- 1Southeast Asia Biodiversity Research Institute, Chinese Academy of Sciences & Center for Integrative Conservation, Xishuangbanna Tropical Botanical Garden, Chinese Academy of Sciences, Mengla, Yunnan, China
- 2University of Chinese Academy of Sciences, Beijing, China
- 3Yunnan International Joint Laboratory of Southeast Asia Biodiversity Conservation, Menglun, Yunnan, China
- 4Department of Health and Environmental Science, Xi’an Jiaotong-Liverpool University, Suzhou, Jiangsu, China
Research on the coexistence of congeneric species is essential for understanding community assemblages. Smaller competitors are expected to avoid larger ones, either spatially or temporally, to reduce interspecific competition. According to the spatial scaling law, the greater the difference in body size, the weaker the competitive interactions of the competitors. However, this is not confirmed in the guild of tropical forest ungulates. In this study, we assessed the competitive interactions of Williamson’s mouse deer (Tragulus williamsoni), an endangered species and one of the smallest ungulates in the world, with sympatric larger ungulates. We hypothesized that: 1) because of its extremely small body size, competition with the larger ungulates would be relatively weak, allowing spatial co-existence but still requiring temporal avoidance, and 2) the strength of avoidance would increases with decreasing differences in body size. We set up 238 camera traps from January 2017 to January 2021 to survey Williamson’s mouse deer and the sympatric larger ungulate species, that is, northern red muntjac (Muntiacus vaginalis), wild boar (Sus scrofa), Chinese serow (Capricornis milneedwardsii), and sambar (Rusa unicolor), in the protected areas of Mengla County, southwestern China. We then performed spatio-temporal analyses, including occupancy models, daily activity patterns, and a time interval analysis. Spatially, there was no significant avoidance. Temporally, Williamson’s mouse deer had different daily activity patterns and direct temporal avoidance of all larger ungulate species. The lack of spatial avoidance and strong temporal avoidance supported our first hypothesis, but the stronger avoidance of much larger species ran counter to our second hypothesis. Our results revealed the coexistence mechanism between Williamson’s mouse deer and sympatric larger ungulates and suggested that the difference in body size is limited in explaining the competitive interactions of tropical forest ungulates due to the effects of multiple ecological processes. This deepens our understanding of the relationship between species trait differences and community assembly in tropical forest ecosystems.
Introduction
Research on the mechanisms of species coexistence is crucial in community ecology and fundamental for understanding the maintenance of biodiversity. Species coexistence refers to the state of two or more species in the same place at the same time (Holt, 2013), which is accompanied by various interspecific interactions, such as competition, predation, parasitism, and mutualism (Hellmann, 2013). Interspecific competition is the main diver of the coexistence of sympatric species, especially congeneric species (MacArthur and Levins, 1967; Chesson, 2000); close competitors attempt to monopolize limited resources and thereby reduce the fitness of each other (Farris et al., 2020). According to the competitive exclusion principle, that is, sympatric species with completely the same niche cannot coexist, interspecific competition forces sympatric species to differentiate their ecological and evolutionary processes, thus shifting their fundamental niches to realized niches and achieving a steady state of coexistence. The degree of overlap among realized niches is usually considered a key indicator of the strength of interspecific competition (Wissinger, 1992; Wandrag et al., 2019).
For animal guilds, niche theory emphasizes that resources, space, and time are the three major niche axes partitioned by competitors (Schoener, 1974). Sympatric species should either reduce the overlap of resources to alleviate exploitation competition or reduce the likelihood of encounters through spatial and temporal avoidance to alleviate direct interference competition (Case and Gilpin, 1974). First, the differentiation of resource use is fundamental for alleviating interspecific competition since resource overlap is the prerequisite for the formation of competition (Chesson, 2000). This implies that sympatric species can achieve coexistence by exploiting different resources at the same sites and at the same time. Second, sympatric species can achieve coexistence by avoiding co-occupying sites with each other (Karanth et al., 2017), which implies that they can exploit the same resources at the same time in different sites, thus resulting in a “checkerboard” distribution pattern (Stone and Roberts, 1990). Additionally, sympatric species with low competitive strength can avoid using sites with abundant competitors (Pimm et al., 1985). Third, sympatric species may achieve coexistence through variation in their daily activity patterns (reducing activity overlap and staggering activity peaks) (Kronfeld-Schor and Dayan, 2003), which implies that they can exploit the same resources in the same sites at different time periods. However, the daily activity patterns of sympatric species, especially congeneric species, usually cannot be completely separated due to widely shared physiological rhythms (Pilorz et al., 2018), which may result in a high likelihood of encounters between them. Finally, a way to reduce the likelihood of an encounter is to avoid each other temporally by delaying using sites that were just used by another species (Niedballa et al., 2019). This is because longstanding interactions will promote the evolution of the capacity to identify the occurrence of competitors through a range of sensory modes.
Interspecific competition is usually asymmetric in that larger species are generally expected to be superior competitors due to their stronger ability to monopolize resources (Morin and Johnson, 1988), leading to them excluding smaller species spatially and temporally (e.g., interference competition) (Karanth et al., 2017). Body size is also considered an important factor affecting the competitive strength of sympatric species (Leyequién et al., 2007) because it is associated with many ecological traits and thus can summarize the difference in niche characteristics among species (Wilson, 1975). Fundamentally, different-sized species have dissimilar resource requirements and foraging strategies (Dickman, 1988). Large species usually consume large-sized and lower-quality food types, while small species specialize in consuming small-sized and higher-quality food types (Cromsig and Olff, 2006). Therefore, theoretically, the dissimilarity of body size between competitors is negatively associated with the degree of competitive strength (Leyequién et al., 2007). Furthermore, the difference in body size may be the potentially unifying first principle to explain community assembly, according to “spatial scaling laws” (Ritchie and Olff, 1999). In this case, competitors may coexist if the mean body length and body mass ratios are greater than 1.3 and 2.0, respectively (Hutchinson, 1959; Bowers and Brown, 1982). This means that the larger species will not competitively exclude the smaller species if their body sizes are extremely different (i.e., exceed the ratios), thus achieving steady coexistence at the shared sites (Roughgarden, 1983), although temporal avoidance may continue to occur (Roth, 1981).
Based on the above theoretical background, we conducted the first spatio-temporal analysis focused on the coexistence between larger ungulates and the Williamson’s mouse deer (Tragulus williamsoni), an endangered species and one of the smallest deer species in the world. Williamson’s mouse deer has thus far only been recorded in northern Thailand (Phrae Province) and southwestern China (Yunnan Province), and it might be one of the least-known mammal species in the world (Meijaard et al., 2017). In Yunnan, this species is only distributed in the transboundary area between China and Laos in Mengla County, with few records and little information about its ecology. Although it has been identified as an endangered species and a national first-class protected animal species in China, research on this species is scarce (Luo et al., 1999; Cao et al., 2010). Its breeding ecology, daily activity patterns, movements, home range, social organization and interactions with other species are still unknown (Meijaard, 2011). There are four larger ungulate species that are also distributed in Mengla County, starting with the species closest in size, although all are much larger than Williamson’s mouse deer: northern red muntjac (Muntiacus vaginalis), wild boar (Sus scrofa), Chinese serow (Capricornis milneedwardsii), and the largest species, sambar (Rusa unicolor) (Table 1). We generally hypothesized that Williamson’s mouse deer would display either spatial or temporal avoidance of these species, with the intensity of avoidance correlated to its size-difference with the other species.
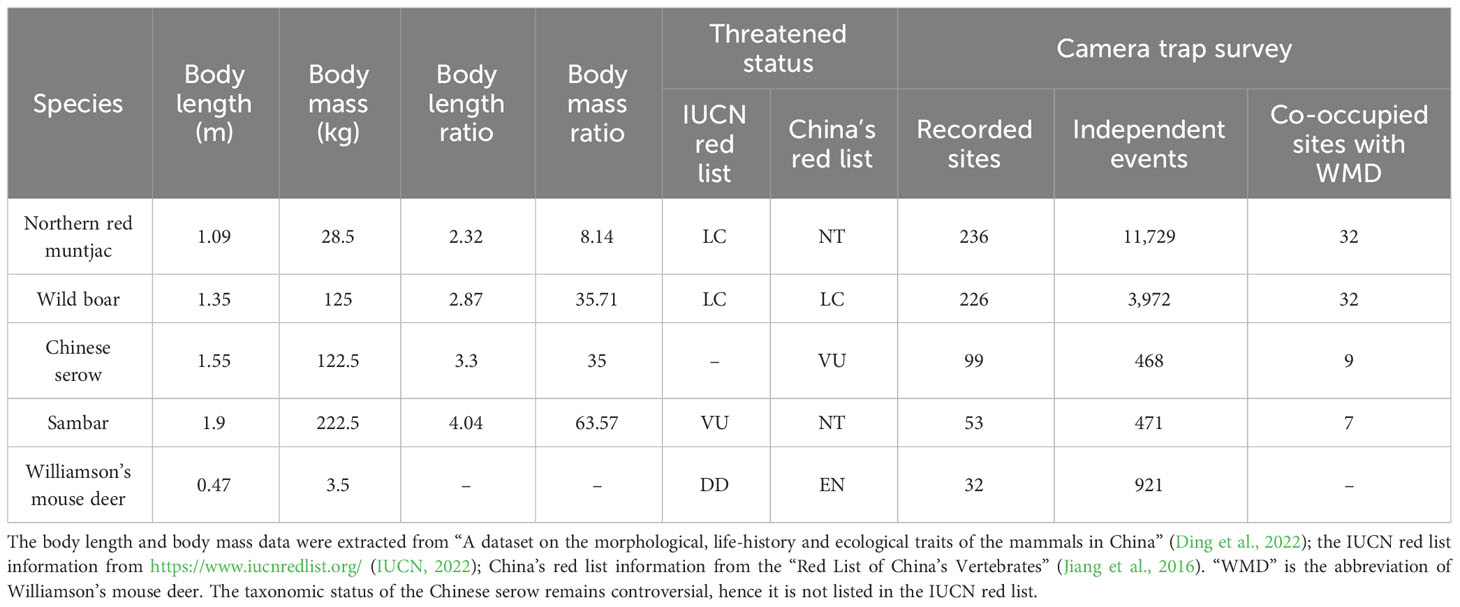
Table 1 Body size, body mass, threatened status and summary of the camera trap survey (n = 238) of the five ungulate species.
We carried out a field survey in three protected areas in Mengla County, using noninvasive camera traps, and then implemented spatial-temporal analyses. Spatially, we performed single-species and two-species occupancy models to examine the spatial avoidance of Williamson’s mouse deer to the larger ungulates, that is, the association of Williamson’s mouse deer’s occupancy with the relative abundance and presence of larger ungulates. Temporally, we conducted a daily activity pattern and a time interval analysis to examine the temporal avoidance of Williamson’s mouse deer to the larger ungulates. We specifically hypothesized that:
1. 1. Temporal avoidance plays a more important role than spatial avoidance for Williamson’s mouse deer to alleviate interspecific competition with larger ungulates, as their competition with other species is not very intense because of their extremely small body size.
2. 2. The strength of avoidance increases with decreasing difference in body size (avoidance of northern red muntjac > wild boar > Chinese serow > sambar).
Materials and methods
Study area
This study was conducted at the Mengla and Shangyong subreserves of the Xishuangbanna National Nature Reserve and Yiwu Prefectural Nature Reserve in Mengla County, Xishuangbanna Dai Autonomous Prefecture, Yunnan Province, southwestern China (21°08′~22°25′ N, 100°50′~101°06′ E). This region borders Laos and harbors rich biodiversity and provides important habitats for some key protected animal species in China, such as Indochinese tiger (Panthera tigris corbetti), Indochinese leopard (Panthera pardus delacouri), Asian elephant (Elephas maximus), clouded leopard (Neofelis nebulosa), Owston’s civet (Chrotogale owstoni), as well as Williamson’s mouse deer, yet anthropogenic disturbance is severe. Large amounts of tropical forests in this region have been displaced by cultivation (i.e., croplands and rubber plantations) and urbanization (e.g., cities, towns, villages, roads) since the 1950s (Zhang et al., 2019), which potentially affects the habitat use of Williamson’s mouse deer and other ungulate species in this region.
Camera trap survey
We used camera traps to monitor the ungulate species in the three protected areas (Mengla, Shangyong, and Yiwu) in Mengla County from January 2017 to January 2021 (Mengla: from January 2017 to June 2020; Shangyong: from November 2018 to January 2021; Yiwu: from July 2017 to October 2020). A total of 238 camera-trapping sites were set up along transects (Figure 1). The transects primarily followed abandoned human or mammal trails, with the inclusion of sampling sites from the core to the edge of each of the protected areas. To ensure sampling independence, the minimum distance between any two adjacent camera trap sites that were concurrently operating was 500 m. Camera traps (Loreda L710, Yi’an Weishi Technology Co, Ltd, Shenzhen) were mounted at 0.5–2 m aboveground on the trunk of trees without baits or lures. The monitoring system of the camera traps was set to work 24 hours per day with high sensitivity, and three photographs were taken at each trigger without a delay. To reduce the disturbance of the observers, the batteries and SD cards for storing animal photographs were changed only every six months. After we collected the camera photographs, we recorded for each photograph the GPS location, species names (including livestock and humans), capture dates, and capture times within the day.
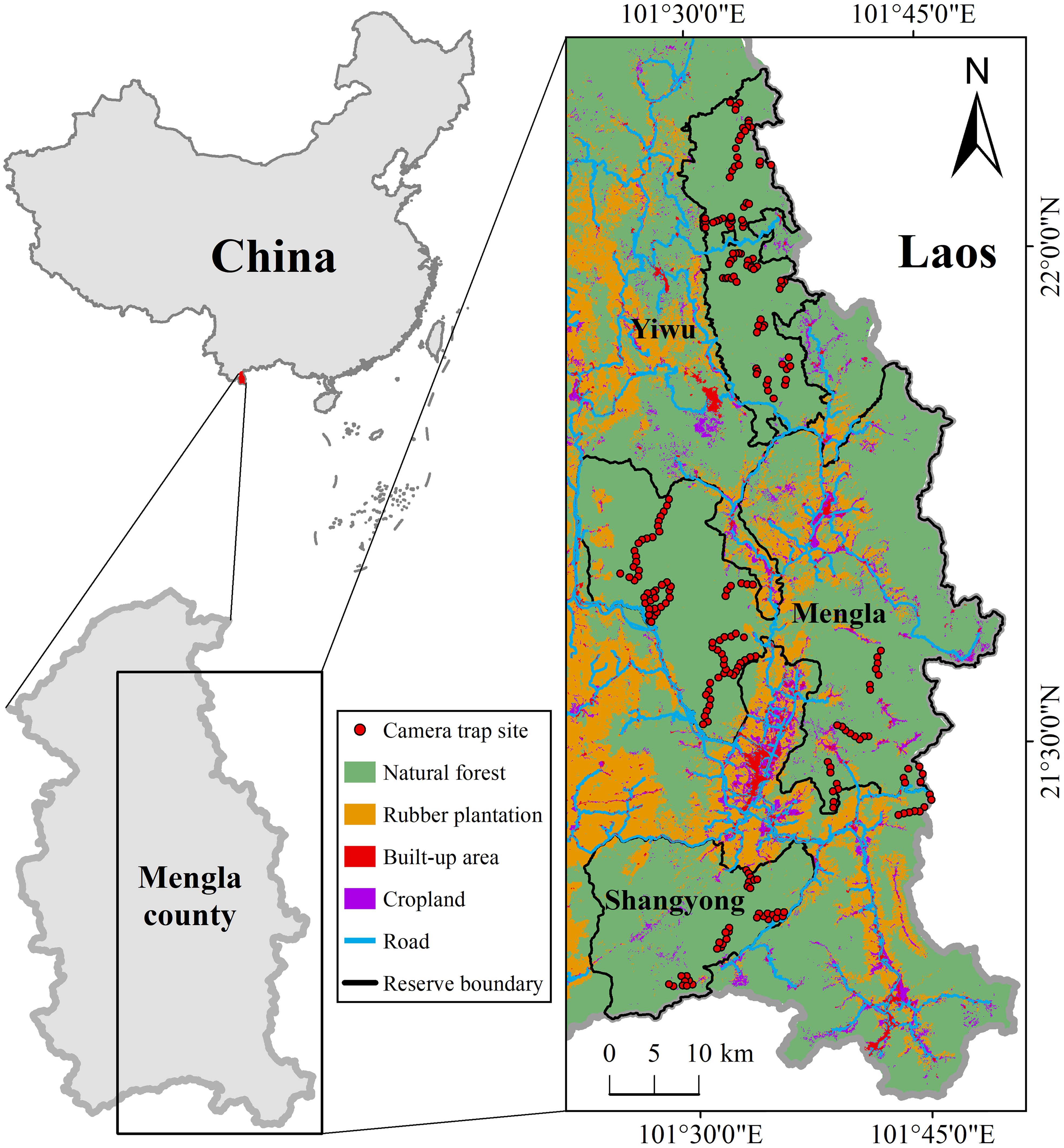
Figure 1 Illustration of the study area and camera trap sites. The land cover map was provided by the Animal Behavior and Changing Environment Research Group, Xishuangbanna Tropical Botanical Garden, Chinese Academy of Science.
Additional environmental covariates
We used the relative abundance index (hereafter RAI) of the larger ungulates (RAI of northern red muntjac, RAI of wild boar, RAI of Chinese serow, RAI of sambar and RAI of all ungulates) as the major variables. Moreover, to eliminate the false signal of spatial interaction between Williamson’s mouse deer and the sympatric larger ungulates (Pollock et al., 2014; D’Amen et al., 2018), we added additional environmental covariates that can potentially affect the occupancy of Williamson’s mouse deer into the analyses. A total of 12 site-level covariates were selected (see more detailed description and data resources in Table S1), including two covariates that could reflect habitat quality (tree density and normalized difference vegetation index, NDVI), four covariates that could reflect topographical variation (elevation, slope, aspect and topographic position index, TPI), and six covariates that represent different types of anthropogenic disturbances (distance to nearest road, distance to nearest built-up area, distance to nearest cropland, distance to nearest plantation land, RAI of local people presence, and RAI of livestock presence. We also added site-level annual average temperature to explain the variation in detection probability because temperature has a potential effect on the detection capability of camera traps, and is often considered to influence detectability (Wang et al., 2018; Wang et al., 2021). All covariates were standardized with a mean of 0 and a standard deviation of 1.
Statistical analysis
Consecutive photographs of a given species at the same camera site within 30 minutes were not considered independent detections to avoid overestimating true occurrences of animals (O’Brien et al., 2003). The RAI of each species in each camera site was denoted by the number of independent detections recorded within 100 trapping days (Jenks et al., 2011), as it is an efficient index to represent the abundance of forest ungulates (Rovero and Marshall, 2009). Matrixes of detection history with 30 days as a sample segment for analysis were created for each species using the camtrapR package (Niedballa et al., 2016) for occupancy modeling. All analyses were conducted with R software 4.1.0 (R Core Team, 2022).
To examine how the relative abundance of sympatric larger ungulates affects the occupancy of Williamson’s mouse deer, single-species occupancy models were implemented by using the occu function in the unmarked package (Fiske and Chandler, 2011). Five full models were established with annual average temperature as the detection variable, and then differing occupancy variables: 12 covariates, 12 covariates + RAI of northern red muntjac, 12 covariates + RAI of wild boar, 12 covariates + RAI of Chinese serow and 12 covariates + RAI of sambar. All variance inflation factor (VIF) values of the occupancy variables were under 5 in the full models, as measured by the vif function, which indicates little collinearity (Akinwande et al., 2015). After the collinearity check of each full model, the candidate models were established with all possible combinations of the occupancy variables and then compared based on the Akaike information criterion (AIC) (Burnham and Anderson, 2002). Models with a ΔAIC < 2 from the top model (the model with the lowest AIC) were selected for weighted model average calculation by using the model.avg function in the AICcmodavg package (Mazerolle, 2020). The full averaged coefficients and 95% confidence intervals were finally reported. The variables were considered significant if their 95% confidence intervals did not cross 0 and the full averaged P value was < 0.05. We also modeled the four ungulate species separately with the same model selection procedure (only with the 12 covariates) in order to provide the precondition of establishment for the following two-species occupancy models (see below). For model validation, we ran goodness-of-fit tests, that is, calculating the Sum of Squared Errors, Freeman-Tukey Chi-squared test, and Pearson’s Chi-squared test with 1,000 bootstraps for the top model, using the parboot function (Fiske and Chandler, 2011). All P-values of goodness-of-fit tests were greater than 0.05, which supports the goodness of fit for our occupancy modeling (Table S2).
We further used conditional two-species occupancy models to determine the spatial interaction between Williamson’s mouse deer and the larger ungulate species (Richmond et al., 2010). This model categorizes the species pair as a dominant competitor A and a subordinate competitor B, and allows the estimation of three occupancy parameters, ψA, ψBA, and ψBa, and 5 detection parameters, pA, rA, pB, rBA, and rBa (see the details in Richmond et al., 2010). The species interaction factor (SIF) can be calculated by the three occupancy parameters, as follows:
SIF = 1 suggests that the species pair is spatially independent, SIF > 1 suggests that species B is more likely to co-occur with species A than expected under the hypothesis of independence (i.e., spatial aggregation), and SIF < 1 suggests that species B is less likely to co-occur with species A than expected under the hypothesis of independence (i.e., spatial avoidance). We modeled the species pairs composed of Williamson’s mouse deer and each of the sympatric ungulate species. We considered the larger ungulates as the dominant species (species A) and Williamson’s mouse deer as the subordinate species (species B), and established four candidate models for each species pair, corresponding to four hypotheses: 1) the occupancy and detection of Williamson’s mouse deer are affected by the presence of larger ungulates, while the larger ungulates are independent from Williamson’s mouse deer (ψA, ψBA, ψBa, pA = rA, pB, rBA = rBa); 2) only the occupancy of Williamson’s mouse deer is affected by the presence of larger ungulates, while the larger ungulates are independent from Williamson’s mouse deer (ψA, ψBA, ψBa, pA = rA, pB = rBA = rBa); 3) only the detection of Williamson’s mouse deer is affected by the presence of larger ungulates, while the larger ungulates are independent from Williamson’s mouse deer (ψA, ψBA = ψBa, pA = rA, pB, rBA = rBa); and 4) Williamson’s mouse deer and the larger ungulates are independent from each other (ψA, ψBA = ψBa, pA = rA, pB = rBA = rBa). We did not hypothesize the effect of larger ungulate detection on Williamson’s mouse deer’s detection since we used a long sample segment (30 days). The detection variable, annual average temperature, and the significant occupancy variables from single-species occupancy models were added to each candidate two-species occupancy model. The candidate models with ΔAIC < 2 from the top model were selected for weighted model average calculation. The mean predicted value ± conditional 95% confidence intervals of the parameter’s coefficients were finally reported. Williamson’s mouse deer was considered significantly aggregated (or avoided) with the presence of larger ungulates if the conditional 95% confidence intervals were higher (or lower) than 1. All the two-species occupancy models were conducted by using the program PRESENCE (https://www.mbr-pwrc.usgs.gov/software/presence.html).
Nonparametric kernel density estimations were used to examine the overlap of the daily activity patterns of Williamson’s mouse deer and the sympatric larger ungulates. The captured clock time of day of independent events was transformed to circular solar time by using the solartime function in the activity package (Rowcliffe, 2022). The overlap of the activity pattern was measured by calculating the coefficient of overlap D-hat (Δ) with the overlapEst function in the overlap package (Ridout and Linkie, 2009), in which a range from 0 (no overlap) to 1 (complete overlap) was generated. Δ4 was used since the number of detections of all species was > 50. The overlap was considered high when Δ > 0.75, moderate when 0.5 < Δ < 0.75, and low when Δ < 0.5 (Monterroso et al., 2014). We also performed randomization tests by using the compareCkern function to test the significant difference in the daily activity patterns of Williamson’s mouse deer and the larger ungulates. We repeated the analysis for Williamson’s mouse deer with the different species of larger ungulates.
We performed time interval analyses to understand whether Williamson’s mouse deer displays direct temporal avoidance of the sympatric larger ungulates in their co-occupied sites. Two types of time intervals were calculated at each camera trap site: 1) the time interval from the detection of a given ungulate species to the next detection of Williamson’s mouse deer, and 2) the time interval between two successive detections of Williamson’s mouse deer (Figure 2). Because of nonnormality, one-tailed Wilcoxon rank sum tests were performed to determine whether the time intervals of a given ungulate species to the next detection of Williamson’s mouse deer were significantly longer than those of two successive detections of Williamson’s mouse deer. A significant test result suggests that interspecific temporal avoidance exceeded temporal avoidance between conspecifics (Harmsen et al., 2009; de Oliveira et al., 2020), which would imply that Williamson’s mouse deer does show direct temporal avoidance of the given ungulate species. We repeated these analysis procedures for Williamson’s mouse deer with the different larger ungulate species. We also calculated the avoidance ratios by using the time interval from the detection of a given ungulate species to the next detection of Williamson’s mouse deer divided by that between two successive detections of Williamson’s mouse deer to determine the difference in the avoidance strength of Williamson’s mouse deer to different-sized larger ungulate species.
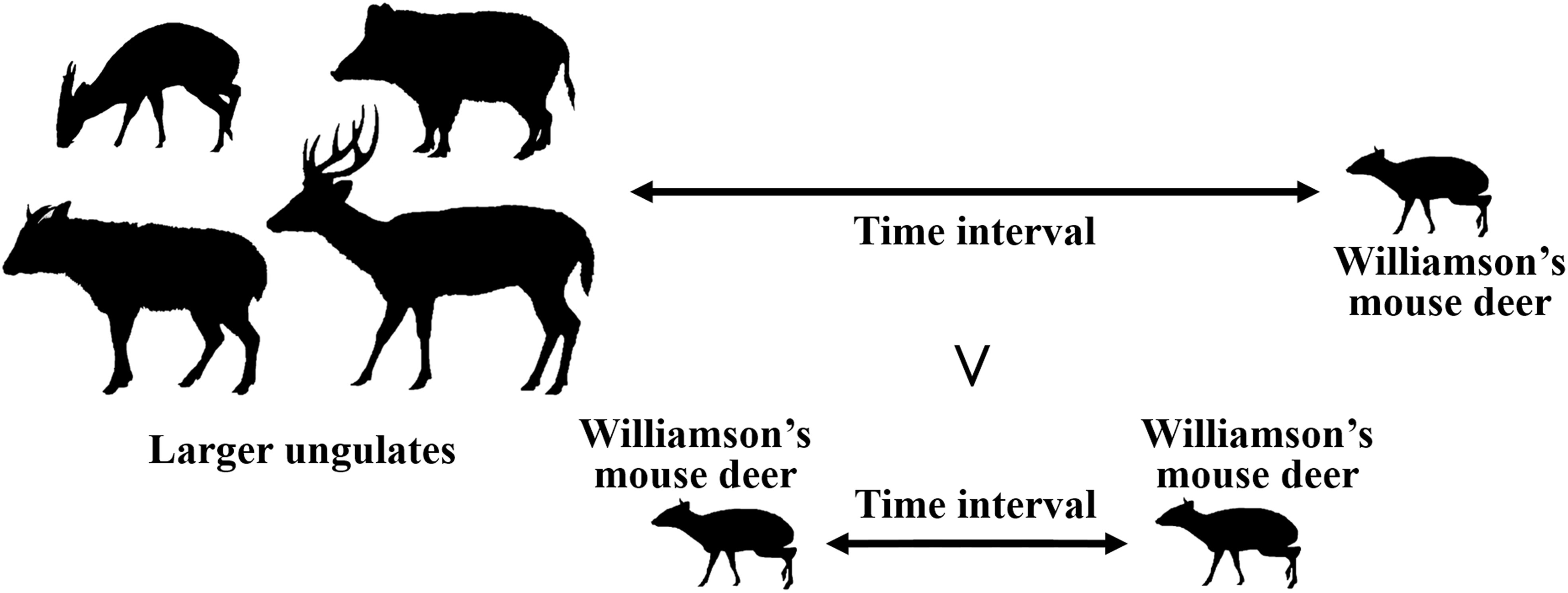
Figure 2 Illustration of direct temporal avoidance. It suggests direct temporal avoidance if the time intervals of a given ungulate species to the next Williamson’s mouse deer detection were longer than between successive Williamson’s mouse deer detections. The illustrations of ungulate species in this figure were drawn by R-CH, following illustrations in Smith and Xie (2009).
Results
From January 2017 to January 2021, a total of 105,087 camera-trapping days were obtained from 238 camera-trap sites. The range of each site’s camera-trapping days was 78–1118 (mean ± SD = 441.54 ± 248.64), and only two sites had less than 100 days (78 and 94). A total of 17,561 independent events of all five ungulate species were obtained (Table 1), of which 921 independent events of Williamson’s mouse deer were recorded at 32 camera trap sites. Of this total, 32, 32, 9 and 7 sites were co-occupied with northern red muntjac, wild boar, Chinese serow and sambar, respectively. The other species were all found at more camera trap sites than Williamson’s mouse deer (Table 1).
Occupancy interactions
The results of single-species occupancy models showed that the occupancy probability of Williamson’s mouse deer was only significantly associated with elevation, in which it was higher in the lowlands (coefficient [95% CI] = −1.26 [−1.97, −0.56], P< 0.001), when interactions with other ungulates were not included (Table S3). The relative abundance of all larger ungulate species had no significant effects on the occupancy probability of Williamson’s mouse deer (northern ren muntjac: coefficient [95% CI] = 0.4 [−0.026, 0.83], P = 0.066; wild boar: coefficient [95% CI] = 0.026 [−0.19, 0.25], P = 0.81; Chinese serow: coefficient [95% CI] = −0.5 [−1.83, 0.83], P = 0.46; sambar: coefficient [95% CI] = 0.0046 [−0.11, 0.12], P = 0.94) (Figure 3).
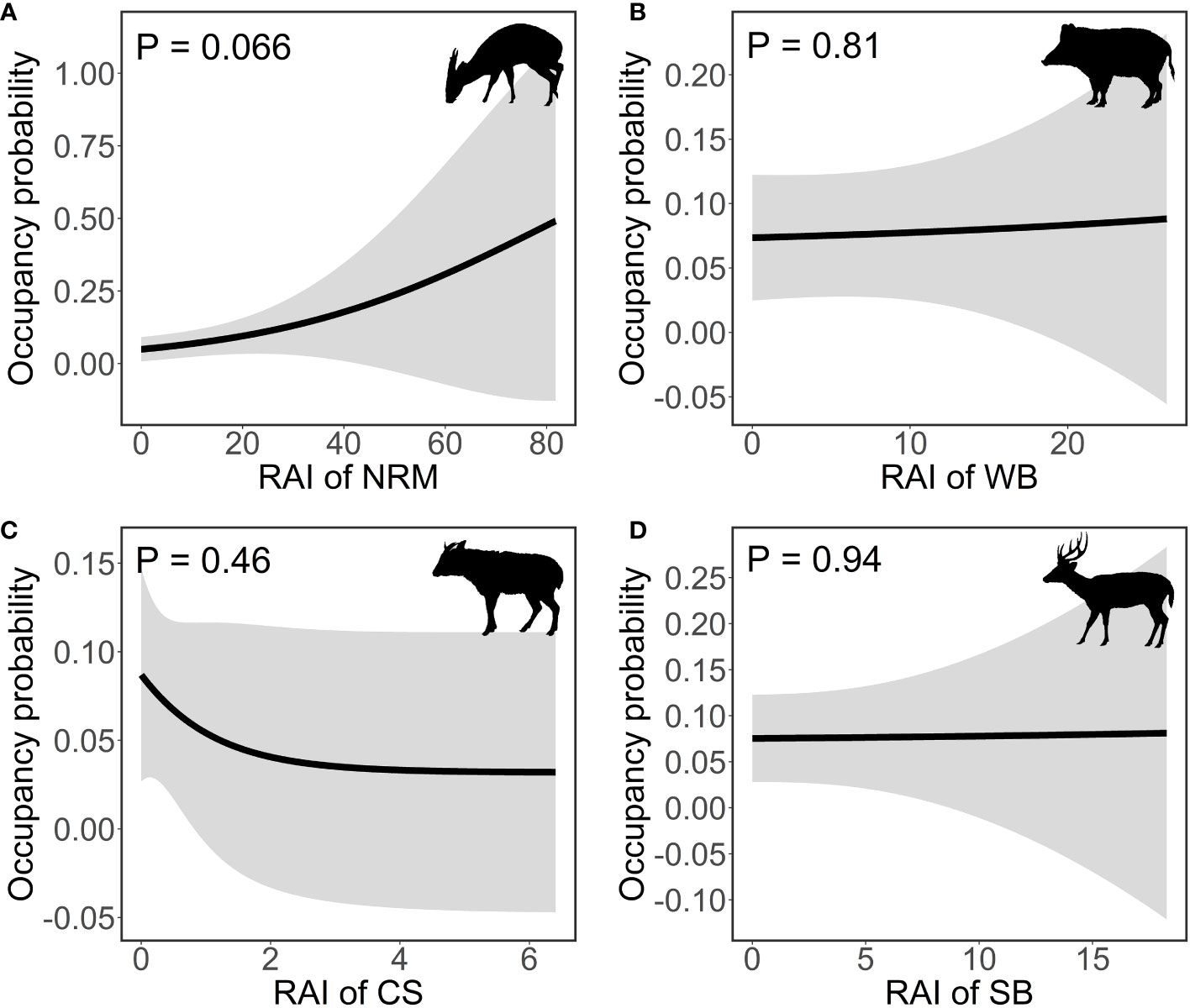
Figure 3 The association between the occupancy probability of Williamson’s mouse deer and the relative abundance of northern red muntjac (A), wild boar (B), Chinese serow (C) and sambar (D). The black lines and the grey areas are fitted lines and 95% confidence intervals, respectively. NRM, WB, CS, SB are the abbreviations of northern red muntjac, wild boar, Chinese serow and sambar, respectively. The illustrations of ungulate species in this figure were drawn by R-CH, following illustrations in Smith and Xie (2009).
We did not deploy two-species occupancy models to analyze the avoidance of Williamson’s mouse deer to the presence of northern red muntjac and wild boar. This is because its recorded sites were fully covered by those of the two larger ungulates, which suggested no avoidance of them. The averaged estimates of the two-species models showed that Williamson’s mouse deer had significant aggregation with Chinese serow (SIF [95% CI] = 1.041 [1.019, 1.064] > 1), and it was independent from the presence of sambar (SIF = 1) (Table 2).
Daily activity pattern
The daily activity pattern of Williamson’s mouse deer was significantly different from that of the sympatric larger ungulates (all P< 0.001), with moderate overlap with northern red muntjac (Δ4 = 0.63), wild boar (Δ4 = 0.66) and Chinese serow (Δ4 = 0.54) and low overlap with sambar (Δ4 = 0.44) (Figure 4). The daily activity pattern of Williamson’s mouse deer was typically crepuscular with 2 peaks at 9:00:00 and 18:00:00 and a trough at 13:00:00. The other four species of larger ungulates had varied degrees of nocturnal activity, with inapparent peaks in the morning and twilight. In particular, sambar was mainly active at night without any obvious peaks and only had an obvious trough in the afternoon.
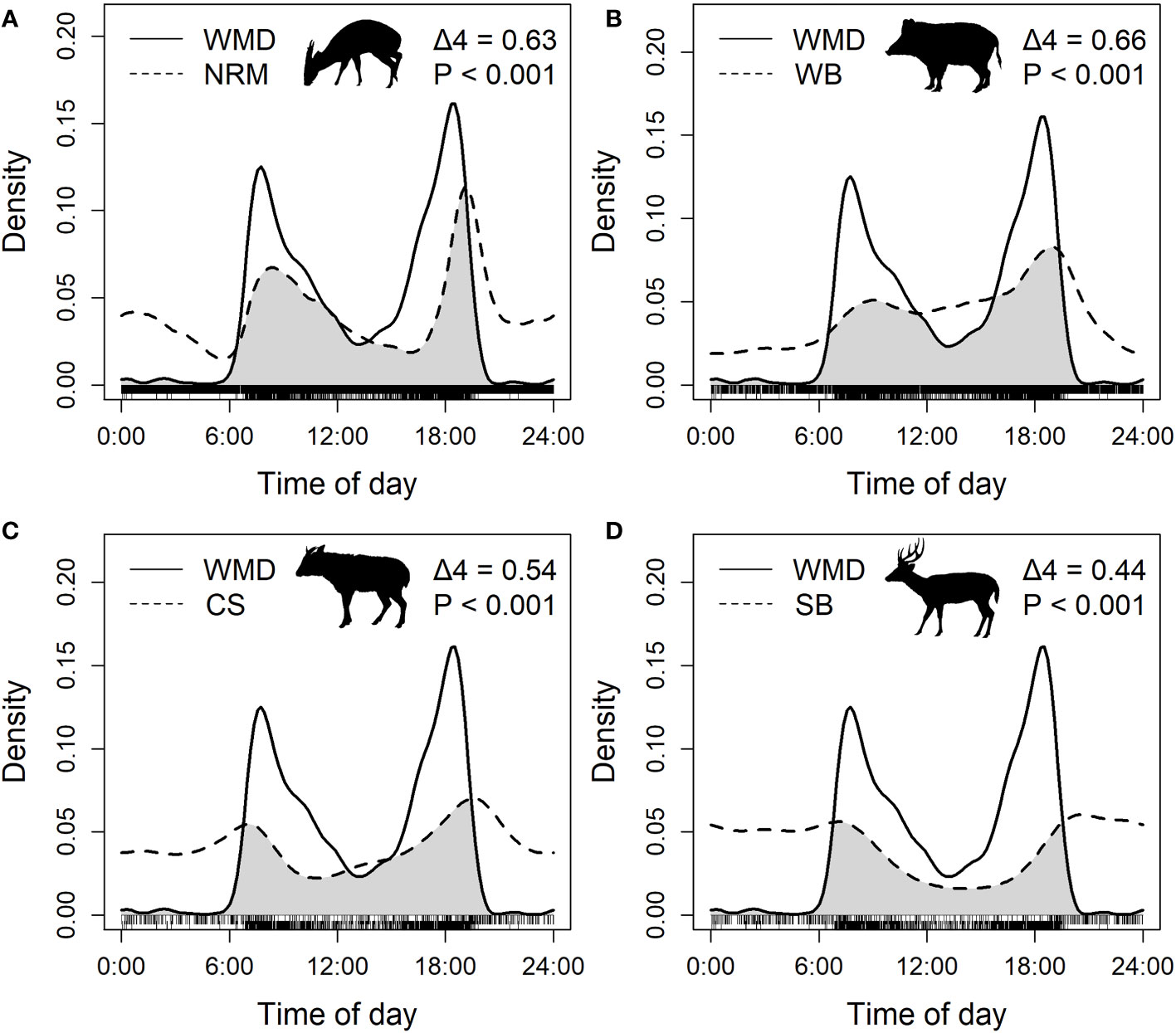
Figure 4 Overlap and difference in the daily activity pattern of Williamson’s mouse deer and northern red muntjac (A), wild boar (B), Chinese serow (C) and sambar (D). The grey areas are the overlap areas. NRM, WB, CS, SB, WMD are the abbreviations of northern red muntjac, wild boar, Chinese serow, sambar, and Williamson’s mouse deer, respectively. The illustrations of ungulate species in this figure were drawn by R-CH, following illustrations in Smith and Xie (2009).
Direct temporal avoidance
The time intervals of the detection of any larger ungulate species to the next Williamson’s mouse deer were significantly longer than those of the successive detections of Williamson’s mouse deer (all P< 0.05) (Figure 5), which means that the interspecific temporal avoidance exceeded temporal avoidance between conspecifics, suggesting that Williamson’s mouse deer do display direct temporal avoidance to these species. Moreover, the strength of avoidance to Chinese serow (avoidance ratio = 3.36) > sambar (avoidance ratio = 3.026) > northern red muntjac (avoidance ratio = 1.96) > wild boar (avoidance ratio = 1.53).
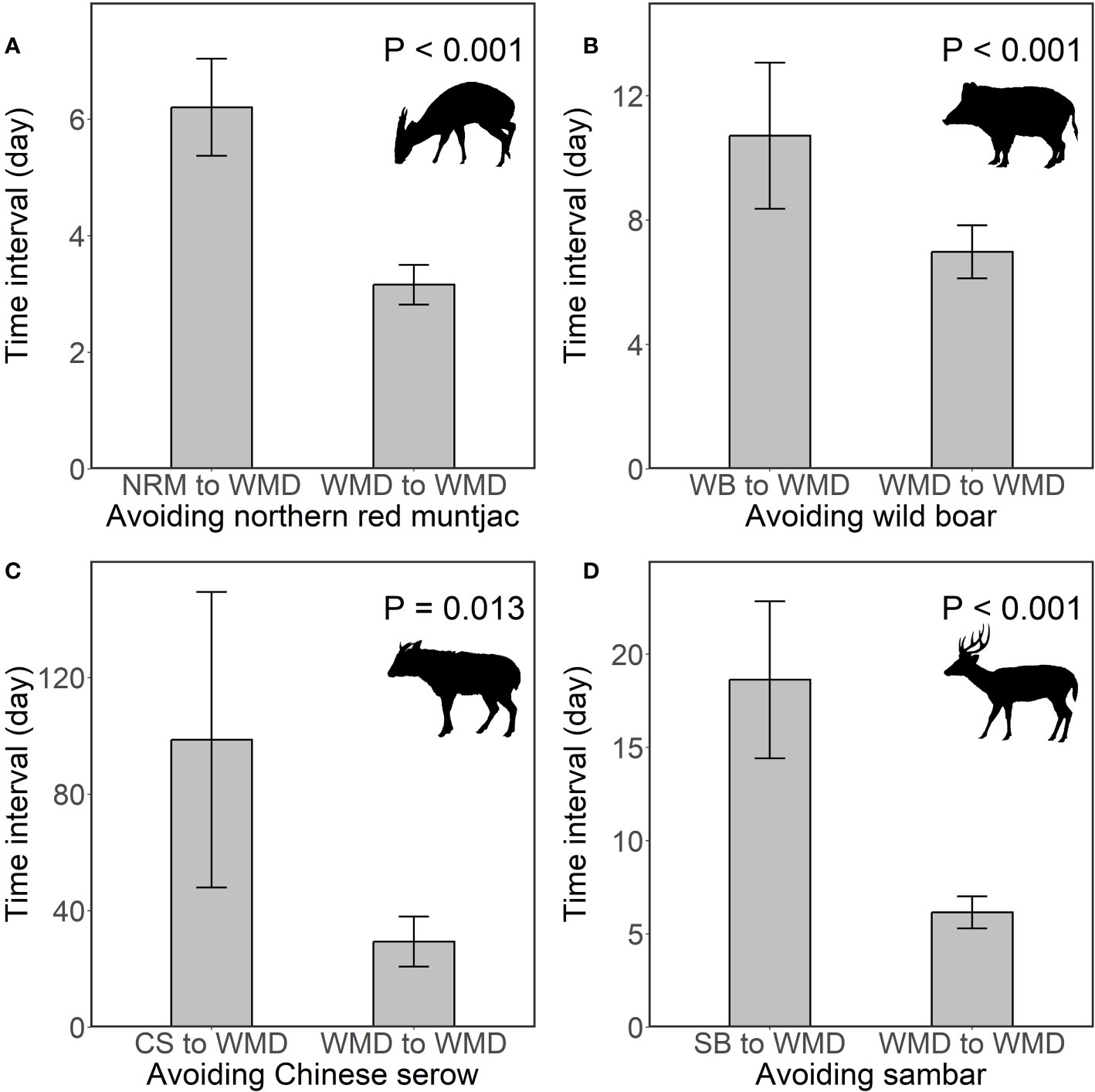
Figure 5 The direct temporal avoidance of Williamson’s mouse deer to northern red muntjac (A), wild boar (B), Chinese serow (C) and sambar (D). The bars and error bars represent mean values and standard errors, respectively. NRM, WB, CS, SB, WMD are the abbreviations of northern red muntjac, wild boar, Chinese serow, sambar, and Williamson’s mouse deer, respectively. The illustrations of ungulate species in this figure were drawn by R-CH, following illustrations in Smith and Xie (2009).
Discussion
In this study, we identified for the first time how Williamson’s mouse deer coexists with the sympatric larger ungulates, northern red muntjac, wild boar, Chinese serow, and sambar with the analyses of long-term camera-trapping data from Mengla County, southwestern China. Williamson’s mouse deer had no spatial avoidance of all larger ungulate species but avoided them temporally, which supported our first specific hypothesis that temporal avoidance plays a more important role than spatial avoidance for Williamson’s mouse to coexist with the sympatric larger ungulates, because its competition with them is relatively weak due to the extreme difference in body size. However, Williamson’s mouse deer had stronger avoidance of the much larger species, which was contrary to our second hypothesis that the strength of avoidance would increase with decreasing differences in body sizes.
No evidence of spatial avoidance
It is usually considered that spatial avoidance is more frequent and important for alleviating interspecific competition than the other two major niche axes, i.e., resource and time (Schoener, 1974). Spatial avoidance would seem to indicate such intense competition that the species are unable to be present together at the same location, whereas in other forms of competition the species are able partition the resources, or temporal access to them. However, our results showed the occupancy probability of Williamson’s mouse deer was not negatively associated with the presence and relative abundance of the larger ungulates, which indicates that Williamson’s mouse deer did not avoid the larger ungulate species spatially. This suggested that spatial avoidance may not play a major role in the coexistence of Williamson’s mouse deer with the sympatric larger ungulates. This lack of spatial avoidance could largely be explained by temporal avoidance (i.e., differing in daily activity patterns and direct temporal avoidance; see below) (Kronfeld-Schor and Dayan, 2003). In addition, the differentiation in dietary niches may be another important reason that facilitates the steady coexistence of sympatric competitors (du Preez et al., 2017).
The diet of Williamson’s mouse deer is unclear (Meijaard, 2011), but it should be much different from the larger ungulates due to the extreme difference in body size (Cromsig and Olff, 2006). From interviews of local villagers in Mengla, a previous paper described the food selection of Williamson’s mouse deer to mainly include fallen fruits of wild figs (Ficus spp.), Phyllanthus emblica and Phyllanthi fructus, and the tender leaves of Alpinia kwangsiensis and Phrynium capitatum (Cao et al., 2010). If it is similar to other species of mouse deer, such as Javan mouse deer (Tragulus javanicus) and lesser mouse deer (T. kanchil), Williamson’s mouse deer’s diet may generally consist of small fallen fruits from Ficus, shoots, tender leaves from understory plants and small mushrooms (Matsubayashi et al., 2003; Meijaard, 2011). In contrast, the diets of larger ungulates are wider and more opportunistic (Ahrestani et al., 2016). The four larger ungulate species can also consume fallen fruits and understory plants, but they are not restricted to items that are of low height and small size, as are the small deer, and can consume more species of plants or other food items. For example, sambar can browse tender leaves, twigs and large fruits of larger plants (Ahrestani et al., 2016); wild boar even frequently consume small animals, such as earthworms, snakes, frogs, small rodents, and ground-dwelling birds (Ballari and Barrios-García, 2014). A previous study in Singapore showed that the presence of wild boar and sambar had no effect on the occupancy of lesser mouse deer, which may be partially explained by different food selection (Khoo et al., 2021).
The occupied sites of Williamson’s mouse deer were all covered by northern red muntjac and wild boar, and it was statistically aggregated with the presence of Chinese serow, which suggests that Williamson’s mouse deer is positively associated with these species in its space use. It is possible that Williamson’s mouse deer could reduce predation risk by co-occurring with the important prey of larger carnivores according to the “dilution effect” hypothesis (Dehn, 1990). In particular, the northern red muntjac is much more abundant than other species in our study area, and the number of independent detection events is almost three times (2.95 times) that of the second most abundant species, the wild boar (Table 1), and muntjac may be the major prey of the Asian golden cat (Catopuma temminckii) and dhole (Cuon alpinus) (Kamler et al., 2012; Kamler et al., 2020). This may be why Williamson’s mouse deer had a near-significant positive association with the relative abundance of northern red muntjac. Additionally, although wild boar, Chinese serow and sambar may be important prey terms of the Asian golden cat, dhole and clouded leopard (Neofelis nebulosa) (Kamler et al., 2012; Kamler et al., 2020; Rasphone et al., 2022), the neutral associations with the relative abundance of wild boar, Chinese serow and sambar and the presence of sambar may be due to Williamson’s mouse deer having a trade-off between the benefits of reducing predation risk and habitat damage (reduced food availability and destruction of the refuges of Williamson’s mouse deer) by the abundant larger ungulates (Foster et al., 2014).
Temporal avoidance is more important than spatial avoidance
Although the possible dietary niche differentiation and dilution effects may be important reasons to explain the positive and neutral spatial associations between Williamson’s mouse deer and the sympatric larger ungulates, the temporal avoidance (i.e., differing in daily activity pattern and direct temporal avoidance) we assessed in this study provides an alternative way of avoiding competition from the perspective of niche theory.
Subordinate competitors commonly partition niches temporally with the dominant competitor (Schoener, 1974), and this is often found in ungulate guilds (de Pinho et al., 2022; You et al., 2022). In this study, Williamson’s mouse deer had significantly different daily activity patterns from the four sympatric larger ungulate species. The four larger ungulate species have more active times at night with inapparent peaks in the morning and twilight, especially sambar, which has primary nocturnal activities without any apparent peaks and only has a trough in the afternoon. Williamson’s mouse deer, in this region, is a typically crepuscular species, a finding which was different from the nocturnal activity pattern mentioned in a previous study in the same area that did not use camera traps (Cao et al., 2010). Similarly, another camera-trap study also reported that lesser mouse deer have a bimodal and crepuscular activity pattern (Khoo et al., 2021), which was different from the nocturnal pattern reported by earlier studies. Here the mouse deer was more active at night, which was different from the sympatric wild boar and sambar, which showed more diurnal and unimodal activities. This may suggest that Williamson’s mouse deer shift their daily activity pattern to avoid the sympatric larger ungulates. The moderate and low overlaps (< 0.7) and different activity peaks imply that Williamson’s mouse deer can use the co-occupied sites with the larger ungulates at different time periods of the day, which efficaciously reduces the likelihood of encounter, thus facilitating the coexistence between them (Kronfeld-Schor and Dayan, 2003).
Although the differentiation of daily activity patterns is an effective way to alleviate interspecific competition, the overlapping of daily activity time periods also implies that the subordinate species still have a low probability of encountering the dominant species (Kronfeld-Schor and Dayan, 2003). Our time interval analysis showed that Williamson’s mouse deer had direct temporal avoidance of the larger ungulates in the co-occupied sites. This suggested that direct temporal avoidance also helps Williamson’s mouse deer relieve competitive pressure from larger ungulates. Prolonged interactions may enable Williamson’s mouse deer to identify the tracks of larger ungulates by signs, scent and vocalizations. This avoidance strategy has rarely been found among the interactions of wild ungulates (Kavčić et al., 2021; Staudenmaier et al., 2021), although it has been found in the response of wild ungulates to livestock (Feng et al., 2021).
Stronger avoidance to much larger species
According to our second specific hypothesis, the coexistence of congeneric species with small differences in body size would prompt fiercely competitive interactions (Leyequién et al., 2007), which means that the smaller northern red muntjac should have had the strongest spatio-temporal exclusion to Williamson’s mouse deer, followed by wild boar, Chinese serow, and sambar. However, Williamson’s mouse deer had stronger avoidance of the much larger species spatially and temporally, which contradicts this hypothesis.
Spatially, Williamson’s mouse deer had positive associations with the presence of the three smaller ungulate species but had a neutral association with sambar (the largest species). Temporally, Williamson’s mouse deer had lower overlaps of daily activity patterns (wild boar [0.66] > northern red muntjac [0.63] > Chinese serow [0.54] > sambar [0.44]) and stronger direct temporal avoidance (wild boar [avoidance ratio = 1.53]< northern red muntjac [avoidance ratio = 1.96]< sambar [avoidance ratio = 3.026]< Chinese serow [avoidance ratio = 3.36]) with/of the much larger ungulate species, which was contrary to the expectation that the more similar the body sizes, the stronger the competition (Roughgarden, 1983). One possible reason is that sufficiently different body sizes represent sufficient differentiation of niches between sympatric species (Wilson, 1975), resulting in an unclear pattern between differences in body sizes and the degree of spatio-temporal competitive interactions. Previous studies pointed out that competitors can steadily coexist spatially if the body size ratio exceeds thresholds that were considered near 1.3 for body length and 2.0 for body mass (Hutchinson, 1959; Bowers and Brown, 1982). In Mengla, the sympatric larger ungulate species is much larger than Williamson’s mouse deer; for example, the body size and body mass of northern red muntjac, the next smallest ungulate species, are 2.32 and 8.14 times those of Williamson’s mouse deer, which implies that it has extremely different niches, representing distinct morphology and physiology (Helaouët and Beaugrand, 2009). Moreover, the sambar may utilize more open sites compared to Williamson’s mouse deer due to its extremely large body size, with a standing height reaching two meters from the foot to the antler. This difference in microhabitat preference may explain why Williamson’s mouse deer was positively associated with the presence of the three smaller ungulate species but neutrally associated with sambar. Another possible reason is that the strength of interspecific competition between the species may be related to multiple ecological factors instead of purely the difference in body size. Evaluation of interspecific competition focusing only on body size could miss processes such as the larger species excluding the smaller species in multiple ways (such as through habitat damage), or competitors having imperceptible symbiotic relationships (such as predator dilution effects). Consequently, we highlight that interspecific competition in this community appears to be more complicated than we expected, and the difference in body size is limited in explaining the coexistence of tropical forest ungulates.
Conservation implications
Our study also can provide the first evidence of the relationship between Williamson’s mouse deer and anthropogenic disturbance. Fortunately, none of the anthropogenic variables had negative effects on Williamson’s mouse deer (Table S3). Williamson’s mouse deer may have good adaptability in disturbed habitats, similar to common ungulate species such as muntjac and wild boar. According to studies of other species of mouse deer, these kinds of deer can occupy disturbed habitats. For example, Javan mouse deer can use dense tea plantations (Farida et al., 2006). Similarly, lesser mouse deer prefer fragmented and logged forest patches over continuous primary forests (Hazwan et al., 2022), and they can occupy fragmented urban forests and even prefer small fragments (Khoo et al., 2021).
Our study was conducted in protected areas with low levels of human disturbance. However, the potential habitats of Williamson’s mouse deer may also exist outside of protected areas, which we will be assessing in the future. Consistent with previous findings, our results showed that Williamson’s mouse deer prefer to use lowlands, and they may prefer to use sites closer to water resources (Cao et al., 2010). We thus suggest that future investigations of the population of Williamson’s mouse deer in nonprotected areas should mainly focus on lowlands closer to water. In Mengla County, the northern red muntjac and wild boar are becoming problematic to local ecosystems (e.g., damaging the local habitats and excluding the local species) due to their overabundance caused by habitat fragmentation and the declining population of larger predators (Cao and Zhang, 2022), which is hard to control and manage. However, we emphasize that these two species have no spatial exclusion from Williamson’s mouse deer. In summary, our study provides important information for understanding the threats to the population of Williamson’s mouse deer, which can guide management and conservation.
Conclusions
Based on a long-term camera trap survey, we assessed the spatio-temporal avoidance of Williamson’s mouse deer to sympatric larger ungulates and the relationship between the strength of avoidance and body size differences. Spatially, there was no spatial avoidance. Temporally, the species had different daily activity patterns and direct temporal avoidance of all larger ungulate species. This suggests that temporal avoidance can play an important role in alleviating interspecific competition with sympatric larger species. Furthermore, we found results that run contrary to the body size–competition hypothesis (the more similar body sizes, the stronger competitive interactions), because Williamson’s mouse deer had a stronger avoidance of the much larger species (sambar and Chinese serow). Our results provide the first evidence of a coexistence mechanism between Williamson’s mouse deer and sympatric larger ungulates. We highlight that the difference in body size has limited power to explain the coexistence of tropical forest ungulates, because natural competitive interactions may be related to multiple ecological processes.
Data availability statement
The raw data supporting the conclusions of this article will be made available by the authors, without undue reservation.
Ethics statement
The animal study was approved by the State Forestry Administration of China and in accordance with the legal requirements of China. The study was conducted in accordance with the local legislation and institutional requirements.
Author contributions
R-CH, LW and R-CQ designed the study. R-CH processed and analyzed the data. R-CH wrote the first draft of the manuscript. R-CH, LW, KL, EG and R-CQ revised the manuscript. All authors contributed to the article and approved the submitted version.
Funding
This research was funded by the National Natural Science Foundation of China (31872963); the Transboundary Cooperation on Biodiversity Research and Conservation in Gaoligong Mountains (E1ZK251); the Major Science and Technique Programs in Yunnan Province (202102AA310055); and the project of 14th Five-Year plan from the CAS.
Acknowledgments
We thank the offices of Xishuangbanna National Nature Reserve and Yiwu Prefectural Nature Reserve for supporting and approving this research. We also thank Xiao-Bao Deng and Guo-Gang Li for field work and Jia-Bin Li, Wei Ao, Jia-Zhou Yang, Xiao-Ying Mi, Ying Geng, Hui Cao, Ying Liu for species identification.
Conflict of interest
The authors declare that the research was conducted in the absence of any commercial or financial relationships that could be construed as a potential conflict of interest.
Publisher’s note
All claims expressed in this article are solely those of the authors and do not necessarily represent those of their affiliated organizations, or those of the publisher, the editors and the reviewers. Any product that may be evaluated in this article, or claim that may be made by its manufacturer, is not guaranteed or endorsed by the publisher.
Supplementary material
The Supplementary Material for this article can be found online at: https://www.frontiersin.org/articles/10.3389/fevo.2023.1125840/full#supplementary-material
References
Ahrestani F. S., Heitkönig I. M. A., Matsubayashi H., Prins H. H. T., Sankaran M. (2016). “Grazing and browsing by large herbivores in south and southeast Asia,” in The Ecology of Large Herbivores in South and Southeast Asia, vol. 225 . Ed. Ahrestani F. (Dordrecht: Springer), 99–120. doi: 10.1007/978-94-017-7570-0_4
Akinwande M. O., Dikko H. G., Samson A. (2015). Variance inflation factor: as a condition for the inclusion of suppressor variable(s) in regression analysis. Open J. Stat. 05, 754–767. doi: 10.4236/ojs.2015.57075
Ballari S. A., Barrios-García M. N. (2014). A review of wild boar Sus scrofa diet and factors affecting food selection in native and introduced ranges. Mamm. Rev. 44, 124–134. doi: 10.1111/mam.12015
Bowers M. A., Brown J. H. (1982). Body size and coexistence in desert rodents: chance or community structure? Ecology 63, 391–400. doi: 10.2307/1938957
Burnham K. P., Anderson D. R. (2002). Model selection and multimodel inference: a practical information-theoretic approach (New York, USA: Springer). doi: 10.1007/b97636
Cao L., Zhang Z. (2022). Loss of top predators and fragmentation lead to the decline of dominant plants in forests: a balance needed for conservation and management on overabundant large herbivore species. Integr. Zool. 17, 231–233. doi: 10.1111/1749-4877.12513
Cao M., Zhou W., Bai B., Zhang Q., Wang B., Chen M. Y. (2010). Habitat use of Williamson’s mouse-deer (Tragulus williamsoni) in Mengla area, southern Yunnan. Zool. Res. 31, 303–309. doi: 10.3724/SP.J.1141.2010.03303
Case T. J., Gilpin M. E. (1974). Interference competition and niche theory. Proc. Natl. Acad. Sci. U. S. A. 71, 3073–3077. doi: 10.1073/pnas.71.8.3073
Chesson P. (2000). Mechanisms of maintenance of species diversity. Annu. Rev. Ecol. Syst. 31, 343–366. doi: 10.1146/annurev.ecolsys.31.1.343
Cromsig J. P. G. M., Olff H. (2006). Resource partitioning among savanna grazers mediated by local heterogeneity: an experimental approach. Ecology 87, 1532–1541. doi: 10.1890/0012-9658(2006)87[1532:RPASGM]2.0.CO;2
D’Amen M., Mod H. K., Gotelli N. J., Guisan A. (2018). Disentangling biotic interactions, environmental filters, and dispersal limitation as drivers of species co-occurrence. Ecography (Cop.). 41, 1233–1244. doi: 10.1111/ecog.03148
Dehn M. M. (1990). Behavioral ecology and sociobiology vigilance for predators: detection and dilution effects. Behav. Ecol. Sociobiol. 26, 337–342. doi: 10.1007/BF00171099
de Oliveira E. S., da Fontoura Rodrigues M. L., Severo M. M., dos Santos T. G., Kasper C. B. (2020). Who’s afraid of the big bad boar? Assessing the effect of wild boar presence on the occurrence and activity patterns of other mammals. PloS One 15, e0235312. doi: 10.1371/journal.pone.0235312
de Pinho F. F., Lemos L. P., Montanarin A., El Bizri H. R., Santos J., Rabelo R. M., et al. (2022). Modelling the impact of hunting on the coexistence of congeneric deer species in Central Amazonia. J. Zool. 317, 195–204. doi: 10.1111/jzo.12970
Dickman C. R. (1988). Body size, prey size, and community structure in insectivorous mammals. Ecology 69, 569–580. doi: 10.2307/1941006
Ding C., Liang D., Xin W., Li C., Ameca E. I., Jiang Z. (2022). A dataset on the morphological, life-history and ecological traits of the mammals in China. Biodivers. Sci. 30, 1–10. doi: 10.17520/biods.2021520
du Preez B., Purdon J., Trethowan P., Macdonald D. W., Loveridge A. J. (2017). Dietary niche differentiation facilitates coexistence of two large carnivores. J. Zool. 302, 149–156. doi: 10.1111/jzo.12443
Farida W. R., Semiadi G., Handayani T. H., Harun (2006). Habitat distribution and diversity of plants as feed resources for mouse deer (Tragulus javanicus) and barking deer (Muntiacus muntjak) in Gunung Halimun National Park. Tropics 15, 371–376. doi: 10.3759/tropics.15.371
Farris Z. J., Gerber B. D., Karpanty S., Murphy A., Wampole E., Ratelolahy F., et al. (2020). Exploring and interpreting spatiotemporal interactions between native and invasive carnivores across a gradient of rainforest degradation. Biol. Invasions 22, 2033–2047. doi: 10.1007/s10530-020-02237-1
Feng R., Lü X., Xiao W., Feng J., Sun Y., Guan Y., et al. (2021). Effects of free-ranging livestock on sympatric herbivores at fine spatiotemporal scales. Landsc. Ecol. 36, 1441–1457. doi: 10.1007/s10980-021-01226-6
Fiske I. J., Chandler R. B. (2011). unmarked: an R package for fitting hierarchical models of wildlife occurrence and abundance. J. Stat. Software 43, 1–23. doi: 10.18637/jss.v043.i10
Foster C. N., Barton P. S., Lindenmayer D. B. (2014). Effects of large native herbivores on other animals. J. Appl. Ecol. 51, 929–938. doi: 10.1111/1365-2664.12268
Harmsen B. J., Foster R. J., Silver S. C., Ostro L. E. T., Doncaster C. P. (2009). Spatial and temporal interactions of sympatric jaguars (panthera onca) and pumas (puma concolor) in a neotropical forest. J. Mammal. 90, 612–620. doi: 10.1644/08-MAMM-A-140R.1
Hazwan M., Samantha L. D., Tee S. L., Kamarudin N., Norhisham A. R., Lechner A. M., et al. (2022). Habitat fragmentation and logging affect the occurrence of lesser mouse-deer in tropical forest reserves. Ecol. Evol. 12, 1–12. doi: 10.1002/ece3.8745
Helaouët P., Beaugrand G. (2009). Physiology, ecological niches and species distribution. Ecosystems 12, 1235–1245. doi: 10.1007/s10021-009-9261-5
Hellmann J. J. (2013). Species interactions. Encycl. Biodivers. Second Ed. 6, 715–725. doi: 10.1016/B978-0-12-384719-5.00134-9
Holt R. D. (2013). “Species coexistence”. In Encyclopedia of biodiversity, 2nd edition. ed. Levin S. A. (Waltham: Academic Press), 667–668.
Hutchinson G. E. (1959). Homage to Santa Rosalia, or why are there so many kinds of animals? Am. Nat. 93, 145–159. doi: 10.1086/282070
IUCN (2022) The IUCN Red List of Threatened Species. Available at: https://www.iucnredlist.org.
Jenks K. E., Chanteap P., Damrongchainarong K., Cutter P., Cutter P., Redford T., et al. (2011). Using relative abundance indices from camera-trapping to test wildlife conservation hypotheses - an example from Khao Yai National Park, Thailand. Trop. Conserv. Sci. 4, 113–131. doi: 10.1177/194008291100400203
Jiang Z., Jiang J., Wang Y., Zhang E., Zhang Y., Li L., et al. (2016). Red list of China’s vertebrates. Biodivers. Sci. 24, 500–551. doi: 10.17520/biods.2016076
Kamler J. F., Inthapanya X., Rasphone A., Bousa A., Vongkhamheng C., Johnson A., et al. (2020). Diet, prey selection, and activity of Asian golden cats and leopard cats in northern Laos. J. Mammal. 101, 1267–1278. doi: 10.1093/jmammal/gyaa113
Kamler J. F., Johnson A., Vongkhamheng C., Bousa A. (2012). The diet, prey selection, and activity of dholes (Cuon alpinus) in northern Laos. J. Mammal. 93, 627–633. doi: 10.1644/11-MAMM-A-241.1
Karanth K. U., Srivathsa A., Vasudev D., Puri M., Parameshwaran R., Samba Kumar N. (2017). Spatio-temporal interactions facilitate large carnivore sympatry across a resource gradient. Proc. R. Soc B Biol. Sci. 284, 20161860. doi: 10.1098/rspb.2016.1860
Kavčić K., Radočaj T., Corlatti L., Safner T., Gračanin A., Mikac K. M., et al. (2021). Spatio-temporal response of forest-dwelling chamois to red deer presence. Mamm. Biol. 101, 907–915. doi: 10.1007/s42991-021-00147-w
Khoo M. D. Y., Lim B. T. M., Soh M. C. K., Loy R. H. Y., Lua H. K., Lee B. P. Y. H., et al. (2021). Persistence of a locally endangered mouse-deer amidst the re-emergence of two larger ungulates in small urban rainforest fragments. Glob. Ecol. Conserv. 30, e01807. doi: 10.1016/j.gecco.2021.e01807
Kronfeld-Schor N., Dayan T. (2003). Partitioning of time as an ecological resource. Annu. Rev. Ecol. Evol. Syst. 34, 153–181. doi: 10.1146/annurev.ecolsys.34.011802.132435
Leyequién E., De Boer W. F., Cleef A. (2007). Influence of body size on coexistence of bird species. Ecol. Res. 22, 735–741. doi: 10.1007/s11284-006-0311-6
Luo A., Huang J., Dong Y., Song J., Tang A., Feng G. (1999). Preliminary study on habitat selection of Tragulus javanicus williamsoni Kloss in Xishuangbanna Nature Reserve. Yunnan For. Sci. Technol. 87, 66–70. doi: 10.16473/j.cnki.xblykx1972.1999.02.013
MacArthur R., Levins R. (1967). The limiting similarity, convergence, and divergence of coexisting species. Am. Nat. 101, 377–385. doi: 10.1086/282505
Matsubayashi H., Bosi E., Kohshima S. (2003). Activity and habitat use of lesser mouse-deer (Tragulus javanicus). J. Mammal. 84, 234–242. doi: 10.1644/1545-1542(2003)084<0234:AAHUOL>2.0.CO;2
Mazerolle M. J. (2020) AICcmodavg: Model selection and multimodel inference based on (Q)AIC(c). Available at: https://cran.r-project.org/package=AICcmodavg.
Meijaard E. (2011). ““Family Tragulidae (chevrotains),”,” in Handbook of the Mammals of the World. Volume 2. Hoofed Mammals, vol. 2 . Eds. Wilson D. E., Mittermeier R. A. (Madrid, Spain: Lynx Edicions), 320–335. doi: 10.5040/9781472926968.part-0020
Meijaard E., Chua M. A. H., Duckworth J. W. (2017). Is the northern chevrotain, Tragulus williamsoni Klos a synonym or one of the least-documented mammal species in Asia? Raffles Bull. Zool. 65, 506–514. doi: 10.5281/zenodo.5357782
Monterroso P., Alves P. C., Ferreras P. (2014). Plasticity in circadian activity patterns of mesocarnivores in Southwestern Europe: implications for species coexistence. Behav. Ecol. Sociobiol. 68, 1403–1417. doi: 10.1007/s00265-014-1748-1
Morin P. J., Johnson E. A. (1988). Experimental studies of asymmetric competition among anurans. Oikos 53, 398–407.
Niedballa J., Sollmann R., Courtiol A., Wilting A. (2016). camtrapR: an R package for efficient camera trap data management. Methods Ecol. Evol. 7, 1457–1462. doi: 10.1111/2041-210X.12600
Niedballa J., Wilting A., Sollmann R., Hofer H., Courtiol A. (2019). Assessing analytical methods for detecting spatiotemporal interactions between species from camera trapping data. Remote Sens. Ecol. Conserv. 5, 272–285. doi: 10.1002/rse2.107
O’Brien T. G., Kinnaird M. F., Wibisono H. T. (2003). Crouching tigers, hidden prey: Sumatran tiger and prey populations in a tropical forest landscape. Anim. Conserv. 6, 131–139. doi: 10.1017/S1367943003003172
Pilorz V., Helfrich-Förster C., Oster H. (2018). The role of the circadian clock system in physiology. Pflugers Arch. Eur. J. Physiol. 470, 227–239. doi: 10.1007/s00424-017-2103-y
Pimm S. L., Rosenzweig M. L., Mitchell W. (1985). Competition and food selection: field tests of a theory. Ecology 66, 798–807. doi: 10.2307/1940541
Pollock L. J., Tingley R., Morris W. K., Golding N., O’Hara R. B., Parris K. M., et al. (2014). Understanding co-occurrence by modelling species simultaneously with a Joint Species Distribution Model (JSDM). Methods Ecol. Evol. 5, 397–406. doi: 10.1111/2041-210X.12180
Rasphone A., Bousa A., Vongkhamheng C., Kamler J. F., Johnson A., Macdonald D. W. (2022). Diet and prey selection of clouded leopards and tigers in Laos. Ecol. Evol. 12, 1–11. doi: 10.1002/ece3.9067
R Core Team (2022). R: A language and environment for statistical computing (Vienna, Austria: R Foundation for Statistical Computing). Available at: https://www.R-project.org/.
Richmond O. M. W., Hines J. E., Beissinger S. R. (2010). Two-species occupancy models: a new parameterization applied to co-occurrence of secretive rails. Ecol. Appl. 20, 2036–2046. doi: 10.1890/09-0470.1
Ridout M. S., Linkie M. (2009). Estimating overlap of daily activity patterns from camera trap data. J. Agric. Biol. Environ. Stat. 14, 322–337. doi: 10.1198/jabes.2009.08038
Ritchie M. E., Olff H. (1999). Spatial scaling laws yield a synthetic theory of biodiversity. Nature 400, 557–560. doi: 10.1038/23010
Roth V. L. (1981). Constancy in the size ratios of sympatric species. Am. Nat. 118, 394–404. doi: 10.1086/283831
Roughgarden J. (1983). Competition and theory in community ecology. Am. Nat. 122, 583–601. doi: 10.1086/284160
Rovero F., Marshall A. R. (2009). Camera trapping photographic rate as an index of density in forest ungulates. J. Appl. Ecol. 46, 1011–1017. doi: 10.1111/j.1365-2664.2009.01705.x
Rowcliffe M. (2022) activity: animal activity Statistics. Available at: https://cran.r-project.org/package=activity.
Schoener T. W. (1974). Resource partitioning in ecological communities. Science 185, 27–39. doi: 10.1126/science.185.4145.27
Smith A. T., Xie Y. (2009). A guide to the mammals of China ( Changsha, Hunan: Hunan education publishing house).
Staudenmaier A. R., Shipley L. A., Bibelnieks A. J., Camp M. J., Thornton D. H. (2021). Habitat use and spatio-temporal interactions of mule and white-tailed deer in an area of sympatry in NE Washington. Ecosphere 12. doi: 10.1002/ecs2.3813
Stone L., Roberts A. (1990). The checkerboard score and species distributions. Oecologia 85, 74–79. doi: 10.1007/BF00317345
Wandrag E. M., Catford J. A., Duncan R. P. (2019). Quantifying niche availability, niche overlap and competition for recruitment sites in plant populations without explicit knowledge of niche axes. J. Ecol. 107, 1791–1803. doi: 10.1111/1365-2745.13137
Wang F., McShea W. J., Li S., Wang D. (2018). Does one size fit all? A multispecies approach to regional landscape corridor planning. Divers. Distrib. 24, 415–425. doi: 10.1111/ddi.12692
Wang F., Winkler J., Viña A., McShea W. J., Li S., Connor T., et al. (2021). The hidden risk of using umbrella species as conservation surrogates: a spatio-temporal approach. Biol. Conserv. 253. doi: 10.1016/j.biocon.2020.108913
Wilson D. S. (1975). The adequacy of body size as a niche difference. Am. Nat. 109, 769–784. doi: 10.1086/283042
Wissinger S. A. (1992). Niche overlap and the potential for competition and intraguild predation between size-structured populations. Ecology 73, 1431–1444. doi: 10.2307/1940688
You Z., Lu B., Du B., Liu W., Jiang Y., Ruan G., et al. (2022). Spatio-temporal niche of sympatric tufted deer (Elaphodus cephalophus) and sambar (Rusa unicolor) based on camera traps in the Gongga mountain national nature reserve, China. Animals 12, 2694. doi: 10.3390/ani12192694
Keywords: body size, coexistence, daily activity pattern, direct temporal avoidance, interspecific competition, occupancy, sympatric ungulates
Citation: He R-C, Wang L, Goodale E, Li K and Quan R-C (2023) The extremely small body size of Williamson’s mouse deer (Tragulus williamsoni) allows coexistence with sympatric larger ungulates through temporal avoidance. Front. Ecol. Evol. 11:1125840. doi: 10.3389/fevo.2023.1125840
Received: 24 February 2023; Accepted: 18 September 2023;
Published: 05 October 2023.
Edited by:
Michael Bonsall, University of Oxford, United KingdomReviewed by:
Zhensheng Liu, Northeast Forestry University, ChinaMarco Apollonio, University of Sassari, Italy
Copyright © 2023 He, Wang, Goodale, Li and Quan. This is an open-access article distributed under the terms of the Creative Commons Attribution License (CC BY). The use, distribution or reproduction in other forums is permitted, provided the original author(s) and the copyright owner(s) are credited and that the original publication in this journal is cited, in accordance with accepted academic practice. No use, distribution or reproduction is permitted which does not comply with these terms.
*Correspondence: Rui-Chang Quan, cXVhbnJjQHh0YmcuYWMuY24=