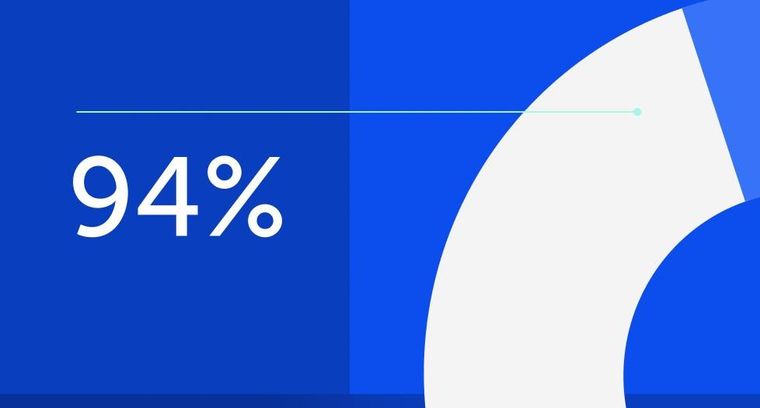
94% of researchers rate our articles as excellent or good
Learn more about the work of our research integrity team to safeguard the quality of each article we publish.
Find out more
ORIGINAL RESEARCH article
Front. Ecol. Evol., 03 March 2023
Sec. Ecophysiology
Volume 11 - 2023 | https://doi.org/10.3389/fevo.2023.1139053
Introduction: Plant richness is thought to improve the function of constructed wetlands (CWs), but most CWs are planted with monocultures, with only a few employed polycultures, which have drawn contradictory conclusions. We suppose functional diversity is the key to better performance of plant communities and hypothesize that CWs planted with diverse growth forms are superior in plant growth and nutrient removal.
Methods: In this study, six emergent plant species categorized into slender type (Schoenoplectus tabernaemontani, Typha orientalis), fan type (Iris sibirica, Acorus calamus) and large type (Canna indica and Thalia dealbata) were planted in monocultures, combinations (two species of the same growth form) and mixed polycultures (six species of three growth forms). We then compared how plant growth and nutrient uptake differed among treatments.
Results: It showed that the polyculture considerably increased the removal of total nitrogen (TN) and total phosphorus (TP), but the combination did not outperform monoculture. High consistency in the patterns between underground biomass and total biomass indicated that plant roots were essential for nutrient consumption. Compared with slender and fan plants, the large plants had a greater biomass increase in polycultures, which greatly accelerated the absorption and assimilation of TN and TP.
Conclusion: Our study indicated that plant community with various growth forms reduced the intensity of interspecific competition, increased the functional diversity, and greatly enhanced the ability of pollutant removal. Our results also provide some suggestions for plant selection and combination designs in CWs.
Urban wastewater has become a global concern due to the rapid progress of urbanization. A significant amount of untreated domestic and industrial wastewater loaded with high levels of nitrogen (N) and phosphorus (P) has been discharged into grounds, rivers, and lakes, gravely harming urban ecosystems with water bloom as one of the most prominent consequences (Villar-Navarro et al., 2018; Russo et al., 2019; Wurtsbaugh et al., 2019). Constructed wetlands (CWs) have been widely used because they are economic and high-efficient compared with traditional approaches. Planted CWs are more efficient than unplanted CWs in wastewater treatment (Paranychianakis et al., 2016; Zhu et al., 2018) because plants play an important role in assimilating nutrition such as N and P and help with nutrient retention (Geng et al., 2017). In addition, plant roots create a natural biofilm filtering system, which not only provides suitable habitats for microorganisms, but also exhibits large surface areas to reduce flow velocity and promote pollutant sedimentation (Carballeira et al., 2016; Sandoval et al., 2019).
Plant selection is a key when constructing CWs, because there may be species-specific effects on biomass accumulation, contaminant removal, and ornamental value (Long et al., 2016; Monokrousos et al., 2020). The most commonly used plants in CWs are Phragmites australis, Typha latifolia, Canna indica, and Cyperus papyrus, owing to their strong adaptability and high pollutant removal potential (Wu et al., 2019; Marín-Muñiz et al., 2020). Another component influencing the rate of pollutant removal is interspecific interaction when more than one species is grown in CWs, the outcome of which is typically dependent on tolerance and competitiveness. For instance, in a polyculture-CW, Agapanthus africanus had fewer new shoots and shorter stems than others and completely vanished from the community after 1 year of operation, possibly due to its weak competitive ability and slow growth (Calheiros et al., 2015). The competitiveness of Pistia stratiotes was found to be lower than that of Phragmites karka and T. latifolia, which resulted in a lower growth rate (Kumar et al., 2022). In comparison to a CW with Oenanthe hookeri and Reineckia carnea, the removal efficiency of P in a CW with O. hookeri and Rumex japonicas was much higher (Geng et al., 2017). All the above studies reveal that both species composition and interaction are crucial to the functioning of CWs.
It is generally considered that higher plant diversity enhances ecosystem resilience, stability, interference resistance, and pollutant removal rate (Hautier et al., 2018; Zhang et al., 2021). It then can be extrapolated that CWs in polycultures should outperform those in monocultures. The main reasoning is that the complementarity of different plant species across time, space, and function can make the CWs more resistant to environmental changes for a longer period of time (Zhang et al., 2010; Kumar et al., 2021). Additionally, diverse root morphology and the vertical distribution and layering in polyculture CWs increased the contact area with pollutants (Liang et al., 2011; Marchand et al., 2014), aided the absorption and storage of nutrients, as well as speeded up the decomposition of organic matters associated with elevated root exudates and microbial communities (Arslan et al., 2017; Hussain et al., 2018). However, many studies found that polyculture CWs were not different from or superior to monocultures in nutrient removal. When Rodriguez and Brisson (2016) compared the removal rates of N and P in the monoculture and polyculture of P. australis and Phalaris arundinacea, the monoculture of P. australis enjoyed a comparable or higher removal rate than polyculture. Furthermore, there was no significant difference in N removal between monoculture and polyculture composed of C. papyrus and Zantedeschia aethiopica (Leiva et al., 2018). The lack of difference was also found for the monoculture of C. indica and polyculture with C. flabelliformis, P. australis, Pennisetum purpureum, and Hymenocallis littoralis (Liang et al., 2011). It is worth mentioning that in the studies above, although more than one species was planted, they did not differ much in individual sizes or shapes. Such a mixture of similar species may not increase the functional richness of CWs, rendering intense interspecific competition and reduced growth in both parties (Samal et al., 2017; Leiva et al., 2018). Functional diversity is a biodiversity indicator to explore the connection between biodiversity and ecosystem function. It can reflect the functional differences of communities and be evaluated based on morphology, physiology, and anatomy (Steudel et al., 2016). Moreover, it is the fundamental driver of how biodiversity affects ecosystem function (Petchey and Gaston, 2002; Poos et al., 2009). Therefore, when building CWs, polycultures with functional diversity should be considered, which might minimize the degree of niche overlap, increase complementarity, and improve nutrient uptake.
Plant growth form, an ecological classification based on the morphology, appearance, and structure of plants (Wang et al., 2020), provides an informative indicator for evaluating functional diversity. It is a comprehensive reflection of the growth conditions and genetic background of plants, and to a certain extent, it reflects the strategies of adaptation in different ecological niches (Antos et al., 2021). Diversified growth forms in a plant community usually result in positive feedbacks (Dell'Osbel et al., 2020; Kumar et al., 2021). Indeed, differences in nutrient demands can elevate complementarity (Ma et al., 2017; Ediviani et al., 2018). Diverse growth rhythms could also extend the period for nutrient uptake across seasons with efficient nutrient retention (Manolaki et al., 2020). To date, most planted CWs are monoculture or with a low plant richness, and only a handful of researches employed polyculture, which has drawn contradictory conclusions (Aguinaga et al., 2018; Fahim et al., 2021; Carrillo et al., 2022). Very few studies have considered polyculture with various growth forms, in order to increase the functional diversity of plants and the nutrition turnover rate of CWs (Calheiros et al., 2015).
In this study, we are interested in how growth forms affect species interactions, which in turn influence biomass accumulation and nutrition removal. We selected six commonly-used species in CWs and categorized them into three growth forms: the slender, fan, and large types (Figure 1A). Growing them with different combination designs in CWs, we test three hypotheses. First, polycultures with various growth forms might be the most efficient in removing N and P from water. Because when the six species are mixed, the complementarity of interspecific relationships and the diversity of functional groups will promote plant growth and hence a greater nutrient removal rate (Zhang et al., 2010; Kumar et al., 2021). Second, an increase in plant richness with the same growth form should have little impact on water purification. In such a plant mixture, although species richness is increased, the functional diversity stays the same. We expect to see intense interspecific competition between plants of the same growth type (Samal et al., 2017; Leiva et al., 2018). Thus, there should be no evident increase in biomass accumulation or nutrient removal compared to monoculture. Third, plants of the large shape may present a higher ability in nutrient removal, because large-sized plants are usually with substantial biomass, complex subterranean systems, fast growth, rapid community establishment, and good tolerance (Licata et al., 2019; Kumar et al., 2022), all contributing to higher nutrient absorption rates. Therefore, they might be particularly useful in CWs given appropriate densities and accompanying species.
Figure 1. Plant species selection and the experimental setting: (A) drawings of six species and their growth form categories; (B) the layout of three treatments in culturing tanks (the location of different plant species in combination and polyculture was randomized); (C) growing condition in the greenhouse. Please note that all species have reproductive structures drawn for the ease of recognition, which did not represent the developmental state of individuals during culturing. See texts for detailed information.
The experiment was carried out in the greenhouse at the Wuchang campus of Hubei University (Wuhan, China) near School of Resources and Environmental Science (30°34′46′′N, 114°19′40′′E) in the year 2021. The room used in the greenhouse is 50 m2 in size and contains four 1 × 4 m2 beds. Three overhead sodium lamps were used as supplementary lighting for each bed. Wet curtains and ventilating fans worked together to keep the moisture and temperature appropriate for plant growth. On average, the temperature was around 30°C during the day (8:00–20:00), and 25°C at night (20:00–8:00), whereas the humidity was about 50%. In this study, surface-flow CWs were built in plastic tanks of 63 × 45 × 39 cm3 (length × width × depth). Sand was added to the bottom to a depth of 8 cm for plant anchorage. Before usage, all sand was washed 5–6 times with tap water until the solution turned clear to avoid any impurities or soluble minerals from influencing the results. To completely eliminate the remained forms of N and P, the sand was immersed in 0.2 mol/l diluted hydrochloric acids for 1–2 days and then successively rinsed with tap water and distilled water.
In order to choose plant species to construct experimental wetlands, we came up with three criteria that plant species should be: (1) commonly used in CWs and easily acquired; (2) emergent with ornamental values; and (3) of different growth forms. Thus, six common aquatic species were chosen with three growth forms, namely, the slender type (Schoenoplectus tabernaemontani, Typha orientalis), the fan type (Iris sibirica, Acorus calamus), and the large type (Canna indica and Thalia dealbata) (Figure 1A). Plants of slender growth form grow longitudinally with long and slender leaves. Two species of the fan form share fan-shaped leaf arrangements, usually with a flat and short appearance, whereas the large type is of big size, with dense leaves and massive rhizomes, and mature plants can reach 1.5–2.0 m tall. We bought seedlings of the six species from the same nursery and trimmed all into a certain height (slender: 45 cm; fan: 45 cm; large: 50 cm). Before planting, we made sure no extra minerals from leaves or roots were brought into culturing tanks by washing the whole plants 2–3 times with tap and distilled water. We also measured the initial fresh weight of each seedling.
Three treatments—monoculture, combination, and polyculture—representing one species, two species of the same growth form, and six species of three growth forms—were set up in this experiment (Figure 1B). After careful pilot trials, it was decided that 12 seedlings to be planted (3 × 4) in each culturing tank, with approximately a planting density of 44 plants/m2. Because the main purpose of our study was to discover the effect of plant richness (with niches overlapping or diverse growth forms), we made sure that each treatment had the same number of repeating tanks (n = 18). For the polyculture treatment, each tank had all six species mixed and two seedling of each species were planted with randomized locations. In the monoculture, each species was repeated for three culturing tanks, together accounting for 18 replications. In the combination, each growth type (slender, fan, large) composed of two species was repeated for six tanks, and six seedlings of each species were planted per tank. In such a manner, not only did the monoculture and combination have the same number of growth types represented at tank level, but also the number of seedlings per plant species was balanced (n = 36) across three treatments. All 54 culturing tanks were evenly distributed among four beds in the greenhouse (Figure 1C). To plant all seedlings, each tank was filled with 30 L of 10% Hoagland nutrient solution (HB8870-1, Qingdao Haibo Biotechnology Co., Ltd.) at the initial stage. In order to replace dead plants and maintain an equal number of each species throughout the experiment, several extra plants of each species were simultaneously grown alongside. Seedlings were planted on July 6 and harvested on September 13, 2021. As a portion of plants showed tissue degradation after 2 months of cultivation, which might affect the effectiveness of nutrient removal if left untreated, we decided to end the experiment then.
In order to simulate the eutrophic state of urban sewage, additional N and P in the form of ammonium chloride (NH4Cl) and potassium dihydrogen phosphate (KH2PO4) were added several times during plant growth (Figure 2). Our plan was to add 3 liters of nutrient solution with 25 mg/L N and 3 mg/L P (that is, 75 mg of N and 9 mg of P) every 5 days from July 25 (about 20 days after all seedlings were planted). Following each addition, the water volume in each tank was refilled with distilled water to 30 L. There were some modifications according to the growth condition and nutrient dynamics in culturing tanks throughout the course of the experiment (see Figure 2).
Figure 2. Concentration changes of total nitrogen [TN, (A)] and total phosphorus [TP, (B)] in culturing tanks during the whole experimental period under different treatments.
To record the dynamics of nutrients with the growth of plants in the CWs, we kept measuring of N and P concentrations from the initial phase (several hours after plants were settled) to the end of the experiment. Each measurement was taken after the addition of N and P when the tanks were metered to a constant volume of 30 L. To collect water samples, we drew 20 mL of water from 5 sites within each tank, namely four corners and the center, and mixed them together for measurement. Total nitrogen (TN) and total phosphorus (TP) concentrations were measured by alkaline potassium persulfate digestion UV spectrophotometry method and ammonium molybdate spectrophotometry method, respectively. Total removal (TR) of N and P were calculated as follows:
where C1 is the initial concentration; A is the total amount added; C2 is the final concentration; V is the total volume of culturing solution (=30 L).
In addition to the concentration of N and P, we also measured water dissolved oxygen (DO) concentration and pH in all tanks with a portable water quality analyzer (HQ40d, HACH). Ten measurements were taken once every 5 days across the experimental period. Each measurement was conducted between 9 and 11 a.m. and the probes were placed at the center of culturing tanks, roughly 5 cm below water surface. The probes were washed and dried between measurements.
At harvest, all plant individuals were cleaned and air-dried, after which final fresh weights were measured. Each plant was then cut into two parts: above- and below-ground. The dry biomass of above-ground, below-ground, and total were weighed and calculated after they had been placed in the drying oven for 48 h at 70°C. The fresh weight increase (FWI) was calculated by comparing final and initial states. During the process of the experiment, several plants died out of no clear reasons. In order to keep all culturing tanks at constant density, we replaced the dead plants with alive ones of similar sizes right away. However, strict controls over plant weight were not possible, which is why some FWIs turned to be negative.
All analyses were done using R version 4.0.2 (R Core Team, 2020) and packages including car (Fox and Weisberg, 2019), psych (Revelle, 2018), lmerTest (Kuznetsova et al., 2017), and emmeans (Lenth, 2022).
For plant biomass variables, each plant individual was an experimental unit. We initially added factors of “planting treatments” (monoculture, combination, and polyculture), “growth forms” (slender, fan, and large types), and “species nested within growth forms” in linear models. However, as the interactions between treatment and growth form, and between treatment and species turned out to be significant, we tested the effects of treatment in three hierarchical levels. First, we employed linear mixed models to test whether biomass was different among treatments by controlling species as a random effect to account for different growing patterns among species. Second, data were separated into three growth forms and the same model was used as above. Third, data were separated into six plant species and treatment effects were tested with ANOVA.
For N and P removal, each culturing tank was an experimental unit. One-way ANOVAs were used to test the effects of planting treatment on the total removal. Furthermore, to simultaneously test the effects of treatments and growth forms, only monoculture and combination tanks were included (polyculture tanks had diverse growth forms and thus were not applicable). Models were initially added with the interaction between treatments and growth forms, which showed a lack of significance and thus were removed. Hence, both variables were tested with two-way ANOVAs.
For water pH and dissolved oxygen (DO), ten measurements of each culturing tank were taken evenly spaced throughout the growing period, across which 5–7 times of nutrition addition were also conducted to disrupt the physicochemical property of the water. Thus, each measurement was regarded as relatively independent, and each measuring time was a block. Linear mixed models were used to test the difference in pH and DO among treatments. To further examine the effects of growth forms, only tanks of monoculture and combination were considered. Since the treatment factor might persist in the sub-dataset, its interaction with growth forms was tested for pH, which was not significant and removed. But for DO, the interaction term was significant, indicating altered response patterns of three growth forms between monoculture and combination. We, therefore, separated the dataset by treatment and looked into the effect of growth form individually. Results showed that in both treatments, the DO of those tanks with large growth forms was significantly lower than others. The only difference was that the degree of decrease appeared stronger in the combination treatment. Given the exact same trend and for a concise result presentation, we employed the same linear mixed model for both pH and DO, where “growth form” and “treatment” were fixed factors and “measuring time” a random factor.
In all ANOVAs, we have made sure the homogeneity of variances and normality of errors were met (pH was log-transformed). Only a few violations of homoscedasticity were detected, and data transformation did not make it better. But given significant differences among treatments and quite similar sample sizes, we considered the results trustable. Least-squares means and standard errors were reported for variables analyzed with mixed models using the package emmeans, otherwise, parametric means were reported. Significance in mixed models was evaluated by Type III Satterthwaite’s method using the package lmerTest. Post-hoc comparisons were tested with Tukey adjustment.
Total dry biomass (TDB) showed significant differences among treatments (F2, 610 = 5.40, p = 0.005), growth forms (F2, 610 = 360.15, p < 0.0001) and species (F3, 610 = 96.17, p < 0.0001). Compared with monoculture and combination, TDB of polyculture was significantly higher (Table 1; Figure 3A). The changes in TDB for each growth form under three treatments varied as well (Table 1; Figure 3B). While the TDB of polyculture was significantly lower than that of monoculture and combination in the case of slender plants, there was no significant difference among the three treatments in the fan-shaped group. Yet, the TDB of large plants in polyculture was significantly higher than in monoculture and combination. Regardless of the treatments, the TDB of the large type was always higher than that of slender and fan (Figure 3B). The TDB of each species also responded differently to treatments (Table 1; Figure 3C). For S. tabernaemontani, plants grown in polyculture had significantly lower TDB than those in combination. Compared to monoculture, the TDB of T. orientalis and I. sibirica were significantly reduced in polyculture. Plants of A. calamus exhibited no significant difference in TDB among three treatments. The TDB of C. indica and T. dealbata in polyculture was significantly higher than in monoculture and combination. Both of large growth form, C. indica had a higher TDB than T. dealbata.
Table 1. Treatment effects (monoculture, combination, and polyculture) on plant biomass variables in linear (mixed) models.
Figure 3. Differences in total dry biomass (A-C), belowground dry biomass (D-F), aboveground dry biomass (G-I), and fresh weight increase (J-L), among monoculture (green), combination (blue), and polyculture (rose) treatments. *Means significant differences with the other two treatments, means significant differences between two treatments, and unlabeled bars mean lack of significant differences among treatments.
When TDB was divided into aboveground (ADB) and belowground (BDB) components, the variations in dry biomass among treatments, growth forms, and species were also evident (Figures 3D–I). By comparing them with the patterns of TDB, it demonstrated great consistency in treatment effect on BDB and TDB. First, among the treatments, polyculture had a stronger impact on plants’ dry biomass. Second, among different growth forms, the large type typically had a higher degree of increase in biomass. This was also true when examined at species level. Thus, the change in plants’ TDB could be largely attributed to the processes happening belowground.
The patterns in the fresh weight increase (FWI) across treatments, growth forms, and species share three important similarities with TDB. First, when compared to monoculture and combination, the increase in polyculture was significantly higher (Table 1; Figure 3J). Second, the degree of increase differed among growth types, where large plants enjoyed greater increase than slender and fan ones (Table 1; Figure 3K). And third, for individual species, polyculture significantly outperformed monoculture and combination in C. indica and T. dealbata (Table 1; Figure 3L). The figure also showed that C. indica had the strongest response among all species. As to the minute bars of fresh weight in slender and fan plants, it suggested greater water content in large plants, which might facilitate their nutrient metabolism.
The concentrations of TN and TP in the culturing tanks started to differ among the three treatments after around 20 days of growth, and the differences persisted till the end of the experiment (Figure 2). The polyculture tanks had the lowest TN and TP concentrations, followed by the combination, and the monoculture with the greatest. The concentrations of TN and TP experienced the steepest drop over the first 20–25 days of the experiment, which should be caused by the rapid initial growth of plants. The concentration of TN rose in three intervals in all three treatments, probably owing to higher dosages of N were added and the plants’ absorption became slower (Figure 2). The concentration of TP reached a peak on Aug 4th due to a high dosage of addition. Nevertheless, plants were able to consume most of the added P and brought the concentration to a low level at the end of the experiment (Figure 2). The concentration of TN considerably dropped at the end of the experiment, possibly because large-typed plants entered the reproductive period and required more nutrition.
The TN and TP removal in culturing water varied significantly among the three treatments. Compared to monoculture and combination, polyculture considerably increased the removal of TN (F2, 51 = 3.17, p = 0.05; Figure 4A) and TP (F2, 51 = 5.16, p = 0.009; Figure 4B). When polyculture was removed, both factors of growth forms and treatments were examined simultaneously in the remaining tanks. The effects of treatment were consistent with previous results, that is, monoculture and combination did not differ in TN (F1, 32 = 0.34, p = 0.562) and TP removal (F1, 32 = 2.58, p = 0.118). However, different growth forms varied remarkably in TN (F2,32 = 17.78, p < 0.0001) and TP removal (F1, 32 = 19.28, p < 0.0001), with a similar pattern that the large plants were of greater nutrition absorption (Figures 4C,D).
Figure 4. Differences of total nitrogen removal [TN, (A,C)] and total phosphorus removal [TP, (B,D)] among three treatments and different growth forms. Different letters above bars represent significant differences.
The concentration of DO had no significant difference among monoculture, combination, and polyculture (Table 2). However, there were notable differences across three growth types, with the large type having a much lower concentration of DO than slender and fan (Table 2). The pH of culturing water varied significantly depending on the treatment and growth type. The water was acidic in all three treatments, and the acidity rose with the number of species (Table 2). The water was also acidic for all three growth types, with large type having the strongest acidity followed by fan, and slender the weakest (Table 2).
Table 2. The concentration of dissolved oxygen (mg/L) and pH in culturing tanks of different treatments and growth forms.
Results showed that CWs planted with three different growth forms were the most efficient in consuming dissolved N and P, which is consistent with our hypothesis that combining plants with various growth forms in a polyculture is the most efficient in removing N and P from water. Given that the substrates, nutrient solution, temperature, light, and growing density of all culturing tanks were the same, the most possible reason is the higher species richness and functional diversity in polycultures with various growth forms (Cardinale, 2011; Huang et al., 2020). Correspondingly, both total dry biomass and fresh weight increase of plants in polyculture was also significantly higher than that of monoculture and combination, suggesting that plant growth effectively contributes to the removal of nutrients. Especially for C. indica and T. dealbata in polyculture, both had their fresh weight increased about 1.5 to 2.0 times than in monoculture, indicating promoted growth in response to niche differentiation and reduced competition. However, such positive effects were not universal for all species. For slender-formed S. tabernaemontani and T. orientalis, and I. sibirica, their growth, especially aboveground weight, was somewhat reduced. This may be due to strong shading effects (or allelopathy, see below) from large plants, which were not present in their monocultures and combinations. But the strength of the reduction in biomass was trivial compared to the strength of the increase in large plants (Figure 2), which had little impact on the overarching pattern.
Studies suggest that the removal of contaminants was strongly tied to belowground processes (Ge et al., 2011; Schultz et al., 2012; Wang et al., 2013). Hence, polyculture may have also elevated the activity of rhizospheric microorganisms through diverse structures and density of roots (Carballeira et al., 2016; Limpert et al., 2020). Indeed, we found greater belowground biomass in polycultures (Figure 2), which implies that belowground roots may have played an essential role. The roots of six planted species are of various structures. Specifically, the four species of slender and fan types grow rhizomes, in which S. tabernaemontani sends out shallow runners with dense root hairs; T. orientalis has horizontal fleshy rhizomes; both A. calamus and I. sibirica develop deeper rhizomes and dense fibrous roots. In contrast, C. indica and T. dealbata have tuberous rootstocks, on which extensive adventitious roots grow. It was quite evident and statistically significant that the roots of C. indica and T. dealbata in polyculture had higher biomass (1.5–1.7 times) than in monoculture and combination, which indicated greater ability in water purification. Increased root activity in polyculture and large plants could also explain the change in dissolved oxygen and pH in the culturing tanks, because rhizosphere should have consumed more oxygen and secreted more organic acids during decomposition (Tanner et al., 2005; Paranychianakis et al., 2016; Zhao et al., 2016). Other researchers have pointed out the crosstalk among distinct belowground microbial communities associated with different plants (Fan et al., 2018), and positive feedback between below and above grounds (Arslan et al., 2017), identifying alternative mechanisms in how plant diversity promotes purification functioning of CWs. It might be the case in our study, but more investigations need to be carried out to interpret the role of microorganisms.
When two species of the same growth form were planted together, we did not find improved growth or nutrient removal. This agrees with our hypothesis that an increase in plant richness with the same growth form had little impact on water purification, further suggesting that it is the functional diversity, not the pure number of plant species that could facilitate the performance of plant communities (Geng et al., 2017). The key probably lies in antagonistic interspecific interactions when two species share a high level of similarities (Ellawala Kankanamge and Kodithuwakku, 2017). Hence, we also expected to see strong competition or even competitive exclusion in the combination treatment (Adler et al., 2018; Geng et al., 2019). Despite that none of the experimental combinations resulted in one species replacing the other, the results clearly suggested competitive relationships in slender and fan combinations, where S. tabernaemontani showed high competitiveness and increased growth in combination than monoculture, while plants of I. sibirica were of weaker ability than A. calamus and suppressed. It has been reported that the rhizome extract of T. dealbata had allelopathic effects on others in seed germination, seedling growth metabolism, and root activity (Miao et al., 2012). In our experiment, the water in some tanks became black after growing C. indica and T. dealbata together. The mortality rate of C. indica was unusually high, with one tank reaching 50% within a week. Such mortality, however, was not observed in the C. indica monocultures, demonstrating that cannas were probably inhibited by the allelopathy of T. dealbata. It should be noted that mortality was not included in our results because dead plants, whenever discovered, were replaced with healthy ones, in order to maintain the same plant density of all treatments. The allelochemicals of T. dealbata should also be present in polycultures (despite of lower concentrations), which partly explains the suppressed growth of S. tabernaemontani, T. orientalis, and I. sibirica. Nonetheless, the performance of A. calamus and C. indica in polycultures were not negatively affected, perhaps suggesting their higher levels of tolerance.
Large-shaped plants, C. indica and T. dealbata, not only had higher rates of TN and TP removal than slender and fan types (Figure 4), but also seemed to contribute disproportionately to the biomass increase in polycultures (Figure 2). This echoes studies that found plants with extensive growth of roots and leaves speeded up nutrition absorption more than medium or small-sized plants (Liu et al., 2020; Teubner et al., 2022), which points out that it is essential to have species of large sizes considered and selected in CWs and sewage treatment (Levi et al., 2015). During our experiment, only the individuals of C. indica and T. dealbata entered the flowering stage, and reproduction might have elevated the uptake of various nutrients as well. Besides, large plants are also considered beneficial in suppressing sediment resuspension and keeping the aquatic environment warm by reducing wind speed in nature (Rehman et al., 2017), thus facilitating the water purification function of CWs. Nevertheless, large-sized plants are certainly not a panacea for all conditions. Studies have indicated that the removal rate of P by slender Schoenoplectus nearly doubled that of large-shaped Phragmites, especially in winter (López et al., 2016; Carrillo et al., 2022). C. indica was better than P. purpureum at TN removal in summer but the opposite was true in winter (Yang et al., 2007). Evidently, seasonality needs to be taken into consideration. While large plants mainly grow in summer, others may be more important in nutrient uptake across other seasons. In addition, plant density might play a role in the better growth of large plants in polycultures (Webb et al., 2013), because the density of large plants was essentially reduced when co-grown with slender- and fan-shaped plants of much lower biomass. However, the improved function of polycultures was more likely a collective effect of three growth forms rather than by the large form itself because each polyculture tank only had 2 large plants, compared with 12 in those monoculture and combination tanks.
Iris sibirica and T. orientalis, both commonly used in CWs, have been reported to greatly increase contaminant removal in wastewater (Ma et al., 2017; Zhu et al., 2017). However, the growth of the two plant species was poor in our study. We observed a higher mortality rate of T. orientalis in polyculture (16.67%) than that in monoculture (5.56%), suggesting that the growth of Typha was not promoted by niche differentiation, but instead inhibited, probably by the allelopathic effect of T. dealbata. Tillers of T. orientalis were not observed until after half a month of culturing. The slow developmental rate of this species might also result in shading by others when grown in a dense and diverse community. The poor growth of I. sibirica was likely ascribed to high temperatures during the experimental period. It has been reported that I. sibirica had the highest nutrient uptake rate in winter and was considered the most effective overwintering plant (Gao et al., 2014; Ma et al., 2017). We may observe better growth of I. sibirica if the trial duration was extended. In the future, it is worthwhile to conduct plant cultivation for longer durations, as it may reveal species interaction and nutrient dynamics under a more realistic scenario.
In conclusion, species richness and growth form did influence CW efficiency. Our results highlight that polyculture with various growth forms is the most efficient in pollutant removal. Meanwhile, various phenology and diverse ornamental value of different species add to the diversity of sceneries in urban wetlands (Rodriguez and Brisson, 2016; Leiva et al., 2018). It is noteworthy that the setting of our experiment was inside a greenhouse, which largely excluded natural pathogens and insect herbivores. If grown in natural habitats, species functional diversity in polyculture might provide additional benefits in diverse interspecific relationships, promoting more stable and sustainable ecosystem functions (Steudel et al., 2016).
The raw data supporting the conclusions of this article will be made available by the authors, without undue reservation.
YL: data analysis, visualization, and writing – original draft. QC: investigation. FL: conceptualization and methodology. CD: conceptualization, data analysis, writing – original draft, and supervision. All authors contributed to the article and approved the submitted version.
This study was funded by the Natural Science Foundation of Hubei Province of China grant 2019CFA066 (CD) and Hubei Engineering Research Center for Protection and Utilization of Special Biological Resources in the Hanjiang River Basin grant 2021-09 (CD).
We thank ZQ Li, LF Yang and JM Su at Hubei University for sharing lab equipment. We are grateful to all colleagues of Lab 302 at School of Resources and Environmental Science, Hubei University for experimental help. They are XT Xie, HQ Tang, ZY Xu, QT Peng, H Yang, HZ Mao, A Hu, BB Huo, SS Cui, C Liu, WH Yang, X Chen, YL Wu, CY Chen, JQ Zhang, and J Zhu.
The authors declare that the research was conducted in the absence of any commercial or financial relationships that could be construed as a potential conflict of interest.
All claims expressed in this article are solely those of the authors and do not necessarily represent those of their affiliated organizations, or those of the publisher, the editors and the reviewers. Any product that may be evaluated in this article, or claim that may be made by its manufacturer, is not guaranteed or endorsed by the publisher.
Adler, P. B., Smull, D., Beard, K. H., Choi, R. T., Furniss, T., Kulmatiski, A., et al. (2018). Competition and coexistence in plant communities: intraspecific competition is stronger than interspecific competition. Ecol. Lett. 21, 1319–1329. doi: 10.1111/ele.13098
Aguinaga, O. E., McMahon, A., White, K. N., Dean, A. P., and Pittman, J. K. (2018). Microbial community shifts in response to acid mine drainage pollution within a natural wetland ecosystem. Front. Microbiol. 9:1445. doi: 10.3389/fmicb.2018.01445
Antos, J. A., Zobel, D. B., and Fischer, D. G. (2021). Belowground morphology and population dynamics of two forest understory herbs of contrasting growth forms. Botany 99, 569–580. doi: 10.1139/cjb-2021-0035
Arslan, M., Imran, A., Khan, Q. M., and Afzal, M. (2017). Plant–bacteria partnerships for the remediation of persistent organic pollutants. Environ. Sci. Pollut. Res. 24, 4322–4336. doi: 10.1007/s11356-015-4935-3
Calheiros, C. S., Bessa, V. S., Mesquita, R. B., Brix, H., Rangel, A. O., and Castro, P. M. (2015). Constructed wetland with a polyculture of ornamental plants for wastewater treatment at a rural tourism facility. Ecol. Eng. 79, 1–7. doi: 10.1016/j.ecoleng.2015.03.001
Carballeira, T., Ruiz, I., and Soto, M. (2016). Effect of plants and surface loading rate on the treatment efficiency of shallow subsurface constructed wetlands. Ecol. Eng. 90, 203–214. doi: 10.1016/j.ecoleng.2016.01.038
Cardinale, B. J. (2011). Biodiversity improves water quality through niche partitioning. Nature 472, 86–89. doi: 10.1038/nature09904
Carrillo, V., Gómez, G., and Vidal, G. (2022). Phosphorus uptake by macrophyte plants in monocultures and polycultures in constructed wetlands for wastewater treatment. Ecol. Eng. 182:106690. doi: 10.1016/j.ecoleng.2022.106690
Dell'Osbel, N., Colares, G. S., Oliveira, G. A., Rodrigues, L. R., da Silva, F. P., Rodriguez, A. L., et al. (2020). Hybrid constructed wetlands for the treatment of urban wastewaters: increased nutrient removal and landscape potential. Ecol. Eng. 158:106072. doi: 10.1016/j.ecoleng.2020.106072
Ediviani, W., Priadi, C. R., and Moersidik, S. S. (2018). Nutrient uptake from liquid digestate using ornamental aquatic macrophytes (Canna indica, Iris pseudacorus, Typha latifolia) in a constructed wetland system. J. Phys. Conf. Ser. 1022:012052. doi: 10.1088/1742-6596/1022/1/012052
Ellawala Kankanamge, C., and Kodithuwakku, H. (2017). Effect of interspecific competition on the growth and nutrient uptake of three macrophytes in nutrient-rich water. Aquat. Ecol. 51, 625–634. doi: 10.1007/s10452-017-9640-5
Fahim, R., Xiwu, L., and Jilani, G. (2021). Feasibility of using divergent plantation to aggrandize the pollutants abatement from sewage and biomass production in treatment wetlands. Ecohydrol. Hydrobiol. 21, 731–746. doi: 10.1016/j.ecohyd.2021.05.003
Fan, X., Ding, S., Gong, M., Chen, M., Gao, S., Jin, Z., et al. (2018). Different influences of bacterial communities on Fe (III) reduction and phosphorus availability in sediments of the cyanobacteria-and macrophyte-dominated zones. Front. Microbiol. 9:2636. doi: 10.3389/fmicb.2018.02636
Fox, J., and Weisberg, S., (2019). An R Companion to Applied Regression. 3rd. Thousand Oaks, CA, USA: Sage Publication.
Gao, J., Wang, W., Guo, X., Zhu, S., Chen, S., and Zhang, R. (2014). Nutrient removal capability and growth characteristics of Iris sibirica in subsurface vertical flow constructed wetlands in winter. Ecol. Eng. 70, 351–361. doi: 10.1016/j.ecoleng.2014.06.006
Ge, Y., Zhang, C., Jiang, Y., Yue, C., Jiang, Q., Min, H., et al. (2011). Soil microbial abundances and enzyme activities in different rhizospheres in an integrated vertical flow constructed wetland. CLEAN–soil. Air, Water. 39, 206–211. doi: 10.1002/clen.201000230
Geng, Y., Ge, Y., Luo, B., Chen, Z., Min, Y., Schmid, B., et al. (2019). Plant diversity increases N removal in constructed wetlands when multiple rather than single N processes are considered. Ecol. Appl. 29:e01965. doi: 10.1002/eap.1965
Geng, Y., Han, W., Yu, C., Jiang, Q., Wu, J., Chang, J., et al. (2017). Effect of plant diversity on phosphorus removal in hydroponic microcosms simulating floating constructed wetlands. Ecol. Eng. 107, 110–119. doi: 10.1016/j.ecoleng.2017.06.061
Hautier, Y., Isbell, F., Borer, E. T., Seabloom, E. W., Harpole, W. S., Lind, E. M., et al. (2018). Local loss and spatial homogenization of plant diversity reduce ecosystem multifunctionality. Nat. Ecol. Evol. 2, 50–56. doi: 10.1038/s41559-017-0395-0
Huang, M., Liu, X., Cadotte, M. W., and Zhou, S. (2020). Functional and phylogenetic diversity explain different components of diversity effects on biomass production. Oikos 129, 1185–1195. doi: 10.1111/oik.07032
Hussain, I., Aleti, G., Naidu, R., Puschenreiter, M., Mahmood, Q., Rahman, M. M., et al. (2018). Microbe and plant assisted-remediation of organic xenobiotics and its enhancement by genetically modified organisms and recombinant technology: a review. Sci. Total Environ. 628-629, 1582–1599. doi: 10.1016/j.scitotenv.2018.02.037
Kumar, S., Nand, S., Pratap, B., Dubey, D., and Dutta, V. (2021). Removal kinetics and treatment efficiency of heavy metals and other wastewater contaminants in a constructed wetland microcosm: does mixed macrophytic combinations perform better? J. Clean. Prod. 327:129468. doi: 10.1016/j.jclepro.2021.129468
Kumar, S., Pratap, B., Dubey, D., and Dutta, V. (2022). Interspecific competition and their impacts on the growth of macrophytes and pollutants removal within constructed wetland microcosms treating domestic wastewater. Int. J. Phytoremediation 24, 76–87. doi: 10.1080/15226514.2021.1926910
Kuznetsova, A., Brockhoff, P. B., and Christensen, R. H. (2017). lmerTest package: tests in linear mixed effects models. J. Stat. Softw. 82, 1–26. doi: 10.18637/jss.v082.i13
Leiva, A. M., Núñez, R., Gómez, G., López, D., and Vidal, G. (2018). Performance of ornamental plants in monoculture and polyculture horizontal subsurface flow constructed wetlands for treating wastewater. Ecol. Eng. 120, 116–125. doi: 10.1016/j.ecoleng.2018.05.023
Lenth, R. (2022). Emmeans: Estimated Marginal Means, Aka Least-Squares Means. R package version 1.8.1-1. Available at: https://CRAN.R-project.org/package=emmeans (Accessed September 10, 2022).
Levi, P. S., Riis, T., Alnøe, A. B., Peipoch, M., Maetzke, K., Bruus, C., et al. (2015). Macrophyte complexity controls nutrient uptake in lowland streams. Ecosystems 18, 914–931. doi: 10.1007/s10021-015-9872-y
Liang, M. Q., Zhang, C. F., Peng, C. L., Lai, Z. L., Chen, D. F., and Chen, Z. H. (2011). Plant growth, community structure, and nutrient removal in monoculture and mixed constructed wetlands. Ecol. Eng. 37, 309–316. doi: 10.1016/j.ecoleng.2010.11.018
Licata, M., Gennaro, M. C., Tuttolomondo, T., Leto, C., and La Bella, S. (2019). Research focusing on plant performance in constructed wetlands and agronomic application of treated wastewater–a set of experimental studies in Sicily (Italy). PLoS One 14:e0219445. doi: 10.1371/journal.pone.0219445
Limpert, K. E., Carnell, P. E., Trevathan-Tackett, S. M., and Macreadie, P. I. (2020). Reducing emissions from degraded floodplain wetlands. Front. Environ. Sci. 8:8. doi: 10.3389/fenvs.2020.00008
Liu, F., Sun, L., Wan, J., Shen, L., Yu, Y., Hu, L., et al. (2020). Performance of different macrophytes in the decontamination of and electricity generation from swine wastewater via an integrated constructed wetland-microbial fuel cell process. J. Environ. Sci. 89, 252–263. doi: 10.1016/j.jes.2019.08.015
Long, Y., Yi, H., Chen, S., Zhang, Z., Cui, K., Bing, Y., et al. (2016). Influences of plant type on bacterial and archaeal communities in constructed wetland treating polluted river water. Environ. Sci. Pollut. Res. 23, 19570–19579. doi: 10.1007/s11356-016-7166-3
López, D., Sepúlveda, M., and Vidal, G. (2016). Phragmites australis and Schoenoplectus californicus in constructed wetlands: development and nutrient uptake. J. Soil Sci. Plant Nutr. 16, 763–777. doi: 10.4067/s0718-95162016005000055
Ma, N., Wang, W., Gao, J., and Chen, J. (2017). Removal of cadmium in subsurface vertical flow constructed wetlands planted with Iris sibirica in the low-temperature season. Ecol. Eng. 109, 48–56. doi: 10.1016/j.ecoleng.2017.09.008
Manolaki, P., Mouridsen, M. B., Nielsen, E., Olesen, A., Jensen, S. M., Lauridsen, T. L., et al. (2020). A comparison of nutrient uptake efficiency and growth rate between different macrophyte growth forms. J. Environ. Manag. 274:111181. doi: 10.1016/j.jenvman.2020.111181
Marchand, L., Nsanganwimana, F., Lamy, J. B., Quintela-Sabaris, C., Gonnelli, C., Colzi, I., et al. (2014). Root biomass production in populations of six rooted macrophytes in response to cu exposure: intra-specific variability versus constitutive-like tolerance. Environ. Pollut. 193, 205–215. doi: 10.1016/j.envpol.2014.07.001
Marín-Muñiz, J. L., Hernández, M. E., Gallegos-Pérez, M. P., and Amaya-Tejeda, S. I. (2020). Plant growth and pollutant removal from wastewater in domiciliary constructed wetland microcosms with monoculture and polyculture of tropical ornamental plants. Ecol. Eng. 147:105658. doi: 10.1016/j.ecoleng.2019.105658
Miao, L., Wang, Y., Gao, Y., and Ji, M. (2012). The allelopathy of aquatic rhizome and root extract of Thalia dealbata to seedling of several aquatic plants. Acta Ecol. Sin. 32, 4488–4495. doi: 10.5846/stxb201107151053
Monokrousos, N., Papatheodorou, E. M., Orfanoudakis, M., Jones, D. G., Scullion, J., and Stamou, G. P. (2020). The effects of plant type, AMF inoculation and water regime on rhizosphere microbial communities. Eur. J. Soil Sci. 71, 265–278. doi: 10.1111/ejss.12882
Paranychianakis, N. V., Tsiknia, M., and Kalogerakis, N. (2016). Pathways regulating the removal of nitrogen in planted and unplanted subsurface flow constructed wetlands. Water Res. 102, 321–329. doi: 10.1016/j.watres.2016.06.048
Petchey, O. L., and Gaston, K. J. (2002). Functional diversity (FD), species richness and community composition. Ecol. Lett. 5, 402–411. doi: 10.1046/j.1461-0248.2002.00339.x
Poos, M. S., Walker, S. C., and Jackson, D. A. (2009). Functional-diversity indices can be driven by methodological choices and species richness. Ecology 90, 341–347. doi: 10.1890/08-1638.1
R Core Team. (2020). R: A Language and Environment for Statistical Computing. R Foundation for Statistical Computing, Vienna, Austria.
Rehman, F., Pervez, A., Khattak, B. N., and Ahmad, R. (2017). Constructed wetlands: perspectives of the oxygen released in the rhizosphere of macrophytes. Clean (Weinh) 45, 1600054.1–1600054.9. doi: 10.1002/clen.201600054
Revelle, W. (2018). Psych: Procedures for Personality and Psychological Research. Northwestern University, Evanston, Illinois, USA.
Rodriguez, M., and Brisson, J. (2016). Does the combination of two plant species improve removal efficiency in treatment wetlands? Ecol. Eng. 91, 302–309. doi: 10.1016/j.ecoleng.2016.02.047
Russo, N., Marzo, A., Randazzo, C., Caggia, C., Toscano, A., and Cirelli, G. L. (2019). Constructed wetlands combined with disinfection systems for removal of urban wastewater contaminants. Sci. Total Environ. 656, 558–566. doi: 10.1016/j.scitotenv.2018.11.417
Samal, K., Dash, R. R., and Bhunia, P. (2017). Treatment of wastewater by vermifiltration integrated with macrophyte filter: a review. J. Environ. Chem. Eng. 5, 2274–2289. doi: 10.1016/j.jece.2017.04.026
Sandoval, L., Zamora-Castro, S. A., Vidal-Álvarez, M., and Marín-Muñiz, J. L. (2019). Role of wetland plants and use of ornamental flowering plants in constructed wetlands for wastewater treatment: a review. Appl. Sci. 9:685. doi: 10.3390/app9040685
Schultz, R. E., Bouchard, V. L., and Frey, S. D. (2012). Overyielding and the role of complementary use of nitrogen in wetland plant communities. Aquat. Bot. 97, 1–9. doi: 10.1016/j.aquabot.2011.10.002
Steudel, B., Hallmann, C., Lorenz, M., Abrahamczyk, S., Prinz, K., Herrfurth, C., et al. (2016). Contrasting biodiversity–ecosystem functioning relationships in phylogenetic and functional diversity. New Phytol. 212, 409–420. doi: 10.1111/nph.R14054
Tanner, C. C., Craggs, R. J., Sukias, J. P. S., and Park, J. B. K. (2005). Comparison of maturation ponds and constructed wetlands as the final stage of an advanced pond system. Water Sci. Technol. 51, 307–314. doi: 10.2166/wst.2005.0489
Teubner, K., Teubner, I. E., Pall, K., Tolotti, M., Kabas, W., Drexler, S. S., et al. (2022). Macrophyte habitat architecture and benthic-pelagic coupling: photic habitat demand of macrophytes to build up large P storage capacity and bio-surface. Front. Environ. Sci. 10:901924. doi: 10.3389/fenvs.2022.901924
Villar-Navarro, E., Baena-Nogueras, R. M., Paniw, M., Perales, J. A., and Lara-Martín, P. A. (2018). Removal of pharmaceuticals in urban wastewater: high rate algae pond (HRAP) based technologies as an alternative to activated sludge based processes. Water Res. 139, 19–29. doi: 10.1016/j.watres.2018.03.072
Wang, H., Chen, Z. X., Zhang, X. Y., Zhu, S. X., Ge, Y., Chang, S. X., et al. (2013). Plant species richness increased belowground plant biomass and substrate nitrogen removal in a constructed wetland. Clean (Weinh) 41, 657–664. doi: 10.1002/clen.201200348
Wang, G. H., Fang, J. Y., Guo, K., Xie, Z. Q., Tang, Z. Y., Shen, Z. H., et al. (2020). Contents and protocols for the classification and description of vegetation formations, alliances and associations of vegetation of China. Chin. J. Plant Ecol. 44, 128–178. doi: 10.17521/cjpe.2019.0272
Webb, J. M., Quintã, R., Papadimitriou, S., Norman, L., Rigby, M., Thomas, D. N., et al. (2013). The effect of halophyte planting density on the efficiency of constructed wetlands for the treatment of wastewater from marine aquaculture. Ecol. Eng. 61, 145–153. doi: 10.1016/j.ecoleng.2013.09.058
Wu, Y., He, T., Chen, C., Fang, X., Wei, D., Yang, J., et al. (2019). Impacting microbial communities and absorbing pollutants by Canna indica and Cyperus Alternifolius in a full-scale constructed wetland system. Int. J. Environ. Res. Public Health 16:802. doi: 10.3390/ijerph16050802
Wurtsbaugh, W. A., Paerl, H. W., and Dodds, W. K. (2019). Nutrients, eutrophication and harmful algal blooms along the freshwater to marine continuum. WIREs. Water. 6:e1373. doi: 10.1002/wat2.1373
Yang, Q., Chen, Z. H., Zhao, J. G., and Gu, B. H. (2007). Contaminant removal of domestic wastewater by constructed wetlands: effects of plant species. J. Integr. Plant Biol. 49, 437–446. doi: 10.1111/j.1744-7909.2007.00389.x
Zhang, C. B., Wang, J., Liu, W. L., Zhu, S. X., Liu, D., Chang, S. X., et al. (2010). Effects of plant diversity on nutrient retention and enzyme activities in a full-scale constructed wetland. Bioresour. Technol. 101, 1686–1692. doi: 10.1016/j.biortech.2009.10.001
Zhang, R., Wang, Z., Niu, S., Tian, D., Wu, Q., Gao, X., et al. (2021). Diversity of plant and soil microbes mediates the response of ecosystem multifunctionality to grazing disturbance. Sci. Total Environ. 776:145730. doi: 10.1016/j.scitotenv.2021.145730
Zhao, Z., Song, X., Wang, Y., Wang, D., Wang, S., He, Y., et al. (2016). Effects of algal ponds on vertical flow constructed wetlands under different sewage application techniques. Ecol. Eng. 93, 120–128. doi: 10.1016/j.ecoleng.2016.05.033
Zhu, S., Huang, X., Ho, S. H., Wang, L., and Yang, J. (2017). Effect of plant species compositions on performance of lab-scale constructed wetland through investigating photosynthesis and microbial communities. Bioresour. Technol. 229, 196–203. doi: 10.1016/j.biortech.2017.01.023
Keywords: growth type, nitrogen, phosphorus, plant richness, sewage treatment, urban wetlands
Citation: Luo Y, Chen Q, Liu F and Dai C (2023) Both species richness and growth forms affect nutrient removal in constructed wetlands: A mesocosm experiment. Front. Ecol. Evol. 11:1139053. doi: 10.3389/fevo.2023.1139053
Received: 06 January 2023; Accepted: 15 February 2023;
Published: 03 March 2023.
Edited by:
Kaixiong Xing, Hainan Normal University, ChinaCopyright © 2023 Luo, Chen, Liu and Dai. This is an open-access article distributed under the terms of the Creative Commons Attribution License (CC BY). The use, distribution or reproduction in other forums is permitted, provided the original author(s) and the copyright owner(s) are credited and that the original publication in this journal is cited, in accordance with accepted academic practice. No use, distribution or reproduction is permitted which does not comply with these terms.
*Correspondence: Can Dai, ZGFpY2FuQGh1YnUuZWR1LmNu
Disclaimer: All claims expressed in this article are solely those of the authors and do not necessarily represent those of their affiliated organizations, or those of the publisher, the editors and the reviewers. Any product that may be evaluated in this article or claim that may be made by its manufacturer is not guaranteed or endorsed by the publisher.
Research integrity at Frontiers
Learn more about the work of our research integrity team to safeguard the quality of each article we publish.