and middle Tampa Bay for the weeks of June 13th to July 18th, 2021. During the K. brevis bloom, there was an increase in extracted and in situ chlorophyll, as well as in situ measurements of PE. The peak biomass value for K. brevis observed at Piney Point was 2.71 mg C L−1 (i.e., 3.85 million cells L−1), which ranks it as a major bloom of concern to the health of the estuary - including the potential for animal mortalities, development of anoxia, and human health issues (Kirkpatrick et al., 2004; Fleming et al., 2005; Landsberg et al., 2009; Heil and Muni-Morgan, 2021). During this period, extensive fish kills were also reported in Middle and Lower Tampa Bay (Beck et al., 2022; Florida Fish and Wildlife Conservation Commission, 2022).
K. brevis is a HAB species whose blooms initiate on the west Florida Shelf from upwelling events and are then advected to the nearshore through wind and currents, where they negatively affect coastal communities, particularly on the southwest coast of Florida between Tampa Bay and the Caloosahatchee Basin (Heil et al., 2014; Weisberg et al., 2019). The source of K. brevis at our study sites was likely the Gulf of Mexico, brought inshore by winds and current, and was facilitated by high salinities and available nutrients in the bay at that time, since K. brevis is known to be somewhat intolerant of low salinities (i.e., <20 psu; Steidinger, 2009; Beck et al., 2022). K. brevis blooms are a common feature along the west coast of Florida (Steidinger, 2009; Vargo, 2009; Heil et al., 2014), and typically originate offshore on the coastal shelf, and then are introduced to coastal estuaries through prevailing nearshore circulation patterns (Weisberg et al., 2019). High nutrient discharge from the Piney Point reservoir was suspected to contribute to the high biomass of K. brevis observed in the Piney Point region in June and July. Once K. brevis reaches the nearshore, terrestrial nutrient sources may influence bloom conditions, and several recent studies have observed positive relationships between elevated nutrient concentrations in discharges from local coastal watersheds and the intensity of red tide events (Medina et al., 2020, 2022; Phlips et al., 2023). However, further research is needed to define whether the high K. brevis biomass was associated with autochthonous production in the region, or allochthonous introduction of K. brevis from Tampa Bay, although evidence suggests both occurred during this event.
Stable isotopes of N have been utilized in Tampa Bay and other coastal ecosystems to evaluate contributions from N sources and investigate N cycling, and our investigation of particulate δ13C and δ15N values revealed that Piney Point discharge had a unique SPM source signature. The discharge water SPM had an exceptionally low δ15N value of −17.88‰ ± 0.76 (n = 5), and a δ13C value of −15.23‰ ± 0.53 (n = 5). A δ15N value of −17.88‰ is unusually low and is considerably lower than values reported for inorganic fertilizers, which can be as low as ~ −10‰ (Bateman and Kelly, 2007). In Tampa Bay, particulate and dissolved N stable isotopes have been used to identify N sources in stormwater runoff (Jani et al., 2020), constrain nutrient sources for K. brevis blooms (Havens, 2004), and asses N sources within the Gulf of Mexico (Knapp et al., 2021), and our unusually low value makes the δ15N value of Piney Point SPM unique, and much more depleted, relative to other nutrient sources in the region. Local rainfall nitrate (NO3−) values range from −4.42 to 5.69‰, stormwater runoff δ15N- NO3− values range from −9.72 to 8.06‰, particulate organic N values range from −1.99 to 6.27‰, and local vegetation sources range from −1.70 to −0.83 ‰ (Quercus virginia leaves), 1.55 to 1.60‰ (Quercus virginia acorns), and −1.93 to 0.68‰ (Stenotaphrum secundatum grass clippings; Jani et al., 2020). Additionally, the Piney Point SPM δ15N values are much lower than those reported for particulate N in the Gulf of Mexico (1.6–5.0‰; Knapp et al., 2021). The only available published study that reported exceptionally low δ15N values comparable to ours was a study of seagrass meadows of Halodule uninervis in Qatar, which documented δ15N values as low as ~ −12.4‰, which was attributed to undefined but localized sediment processes (Walton et al., 2016).
This unusual δ15N value is likely due to the unique characteristics of the reservoir and the water discharged from it. The discharge water was not pure diammonium phosphate, but rather a combination of waste derived from the production of diammonium phosphate, seawater from dredging operations, and rainwater, which have created unique conditions within the reservoir since it was partially filled in 2011 (Beck et al., 2022). Thus, the SPM collected from the reservoir contained particulate material that included phytoplankton and other microorganisms that were growing in the reservoir water, and as such, should not be expected to have δ15N values that exactly resemble ammonium (NH4+) fertilizer. Given the novel growth conditions presented at the reservoir, it is likely that the highly depleted values seen in the discharge water in April 2021 (−17.88‰ ± 0.76), and in the reservoir water in October 2021 (−19.15‰ ± 0.05), were driven by isotope fractionation associated with ammonium transport and assimilation within the reservoir. Differences between the April and October δ15N and nutrient values are likely from on-site treatment technologies used since April 2021 to reduce total P and total N within the reservoir.
Stable isotope values of δ15N can be influenced by various isotopic fractionation pathways which we suggest explains the extreme δ15N values we observed. Many phytoplankton and other microorganisms preferentially utilize NH4+ rather than NO3−. When ammonium is transported and assimilated by phytoplankton or other microorganisms, isotopic fractionation can occur when they preferentially utilize the light (14N) isotope and leave the heavy 15N isotope behind. This process can be concentration dependent, and when the ammonium concentration is higher, organisms discriminate more against the heavy isotope (15N) and take up more of the lighter (14N) isotope. This transport and assimilation of 14N into their biomass decreases the relative proportion of 15N in their biomass, and subsequently results in lower δ15N values of the SPM (δ15NSPM). We hypothesize that, under the extremely high (~210 mg L−1) ammonium concentrations in the Piney Point reservoir, this isotope fractionation occurred to such an extent that it resulted in extremely low δ15N values observed in the SPM of the reservoir.
While no laboratory studies to date have explored isotopic fractionation associated with ammonium concentrations as high as those in the study reservoir, Liu et al. (2015) reported the occurrence of concentration dependent isotopic fractionation when Chlorella vulgaris F1068 was grown in media with 4, 10 and 50 mg L−1 ammonium. C. vulgaris F1068 is a member of the division Chlorophyta, which was also the division of the dominant alga identified in the Piney Point reservoir. While the maximum C. vulgaris F1068 growth rate was seen at 4 mg L−1, this species is capable of tolerating high (50 mg L−1) concentrations of ammonium (Liu et al., 2015). While Liu et al. (2015) did not quantify the isotope enrichment factor (ɛ; a measure of isotopic discrimination against heavier isotopes) at 50 mg L−1, they found an ɛ of −2.37‰ when C. vulgaris was grown at 10 mg L−1 ammonium, and that there was greater discrimination against the heavy isotope when C. vulgaris was grown under higher ammonium concentrations (Liu et al., 2015). Concentration dependent isotopic fractionation is not solely limited to members of Chlorophyta, as another laboratory study found that ɛ values of the diatom Skeletonema significantly decreased from −7.8‰ to −27.2‰ when grown on increasing concentrations of ammonium, indicating that when ammonium concentrations are higher, 14N is incorporated faster than 15N, thereby depleting (lowering) the δ15N value of the phytoplankton and SPM (Pennock et al., 1996).
Anomalously low SPM δ15N values seen at our study sites in May and June of 2021 suggest that there was incorporation of highly depleted Piney Point discharge into particulate material in the region. Previous work in Tampa Bay has suggested that K. brevis utilizes sources with low δ15N values (Havens, 2004), however, the low SPM δ15N values occurred prior to the dates of the peak K. brevis bloom, suggesting that the NH4+ from the discharge water was either (1) taken up by fast growing diatoms in May/June and incorporated into phytoplankton biomass/SPM; or (2) NH4+ may have been utilized during the early stage of the bloom, as initially low K. brevis cell counts were seen in lower Tampa Bay as early as the week of April 18th, 2021. However, we do not have sufficient evidence to explicitly evaluate these two scenarios.
The discharge water had a δ13C value of −15.23‰ ± 0.53, which is within the range of bacteria or C4 terrestrial organic matter (Lamb et al., 2006). All sites exhibited a increase in δ13C values concomitant with the decrease in δ15N values, suggesting that C derived from the Piney Point discharge was also incorporated into SPM at the same time that δ15N and C:N values declined. Interestingly, while phytoplankton biomass was lower overall at the reference site, St. Joseph Sound, the same trends in SPM stable isotope values were seen in the reference site. This may be an indication of the export of discharge C and N into regions outside of the bay, which is supported by initial findings from Liu et al. (2021) that showed the discharge was gradually flushed out of the bay and into adjacent coastal waters (Liu et al., 2021). The persistence of highly depleted SPM δ15N values approximately 50 km from the discharge site is unexpected, but other regions distant to the discharge site, such as upper Sarasota Bay were also likely affected by the discharged waters (Tomasko, 2023). Additionally, we found that the reference site had elevated orthoP values in April 2021 relative to April 2022, suggesting that orthoP from the discharge was also transported to the reference site.
It must be emphasized that while the stable isotope values reported here indicate a transport of discharge outside of the bay, we did not observe large, associated shifts in chlorophyll values and phytoplankton community structure at the reference site, suggesting that, even if the SPM at the reference site reflects N contributions from the Piney Point discharge, these values may not have had adverse ecological effects at this location on the phytoplankton community. However, it should be noted that the released nutrients may have had other fates such as uptake into macroalgae and seagrasses, as well as deposition into bay sediments, both of which may have important implications for the long-term health of the Bay. For example, macroalgae blooms have contributed to seagrass losses throughout the Bay, which have been ongoing since before Piney Point. After Piney Point, Ulva was abundant in Hillsborough Bay and Dapis was abundant in most of the Bay (Tomasko, 2023), which may adversely affect seagrass meadows. Deposition into Bay sediments may also be an important mechanism by which nutrients from the discharge may have been removed from the water column and stored in the sediments. This legacy nutrient source may have implications for future water quality, as sedimentary resuspension, and fluxes of inorganic nutrients from sediments can provide an important source of nutrients to primary producers, including phytoplankton and HABs (Dixon et al., 2014). Further investigation into macroalgae and seagrass stable isotope values and sedimentary records from the study area will provide a more complete picture of nutrient effects on the ecosystem.
In addition to capturing some of the effects of the Piney Point discharge on Tampa Bay’s phytoplankton communities and particulate material, we also detected a signal of Tropical Storm Elsa on the water quality of different regions within the Bay. The passage of tropical storm Elsa through the Tampa Bay area (July 5th, 2021) resulted in approximately 8–18 cm of rain delivered to the west coast of Florida (Cangialosi et al., 2022), and increased stream flows of the Anclote River and Little Manatee River which are tributaries to our study area (USGS, 2021a,b). The maximum stream flow reported for the Anclote river (USGS monitoring location 02310000), near the reference site St. Joseph Sound, was 954 ft3 s−1 on July 10th, 2021, compared to 5.05 ft3 s−1 during pre-storm conditions on July 1st, 2021 (USGS, 2021a), and the maximum stream flow reported for the Little Manatee River (USGS monitoring location 02300500), closest to the lower Tampa Bay sites, was 1,510 ft3 s−1 on July 8th compared to 43.1 ft3 s−1 during pre-storm conditions on June 28th, indicating that there were considerable increases in stream flow rates associated with this storm event.
The increased rainfall and streamflow into the study sites was associated with lower salinities, particularly at the lower bay sites. A decrease in fDOM, extracted and in situ chlorophyll, as well as PE were seen at the lower Tampa Bay sites, likely due to dilution of terrestrial DOM, in the case of fDOM, and possibly due to flushing of phytoplankton from the bay, in the case of chlorophyll and PE. The effects of Tropical Storm Elsa also likely influenced particulate TC values, as the lowest TC values (0.2 ± 0.02%) at Bishop Harbor and St. Joseph Sound site were seen immediately following the storm and likely driven by a dilution effect from freshwater inflows. We also observed a decline in C:N values at these sites, likely driven by an influx of N derived from the surrounding watershed associated with the storm. Past work in the region found that after some storm events, the concentration of all forms of N decreased, which was attributed to a dilution effect followed by a slight increase in TN concentrations after rainfall ceased (Jani et al., 2020). This was seen in 3 out of 4 sites in this study following the passage of Tropical Storm Elsa, where particulate N values exhibited an initial decrease after the storm, followed by a slight increase at Bishop Harbor and St. Joseph Sound sites, highlighting the important role that tropical storms play in influencing nutrient fluxes from terrestrial systems.
In addition, the occurrence of tropical storms and hurricanes can have interactive effects with built infrastructure, potentially resulting in increased threats to coastal systems and changes in nutrient regimes. For example, the capacity of the holding reservoirs at Piney Point had decreased due to rain events and tropical storms in the years prior to the event (Beck et al., 2022) increasing the risk of overflow or breaching which likely contributed to the 2021 event. Closure activities at the site are now centered on reducing the accumulation of rainfall within the wastewater reservoir system. As tropical storms and hurricanes are projected to increase in frequency and intensity, events such as the Piney Point 2021 wastewater discharge emphasize the vulnerability of coastal infrastructure and the potential ongoing threats these vulnerable facilities pose to coastal ecosystems.
Here we document changes in phytoplankton community composition, water quality, and SPM in the year following the 2021 Piney Point discharge event. Soon after the discharge, elevated diatom biomass was seen in the lower Tampa Bay sites, followed by high biomass of the harmful algae K. brevis in the summer of 2021. Nitrogen stable isotope values in SPM were very low in May and June of 2021 and were similar to δ15N values from the discharge, suggesting that N from the Piney Point discharge was incorporated into SPM in the region. Low δ15N values were also seen in the SPM samples collected from the reference site, St. Joseph Sound, and higher orthophosphate concentrations were seen at the site in April 2021 versus 2022, further suggesting that some of the Piney Point discharge was exported out of Tampa Bay proper. While the stable isotope values reported here indicate a transport of discharge outside of the bay, chlorophyll values and phytoplankton communities did not exhibit notable shifts at the reference site, suggesting that, even though SPM at the reference site reflects N contributions from the Piney Point discharge, these values may not have had adverse ecological effects at this location, at least from a phytoplankton perspective. While onsite treatment technologies have reduced the N and P concentrations in the reservoir, and have reduced the risk to nearby coastal ecosystems, the Piney Point event highlights the threat that industrial infrastructure failures can cause along Florida’s coastlines. In addition, these vulnerabilities should be assessed with other factors, such as estuarine flushing rates, water residence times, climatic factors, and storms and hurricanes, which can also influence the initiation and persistence of HABs and the health of Florida’s coastal ecosystems (Phlips et al., 2020; Tomasko et al., 2020). Here, the effects of Tropical Storm Elsa showed changes in water quality in the region, and storms such as Hurricane Ian, which passed through the region in September 2022, may also have interactive effects with the discharge-impacted regions in the Bay. This work underscores the need for comprehensive nutrient management strategies and convergent research to assess and manage the full range of consequences associated with anthropogenic nutrient inputs into coastal ecosystems. Ongoing and anticipated impacts of accelerated climate change – such as increasing tropical storm intensity, temperatures, rainfall, and sea level rise – will amplify this need.
The datasets presented in this study can be found in online repositories. The names of the repository/repositories and accession number(s) can be found at: https://github.com/elisemorrison/PineyPoint2021
EM: conceptualization, data collection, field sampling, data curation, formal analysis, methodology, writing – original draft, and writing – review and editing. EP: conceptualization, data curation, formal analysis, methodology, writing – original draft, and writing – review and editing. SB: data curation, formal analysis, methodology, and writing – review and editing. AC and AA: data collection, field sampling, data curation, methodology, and writing – review and editing. TO: data curation and writing – review and editing. DT: data curation and writing – review and editing. MB and ES: writing – review and editing. All authors contributed to the article and approved the submitted version.
This research was supported by the National Science Foundation (RAPID Award Number 2130675 to EM and EP and Award Number 2019435), and the Ocean Conservancy award to EM, EP, AA and Christine Angelini.
The authors would like to thank: Todd Van Natta, Patrick Norby, Charli Pezoldt, and Adam Hymel for their assistance with sampling, sonde maintenance, and data management; Jason Curtis at the UF Stable Isotope Laboratory; Jean Lockwood for assistance with phytoplankton analyses and Leslie Landauer for assistance with chlorophyll analyses; and Megan Sanford for laboratory support and sample preparation.
The authors declare that the research was conducted in the absence of any commercial or financial relationships that could be construed as a potential conflict of interest.
All claims expressed in this article are solely those of the authors and do not necessarily represent those of their affiliated organizations, or those of the publisher, the editors and the reviewers. Any product that may be evaluated in this article, or claim that may be made by its manufacturer, is not guaranteed or endorsed by the publisher.
The Supplementary material for this article can be found online at: https://www.frontiersin.org/articles/10.3389/fevo.2023.1144778/full#supplementary-material
Ahlgren, G. (1983). Comparison of methods for estimation of phytoplankton carbon. Arch. Hydrobiol. 98, 489–508.
Ajani, P. A., Armbrecht, L. H., Kersten, O., Kohli, G. S., and Murray, S. A. (2016). Diversity, temporal distribution and physiology of the centric diatom Leptocylindrus Cleve (Bacillariophyta) from a southern hemisphere upwelling system. Diatom Res. 31, 351–365. doi: 10.1080/0269249X.2016.1260058
American Public Health Association (2005). Standard methods for the examination of water and wastewater. Washington, DC, USA: American Public Health Association (APHA).
Anderson, S. I., Franzè, G., Kling, J. D., Wilburn, P., Kremer, C. T., Menden-Deuer, S., et al. (2022). The interactive effects of temperature and nutrients on a spring phytoplankton community. Limnol. Oceanogr. 67, 634–645. doi: 10.1002/lno.12023
Anderson, S. I., and Rynearson, T. A. (2020). Variability approaching the thermal limits can drive diatom community dynamics. Limnol. Oceanogr. 65, 1961–1973. doi: 10.1002/lno.11430
Badylak, S., and Phlips, E. (2004). Spatial and temporal patterns of phytoplankton composition in subtropical coastal lagoon, the Indian River lagoon, Florida, USA. J. Plankton Res. 26, 1229–1247. doi: 10.1093/plankt/fbh114
Badylak, S., Phlips, E. J., Baker, P., Fajans, J., and Boler, R. (2007). Distributions of phytoplankton in Tampa Bay estuary, USA 2002-2003. Bull. Mar. Sci. 80, 295–317.
Badylak, S., Phlips, E. J., and Mathews, A. L. (2014). Akashiwo sanguinea (Dinophyceae) blooms in a sub-tropical estuary: an alga for all seasons. Plank. Benthos Res. 9, 147–155. doi: 10.3800/pbr.9.147
Balzano, S., Sarno, D., and Kooistra, W. H. (2011). Effects of salinity on the growth rate and morphology of ten Skeletonema strains. J. Plankton Res. 33, 937–945. doi: 10.1093/plankt/fbq150
Bateman, A. S., and Kelly, S. D. (2007). Fertilizer nitrogen isotope signatures. Isot. Environ. Health Stud. 43, 237–247. doi: 10.1080/10256010701550732
Beck, M. W., Altieri, A., Angelini, C., Burke, M. C., Chen, J., Chin, D. W., et al. (2022). Initial estuarine response to inorganic nutrient inputs from a legacy mining facility adjacent to Tampa Bay Florida. Mar. Pollut. Bull. 178:113598. doi: 10.1016/j.marpolbul.2022.113598
Beck, M. W., Sherwood, E. T., Henkel, J. R., Dorans, K., Ireland, K., and Varela, P. (2019). Assessment of the cumulative effects of restoration activities on water quality in Tampa Bay, Florida. Estuar. Coasts 42, 1774–1791. doi: 10.1007/s12237-019-00619-w
Beusen, A., Van Beek, L., Bouwman, A., Mogollón, J., and Middelburg, J. (2015). Coupling global models for hydrology and nutrient loading to simulate nitrogen and phosphorus retention in surface water–description of IMAGE–GNM and analysis of performance. Geosci. Model Dev. 8, 4045–4067. doi: 10.5194/gmd-8-4045-2015
Brand, L. E. (1984). The salinity tolerance of forty-six marine phytoplankton isolates. Estuar. Coast. Shelf Sci. 18, 543–556. doi: 10.1016/0272-7714(84)90089-1
Buschmann, A. H., Riquelme, V. A., Hernández-González, M. C., Varela, D., Jiménez, J. E., Henríquez, L. A., et al. (2006). A review of the impacts of salmonid farming on marine coastal ecosystems in the Southeast Pacific. ICES J. Mar. Sci. 63, 1338–1345. doi: 10.1016/j.icesjms.2006.04.021
Cangialosi, J. P., Delgado, S., and Berg, R. (2022). National Hurricane Center Tropical Cyclone Report Hurricane Elsa (AL052021) Miami FL: ELSA.
Cermeño, P., Lee, J.-B., Wyman, K., Schofield, O., and Falkowski, P. G. (2011). Competitive dynamics in two species of marine phytoplankton under non-equilibrium conditions. Mar. Ecol. Prog. Ser. 429, 19–28. doi: 10.3354/meps09088
Chen, J., Weisberg, R. H., Liu, Y., and Zheng, L. (2018). The Tampa Bay coastal ocean model performance for hurricane Irma. Mar. Technol. Soc. J. 52, 33–42. doi: 10.4031/MTSJ.52.3.6
Chen, J., Weisberg, R. H., Liu, Y., Zheng, L., and Zhu, J. (2019). On the momentum balance of Tampa Bay. J. Geophys. Res. Oceans 124, 4492–4510. doi: 10.1029/2018JC014890
Cloern, J. E. (2001). Our evolving conceptual model of the coastal eutrophication problem. Mar. Ecol. Prog. Ser. 210, 223–253. doi: 10.3354/meps210223
Deangelis, B. M., Sutton-Grier, A. E., Colden, A., Arkema, K. K., Baillie, C. J., Bennett, R. O., et al. (2020). Social factors key to landscape-scale coastal restoration: lessons learned from three US case studies. Sustainability 12:869. doi: 10.3390/su12030869
Dixon, L. K., Murphy, P. J., Becker, N. M., and Charniga, C. M. (2014). The potential role of benthic nutrient flux in support of Karenia blooms in West Florida (USA) estuaries and the nearshore Gulf of Mexico. Harmful Algae 38, 30–39. doi: 10.1016/j.hal.2014.04.005
Doney, S. C., Ruckelshaus, M., Emmett Duffy, J., Barry, J. P., Chan, F., English, C. A., et al. (2012). Climate change impacts on marine ecosystems. Annu. Rev. Mar. Sci. 4, 11–37. doi: 10.1146/annurev-marine-041911-111611
Fahnenstiel, G. L., and Carrick, H. J. (1992). Phototrophic picoplankton in lakes Huron and Michigan: abundance, distribution, composition, and contribution to biomass and production. Can. J. Fish. Aquat. Sci. 49, 379–388. doi: 10.1139/f92-043
Fleming, L. E., Kirkpatrick, B., Backer, L. C., Bean, J. A., Wanner, A., Dalpra, D., et al. (2005). Initial evaluation of the effects of aerosolized Florida red tide toxins (brevetoxins) in persons with asthma. Environ. Health Perspect. 113, 650–657. doi: 10.1289/ehp.7500
Florida Department of Environmental Protection (2021). Piney point update - April 6, 2021 [online]. Available at: https://protectingfloridatogether.gov/node/285# (Accessed October 1, 2023).
Florida Fish and Wildlife Conservation Commission (2022). Fish kill database search result report [online]. Available at: https://app.myfwc.com/FWRI/FishKillReport/ReportWorkSheet.aspx (Accessed 14 January, 2023).
Garrett, M., Wolny, J., Truby, E., Heil, C., and Kovach, C. (2011). Harmful algal bloom species and phosphate-processing effluent: field and laboratory studies. Mar. Pollut. Bull. 62, 596–601. doi: 10.1016/j.marpolbul.2010.11.017
Glibert, P. M. (2020). Harmful algae at the complex nexus of eutrophication and climate change. Harmful Algae 91:101583. doi: 10.1016/j.hal.2019.03.001
Glibert, P. M., Icarus Allen, J., Artioli, Y., Beusen, A., Bouwman, L., Harle, J., et al. (2014). Vulnerability of coastal ecosystems to changes in harmful algal bloom distribution in response to climate change: projections based on model analysis. Glob. Chang. Biol. 20, 3845–3858. doi: 10.1111/gcb.12662
Gobler, C. J. (2020). Climate change and harmful algal blooms: insights and perspective. Harmful Algae 91:101731. doi: 10.1016/j.hal.2019.101731
Greening, H., Janicki, A., Sherwood, E. T., Pribble, R., and Johansson, J. O. R. (2014). Ecosystem responses to long-term nutrient management in an urban estuary: Tampa Bay, Florida, USA. Estuar. Coast. Shelf Sci. 151, A1–A16. doi: 10.1016/j.ecss.2014.10.003
Griffith, A. W., and Gobler, C. J. (2020). Harmful algal blooms: a climate change co-stressor in marine and freshwater ecosystems. Harmful Algae 91:101590. doi: 10.1016/j.hal.2019.03.008
Grill, G., Lehner, B., Lumsdon, A. E., Macdonald, G. K., Zarfl, C., and Liermann, C. R. (2015). An index-based framework for assessing patterns and trends in river fragmentation and flow regulation by global dams at multiple scales. Environ. Res. Lett. 10:015001. doi: 10.1088/1748-9326/10/1/015001
Hagy Iii, J. D., Kreakie, B. J., Pelletier, M. C., Nojavan, F., Kiddon, J. A., and Oczkowski, A. J. (2022). Quantifying coastal ecosystem trophic state at a macroscale using a Bayesian analytical framework. Ecol. Indic. 142:109267, –109212. doi: 10.1016/j.ecolind.2022.109267
Hart, J., Phlips, E., Badylak, S., Dix, N., Petrinec, K., Mathews, A., et al. (2015). Phytoplankton biomass and composition in a well-flushed, sub-tropical estuary: the contrasting effects of hydrology, nutrient loads and allochthonous influences. Mar. Environ. Res. 112, 9–20. doi: 10.1016/j.marenvres.2015.08.010
Havens, J. A. (2004). A stable isotopic examination of particulate organic matter during Karenia brevis blooms on the central West Florida shelf: Hints at nitrogen sources in oligotrophic waters.
Heil, C. A., Dixon, L. K., Hall, E., Garrett, M., Lenes, J. M., Oneil, J. M., et al. (2014). Blooms of Karenia brevis (Davis) G. Hansen & Ø. Moestrup on the West Florida shelf: nutrient sources and potential management strategies based on a multi-year regional study. Harmful Algae 38, 127–140. doi: 10.1016/j.hal.2014.07.016
Heil, C. A., and Muni-Morgan, A. L. (2021). Florida’s harmful algal bloom (HAB) problem: escalating risks to human, environmental and economic health with climate change. Front. Ecol. Evol. 9:646080. doi: 10.3389/fevo.2021.646080
Heisler, J., Glibert, P. M., Burkholder, J. M., Anderson, D. M., Cochlan, W., Dennison, W. C., et al. (2008). Eutrophication and harmful algal blooms: a scientific consensus. Harmful Algae 8, 3–13. doi: 10.1016/j.hal.2008.08.006
Herren, L., Brewton, R., Wilking, L., Tarnowski, M., Vogel, M., and Lapointe, B. (2021). Septic systems drive nutrient enrichment of groundwaters and eutrophication in the urbanized Indian River lagoon. Florida. Mar. Pollut. Bull. 172:112928. doi: 10.1016/j.marpolbul.2021.112928
Hoegh-Guldberg, O., and Bruno, J. F. (2010). The impact of climate change on the world’s marine ecosystems. Science 328, 1523–1528. doi: 10.1126/science.1189930
Ianora, A., Casotti, R., Bastianini, M., Brunet, C., D’ippolito, G., Acri, F., et al. (2008). Low reproductive success for copepods during a bloom of the non-aldehyde-producing diatom Cerataulina pelagica in the North Adriatic Sea. Mar. Ecol. 29, 399–410. doi: 10.1111/j.1439-0485.2008.00226.x
Irigoien, X., Flynn, K., and Harris, R. (2005). Phytoplankton blooms: a “loophole” in microzooplankton grazing impact? J. Plankton Res. 27, 313–321. doi: 10.1093/plankt/fbi011
Jani, J., Yang, Y.-Y., Lusk, M. G., and Toor, G. S. (2020). Composition of nitrogen in urban residential stormwater runoff: concentrations, loads, and source characterization of nitrate and organic nitrogen. PLoS One 15:e0229715. doi: 10.1371/journal.pone.0229715
Karthik, R., Padmavati, G., Elangovan, S. S., and Sachithanandam, V. (2017). Monitoring the diatom bloom of Leptocylindrus danicus (Cleve 1889, Bacillariophyceae) in the coastal waters of south Andaman Island. Indian J. Geo-Mar. Sci. 46, 958–965. http://nopr.niscpr.res.in/handle/123456789/41662
Kirkpatrick, B., Fleming, L. E., Squicciarini, D., Backer, L. C., Clark, R., Abraham, W., et al. (2004). Literature review of Florida red tide: implications for human health effects. Harmful Algae 3, 99–115. doi: 10.1016/j.hal.2003.08.005
Knapp, A. N., Thomas, R. K., Stukel, M. R., Kelly, T. B., Landry, M. R., Selph, K. E., et al. (2021). Constraining the sources of nitrogen fueling export production in the Gulf of Mexico using nitrogen isotope budgets. J. Plankton Res. 44, 692–710.
Lamb, A. L., Wilson, G. P., and Leng, M. J. (2006). A review of coastal palaeoclimate and relative sea-level reconstructions using δ13C and C/N ratios in organic material. Earth Sci. Rev. 75, 29–57. doi: 10.1016/j.earscirev.2005.10.003
Landsberg, J., Flewelling, L., and Naar, J. (2009). Karenia brevis red tides, brevetoxins in the food web, and impacts on natural resources: decadal advancements. Harmful Algae 8, 598–607. doi: 10.1016/j.hal.2008.11.010
Lassus, P., Chaumérat, N., Hess, P., and Nézan, E. (2015). Toxic and harmful microalgae of the World Ocean, International Society for the Study of harmful algae and the United Nations ….
Lehner, B., Liermann, C. R., Revenga, C., Vörösmarty, C., Fekete, B., Crouzet, P., et al. (2011). High-resolution mapping of the world's reservoirs and dams for sustainable river-flow management. Front. Ecol. Environ. 9, 494–502. doi: 10.1890/100125
Litchman, E., Klausmeier, C. A., Schofield, O. M., and Falkowski, P. G. (2007). The role of functional traits and trade-offs in structuring phytoplankton communities: scaling from cellular to ecosystem level. Ecol. Lett. 10, 1170–1181. doi: 10.1111/j.1461-0248.2007.01117.x
Liu, N., Li, F., Ge, F., Tao, N., Zhou, Q., and Wong, M. (2015). Mechanisms of ammonium assimilation by Chlorella vulgaris F1068: isotope fractionation and proteomic approaches. Bioresour. Technol. 190, 307–314. doi: 10.1016/j.biortech.2015.04.024
Liu, Y., Weisberg, R., Zheng, L., Sun, Y., and Chen, J. Nowcast/forecast of the Tampa Bay, piney point effluent plume: a rapid response AGU fall Meeting Abstracts (2021). OS35B–1036.
Lundholm, N., Churro, C., Fraga, S., Hoppenrath, M., Iwataki, M., Larsen, J., et al. (Eds) (2009 onwards). IOC-UNESCO Taxonomic Reference List of Harmful Micro Algae. Accessed at https://www.marinespecies.org/hab on yyyy-mm-dd. doi: 10.14284/362
Maavara, T., Parsons, C. T., Ridenour, C., Stojanovic, S., Dürr, H. H., Powley, H. R., et al. (2015). Global phosphorus retention by river damming. Proc. Natl. Acad. Sci. U. S. A. 112, 15603–15608. doi: 10.1073/pnas.1511797112
Martin, J. L., and Legresley, M. (2014). Phytoplankton monitoring in the Western isles region of the bay of Fundy during 2003–2006, Biological Station: Fisheries and Oceans Canada.
Matsubara, T., Nagasoe, S., Yamasaki, Y., Shikata, T., Shimasaki, Y., Oshima, Y., et al. (2007). Effects of temperature, salinity, and irradiance on the growth of the dinoflagellate Akashiwo sanguinea. J. Exp. Mar. Biol. Ecol. 342, 226–230. doi: 10.1016/j.jembe.2006.09.013
Medina, M., Huffaker, R., Jawitz, J. W., and Muñoz-Carpena, R. (2020). Seasonal dynamics of terrestrially sourced nitrogen influenced Karenia brevis blooms off Florida's southern Gulf Coast. Harmful Algae 98:101900. doi: 10.1016/j.hal.2020.101900
Medina, M., Kaplan, D., Milbrandt, E. C., Tomasko, D., Huffaker, R., and Angelini, C. (2022). Nitrogen-enriched discharges from a highly managed watershed intensify red tide (Karenia brevis) blooms in Southwest Florida. Sci. Total Environ. 827:154149. doi: 10.1016/j.scitotenv.2022.154149
Metcalf, J., Banack, S., Wessel, R., Lester, M., Pim, J., Cassani, J., et al. (2021). Toxin analysis of freshwater cyanobacterial and marine harmful algal blooms on the west coast of Florida and implications for estuarine environments. Neurotox. Res. 39, 27–35. doi: 10.1007/s12640-020-00248-3
Milbrandt, E. C., Martignette, A., Thompson, M., Bartleson, R., Phlips, E., Badylak, S., et al. (2021). Geospatial distribution of hypoxia associated with a Karenia brevis bloom. Estuar. Coast. Shelf Sci. 259:107446. doi: 10.1016/j.ecss.2021.107446
Montagnes, D. J., and Franklin, M. (2001). Effect of temperature on diatom volume, growth rate, and carbon and nitrogen content: reconsidering some paradigms. Limnol. Oceanogr. 46, 2008–2018. doi: 10.4319/lo.2001.46.8.2008
Nelson, N. G., Cuchiara, M. L., Hendren, C. O., Jones, J. L., and Marshall, A.-M. (2021). Hazardous spills at retired fertilizer manufacturing plants will continue to occur in the absence of scientific innovation and regulatory enforcement. Environ. Sci. Technol. 55, 16267–16269. doi: 10.1021/acs.est.1c05311
Nixon, S. W. (1995). Coastal marine eutrophication: a definition, social causes, and future concerns. Ophelia 41, 199–219. doi: 10.1080/00785236.1995.10422044
Oneil, J. M., Davis, T. W., Burford, M. A., and Gobler, C. J. (2012). The rise of harmful cyanobacteria blooms: the potential roles of eutrophication and climate change. Harmful Algae 14, 313–334. doi: 10.1016/j.hal.2011.10.027
Paerl, H. W., Valdes, L. M., Peierls, B. L., Adolf, J. E., and Harding, L. J. W. (2006). Anthropogenic and climatic influences on the eutrophication of large estuarine ecosystems. Limnol. Oceanogr. 51, 448–462. doi: 10.4319/lo.2006.51.1_part_2.0448
Pennock, J. R., Velinsky, D. J., Ludlam, J. M., Sharp, J. H., and Fogel, M. L. (1996). Isotopic fractionation of ammonium and nitrate during uptake by Skeletonema costatum: implications for δ15N dynamics under bloom conditions. Limnol. Oceanogr. 41, 451–459. doi: 10.4319/lo.1996.41.3.0451
Phlips, E., Badylak, S., Bledsoe, E., and Cichra, M. (2006). Factors influencing the distribution and abundance of Pyrodinium bahamense in coastal ecosystems of Florida. Mar. Ecol. Prog. Ser. 322, 99–115. doi: 10.3354/meps322099
Phlips, E. J., Badylak, S., Christman, M. C., and Lasi, M. A. (2010). Climatic trends and temporal patterns of phytoplankton composition, abundance, and succession in the Indian River lagoon, Florida, USA. Estuar. Coasts 33, 498–512. doi: 10.1007/s12237-009-9166-8
Phlips, E. J., Badylak, S., Christman, M., Wolny, J., Brame, J., Garland, J., et al. (2011). Scales of temporal and spatial variability in the distribution of harmful algae species in the Indian River lagoon, Florida, USA. Harmful Algae 10, 277–290. doi: 10.1016/j.hal.2010.11.001
Phlips, E. J., Badylak, S., and Lynch, T. C. (1999). Blooms of the picoplanktonic cyanobacterium Synechococcus in Florida bay, a subtropical inner-shelf lagoon. Limnol. Oceanogr. 44, 1166–1175. doi: 10.4319/lo.1999.44.4.1166
Phlips, E., Badylak, S., Matthews, A. L., Milbrandt, E. C. R. M. L., Morrison, E., Nelson, N. G., et al. (2023). Algal blooms in a river-dominated estuary and nearshore region of Florida, USA: the influence of regulated discharges from water control structures on hydrology and nutrient conditions. Hydrobiologia. doi: 10.1007/s10750-022-05135-w.
Phlips, E. J., Badylak, S., Nelson, N. G., and Havens, K. E. (2020). Hurricanes, El Niño and harmful algal blooms in two sub-tropical Florida estuaries: direct and indirect impacts. Sci. Rep. 10, 1–12. doi: 10.1038/s41598-020-58771-4
Phlips, E., and Mitsui, A. (1982). “Temperature preference and tolerance of aquatic photosynthetic microorganisms [including blue-green algae],” in CRC handbook of biosolar resources. eds. A. Mitsui and C. Black (Boca, Florida: CRC Press), 335–361.
Quinlan, E. L., and Phlips, E. J. (2007). Phytoplankton assemblages across the marine to low-salinity transition zone in a Blackwater dominated estuary. J. Plankton Res. 29, 401–416. doi: 10.1093/plankt/fbm024
R Core Development Team (2008). R: A language and environment for statistical computing. R Foundation for Statistical Computing: Vienna, Austria.
Sartory, D., and Grobbelaar, J. (1984). Extraction of chlorophyll a from freshwater phytoplankton for spectrophotometric analysis. Hydrobiologia 114, 177–187. doi: 10.1007/BF00031869
Sherwood, E. T., Greening, H. S., Johansson, J. R., Kaufman, K., and Raulerson, G. E. (2017). Tampa Bay (Florida, USA) documenting seagrass recovery since the 1980’s and reviewing the benefits. Southeast. Geogr. 57, 294–319. doi: 10.1353/sgo.2017.0026
Sicko-Goad, L. M., Schelske, C. L., and Stoermer, E. F. (1984). Estimation of intracellular carbon and silica content of diatoms from natural assemblages using morphometric techniques 1. Limnol. Oceanogr. 29, 1170–1178. doi: 10.4319/lo.1984.29.6.1170
Sin, Y., Hyun, B., Jeong, B., and Soh, H. Y. (2013). Impacts of eutrophic freshwater inputs on water quality and phytoplankton size structure in a temperate estuary altered by a sea dike. Mar. Environ. Res. 85, 54–63. doi: 10.1016/j.marenvres.2013.01.001
Smayda, T. J. (1978). “From phytoplankters to biomass”. In Phytoplankton Manual. ed. A. Sournia (Paris: UNESCO), 273–279.
Steidinger, K. A. (2009). Historical perspective on Karenia brevis red tide research in the Gulf of Mexico. Harmful Algae 8, 549–561. doi: 10.1016/j.hal.2008.11.009
Stolte, W., and Garcés, E. (2006). “Ecological aspects of harmful algal in situ population growth rates,” in Ecology of harmful algae (London: Springer).
Strathmann, R. R. (1967). Estimating the organic carbon content of phytoplankton from cell volume or plasma volume 1. Limnol. Oceanogr. 12, 411–418. doi: 10.4319/lo.1967.12.3.0411
Sun, J., and Liu, D. (2003). Geometric models for calculating cell biovolume and surface area for phytoplankton. J. Plankton Res. 25, 1331–1346. doi: 10.1093/plankt/fbg096
Switzer, T. S., Tyler-Jedlund, A. J., Rogers, K. R., Grier, H., Mcmichael, R. H., and Fox, S. (2011). Response of estuarine nekton to the regulated discharge of treated phosphate-production process water. TR-16. Florida Fish and Wildlife Conservation Commission. Fish and Wildlife Research Institute. Technical Report. St. Petersburg, FL. http://hdl.handle.net/1834/41127
Tampa Bay Estuary Program (2022). State of the bay 2019-2021. Available at: https://tbep.org/estuary/state-of-the-bay/ (Accessed October 1, 2023).
Tampa Bay Nitrogen Management Consortium (TBNMC) (2022). Tampa Bay Reasonable Assurance Compliance Assessment Report. Available at: https://tbep-tech.github.io/tbnmc-compliance-assessment-2022/index.html
Taylor, F., Taylor, N., and Walsby, J. (1985). A bloom of the planktonic diatom, Cerataulina pelagica, off the coast of northeastern New Zealand in 1983, and its contribution to an associated mortality of fish and benthic fauna. Internationale Revue der gesamten Hydrobiologie und Hydrographie 70, 773–795. doi: 10.1002/iroh.19850700602
Tomasko, D. (2023). Ecological impacts to Sarasota Bay from Piney Point discharges - examining the evidence. Florida Scientist.
Tomasko, D., Alderson, M., Burnes, R., Hecker, J., Iadevaia, N., Leverone, J., et al. (2020). The effects of hurricane Irma on seagrass meadows in previously eutrophic estuaries in Southwest Florida (USA). Mar. Pollut. Bull. 156:111247. doi: 10.1016/j.marpolbul.2020.111247
Tomasko, D., Alderson, M., Burnes, R., Hecker, J., Leverone, J., Raulerson, G., et al. (2018). Widespread recovery of seagrass coverage in Southwest Florida (USA): temporal and spatial trends and management actions responsible for success. Mar. Pollut. Bull. 135, 1128–1137. doi: 10.1016/j.marpolbul.2018.08.049
USGS (2021a). USGS water data Anclote River [online]. Available at: https://waterdata.usgs.gov/monitoring-location/02310000/#parameterCode=00060&startDT=2021-04-01&endDT=2022-05-01 (Accessed November 1, 2023).
USGS (2021b). USGS water data little Manatee River [online] Available at: https://waterdata.usgs.gov/monitoring-location/02300500/#parameterCode=00060&startDT=2021-04-01&endDT=2022-05-01 (Accessed November 1, 2023).
Utermohl, H. (1958). To the perfection of quantitative phytoplankton methodology. Mitt. Int. Ver. Theor. Angew. Limnol. 9, 1–38.
Vargo, G. A. (2009). A brief summary of the physiology and ecology of Karenia brevis Davis (G. Hansen and Moestrup comb. nov.) red tides on the West Florida shelf and of hypotheses posed for their initiation, growth, maintenance, and termination. Harmful Algae 8, 573–584. doi: 10.1016/j.hal.2008.11.002
Verity, P. G., Robertson, C. Y., Tronzo, C. R., Andrews, M. G., Nelson, J. R., and Sieracki, M. E. (1992). Relationships between cell volume and the carbon and nitrogen content of marine photosynthetic nanoplankton. Limnol. Oceanogr. 37, 1434–1446. doi: 10.4319/lo.1992.37.7.1434
Walton, M., Al-Maslamani, I., Haddaway, N., Kennedy, H., Castillo, A., Al-Ansari, E., et al. (2016). Extreme 15N depletion in seagrasses. Estuar. Coasts 39, 1709–1723. doi: 10.1007/s12237-016-0103-3
Webster, P. J., Holland, G. J., Curry, J. A., and Chang, H.-R. (2005). Changes in tropical cyclone number, duration, and intensity in a warming environment. Science 309, 1844–1846. doi: 10.1126/science.1116448
Weisberg, R. H., Liu, Y., Lembke, C., Hu, C., Hubbard, K., and Garrett, M. (2019). The coastal ocean circulation influence on the 2018 West Florida Shelf K. brevis red tide bloom. J. Geophys. Res. Oceans 124, 2501–2512. doi: 10.1029/2018JC014887
Wetz, M. S., and Yoskowitz, D. W. (2013). An “extreme” future for estuaries? Effects of extreme climatic events on estuarine water quality and ecology. Mar. Pollut. Bull. 69, 7–18. doi: 10.1016/j.marpolbul.2013.01.020
Work, K., Havens, K., Sharfstein, B., and East, T. (2005). How important is bacterial carbon to planktonic grazers in a turbid, subtropical lake? J. Plankton Res. 27, 357–372. doi: 10.1093/plankt/fbi013
Keywords: phosphogypsum, stable isotopes, phytoplankton, harmful algal bloom, carbon, nitrogen, Piney Point
Citation: Morrison ES, Phlips E, Badylak S, Chappel AR, Altieri AH, Osborne TZ, Tomasko D, Beck MW and Sherwood E (2023) The response of Tampa Bay to a legacy mining nutrient release in the year following the event. Front. Ecol. Evol. 11:1144778. doi: 10.3389/fevo.2023.1144778
Received: 15 January 2023; Accepted: 24 March 2023;
Published: 24 May 2023.
Edited by:
Albertus J. Smit, University of the Western Cape, South AfricaReviewed by:
S. Lan Smith, Japan Agency for Marine-Earth Science and Technology (JAMSTEC), JapanCopyright © 2023 Morrison, Phlips, Badylak, Chappel, Altieri, Osborne, Tomasko, Beck and Sherwood. This is an open-access article distributed under the terms of the Creative Commons Attribution License (CC BY). The use, distribution or reproduction in other forums is permitted, provided the original author(s) and the copyright owner(s) are credited and that the original publication in this journal is cited, in accordance with accepted academic practice. No use, distribution or reproduction is permitted which does not comply with these terms.
*Correspondence: Elise S. Morrison, ZWxpc2UubW9ycmlzb25AZXNzaWUudWZsLmVkdQ==
Disclaimer: All claims expressed in this article are solely those of the authors and do not necessarily represent those of their affiliated organizations, or those of the publisher, the editors and the reviewers. Any product that may be evaluated in this article or claim that may be made by its manufacturer is not guaranteed or endorsed by the publisher.
Research integrity at Frontiers
Learn more about the work of our research integrity team to safeguard the quality of each article we publish.