- 1College of Biology and the Environment, Nanjing Forestry University, Nanjing, China
- 2Co-Innovation Center for Sustainable Forestry in Southern China, Nanjing, China
- 3Faculty of Natural Resources Management, Lakehead University, Thunder Bay, ON, Canada
Introduction: Soil enzymes play a critical role in organic matter decomposition and nutrient cycling in forest ecosystems. However, the effects of forest gaps on soil enzyme activities remain uncertain.
Methods: This study aims to investigate the short-term effects of forest gap size on soil enzyme activities in Platycladus orientalis plantations. We conducted a study in a 50-year Platycladus orientalis plantation in Xuzhou, sampling soils from three levels of forest gap size (4 m radius, S; 8 m radius, M and 12 m radius, L) at different positions (within gap, edge, and outside the gap) and control plots (CK, no gaps) 2a after the creation of gaps. Soil peroxidase, dehydrogenase, urease, and invertase activities were measured.
Results: Specifically, we found that M and S gaps had significantly (p < 0.05) higher soil peroxidase activity at the outside position in April and October, respectively, than CK. Additionally, L gaps had significantly (p < 0.05) higher soil dehydrogenase activity at the outside position in April than CK. Furthermore, L and S gaps had significantly (p < 0.05) higher soil urease activity at the outside position in October and July, respectively, than CK. Lastly, L and S gaps had significantly (p < 0.05) higher soil urease activity at the outside position in July than CK.
Conclusion: Our findings highlight the significant impact of canopy gaps on soil enzyme activities, which has important implications for forest management and conservation.
Introduction
Forest gaps have received considerable attention in recent decades as a type of disturbance and are widely recognized as a significant factor in forest dynamics (Martinez-Ramos et al., 1989; Clark, 1990). Gaps commonly arise as part of forest management activities and are thought to play a crucial role in nutrient cycling and forest regeneration (Gray et al., 2012; Yang et al., 2017; Wang et al., 2019). Due to increased irradiance, gaps are brighter and warmer, but their surface soils contain more water due to the reduction in plant transpiration (Denslow, 1987). Furthermore, gaps have a significant impact on soil microbial biomass and activity by altering microclimatic conditions and reducing litter inputs, which can lead to changes in soil biochemical properties (Denslow, 1987).
The effects of gap size on soil microbial properties remains uncertain. Although several studies conducted in conifer stands have found no significant differences in mean air temperature during the growing season between gaps of varying sizes (Gray et al., 2002; Muscolo et al., 2007a,b), many others have demonstrated that soil temperature increases with increasing gap size (Gray et al., 2002; Gugliotta et al., 2006; Muscolo et al., 2007a,b). Gap opening has a particularly significant impact on soil moisture content, which is higher inside gaps than under the surrounding closed canopy, as observed in a wide range of forest types (Ostertag, 1998; Zhu et al., 2003; Albanesi et al., 2008; Sariyildiz, 2008).
Soil enzyme activity, as the main catalyst of many biochemical reactions in soil, is influenced by a series of physico-chemical and biological factors. Muscolo et al. (2007a) demonstrated that small gaps in pine forests, which had greater microbial biomass and larger populations of bacteria and fungi, contributed to a more rapid and balanced turnover of organic matter and nutrients, suggesting that creating small gaps is a silvicultural practice with minimal environmental impact. This study also suggested that small gaps enhance soil enzyme activity and concluded that creating small gaps was an appropriate method of forest management. Yang et al. (2017) found that approximately one year after creating gaps in the forest, small gaps promoted microbial communities and enzyme activity compared with closed canopies. They also observed that soil β-glucosidase activity and ι-leucine aminopeptidase activity were lowest in sites with large gaps and highest in those with small gaps in a Chinese pine forest. It is essential to gain a better understanding of how gap size affects soil microbial biomass and enzyme activities to develop effective forest management and conservation strategies accordingly.
Platycladus orientalis is one of the primary afforestation species in Jiangsu Province, and the creation of artificial gaps in Platycladus orientalis forests is a commonly employed silvicultural practice to reduce forest density. However, the relationship between gap size and soil enzyme activity in this species requires further investigation.
In this study, we investigated the effects of small gaps (4 m radius, S), medium gaps (8 m radius, M), and large gaps (12 m radius, L) on enzyme activity 1a after gap creation, in Platycladus orientalis plantation located in Xuzhou, eastern China. Along with characterizing soil physico-chemical and biological factors, such as soil soluble organic carbon (SOC), total carbon (TC) and total nitrogen (TN), microbial biomass carbon and nitrogen (MBC and MBN), we tested two hypotheses: (1) temperature-sensitive enzyme activity in forest gaps changes with gap size due to the variation in soil temperature, and (2) the establishment of forest gaps alters the surrounding microclimate conditions, and the impact of forest gaps on soil enzyme activity also increases with gap size. We anticipate that this study will provide valuable insights into the relationship between soil enzyme activity and forest gap size, as well as forest management practices.
Materials and methods
Study region
This study was conducted on Jiuli Hill (34°18’N, 117°14′E), located in Xuzhou City, with elevations ranging from 125 to 150 m a.s.l (Figure 1A). This region is situated in a warm-temperate zone and has a monsoon climate with a mean annual temperature of 14.0°C and a mean annual precipitation of 872 mm (2002–2013 annual average). The plant community is dominated by Oriental arborvitae (Platycladus orientalis) plantations at a density of 1,317 stems ha-1, along with a few coniferous-broad-leaved mixed forests. The shrub layer under the forest canopy is primarily composed of a large number of Broussonetia papyrifera (L.) L’Hér. ex Vent.
Experimental design and soil sampling
In January 2012, circular forest gaps of three sizes were randomly established on the southwest slope of Jiuli Hill. The size of the forest gaps was proportionate to the average crown canopy height: 0.5, 1.0, and 1.5. The gap diameters were 8 m, 16 m, and 24 m. After the trees were cut to create the gaps, plant debris was removed from the gap area, but stumps were not extracted. Three replicates were established for each gap size, as well as for control plots (CK) situated in a nearby closed forest that was at least 100 m from any canopy gap. Within each gap, 13 sampling points were arranged at the center and at 0.5, 1.0, and 1.5 times the gap radius in each of the four cardinal directions. Thus, in each direction, a point was located within the gap (I), at the gap edge (E), and outside the gap (O) in the undisturbed forest (Figure 1B).
Soil samples were collected from the nine treatment and three control plots by removing the undecomposed litter layer collecting mineral soil from a depth of 0 to 10 cm at each sampling point. Soils collected at the 4 sampling points (I, E, O, and CK) were combined to form a uniform mixture for each sample location. Soil samples were sieved (2 mm), labeled, and promptly brought back to the laboratory for analysis. The soils were sampled in October 2013, January 2014, April 2014, and July 2014.
Laboratory analysis
Soil physico-chemical property determination
Soil temperature was measured in the field using a button-type temperature sensor (DS1921-F5#, Maxim, USA) with a recording interval of 30 min. Soil moisture content was calculated by measuring weight loss after oven drying approximately 10 g of fresh soil for 24 h at 105°C. Soil total carbon (TC) and total nitrogen (TN) were measured using an elemental analyzer (Vario EL III, Elementar, Germany). Soil pH was determined using potentiometry with a water/soil ratio of 1: 2.5.
Determination of soil soluble organic carbon, microbial biomass carbon, and nitrogen content
The soil soluble organic carbon (SOC) content was quantified using a carbon analyzer (Shimadzu TOC-VCPN, Japan). Microbial biomass carbon and nitrogen (MBC and MBN) content of the soil were determined using the chloroform fumigation method (Brookes et al., 1985; Vance et al., 1987). Briefly, 20 g of fresh soil was fumigated for 24 h at 25°C with alcohol-free CHCl3 in a desiccator. Following removal of the CHCl3, the soil was extracted (fumigated and unfumigated) with 80 ml of 0.5 M K2SO4 for 30 min. Organic C in the solution was analyzed using a carbon analyzer (Shimadzu TOC-VCPN, Japan). The difference between fumigated and unfumigated samples was used to calculate MBC, using a conversion factor of kEC = 0.45 (Joergensen and Mueller, 1996): MBC = EC/kEC, while MBN was calculated using a conversion factor of kEN = 0.68 (Brookes et al., 1985): MBN = EN/kEN.
Soil enzyme activity
Soil enzyme activity was assessed using the colorimetric method with a spectrophotometer. Soil peroxidase activity was determined based on the procedure outlined by Markkola et al. (1990) and expressed as μg purple gallic acid g−1 soil h−1. Soil dehydrogenase activity was estimated using the method described by Casida et al. (1964) and expressed as μg TPF g−1 soil h−1. Soil urease activity was determined according to the method of Kandeler and Gerber (1988) and expressed as mg NH4-N g−1 soil d−1. Soil invertase activity was estimated based on the method provided by Frankeberger and Johanson (1983) and expressed as mg glucose g−1 soil d−1.
Statistical analysis
The differences among different gap sizes were analyzed using one-way analysis of variance (ANOVA) in SPSS 20.0 and multiple comparisons were conducted with the LSD method (p ≤ 0.05). Pearson correlation analysis was applied to explore the relationships between soil enzyme activities and related explanatory factors.
Results
Response of soil temperature and moisture to different gap sizes
Forest gap size had greater effects on soil temperature in autumn and spring. As shown in Figure 2, the daily average temperature at a depth of 10 cm exhibited a peak in July (26.0°C) and a nadir in December (4.0°C) across all gap sizes. In October, the trend of daily average soil temperature was CK>S>L>M, whereas in January, L>CK>S>M. In April, the trend was M>L>S>CK, and in July, L>M>CK>S.
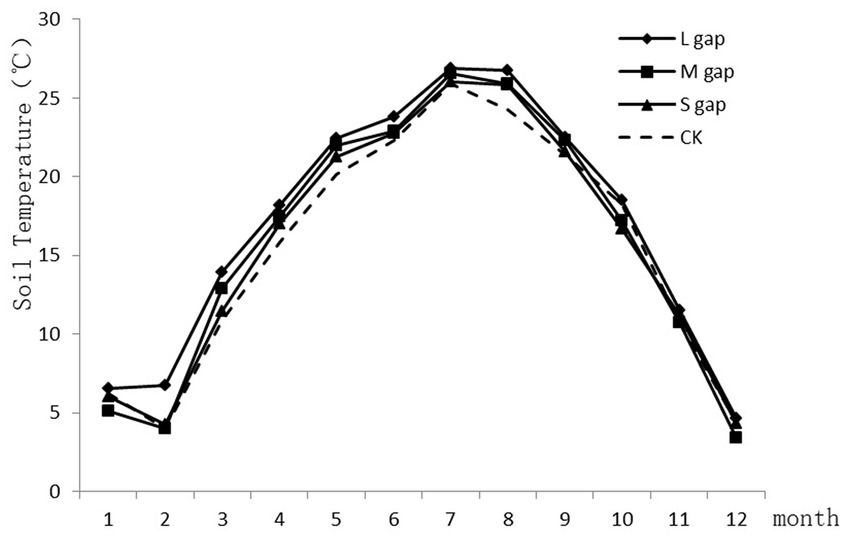
Figure 2. Monthly mean soil temperature in different sizes of gaps in Platycladus orientalis plantation.
As illustrated in Figure 3, soil moisture was highest in April and July (25%), lowest in October (10%), and 15% in April. In comparison with CK, there was no significant difference in soil moisture in October, while significant increases in soil moisture were observed under M gaps in January and April as well as S gaps in July. On the other hand, a reduction in soil moisture under L gaps were observed in July.
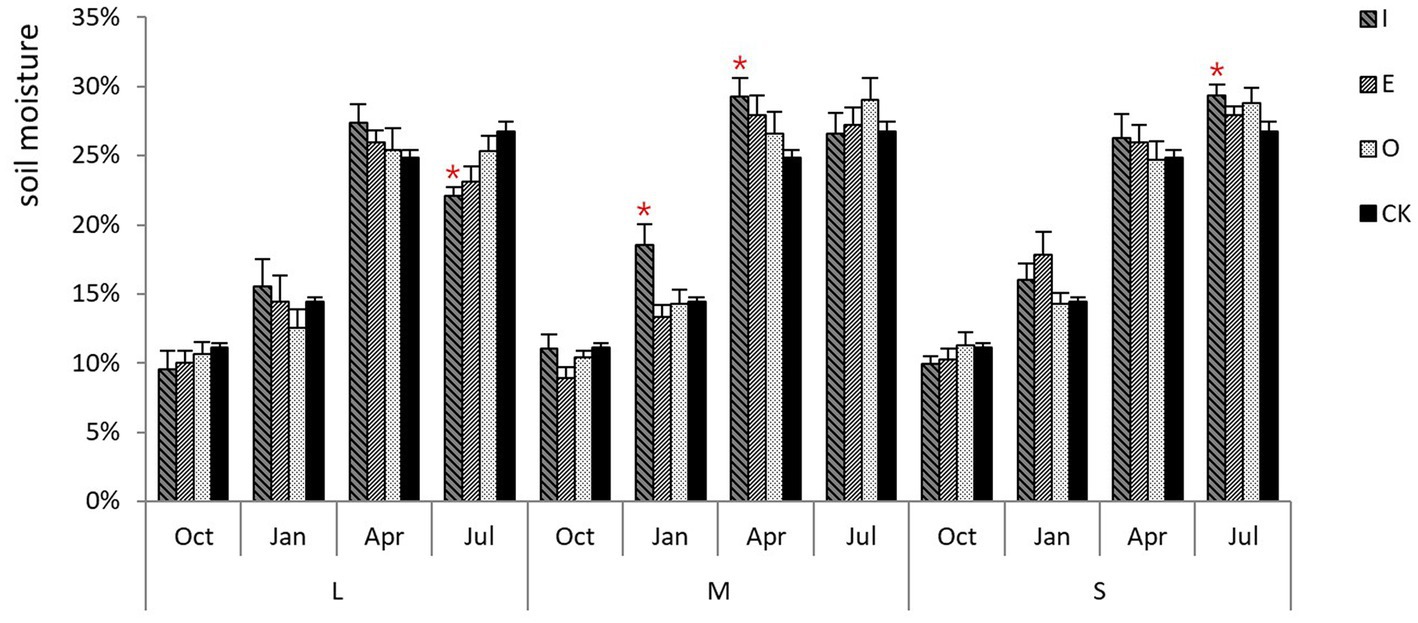
Figure 3. Soil moisture in different sizes of gaps in Platycladus orientalis plantation, the “*“signs represent significant differences.
Response of soil physico-chemical properties to different gap sizes
Compared to CK, this study found differences in SOC, TC, TN, and TC/TN among different gap sizes (Table 1). Additionally, MBC was found to increase at I and E location of M gaps in April compared to CK. Meanwhile, the S gaps resulted in a significant reduction in soil MBN (p < 0.05) in April at both I and O location, while the L gaps significantly increased the soil MBN in July (p < 0.05) at O location.
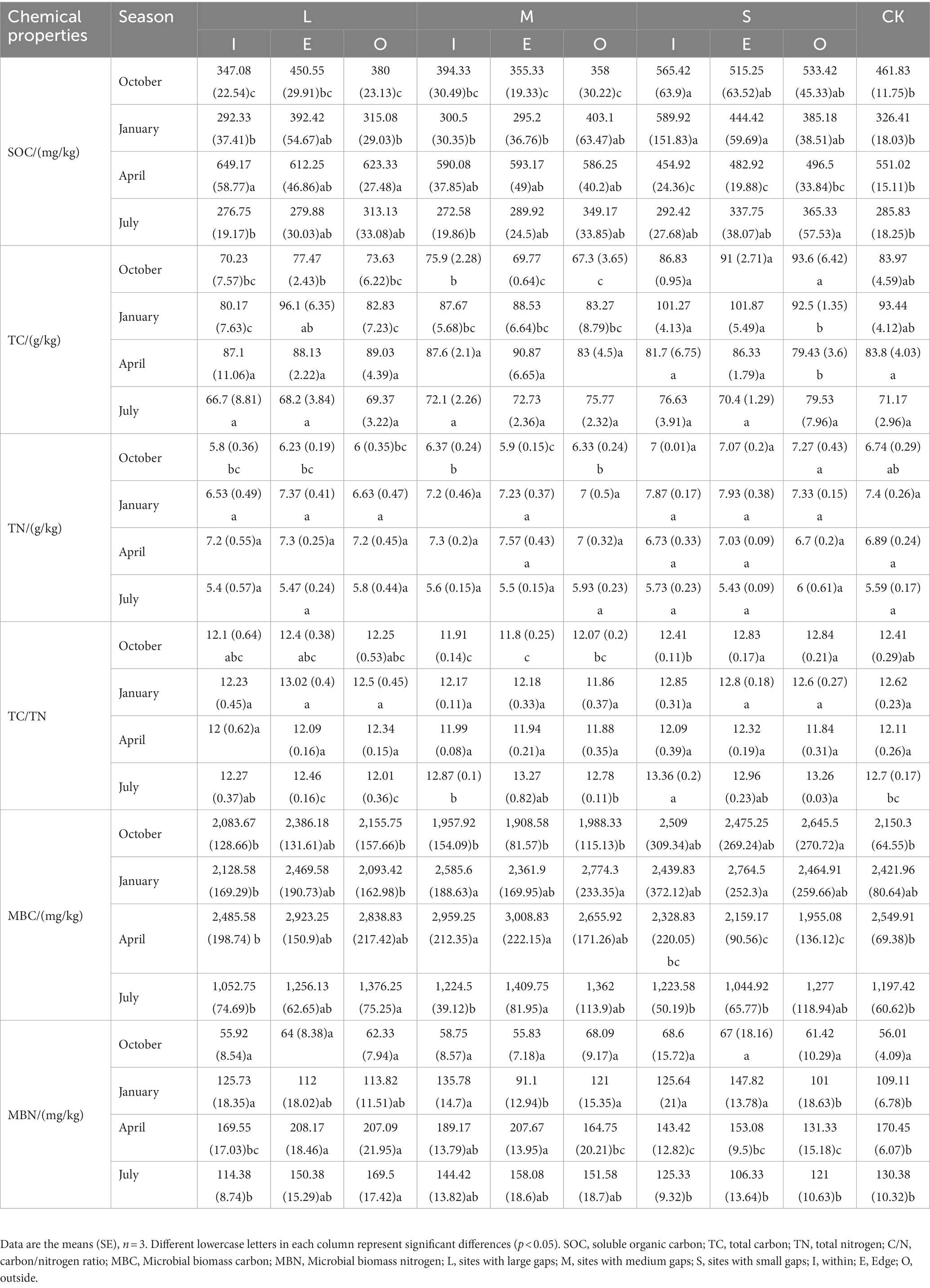
Table 1. Summary of soil chemical properties, MBC and MBN of samples from large-gap, medium-gap, small-gap, and control plots.
Response of four soil enzyme activities to different locations of gaps
Compared to CK, the soil peroxidase activity was significantly (p < 0.05) increased by M gaps (6.42 μg purple gallic acid g−1 soil h−1) at E location in July and significantly increased in April at O location (3.42 μg purple gallic acid g−1 soil h−1) (Figure 4). The gaps also significantly increased the soil peroxidase activity (p < 0.05) in October at O location (6.29 μg purple gallic acid g−1 soil h−1) while those significantly increased the soil peroxidase activity (p < 0.05) in April at E location (3.80 μg purple gallic acid g−1 soil h−1).
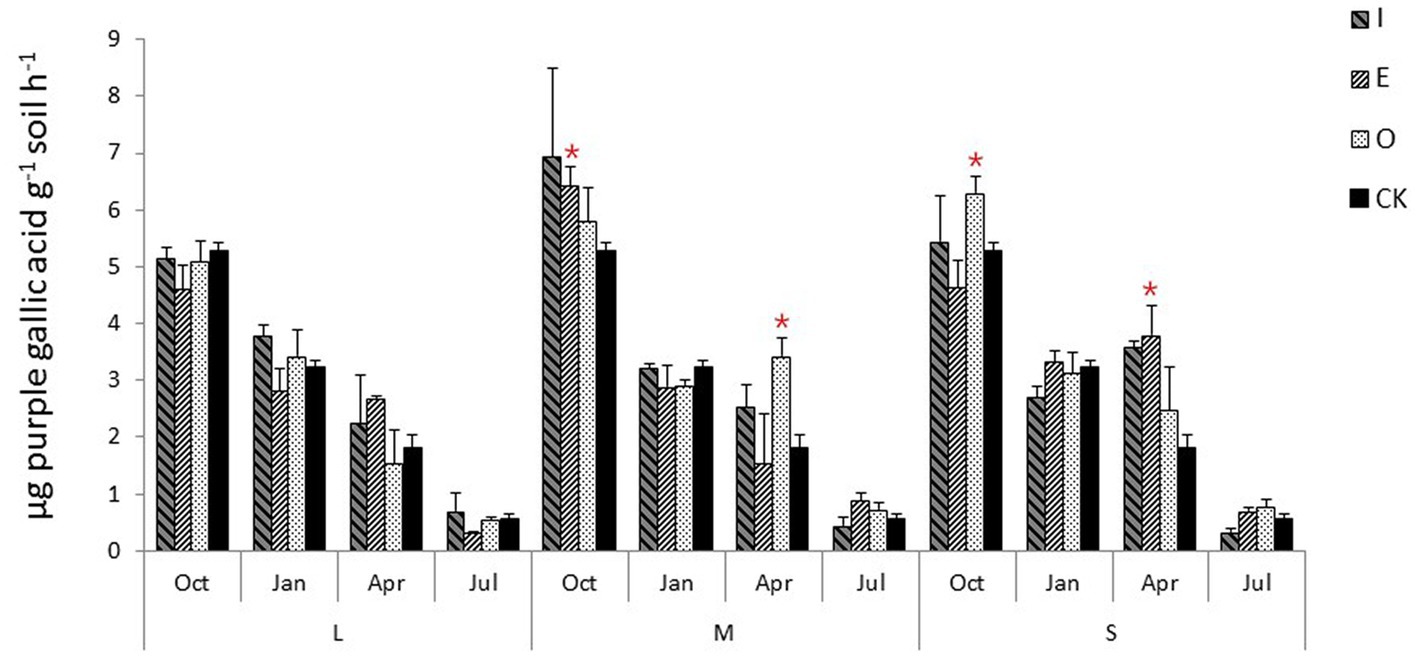
Figure 4. Soil peroxidase activity of different position in different sizes of gaps, the “*“signs represent significant differences.
Compared to CK, the L gaps significantly increased the soil dehydrogenase activity (p < 0.05) in January at E location (2,988.25 μg TPF g−1 soil h−1) (Figure 5). Additionally, the L gaps significantly increased the soil dehydrogenase activity (p < 0.05) in April at I (2,437.51 μg TPF g−1 soil h−1), E (2,658.04 μg TPF g−1 soil h−1) and O (2,190.87 μg TPF g−1 soil h−1) locations. The M gaps also increased the soil dehydrogenase activity (p < 0.05) in April at I (2,133.61 μg TPF g−1 soil h−1) and E (2,342.00 μg TPF g−1 soil h−1) locations. Meanwhile, the S gaps significantly increased the soil dehydrogenase activity (p < 0.05) in October at I location (2,391.81 μg TPF g−1 soil h−1).
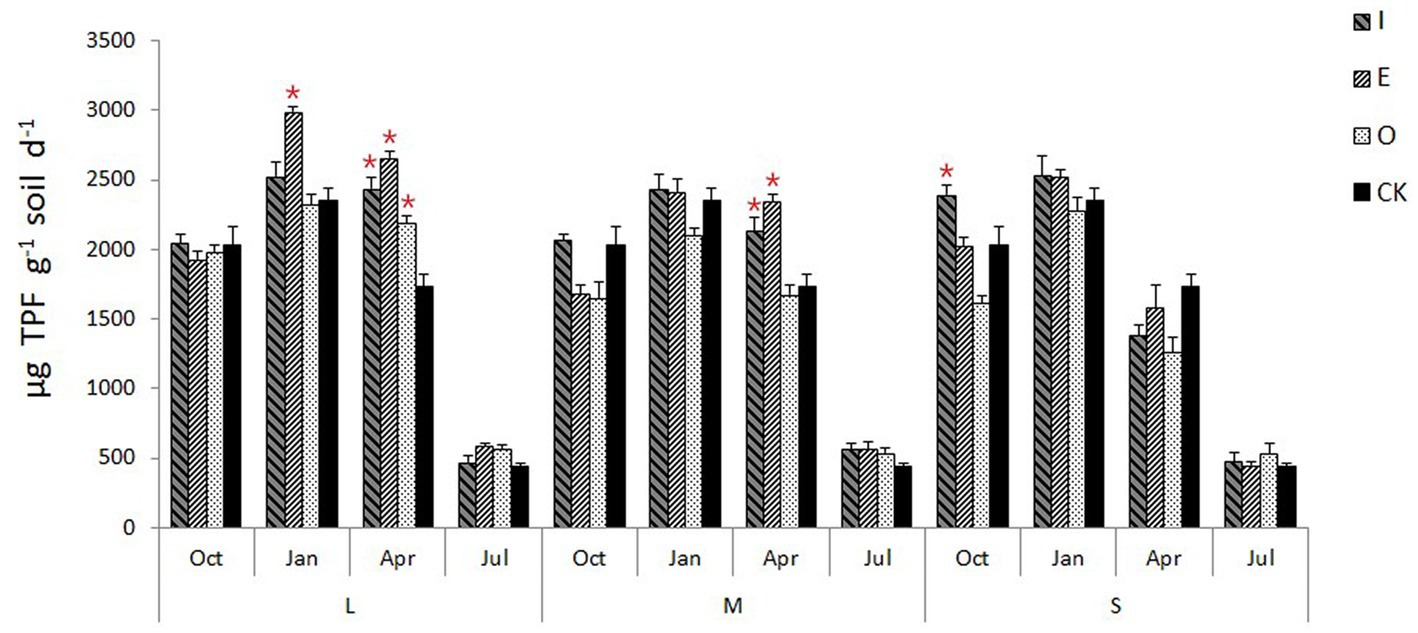
Figure 5. Soil dehydrogenase activity of different position in different sizes of gaps, the “*“signs represent significant differences.
Compared to CK, L gaps significantly increased the soil urease activity (p < 0.05) at I (946.8 mg NH4-N g−1 soil d−1) and O location (921.3 mg NH4-N g−1 soil d−1) in October, while significantly increased the soil urease activity (p < 0.05) in April (1,893.8 mg NH4-N g−1 soil d−1) and July (1,110.2 mg NH4-N g−1 soil d−1) at E location; (Figure 6). M gaps increased the soil urease activity (p < 0.05) in July at I (1,036.7 mg NH4-N g−1 soil d−1) and E location (1,068.3 mg NH4-N g−1 soil d−1). S gaps significantly increased the soil urease activity (p < 0.05) in July at both I (1,060.0 mg NH4-N g−1 soil d−1) and O location (916.7 mg NH4-N g−1 soil d−1).
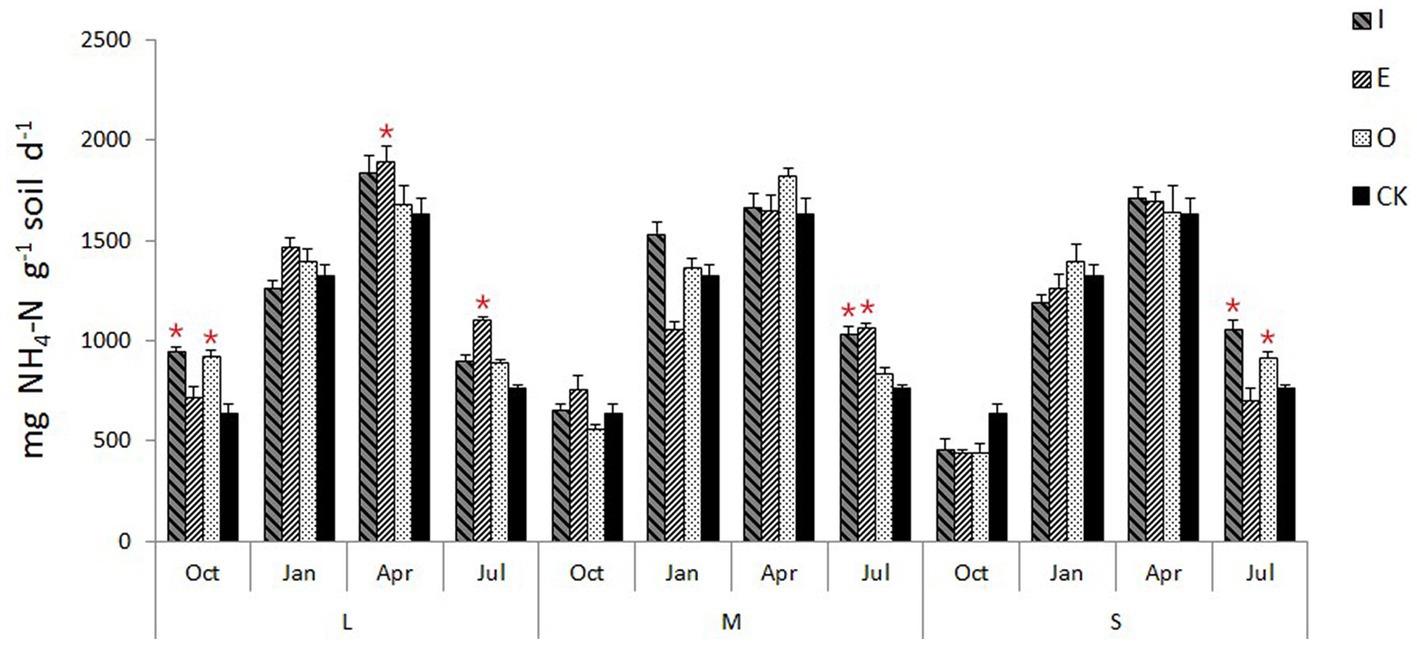
Figure 6. Soil urease activity of different position in different sizes of gaps, the “*“signs represent significant differences.
Compared to CK, L gaps significantly increased the soil invertase activity (p < 0.05) in January (2.93 mg glucose g−1 soil d−1) and July (4.12 mg glucose g−1 soil d−1) at E location and O locations, respectively (Figure 7). Similarly, M gaps significantly increased the soil invertase activity (p < 0.05) in April at I location (2.67 mg glucose g−1 soil d−1), while S gaps significantly increased the soil invertase activity (p < 0.05) in July at both I location (4.58 mg glucose g−1 soil d−1) and O location (3.68 mg glucose g−1 soil d−1).
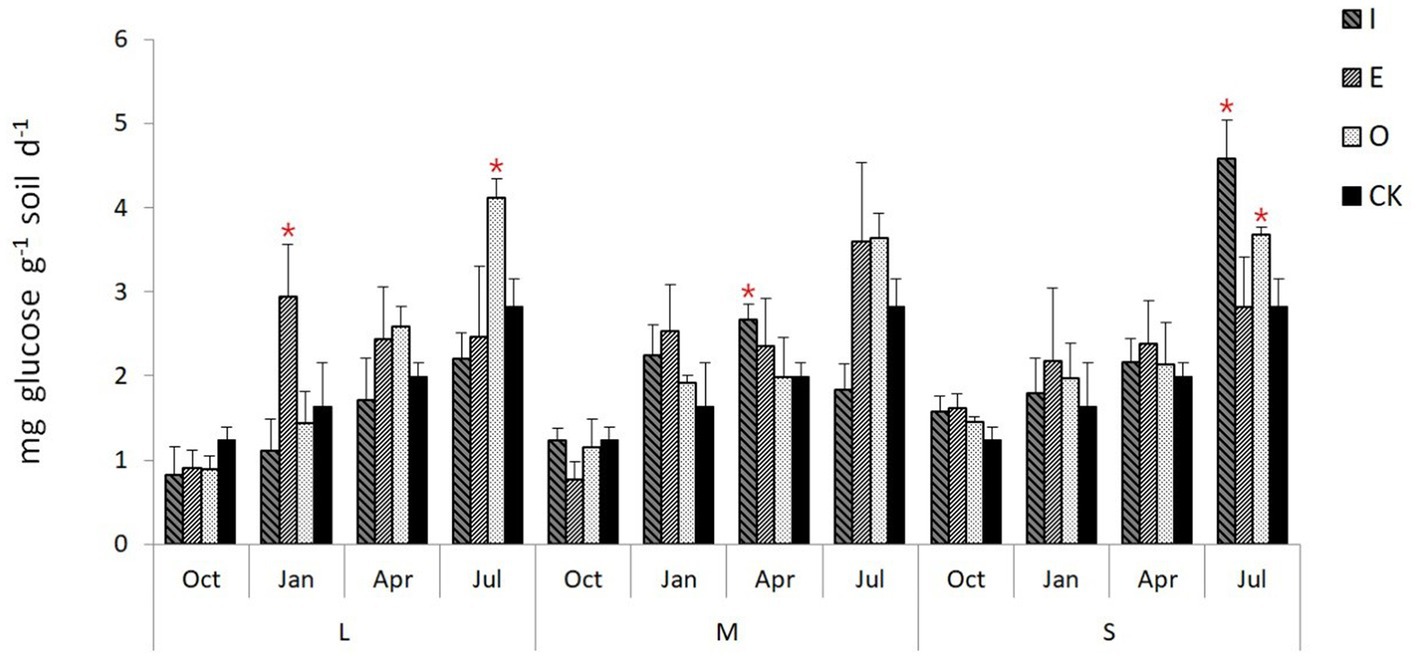
Figure 7. Soil invertase activity of different position in different sizes of gaps, the “*“signs represent significant differences.
Correlation analysis of 4 soil enzyme activities, and soil physico-chemical properties and microbial biomass
Table 2 indicated that there is a significantly and positive correlation between soil peroxidase activity and soil TC (p < 0.05), while a significant negative correlation existed between soil peroxidase activity and soil temperature, soil moisture, TN, C/N, and MBN (p < 0.01). Soil dehydrogenase activity showed an extremely significant positive correlation with soil TC and MBC (p < 0.01), and a significant positive correlation with SOC (p < 0.05). Moreover, soil dehydrogenase activity was significantly and negatively correlated with soil temperature and soil moisture (p < 0.01) and negatively correlated with soil TN (p < 0.05). Soil urease activity exhibited an extremely significant positive correlation with soil TC, SOC, MBC, and MBN (p < 0.01) as well as an extremely significant negative correlation with soil temperature, soil TN and C/N ratio (p < 0.01). Soil invertase activity displayed an extremely significant positive correlation with soil temperature, soil moisture, soil TN and soil MBN (p < 0.01) and a significantly negative correlation with soil MBC (p < 0.05).
Discussion
Luo (2012) investigated the soil enzyme activity of Chinese fir plantation and found a significant correlation between peroxidase activity and soil pH value. However, in the present study, no significant correlation was found between peroxidase activity and pH value. One possible reason for this discrepancy is that the time since gap creation was relatively short, and soil pH, being a fundamental soil property, may not have been significantly influenced by the gaps in the short term. Therefore, soil pH may not be a driving factor for changes in peroxidase activity. The results of this study showed a negative correlation between soil peroxidase activity and soil temperature, possibly due to the higher solar radiation and temperature received by large forest gaps. As a temperature-sensitive enzyme, peroxidase activity was significantly increased in I and O positions of M and S gaps, indicating a promoting effect of the forest understory environment influenced by M and S gaps on peroxidase activity. These findings are consistent with previous studies by Yang et al. (2017) and Muscolo et al. (2007a) and contradict the hypothesis 1 of this study, which suggested that larger forest gaps would have a positive effect on peroxidase activity due to their higher temperature. Instead, it appears that M and S gaps have a positive effect on peroxidase activity in the surrounding soil. Sinsabaugh (2010) found that peroxidase activity is positively correlated with litter quantity. Accordingly, the smaller forest gaps had higher peroxidase activity due to their greater litter quantity compared to the larger ones.
Due to the intracellular nature of dehydrogenase, which does not accumulate extracellularly in soil (Filipović et al., 2020), and the ability of enzymatic activity to rapidly respond to even small changes in environmental conditions (Dussault et al., 2008), dehydrogenase is commonly used as an indicator of soil microbial activity (Makoi and Ndakidemi, 2008). The results of this study showed that soil dehydrogenase activity was significantly higher in all gap sizes compared to CK, and was positively correlated with MBC (p < 0.01), and positively correlated with SOC (p < 0.05), consistent with Lungmuana et al. (2017) who found a strong positive relationship between MBC and enzyme activities in the surface soil layers. Thus, it can be said that the establishment of forest gaps has led to better soil chemical conditions and higher biological activity compared to the forest under canopy, which also supported by the study of Avazpoor et al. (2019). Soil enzymes play a key role in nutrient cycling and SOM mineralization (Gianfreda, 2015).
The findings of this study indicated that soil urease activity was positively correlated with SOC, MBC and MBN (p < 0.01), because many bacteria, fungi, archaea and plants present in the soil produce urease (Fisher et al., 2017). Consistent with the results of this study, Wang et al. (2005) also demonstrated that the transformation of nitrogen in soil was related to urease activity. Furthermore, this study revealed significantly higher urease activity in all types of gaps in July, with larger forest gaps exhibiting higher urease activity due to the humus-urease complexes extracted from soil being highly resistant to extreme temperatures (Makoi and Ndakidemi, 2008). These findings are in line with hypothesis 1, which proposed that enzyme activity in forest gaps changes with gap size due to soil temperature variations.
The study by Yu et al. (2017) showed that invertase activity in soil can hydrolyze sucrose into glucose and fructose as available energy sources for plants and soil microorganisms. Our study found that soil invertase activity was positively correlated with soil temperature, soil moisture, and MBN (p < 0.01), which could be attributed to the increased metabolism of soil microorganisms and the stimulation of microbial activity. In April and July, all three sizes of forest gaps had increased soil invertase activity, indicating that the establishment of forest gaps increased solar radiation and soil temperature. In particular, the soil invertase activity was significantly higher in larger forest gaps and their surroundings, consistent with hypothesis 2 that the impact of forest gaps on soil enzyme activity increases with gap size. The increase in soil invertase activity can increase the microbial population and provide energy and a favorable environment for soil enzymes, which is consistent with the results of the study by Wu et al. (2020).
Conclusion
In this study, forest gap size had different effects on different enzymes. The M and S gaps increased the activity of peroxidase, which is contrary to hypothesis 1 mentioned earlier. The L gaps and its surrounding area showed the most significant increase in dehydrogenase activity, consistent with hypothesis 2. Urease activity increased with gap size, consistent with hypothesis 1. The impact of forest gaps on invertase activity expanded with increasing gap size, consistent with hypothesis 2.
This study explored the impact of forest gap size on soil enzyme activities. and investigates their correlation with physico-chemical properties. The creation of gaps as a silvicultural approach has significant potential to promote ecologically sustainable forest development. Nonetheless, the existing body of research on the interplay between above-and below-ground nutrient cycling in forest gaps remains insufficient, which hinders our comprehensive understanding of gap dynamics and their impact on forest ecosystems. Further research in this area is essential to fill this knowledge gap and improve our understanding of forest ecology.
Data availability statement
The raw data supporting the conclusions of this article will be made available by the authors, without undue reservation.
Author contributions
FF: conceptualization, methodology, software, data curation, and writing–original draft preparation. QG: supervision. XC: writing–reviewing and editing. All authors contributed to the article and approved the submitted version.
Funding
This work was financially supported by the Priority Academic Program Development of Jiangsu Higher Education Institutions (PAPD) and the National Natural Science Foundation of China (No. 31971453).
Conflict of interest
The authors declare that the research was conducted in the absence of any commercial or financial relationships that could be construed as a potential conflict of interest.
Publisher’s note
All claims expressed in this article are solely those of the authors and do not necessarily represent those of their affiliated organizations, or those of the publisher, the editors and the reviewers. Any product that may be evaluated in this article, or claim that may be made by its manufacturer, is not guaranteed or endorsed by the publisher.
References
Albanesi, E., Gugliotta, O. I., Mercurio, I., and Mercurio, R. (2008). Effects of gap size and within-gap position on seedlings establishment in silver fir stands. IForest 1, 55–59. doi: 10.3832/ifor0448-0010055
Avazpoor, Z., Moradi, M., Basiri, R., Mirzaei, J., Taghizadeh-Mehrjardi, R., and Kerry, R. (2019). Soil enzyme activity variations in riparian forests in relation to plant species and soil depth. Arab. J. Geosci. 12:708. doi: 10.1007/s12517-019-4910-2
Brookes, P. C., Landman, A., Pruden, G., and Jenkinson, D. S. (1985). Chloroform fumigation and the release of soil nitrogen: a rapid direct extraction method to measure microbial biomass nitrogen in soil. Soil Biol. Biochem. 17, 837–842. doi: 10.1016/0038-0717(85)90144-0
Casida, L. E., Klein, D. A., and Santoro, T. (1964). Soil dehydrogenase activity. Soil Sci. 98, 371–376. doi: 10.1097/00010694-196412000-00004
Clark, D. B. (1990). The role of disturbance in the regeneration of Neotropical moist forest. Reproduct. Ecol. Trop. Forest Plants 1990, 291–315.
Denslow, J. S. (1987). Tropical rainforest gaps and tree species diversity. Annu. Rev. Ecol. Syst. 18, 431–451. doi: 10.1146/annurev.es.18.110187.002243
Dussault, M., Bécaert, V., François, M., Sauvé, S., and Deschênes, L. (2008). Effect of copper on soil functional stability measured by relative soil stability index (RSSI) based on two enzyme activities. Chemosphere 72, 755–762. doi: 10.1016/j.chemosphere.2008.03.019
Filipović, L., Romić, M., Sikora, S., Huić Babić, K., Filipović, V., Gerke, H. H., et al. (2020). Response of soil dehydrogenase activity to salinity and cadmium species. J. Soil Sci. Plant Nutr. 20, 530–536. doi: 10.1007/s42729-019-00140-w
Fisher, K. A., Yarwood, S. A., and James, B. R. (2017). Soil urease activity and bacterial ureC gene copy numbers: effect of pH. Geoderma 285, 1–8. doi: 10.1016/j.geoderma.2016.09.012
Frankeberger, W. T., and Johanson, J. B. (1983). Method of measuring invertase activity in soils. Plant Soil 74, 301–311. doi: 10.1007/BF02181348
Gianfreda, L. (2015). Enzymes of importance to rhizosphere processes. J. Soil Sci. Plant Nutr. 15, 283–306. doi: 10.4067/s0718-95162015005000022
Gray, A. N., Spies, T. A., and Easter, M. J. (2002). Microclimatic and soil moisture responses to gap formation in coastal Douglas-fir forests. Can. J. For. Res. 32, 332–343. doi: 10.1139/x01-200
Gray, A. N., Spies, T. A., and Pabst, R. J. (2012). Canopy gaps affect long-term patterns of tree growth and mortality in mature and old-growth forests in the Pacific northwest. For. Ecol. Manag. 281, 111–120. doi: 10.1016/j.foreco.2012.06.035
Gugliotta, O., Mercurio, R., and Albanesi, E. (2006). Dynamics of natural regeneration in Pinus laricio stands from southern Apennines (Italy). Forest Rivista Di Selvicoltura Ed Ecologia Forestale 3, 380–386. doi: 10.3832/efor0401-0030380
Joergensen, R. G., and Mueller, T. (1996). The fumigation-extraction method to estimate soil microbial biomass: calibration of the kEN value. Soil Biol. Biochem. 28, 33–37. doi: 10.1016/0038-0717(95)00101-8
Kandeler, E., and Gerber, H. (1988). Short-term assay of soil urease activity using colorimetric determination of ammonium. Biol. Fertil. Soils 6, 68–72. doi: 10.1007/BF00257924
Lungmuana, H., Singh, S. B., Vanthawmliana, H., Saha, S., Dutta, S. K., Rambuatsaiha,, et al. (2017). Impact of secondary forest fallow period on soil microbial biomass carbon and enzyme activity dynamics under shifting cultivation in north Eastern Hill region, India. Catena 156, 10–17. doi: 10.1016/j.catena.2017.03.017
Luo, F. (2012). Seasonal dynamics of the soil enzyme activities under Cunninghamia Lanceolata plantations with different tree ages in acid rain area. Fujian Agriculture and Forestry University.
Makoi, J. H. J. R., and Ndakidemi, P. A. (2008). Selected soil enzymes: examples of their potential roles in the ecosystem. Afr. J. Biotechnol. 7, 181–191. doi: 10.4314/ajb.v7i3.58355
Markkola, A. M., Ohtonen, R., and Tarvainen, O. (1990). Peroxidase activity as an indicator of pollution stress in the fine roots of Pinus sylvestris. Water Air Soil Pollut. 52, 149–156. doi: 10.1007/BF00283120
Martinez-Ramos, M., Alvarez-Buylla, E., and Sarukhan, J. (1989). Tree demography and gap dynamics in a tropical rain Forest. Ecology 70, 555–558. doi: 10.2307/1940203
Muscolo, A., Sidari, M., and Mercurio, R. (2007a). Influence of gap size on organic matter decomposition, microbial biomass and nutrient cycle in Calabrian pine (Pinus laricio, Poiret) stands. For. Ecol. Manag. 242, 412–418. doi: 10.1016/j.foreco.2007.01.058
Muscolo, A., Sidari, M., and Mercurio, R. (2007b). Variations in soil chemical properties and microbial biomass in artificial gaps in silver fir stands. Eur. J. For. Res. 126, 59–65. doi: 10.1007/s10342-006-0145-3
Ostertag, R. (1998). Belowground effects of canopy gaps in a tropical wet forest. Ecology 79, 1294–1304. doi: 10.1890/0012-9658(1998)079[1294:BEOCGI]2.0.CO;2
Sariyildiz, T. (2008). Effects of gap-size classes on long-term litter decomposition rates of beech, oak and chestnut species at high elevations in Northeast Turkey. Ecosystems 11, 841–853. doi: 10.1007/s10021-008-9164-x
Sinsabaugh, R. L. (2010). Phenol oxidase, peroxidase and organic matter dynamics of soil. Soil Biol. Biochem. 42, 391–404. doi: 10.1016/j.soilbio.2009.10.014
Vance, E. D., Brookes, P. C., and Jenkinson, D. S. (1987). An extraction method for measuring soil microbial biomass C. Soil Biol. Biochem. 19, 703–707. doi: 10.1016/0038-0717(87)90052-6
Wang, H., Gong, Y., and Gong, W. (2005). Review on the Relationships of Soil Fertility with Soil Microorganism and Soil Enzyme Activity in the Forest Stand. Sichuan Forestry Exploration and Design 3, 9–12.
Wang, Z., Yang, H., Wang, D., and Zhao, Z. (2019). Spatial distribution and growth association of regeneration in gaps of Chinese pine (Pinus tabuliformis Carr.) plantation in northern China. For. Ecol. Manag. 432, 387–399. doi: 10.1016/j.foreco.2018.09.032
Wu, L., Ma, H., Zhao, Q., Zhang, S., Wei, W., and Ding, X. (2020). Changes in soil bacterial community and enzyme activity under five years straw returning in paddy soil. Eur. J. Soil Biol. 100:103215. doi: 10.1016/j.ejsobi.2020.103215
Yang, Y., Geng, Y., Zhou, H., Zhao, G., and Wang, L. (2017). Effects of gaps in the forest canopy on soil microbial communities and enzyme activity in a Chinese pine forest. Pedobiologia 61, 51–60. doi: 10.1016/j.pedobi.2017.03.001
Yu, P., Liu, S., Han, K., Guan, S., and Zhou, D. (2017). Conversion of cropland to forage land and grassland increases soil labile carbon and enzyme activities in northeastern china. Agriculture, Ecosystems & Environment 245, 83–91. doi: 10.1016/j.agee.2017.05.013
Keywords: forest soil, enzyme activity, Platycladus orientalis plantation, forests ecology, forest gap size
Citation: Fei F, Chen X and Guan Q (2023) Short-term effects of forest gap size on soil enzyme activity in a Platycladus orientalis plantation. Front. Ecol. Evol. 11:1122796. doi: 10.3389/fevo.2023.1122796
Edited by:
Purabi Saikia, Central University of Jharkhand, IndiaReviewed by:
Javid Ahmad Dar, SRM University, IndiaMohammad Aminur Rahman Shah, University of Prince Edward Island, Canada
Copyright © 2023 Fei, Chen and Guan. This is an open-access article distributed under the terms of the Creative Commons Attribution License (CC BY). The use, distribution or reproduction in other forums is permitted, provided the original author(s) and the copyright owner(s) are credited and that the original publication in this journal is cited, in accordance with accepted academic practice. No use, distribution or reproduction is permitted which does not comply with these terms.
*Correspondence: Qingwei Guan, Z3VhbmphcGFuOTk5QDE2My5jb20=
†ORCID: Qingwei Guan, https://orcid.org/0000-0002-4146-5731