- 1Laboratorio de Ecología Fisiológica y del Comportamiento, Instituto de Investigaciones Marinas y Costeras (IIMyC), Consejo Nacional de Investigaciones Científicas y Técnicas (CONICET) - Universidad Nacional de Mar del Plata, Mar del Plata, Argentina
- 2Grupo Multidisciplinar de Oncología Traslacional, Universitat de les Illes Balears e Instituto de Investigación Sanitaria de las Islas Baleares (IdISBa), Palma de Mallorca, Spain
- 3Ciber Fisiopatología Obesidad y Nutrición, Instituto Salud Carlos III, Madrid, Spain
In mammals, during the pup’s development and adult life, integrated requirements of all activities of the individual must conform to a sustained rate of metabolism. Thus, partitioning the available energy according to short-term priorities at a specific moment allows animals to survive and optimize long-term reproductive success. In altricial rodents, thermal balance is a key factor for survival. When no exogenous source of heat is present, altricial pups rapidly lose heat, reaching ambient temperature (Ta). Fossorial rodents showed a strong dependence on burrows, where Ta remains relatively stable within narrow ranges. Pups of the fossorial rodent Ctenomys talarum are altricial, making them an excellent model to evaluate the development of thermogenic capacity. In this study, the ontogeny of the thermogenic capacity of pups of C. talarum was evaluated. Using respirometry techniques, non-shivering thermogenesis (NST), total thermogenic capacity (cold-induced maximum metabolic rate, MMR), and resting metabolic rate (RMR) in pups until post-weaning age (day 60) were analyzed. No NST was present in pups until day 60 despite the presence of molecular markers for NST in brown adipose tissue deposits, which became functional in adults. Although pups are altricial at birth, they maintain their thermal balance behaviorally during lactation. Total thermogenic capacity became fixed at an early age, indicating an improvement in shivering thermogenesis (ST) efficiency after day 10, which might be related to the development of musculature related to digging. Before the aboveground dispersal period (~day 60), pups gradually reached adult Tb by improving ST and thermal isolation, allowing them to confront climatic fluctuations on the surface.
Introduction
The energy budget, that is, a sum of processes associated with energy intake and expenditure, has enormous relevance to individual fitness since it integrates all aspects of the life of organisms. The changes in the energy budget during the lifespan are related to requirements and the use of time and space (Withers et al., 2016). Thus, partitioning the available energy according to short-term priorities at a specific moment allows animals to survive and optimizes long-term reproductive success (Wade and Schneider, 1992). In endotherms, the development of thermoregulatory mechanisms after birth is the determinant of the adult’s fitness. The development of functional mechanisms that ensure thermal homeostasis is one of the main requirements that must be achieved before parental care independence (Thompson, 1992). Defined by their physiological maturity, different strategies for the development of endotherm pups have been proposed to exist between altriciality and precociality extremes (Hill, 1992). The distinction between altricial and precocial development is related to the time at which pups become furred, possess capable eyesight, coordinate their locomotor abilities, or, particularly, reach endothermy (McClure and Randolph, 1980). Precocial pups can use physiological thermoregulation at an early stage, whereas altricial pups show a low capacity to control their body temperature (Tb) due to the lack of functional thermogenic mechanisms, losing heat rapidly when they face an ambient temperature (Ta) below thermoneutral zone (TNZ; Hill, 1992). In the case of altricial pups, the requirement for warmth might be provided by the exogenous source of heat. Direct physical contact of pups with their parents might help to overcome the thermal imbalance. Thus, when physiological thermogenic capacity is limited, behavioral thermoregulation could restrict heat loss, for example, either by huddling with other members of the litter (Geiser and Kenagy, 1990), by behavioral thermotaxis (Farrell and Alberts, 2007), or by remaining in the nest (Antinuchi and Busch, 2001). Total thermogenic capacity is defined as the maximum amount of heat that can be produced to maintain a constant Tb and can be approximated by cold-induced maximum metabolic rate (MMR). The components of total thermogenic capacity are shivering. Shivering thermogenesis involves rapid, involuntary contractions of skeletal muscles to produce heat, related to biochemical-mechanical processes at the cellular level, such as inefficiency of biochemical pathways or friction among myofibrils (refer to Hohtola, 2004). Unlike ST, NST has specialized tissues to produce heat without any muscle contraction. Brown adipose tissue (BAT) is the main source of NST (Janský, 1973; Foster and Frydman, 1979; but see Nowack et al., 2017 for a discussion about muscle-mediated NST). BAT is a highly vascularized tissue with a large number of mitochondria and respiratory enzymes and is found in discrete areas in the body, mostly surrounded by the main organs (Cannon and Nedergaard, 2004). The activation of BAT depends on the release of norepinephrine (NE) by the sympathetic nervous system, and its functioning is related to uncoupling the oxidative phosphorylation in BAT’s mitochondria. The presence of uncoupling proteins (UCP), particularly UCP1, short-circuits the electron transport chain circuit, producing heat (Oelkrug et al., 2015; Chouchani et al., 2019).
Depending on the pup’s development strategy, the onset of functional total thermogenic capacity can vary (Brück and Hinckel, 1996). While thermogenic mechanisms in precocial pups are well developed right after birth, complete development takes days in altricial pups (Berthon et al., 1994; Farrell and Alberts, 2007; Canals et al., 2009; Lezama-García et al., 2022). For example, in rats, behavioral control of Tb is important during the first days of life; by the second week, with the increase of body mass and thermal insulation, the appearance of ST enhances thermogenic capacity (Sant Anna and Mortola, 2003). In the white-footed mouse Peromyscus leucopus, NST appears by day 10, whereas ST was observed around 2 weeks after birth (Hill, 1976; Robertson et al., 2019). Deer mice Peromyscus maniculatus from a low-altitude population can shiver at day 14, but only NST is observed in individuals from a high-altitude population at this age (Robertson and McClelland, 2019). Given the variation between altricial species, it is difficult to establish a temporal pattern of postnatal development of different thermogenic mechanisms, but it is generally recognized that, in mammal pups, shivering is not functional at early stages, but NST tends to be active. Deposits of BAT are well developed and matured only a few days after birth, contrary to muscles, which show a slow development during ontogeny (Lagerspetz, 1966; Robertson and McClelland, 2019).
In this general framework, inhabiting a relatively thermally stable environment can diminish the cost of thermoregulation. Some mammalian species live in underground burrows that can buffer cold external Tas and its fluctuation. In particular, subterranean rodents occupy almost all available habitats, from cold to tropical, from mountain slopes to deserts, inhabiting tunnel systems parallel to the surface. Although subterranean burrows buffer Ta and protect against predators, they are stressful in most cases because they are humid, dark, stagnant, and lack most clues available for orientation (Burda et al., 2007). Among rodents, different degrees of adaptation to the use of burrows are present (Begall et al., 2007), with some species adapted to digging and living almost exclusively underground (i.e., subterranean) and some other species also adapted to digging but with extensive use of surface (i.e., fossorial). Either subterranean or fossorial rodents, underground ecotope had determined the observed convergence in morpho-physiological features observed between both groups of rodents (e.g., low mass-specific minimal metabolic rate, a wide range of thermoneutrality, high thermal conductance, and low Tb; McNab, 1966; Vleck, 1979). Different studies have been carried out for evaluating the total thermogenic capacity, ST, and NST in adults of subterranean and fossorial species, finding significant variability in the type and proportion of the mechanisms on total thermogenic capacity. While in some South American Ctenomyid species, a combination of NST and ST is observed (e.g., C. porteusi), in other species, only ST is present (C. tuconax; Luna et al., 2019). Similarly, in African bathyergids, the cost of NST varies depending on the body mass and sociality (Luna et al., 2021). However, no studies have been performed to evaluate the ontogeny of total thermogenic capacity and the nature of thermogenic mechanisms involved in pups of subterranean or fossorial species.
The genus Ctenomys is distributed in several environments in the southern cone of South America, being one of the most speciose genera among subterranean and fossorial rodents, including species from ∼100 g (C. pundti) to more than 1,000 g (C. conoveri; Reig et al., 1990). Particularly, Ctenomys talarum is a solitary fossorial rodent that lives in the coastal grasslands of Argentina (Antenucci and Busch, 1992). Similar to other Ctenomys species, tunnel systems show a linear pattern with no differences in total burrow length between sexes, feeding mainly on aerial plant parts near the burrow openings (Antenucci and Busch, 1992; Comparatore et al., 1995). Males of C. talarum established a dominance hierarchy among them, monopolizing mating activity, and consequently, dominant male burrows occupied central distribution surrounded by several female burrows (Busch et al., 1989; Antenucci and Busch, 1992). The reproductive season lasted ~9 months, beginning in June–July (austral winter) and continuing until February (austral summer). Birth takes place from August through early December after a long gestation period (~100 days; Malizia and Busch, 1991; Fanjul et al., 2006). Females produced as many as two litters per reproductive season, with a mean litter size of 4 (Busch et al., 1989). For C. talarum, the cost of acceding to new food patches made through the construction of new tunnel systems is relatively high (Luna et al., 2002).
During the ontogeny, C. talarum pups show an initial altricial phase, represented by a period of high thermal vulnerability. During lactation (~10 days), pups are not capable of maintaining Tb on their own, depending, therefore, on their mother to thermoregulate. After this period, pups enhance their thermoregulatory capacity and start eating solid food. At approximately 30–40 days after birth, pups reach the Tb of adults and become behaviorally and physiologically independent. At this age, the weaning period starts, and pups show active exploratory behavior. Around day 60, solitary pups disperse aboveground from their maternal burrow and construct their burrow (Malizia et al., 1995; Zenuto et al., 2002; Antenucci et al., 2003; Cutrera et al., 2003; Baldo et al., 2014). In this context, the aim of this study was to evaluate the thermogenic capacity development of pups of the fossorial rodent Ctenomys talarum. We also evaluated the presence of BAT during ontogeny and the timing of mitochondrial uncoupling proteins related to BAT-mediated NST. We hypothesized that no thermogenic capacity is attained during the altricial phase of their development (~day 10), increasing their thermogenic capacity after weaning, before the dispersal period. Furthermore, we expect that NST is completely functional after day 10, reaching the value of the adult during this period.
Materials and methods
Animal capture and husbandry
Pregnant females were live-trapped in Mar de Cobo (37°45’S, 57°56’W, Buenos Aires Province, Argentina). In the laboratory, females were housed in individual cages (0.30 × 0.40 × 0.25 m), with wood shavings as nesting material. A total of 27 pregnant females were used in this study. The animal room was kept at 25 ± 1°C, and the photoperiod was LD 12:12 (lights on at 7.00 a.m.). Animals were fed with mixed native grasses, carrots, lettuce, corn, alfalfa, and sunflower seeds ad lib. As C. talarum does not drink free water, it was not provided. Pregnant females were monitored daily until pups were born. After birth, pups were kept in the same cage with their mother and siblings until day 60, after which the remaining pups were placed in individual boxes until they were released in the field. On days 2, 6, 10, 15, 30, and 60 after parturition, 1 pup from a randomly assigned mother was weighed, and metabolic variables and Tb were measured. To avoid possible maternal effects during the experiment, only one pup of each litter on each day was used in NSTmax and MMR trials. Due to the difficulty in maintaining pregnant females and obtaining parturition in the laboratory, we used 4 pups from 4 different females that delivered more than 4 pups. In this case, 1 pup was used between days 2 and 6, and another between days 30 and 60. A total of 31 pups were used to estimate the pattern of NSTmax and MMR. The sex of the pups in the experimental design was not considered, using both males and females independently, because sex cannot be stablished externally in the early stages of development. Given that our primary focus was thermogenic capacity, we used another set of pups (7 pups from different litters) for the RMR experiment. This set of pups was maintained in the same husbandry conditions and was related to an undergoing study on thermoregulatory physiology.
Resting metabolic rate and non-shivering thermogenesis of pups
Oxygen consumption was measured using a computerized positive pressure open-flow respirometry system (Sable System, United States). A cylindrical chamber (volume 500 mL) was used to estimate O2 consumption. Chamber was placed inside an incubator (Simedix, model I-501, Ingelab, Argentina). Ambient temperature (Ta) was maintained with an accuracy of 0.2°C. The chamber received dry and CO2-free air ranging from 300 mL min−1 to 600 mL min−1, depending on the body mass of the pups, from a mass-flowmeter (Side-Trak Sierra, model 830/840, Sierra Instruments, United States). To scrub CO2 and water, the air was passed through a CO2 absorbent (self-indicating soda lime, Laboratorios IQB, Argentina) and water scrubber (Drierite, W. A. Hammond Drierite Company, United States) before and after passing through the chamber. Excurrent air from the chamber was subsampled at 80 ± 10 mL min−1, and oxygen consumption was obtained from an Oxygen Analyzer FC-1B every 1 s by an EXPEDATA data acquisition program (Sable System, United States). Rates of oxygen consumption were calculated using equation 4a of Withers (1977). All metabolic trials were performed between 9:00 and 17:00 h. The body mass of individuals (M) was measured before each metabolic trial using an electronic balance (model FX-3000, ± 0.01 g, A&D Company Limited, United States), and the pharyngeal temperature was recorded at the beginning and the end of each experiment with a YSI probe (model 93 k73545-402) connected to a Cole-Parmer thermistor meter (model 8,402–10, ± 0.1°C, Cole-Parmer Instrument Company, United States).
Resting metabolic rate was estimated as the lowest 5–8 min steady-state O2 consumption of a total trial period of 35–40 min. A total trial period was chosen because C. talarum pups are highly dependent, during the early stage of their development, on mother and sibling contact (Zenuto et al., 2002), showing signs of hypothermia if pups are alone for more than 30 min (Cutrera et al., 2003). However, brief isolation did not affect the development of thermoregulatory physiology (Baldo et al., 2014). In addition, total chamber volume and air flow allowed a short equilibration period of the chamber (2–4 min to achieve 90% of equilibration, depending on the air flow; Lasiewski et al., 1966). After being placed in the metabolic chamber, pups showed an exploratory behavior, which was performed during the period in which the metabolic chamber was equilibrating (~5 min).
Maximal non-shivering thermogenesis (NSTmax) was estimated using the same chamber and protocol used to estimate RMR, but after intramuscular injection of norephinefrine (NE). In eutherians, the O2 consumption in response to NE occurs 10 min after the injection and lasts at least 5–10 min (Feist and Rosenmann, 1976). Doses of NE were estimated according to Wunder and Gettinger (1996) and described as NE (mg Kg−1) doses = 2.53 M-0.4. Doses of NE and procedures were validated for adults of this species (Luna et al., 2012). The maximum 10-min steady-state oxygen consumption after the injection of NE was considered to be NSTmax, which includes both RMR and thermoregulatory NST (Wunder and Gettinger, 1996). To avoid unnecessary long-time exposition in pups, we did not assess the effect of injection on pups. Values of O2 consumption of adults between the control group and after saline solution injection were similar (Luna et al., 2012). For RMR and NSTmax estimations, the metabolic chamber was maintained at Ta of 25°C, which is within the thermoneutral zone of adults for this species (Busch, 1989).
Cold-induced maximum metabolic rate
Maximum thermogenic metabolism was estimated in a HELOX atmosphere according to the procedure described by Rosenmann and Morrison (1974). A mixture of He (79%) and O2 (21%) was passed through a mass flowmeter before entering the metabolic chamber (Side-Trak, Sierra model 830/840, Sierra Instruments, United States). Before MMR estimation, the flow rate was corrected for the He-O2 gas mixture. As described before, the mixture was passed through a CO2-absorbent and water scrubber before and after passing through the chamber. Oxygen consumption of pups was recorded for a 30–40 min period at an ambient temperature of 19°C. This Ta was chosen based on previous studies that determined the lowest Ta within burrows during the reproductive period when pups are born (Cutrera and Antinuchi, 2004) and because pups fall into deep hypothermia even when there are huddling (Cutrera et al., 2003).
Shivering thermogenesis
Shivering thermogenesis (ST) was estimated for each pup according to the equation proposed for eutherian mammals (MMR = BMR + NST + ST; Wunder and Gettinger, 1996). Experimentally, ST was estimated as O2 consumption after cold-induced minus NSTmax.
Measurement of UCP1 and COXII content and COX activity
After NSTmax and MMR, pups were euthanized, and interscapular brown adipose tissue (iBAT) was carefully dissected, frozen in liquid nitrogen, and stored at −80°C. All other main organs were also frozen and stored at −80°C for further analysis. We adhered to the 2012 Revised International Guiding Principles for Biomedical Research Involving Animals developed by the Council for International Organizations of Medical Sciences (CIOMS) and the International Council for Laboratory Animal Science (ICLAS). Pups used in RMR experiments were released at the capture site after day 120. Interscapular BAT was homogenized with a manual homogenizer in STE buffer (250 mM sucrose, 5 mM Tris–HCl, 2 mM EDTA, pH 7.4) with protease and phosphatase inhibitors (10 μM Leupeptin, 10 μM Pepstatin, 0.2 mM PMSF, and 0.2 mM Orthovanadate) in a proportion of 10 mL of buffer per gram of tissue and filtered through a layer of inert gauze. Total protein content was determined using the Bradford method (Bradford, 1976). Samples of iBAT were denatured, and 40 μg of proteins per line were loaded and run in an SDS-PAGE (3% stacking gel and 12% running gel), according to Laemmli (1970), and electrotransferred onto a nitrocellulose filter, as described by Puigserver et al. (1991), using a Trans-Blot® Turbo™ Transfer System (Bio-Rad, United States). After that, membranes were blocked in 5% nonfat powdered milk in TBS-Tween and incubated with the corresponding antibodies (UCP1, COXII antibodies, Alpha Diagnostics, United States). Protein bands on the nitrocellulose filters were visualized by Immun-Star® Western C® Kit reagent (Bio-Rad, United States) using the Western blotting detection systems. The chemiluminiscence signal was captured using a Chemidoc XRS densitometer (Bio-Rad, United States) and analyzed with Quantity One Software (Bio-Rad, United States). COX activity was measured using a spectrophotometric method (Wharton and Tzagoloff, 1967; Chrzanowska-Lightowlers et al., 1993). Briefly, aliquots of the iBAT homogenates were incubated in 0.1 M NaPO4H2, pH 7.0, in the presence of 2 μg/mL Catalase and 5 mM substrate DAB (3, 3′ diaminebenzidine-tetrachloride). After 30 s, 100 μM reduced cytochrome c was added to start the reaction, and the absorbance variation was recorded for 20 min at 450 nm.
Statistics
Physiological variables (NSTmax, MMR, and RMR) were analyzed by linear mixed-effects model with the R package “nlme” (Pinheiro et al., 2022) in R (R Core Team, 2021) inside RStudio (R Studio Team, 2020). To account for repeated measurements, individual IDs were included as a random factor when comparing NSTmax and MMR. Body mass was included as a covariate in the model. Assumptions were verified using residual plots and tested for normality of residuals by the Shapiro–Wilk test. To evaluate each fixed factor (treatment and day), we used hypothesis tests with a significance level set to α = 0.05 for all analyses. To explore changes in metabolic rate, the variance correction function varIdent was used. We performed a general linear mixed model with heterogeneous variance for the absolute Tb obtained before metabolism measurement (cold-induced or NE injection) with the same fixed and random effects as the models detailed above, without using body mass as a covariate. To compute p-values for multiple comparisons, the R package “emmeans” (Lenth, 2022) was used when we found significant differences between means. For ΔTb, we used linear models to test for differences between days in each experimental condition (cold-induced or after NE injection). In addition, linear models were used to evaluate differences in the enzymatic activity of COX between days. Finally, we used the Kruskal-Wallis test for differences in protein content (UCP1 and COXII) in iBAT. Linear models and the Kruskal-Wallis tests were performed in the R package “R stats” in R (R Core Team, 2021) inside R Studio (R Studio Team, 2020).
Results
When RMR and NSTmax were compared, MR was affected by age (F5,66 = 108.28, p < 0.001) and treatment (F1,66 = 33.95, p < 0.001). However, no significant effects of the interaction between age and treatment (F5,66 = 1.92, p = 0.1) were found (Figure 1; Table 1). There is an increment in MR from day 2 until day 10 [P(day 2–day 10) = 0.008, P(day 6–day 10) < 0.001]. When pups were older than day 10, age affected MR [P(day 10–day 30) < 0.005, P(day 15–day 30) = 0.01]. Moreover, differences in MR were found between day 6 and day 30 [P(day 6–day 30) < 0.005]. When MMR and NSTmax were compared, we found age differences (F5,31 = 93.21, p < 0.001) and treatment (F1,30 = 36.01, p < 0.001). The interactions between age and treatment were also different (F5,30 = 11.55, p < 0.001, Figure 1; Table 1). There is an increment in MMR from day 2 to day 10 [P(day 2–day 10) = 0.01, P(day 6–day 10) = 0.006], with values of MMR between day 2 and day 6 being similar (p = 0.99). After day 10, MMR was different from the following days [P(day 10–day 15) = 0.99, P(day 10–day 30) = 0.99, P(day 10–day 60) = 0.81]. After day 15, due to the relatively lower value at day 30, MMR was different only from day 60 [P(day 15–day 30) = 0.99, P(day 15–day 60) = 0.004, P(day 30–day 60) = 0.002]. When comparing MMR with NSTmax, differences between days were observed. In this sense, MMR was higher than NSTmax at day 15 (p = 0.002), day 30 (p = 0.049), and day 60 (p < 0.001). Similarly, as no differences were observed between RMR and NSTmax, MMR was different from RMR between days (F5,66 = 168.05, p < 0.001), treatment (F1,66 = 124.13, p < 0.001), and the interaction between days and treatment (F5,66 = 45.48, p < 0.001, Figure 1; Table 1). MMR was higher than RMR at day 15 (p = 0.002), day 30 (p = 0.049), and day 60 (p < 0.001). For comparative purposes, in Figure 2A, physiological variables were expressed in mass-specific MR.
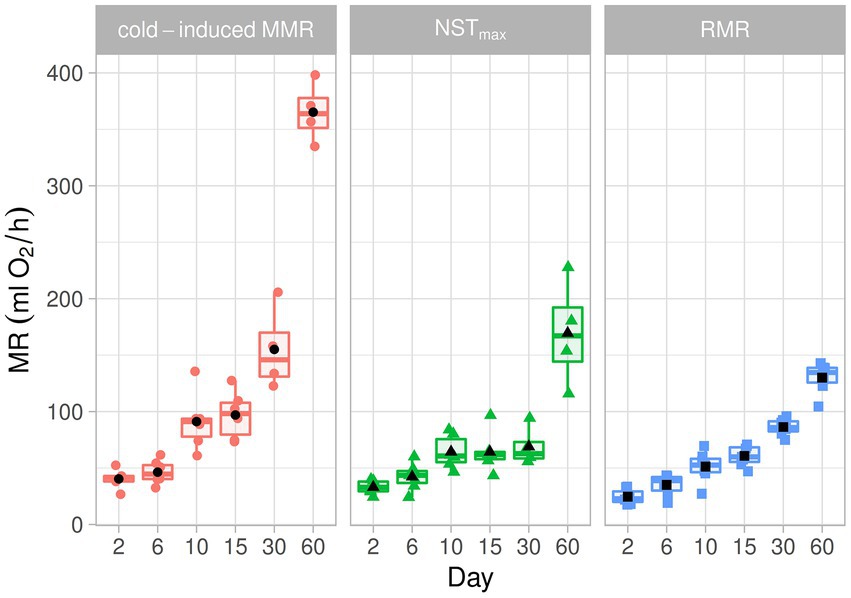
Figure 1. A box plot of metabolic rate in different experimental conditions during postnatal development in the fossorial rodent Ctenomys talarum. The lower and upper boxes are the 25th and 75th percentiles, respectively. The line inside the box is the median, and the black symbol is mean. The lower and upper error lines are the 10th and 90th percentiles, respectively.
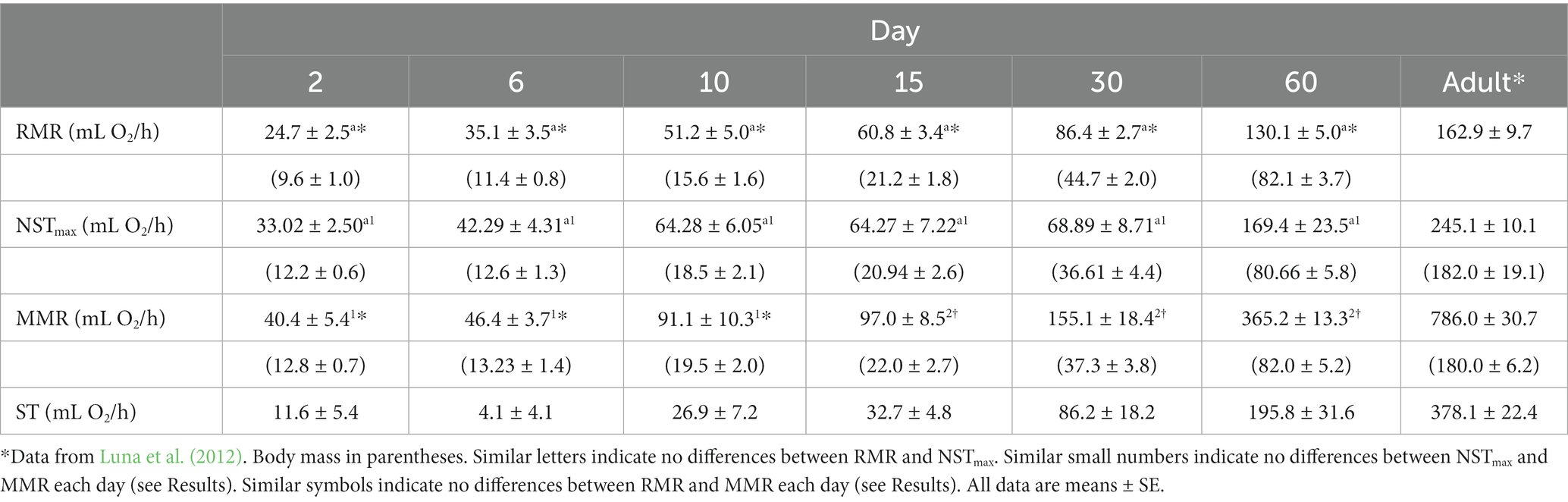
Table 1. Resting metabolic rate (RMR), maximal non-shivering thermogenesis (NSTmax), shivering thermogenesis (ST), and cold-induced maximum metabolic rate (MMR) during postnatal development in the fossorial rodent Ctenomys talarum.
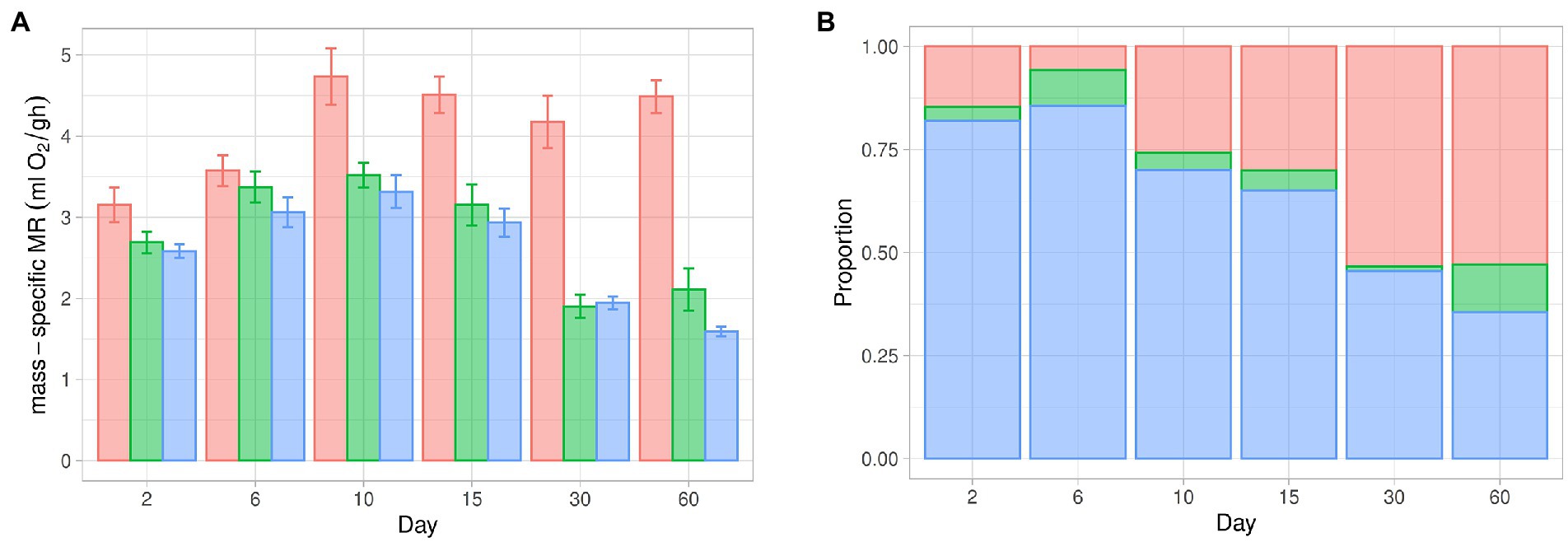
Figure 2. Thermogenic capacity during postnatal development in the fossorial rodent Ctenomys talarum. (A) Mass-specific metabolic rate in different experimental conditions. Red: cold-induced maximum metabolic rate (MMR). Green: maximal non-shivering thermogenesis (NSTmax). Blue: resting metabolic rate (RMR). (B) Relative contribution of non-shivering thermogenesis (NST), shivering thermogenesis (ST), and resting metabolic rate (RMR) to cold-induced maximum metabolic rate (MMR).
When comparing Tb before the exposure to cold conditions to estimate MMR or NE injection, neither the exposure alone nor their interaction with age was different (F1,31 = 1.49, p = 0.23 and F5,31 = 1.52, p = 0.21, respectively), whereas Tb between days was different (F1,31 = 17.64, p < 0.001; Table 2). Body temperature at day 60 was higher than those observed at days 2, 6, 10, and 15 (all, p < 0.001) and also Tb at day 30 compared to day 2 (p = 0.01) and day 6 (p = 0.03). After cold exposure, ΔTb was similar between days (F5,31 = 0.88, p = 0.51). After NE injection, ΔTb on day 2 was lower than ΔTb on all other days (F5,31 = 8.58, p < 0.001; Table 2). Interscapular BAT mass increased linearly with body mass. The relationship of iBAT mass with body mass was iBAT mass = 0.009 M – 0.011 (R2 = 0.89, F1,39 = 293.25, p < 0.001, Figure 3). UCP1 and COXII content in iBAT were similar between days (T = 5.88, df = 5, p = 0.32 and T = 8.96, df = 5, p = 0.11, respectively; Table 3). The activity of the COX complex in iBAT was similar at all ages (F5,32 = 1.12, p = 0.37, Table 3).
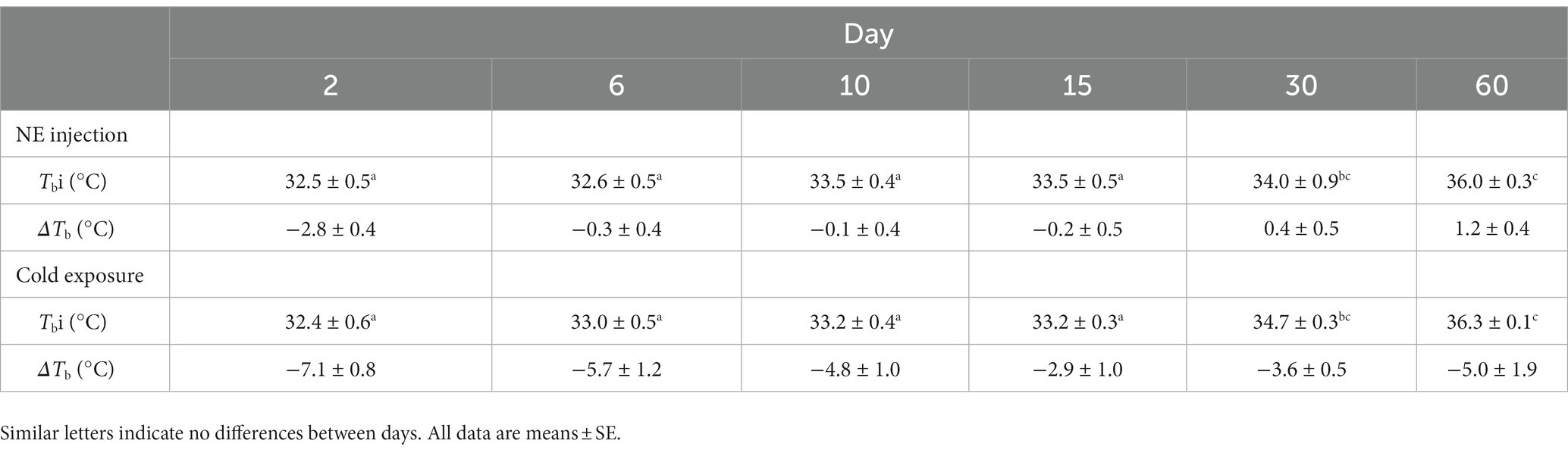
Table 2. Body temperature (Tb) and ΔTb after norepinephrine (NE) injection after cold exposure during postnatal development in the fossorial rodent Ctenomys talarum.
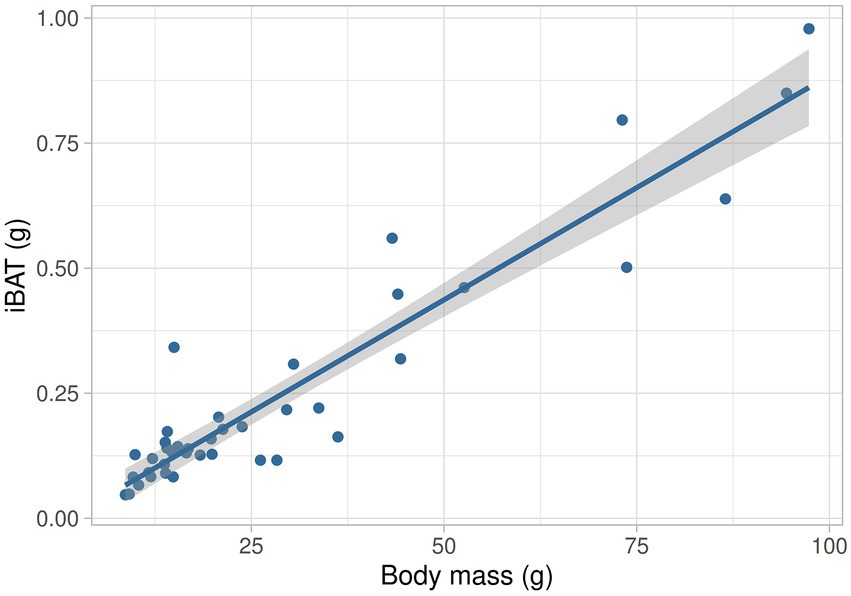
Figure 3. Relationship between the mass of interscapular brown adipose tissue patch (iBAT) and body mass during postnatal development in the fossorial Ctenomys talarum. The solid line represents the regression line.
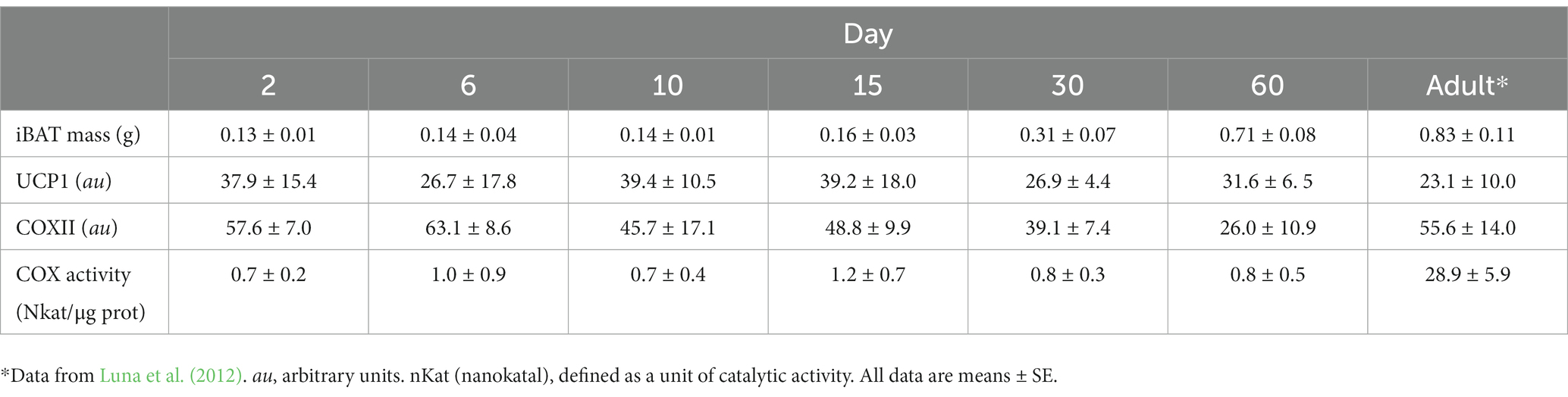
Table 3. Content of uncoupling protein I (UCP1) and cytochrome c oxidase II (COXII) and activity of COX in interscapular brown adipose tissue (iBAT) during postnatal development in the fossorial rodent Ctenomys talarum.
Discussion
To attain thermal independence before the dispersal period, pups must fully acquire thermoregulatory capacity (Hill, 1992). The main objective of this study was to determine the thermogenic development of pups of the fossorial C. talarum, stablishing the onset of thermogenic capacity and its components. To the best of our knowledge, this is the first study to establish the postnatal development of non-shivering thermogenesis (NST) and cold-induced maximum metabolic rate (MMR) in a fossorial species. Pups of C. talarum showed a biphasic pattern of mass-specific RMR during their development. Mass-specific RMR increases until day 10, with a subsequent decline to day 60, confirming the pattern found by Zenuto et al. (2002). In this species, acquisition of thermoregulatory capacity takes days, since the onset of homeothermy is observed around day 30, a period in which pups showed Tb of adults (Zenuto et al., 2002; Cutrera et al., 2003; Baldo et al., 2014). Notably, no thermogenic mechanism is observed until day 10, and NSTmax does not differ from RMR up to day 60.
In altricial species, it has been described that the first thermogenic mechanism to appear is NST (Robertson and McClelland, 2019; Lezama-García et al., 2022), involving the maturation of BAT a few days after birth (Robertson et al., 2019). In pups of the golden hamster Mesocricetus auratus, the onset of NST occurs around 14 days after birth, whereas in the Djungarian hamster Phodopus campbelli, the onset of NST occurs after day 9 (Newkirk et al., 1995). Despite the high metabolic activity of BAT, the common rat showed a weak NST on day 5 after birth (Spiers and Adair, 1986). In the golden Syrian hamster Mesocricetus auratus, thermogenic capacity develops within a week after birth and is determined by qualitative changes in mitochondrial function related to the initiation of UCP synthesis (Houštěk et al., 1990). In newborn rats, NST capacity appears after weaning, but UCP1 is detectable in iBAT just after birth, reaching its maximum value on day 10 (Porras et al., 1990). Interestingly, C. talarum pups showed a complete absence of NST until day 60, but this does not imply the complete absence of BAT. Interscapular BAT patch is directly recognizable, increasing in size with age but never exceeding 1% of the body mass. Neonatal exposure to cold can establish modifications in NST capacity, induced by changes in neuronal stimulation and content of UCP in BAT (Morrison et al., 2000; Sant Anna and Mortola, 2003). However, even though pups of C. talarum can be exposed to cold when isolated from the mother and siblings, UCP1 content does not vary between days. In the same way, different molecular markers of the oxidative capacity of BAT ensure NST (Klingenspor, 2003) does not vary between days. For example, the activity of cytochrome c oxidase (COX), a marker enzyme for the mitochondrial membrane, and the content of COXII, commonly used to estimate respiratory capacity in BAT mitochondria (Klaus et al., 1988; Klingenspor et al., 1996), remain similar between days. Although unlikely, given the relative thermal stability of burrows, it would be worth establishing whether acclimatization to low Tas by mothers might affect the magnitude of pup’s thermogenesis, as has been observed in the surface-dwelling altricial leaf-eared mouse Phyllotis darwini during the first days of life (Canals et al., 2009).
Day 60 corresponds to the beginning of the period of dispersion of this species, which occurs on the surface (Malizia et al., 1995). Interestingly, the size of iBAT and the amount of UCP1, as well as markers of the oxidative capacity of BAT in adults, are similar to that in juveniles (Luna et al., 2012). Due to the complete maturation of endocrine and neural pathways (Symonds et al., 2012; Symonds, 2013; Lezama-García et al., 2022), changes in the efficiency of BAT-related NST might establish the functional NST after day 60. Complete maturation of neuro-endocrine pathways would allow an increased capacity for fatty acid oxidation and tricarboxylic acid cycle activity to uncouple oxidative phosphorylation to produce heat (Chouchani et al., 2019). For example, in P. maniculatus, a delay in the onset of NST in pups reared at high altitude was associated with a delay in the maturation of nervous and O2 supply systems (Robertson et al., 2019; Robertson and McClelland, 2019; Velotta et al., 2020).
As proposed, ST matures later than NST because of the slow development of muscles (Robertson and McClelland, 2019; Lezama-García et al., 2022). In C. talarum pups, shivering was characteristically observed over the first days (Baldo et al., 2014) but appeared to be fully functional after day 15. Aerobic-resistant muscles that generate repeated contractions could be mainly recruited to produce heat; thus, the proportion of this type of muscle can establish ST potential (Hohtola, 2004). Muscles of altricial rodents are relatively immature at birth, and they mature due to changes in muscle fiber structure and hypertrophy (White et al., 2010). From day 10, offspring of C. talarum start to show exploratory behaviors, wandering the tunnel systems and beginning to scratch and push the soil (Vassallo, 2006; Echeverría et al., 2016). The progression of digging behavior has particular energetic requirements to loosen, remove, and break down the soil to construct a new burrow during dispersal. Similar to ST, aerobic-resistant muscles are used during digging activities (Alvarez et al., 2004). Thus, muscular work performed during digging could also be exploited as a source of heat via ST (Luna and Antinuchi, 2007). The onset of functional ST overlaps with the beginning of the exploration of tunnels near the thermally stable nest, tunnels that usually show Tas below TNZ (Cutrera and Antinuchi, 2004). In this context, even though it is not clear how muscle maturation occurs during the first days of life, the capacity for ST can be associated with an increase in digging capacity. Furthermore, cold acclimation in adult rodents was proposed to lead to muscle training and an increase in ST capacity (Shefer and Talan, 1998). As digging becomes more efficient, ST might also do so. Interestingly, from day 15 until dispersal, total thermogenic capacity relies entirely on ST, increasing its specific energetic cost due to the progressive decrease in mass-specific RMR and the constancy of total thermogenic capacity.
The total thermogenic capacity of pups from day 10 is similar to those observed in adults (Luna et al., 2012). The consistency of mass-specific MMR over the lifespan of this species addresses important questions about the mechanistic meaning of physiological adjustments within upper and lower metabolic limits. A fixed metabolic ceiling determines that any energetic modification must be within the aerobic scope. The net aerobic scope represents the capacity of aerobic processes above minimal metabolism (see Nespolo et al., 2017 for a discussion). For example, unlike juveniles, adults of C. talarum show a functional NST that represents ~40% of the total thermogenic capacity (Luna et al., 2012). Thus, from day 10, when the metabolic ceiling is established, there is an increase in the net aerobic scope given the progressive decrease in mass-specific RMR. Any energy input intended for other processes, in addition to growth, must be within metabolic limits. In fact, the part of the energy cost of NST in adults could be related to the difference between mass-specific RMR at day 60 (1.75 mL O2/gh; this study) and in adults (0.89 mL O2/gh; Luna et al., 2012). Such metabolic trade-offs between different energy-consuming mechanisms might ensure a balanced daily energy expenditure, hence survival. Maximal metabolism is an ecologically relevant trait (Bozinovic and Rosenmann, 1989), and although it appears to be infrequently reached during life, maximal metabolism can be affected by natural selection (Rezende et al., 2004). It is not the focus of this study to evaluate which factor determines mass-specific MMR constancy, but it has been proposed that there would have been an evolutionary limitation on reaching high MMR values (Luna et al., 2012, 2019) because of limitations in respiratory and cardiovascular systems imposed by burrow’s atmosphere (i.e., low O2 and high CO2). Therefore, the value of maximal metabolism might be independent of the physiological mechanism that elicits it. In adults of C. talarum, cold-induced MMR also does not vary after acclimation to cold, being similar to exercise-induced MMR (see Luna et al., 2012). As proposed, further studies are needed to assess the consequences of fixed ceilings and net aerobic scope in subterranean and fossorial species when compared to surface-dwelling species (Luna et al., 2015, 2019).
Heat balance in endotherms results from the integration of a coordinated system of heat production and loss (Naya et al., 2013). One of the main characteristics of underground rodents is their high thermal conductance (see McNab, 1966). Thus, not only changes in thermogenic capacity but also thermal insulation are important to maintaining thermal homeostasis (Withers et al., 2016). Early development in altricial pups represents a stage of dependence on external thermal sources (MacArthur and Humphries, 1999; Antinuchi and Busch, 2001; Zepeda et al., 2018), which mostly depends on thermal resources provided by the mother and behavioral thermoregulation, such as huddling with their siblings (Zenuto et al., 2002). The effectiveness of huddling depends not only on the reduction of the individual’s exposed surface but also on the augmented heat transfer between littermates and the mother (Webb et al., 1990; Gilbert et al., 2007). In the altricial P. darwini, a strong maternal effect on the thermoregulatory development of pups was observed until day 7 (Canals et al., 2009). McClure and Randolph (1980) found in the eastern wood rat Neotoma floridana pups that poor insulation after birth is related to the maximization of heat transfer. In C. talarum, as no thermogenic mechanisms are observed until day 10, behavior can alleviate the need for these energetically costly mechanisms. Until day 10, hairless pups spend most of the time in contact with their mother and siblings (Cutrera et al., 2003; Baldo et al., 2014). Zenuto et al. (2002) established that the increase in mass-specific RMR until day 10 was related to the increase in fur coverage and the increase in the intake of solid food after day 7. After day 10, pups remained huddled for shorter periods of time due to the increment of agonistic aggressive behavior between them (Cutrera et al., 2003; Baldo et al., 2014). Thus, the onset of ST also coincides with the time that pups start to spend less time huddled and become furrier, increasing their insulation. Therefore, thermoregulatory benefits associated with the underground environment might allow a short period where behavioral strategies are important to avoid thermogenic costs. During lactation, pups might invest energy to accelerate the production of new tissue and its maintenance (Hill, 1992), without compromising their thermal balance. The thermally stable environment of the nest and behavior might lead to a delay in the onset of ST, hence independent thermoregulation, until the period in which pups find new thermal environments by exploring and digging nearby tunnels.
In conclusion, although pups of C. talarum were altricial at birth (Zenuto et al., 2002; Cutrera et al., 2003; Baldo et al., 2014), they maintained behaviorally their thermal balance during lactation. Until day 10, pups are dependent on their mother to obtain heat, remaining in the nest where they commonly huddle with their littermates (Cutrera et al., 2003; Baldo et al., 2014). During this period, resting metabolism increases, but no thermogenic mechanisms were observed. As most of the energy is destined for growth, pups at this age showed impossibility to maintain Tb when confronted with Tas below adults TNZ. From day 10, mass-specific RMR showed a progressive decrease and MMR became fixed, establishing a specific increment of ST until day 60. Postnatal development of musculature related to digging could be employed as a heat source when pups start to explore and dig in the surrounding of the nest, but it appears to be inefficient until day 30 when pups achieved adult Tb. No functional NST was observed in pups until day 60 besides the presence of UCP1 and other molecular markers (e.g., COX activity) of NST in BAT. Attaining complete thermal independence is critical after day 60 since juveniles disperse at this age (Malizia et al., 1995). During their aboveground dispersal, juveniles must dig a new burrow or eventually find an empty burrow, so they are exposed to aboveground climatic fluctuations. Interestingly, in adults, NST complies with around 40% of the total thermogenic capacity (Luna et al., 2012), so, at some point during or after dispersal, NST becomes functional.
Data availability statement
The raw data supporting the conclusions of this article will be made available by the authors, without undue reservation.
Ethics statement
The animal study was reviewed and approved by the Institutional Committee for the Care and Use of Laboratory Animals (number 2555-06-14 RD141/15; CICUAL), Facultad de Ciencias Exactas y Naturales (FCEYN), and Universidad Nacional de Mar del Plata (UNMDP).
Author contributions
FL designed the study. CDA supported the respirometric experimental procedures. JS-S and JO supported the molecular experimental procedures. FL and CDA analyzed the data, prepared the manuscript, and created figures and tables. All authors contributed to the article and approved the submitted version.
Funding
This project was supported by the Consejo Nacional de Investigaciones Científicas y Técnicas of Argentina (CONICET, PIP3164) and the Agencia Nacional de Promoción de la Investigación, el Desarrollo Tecnológico y la Innovación (Agencia I+D+i, PICT 3785).
Acknowledgments
We are grateful to the members of the Laboratorio de Ecología Fisiológica y del Comportamiento (IIMyC, CONICET-UNMdP) for their support and assistance in the field. We are also grateful to Guadalupe Luna for helping us with the edition and language revision of an earlier version of the manuscript.
Conflict of interest
The authors declare that the research was conducted in the absence of any commercial or financial relationships that could be construed as a potential conflict of interest.
Publisher’s note
All claims expressed in this article are solely those of the authors and do not necessarily represent those of their affiliated organizations, or those of the publisher, the editors and the reviewers. Any product that may be evaluated in this article, or claim that may be made by its manufacturer, is not guaranteed or endorsed by the publisher.
References
Alvarez, G. I., Vassallo, A. I., and Diaz, A. O. (2004). Morphometry of muscle fiber types of a burrowing mammal (Ctenomys talarum). Biocell 28:237. doi: 10.1111/j.1439-0264.2012.01137.x
Antenucci, C. D., and Busch, C. (1992). Burrow structure in the subterranean rodent Ctenomys talarum. Z. Für Säugetierkd. 57, 163–168.
Antenucci, C. D., Cutrera, A. P., Luna, F., and Zenuto, R. R. (2003). “Animales y temperatura: estrategias termorregulatorias durante la ontogenia” in Fisiología ecológica y evolutiva: teoría y casos de estudios en animales. ed. F. Bozinovic (Santiago, Chile: Universidad Católica de Chile), 399–420.
Antinuchi, C. D., and Busch, C. (2001). Reproductive energetics and thermoregulatory status of nestlings in pampas mice Akodon azarae (Rodentia: Sigmodontinae). Physiol. Biochem. Zool. 74, 319–324. doi: 10.1086/320416
Baldo, M. B., Luna, F., Schleich, C. E., and Antenucci, C. D. (2014). Thermoregulatory development and behavior of Ctenomys talarum pups during brief repeated postnatal isolation. Comp. Biochem. Physiol. A Mol. Integr. Physiol. 173, 35–41. doi: 10.1016/j.cbpa.2014.03.008
Begall, S., Burda, H., and Schleich, C. E. (2007). Subterranean Rodents: News from Underground. Berlin: Springer.
Berthon, D., Herpin, P., and Le Dividich, J. (1994). Shivering thermogenesis in the neonatal pig. J. Therm. Biol. 19, 413–418. doi: 10.1016/0306-4565(94)90040-X
Bozinovic, F., and Rosenmann, M. (1989). Maximum metabolic rate of rodents: physiological and ecological consequences on distributional limits. Funct. Ecol. 3:173. doi: 10.2307/2389298
Bradford, M. M. (1976). A rapid and sensitive method for the quantitation of microgram quantities of protein utilizing the principle of protein-dye binding. Anal. Biochem. 72, 248–254. doi: 10.1016/0003-2697(76)90527-3
Brück, K., and Hinckel, P. (1996). “Ontogenetic and adaptive adjustments in the thermoregulatory system” in Comprehensive Physiology. ed. R. Terjung (Hoboken, NJ: Wiley), 597–611.
Burda, H., Šumbera, R., and Begall, S. (2007). “Microclimate in burrows of subterranean rodents — revisited” in Subterranean Rodents. eds. S. Begall, H. Burda, and C. E. Schleich (Berlin, Heidelberg: Springer), 21–33.
Busch, C. (1989). Metabolic rate and thermoregulation in two species of tuco-tuco, Ctenomys talarum and Ctenomys australis (Caviomorpha, Octodontidae). Comp. Biochem. Physiol. A 93, 345–347. doi: 10.1016/0300-9629(89)90048-0
Busch, C., Malizia, A. I., Scaglia, O. A., and Reig, O. A. (1989). Spatial distribution and attributes of a population of Ctenomys talarum (Rodentia: Octodontidae). J. Mammal. 70, 204–208. doi: 10.2307/1381691
Canals, M., Figueroa, D. P., Miranda, J. P., and Sabat, P. (2009). Effect of gestational and postnatal environmental temperature on metabolic rate in the altricial rodent, Phyllotis darwini. J. Therm. Biol. 34, 310–314. doi: 10.1016/j.jtherbio.2009.04.003
Cannon, B., and Nedergaard, J. (2004). Brown adipose tissue: function and physiological significance. Physiol. Rev. 84, 277–359. doi: 10.1152/physrev.00015.2003
Chouchani, E. T., Kazak, L., and Spiegelman, B. M. (2019). New advances in adaptive thermogenesis: UCP1 and beyond. Cell Metab. 29, 27–37. doi: 10.1016/j.cmet.2018.11.002
Chrzanowska-Lightowlers, Z. M. A., Turnbull, D. M., and Lightowlers, R. N. (1993). A microtiter plate assay for cytochrome c oxidase in Permeabilized whole cells. Anal. Biochem. 214, 45–49. doi: 10.1006/abio.1993.1454
Comparatore, V. M., Cid, M. S., and Busch, C. (1995). Dietary preferences of two sympatric subterranean rodent populations in Argentina. Rev. Chil. Hist. Nat. 68, 197–206.
Cutrera, A. P., and Antinuchi, C. D. (2004). Cambios en el pelaje del roedor subterráneo Ctenomys talarum: posible mecanismo térmico compensatorio. Rev. Chil. Hist. Nat. 77:3. doi: 10.4067/S0716-078X2004000200003
Cutrera, A. P., Antinuchi, C. D., and Busch, C. (2003). Thermoregulatory development in pups of the subterranean rodent Ctenomys talarum. Physiol. Behav. 79, 321–330. doi: 10.1016/S0031-9384(03)00116-1
Echeverría, A. I., Biondi, L. M., Becerra, F., and Vassallo, A. I. (2016). Postnatal development of subterranean habits in tuco-tucos Ctenomys talarum (Rodentia, Caviomorpha, Ctenomyidae). J. Ethol. 34, 107–118. doi: 10.1007/s10164-015-0453-5
Fanjul, M. S., Zenuto, R. R., and Busch, C. (2006). Seasonality of breeding in wild tuco-tucos Ctenomys talarum in relation to climate and food availability. Acta Theriol. 51, 283–293. doi: 10.1007/BF03192680
Farrell, W. J., and Alberts, J. R. (2007). Rat behavioral thermoregulation integrates with nonshivering thermogenesis during postnatal development. Behav. Neurosci. 121, 1333–1341. doi: 10.1037/0735-7044.121.6.1333
Feist, D. D., and Rosenmann, M. (1976). Norepinephrine thermogenesis in seasonally acclimatized and cold acclimated red-backed voles in Alaska. Can. J. Physiol. Pharmacol. 54, 146–153. doi: 10.1139/y76-023
Foster, D. O., and Frydman, M. L. (1979). Tissue distribution of cold-induced thermogenesis in conscious warm- or cold-acclimated rats reevaluated from changes in tissue blood flow: the dominant role of brown adipose tissue in the replacement of shivering by nonshivering thermogenesis. Can. J. Physiol. Pharmacol. 57, 257–270. doi: 10.1139/y79-039
Geiser, F., and Kenagy, G. J. (1990). Development of thermoregulation and torpor in the Golden-mantled ground squirrel Spermophilus saturatus. J. Mammal. 71, 286–290. doi: 10.2307/1381938
Gilbert, C., Blanc, S., Giroud, S., Trabalon, M., Maho, Y. L., Perret, M., et al. (2007). Role of huddling on the energetic of growth in a newborn altricial mammal. Am. J. Physiol. Regul. Integr. Comp. Physiol. 293, R867–R876. doi: 10.1152/ajpregu.00081.2007
Hill, R. W. (1976). The ontogeny of Homeothermy in neonatal Peromyscus leucopus. Physiol. Zool. 49, 292–306. doi: 10.1086/physzool.49.3.30155689
Hill, R. W. (1992). “The Altricial/Precocial contrast in the thermal relations and energetics of small mammals” in Mammalian Energetics: Interdisciplinary Views of Metabolism and Reproduction. eds. T. E. Tomasi and T. H. Horton (Ithaca, NY: Comstock Pub. Associates), 122–159.
Hohtola, E. (2004). “Shivering thermogenesis in birds and mammals” in Life in the Cold: Evolution, Mechanisms, Adaptation, and Application. Twelfth International Hibernation Symposium (Fairbanks: Biological Papers of the University of Alaska), 241–252.
Houštěk, J., Janíková, D., Bednár, J., Kopecký, J., Šebestián, J., and Soukup, T. (1990). Postnatal appearance of uncoupling protein and formation of thermogenic mitochondria in hamster brown adipose tissue. Biochim. Biophys. Acta BBA Bioenerg. 1015, 441–449. doi: 10.1016/0005-2728(90)90077-H
Janský, L. (1973). Non-shivering thermogenesis and its thermoregulatory significance. Biol. Rev. 48, 85–132. doi: 10.1111/j.1469-185X.1973.tb01115.x
Klaus, S., Heldmaier, G., and Ricquier, D. (1988). Seasonal acclimation of bank voles and wood mice: nonshivering thermogenesis and thermogenic properties of brown adipose tissue mitochondria. J. Comp. Physiol. B 158, 157–164. doi: 10.1007/BF01075829
Klingenspor, M. (2003). Cold-induced recruitment of Brown adipose tissue thermogenesis. Exp. Physiol. 88, 141–148. doi: 10.1113/eph8802508
Klingenspor, M., Ivemeyer, M., Wiesinger, H., Haas, K., Heldmaier, G., and Wiesner, R. J. (1996). Biogenesis of thermogenic mitochondria in brown adipose tissue of Djungarian hamsters during cold adaptation. Biochem. J. 316, 607–613. doi: 10.1042/bj3160607
Laemmli, U. K. (1970). Cleavage of structural proteins during the assembly of the head of bacteriophage T4. Nature 227, 680–685. doi: 10.1038/227680a0
Lagerspetz, K. Y. H. (1966). Postnatal development of thermoregulation in laboratory mice. Helgoländer Wiss. Meeresunters. 14, 559–571. doi: 10.1007/BF01611645
Lasiewski, R. C., Acosta, A. L., and Berstein, M. H. (1966). Evaporative water loss in birds. I. Characteristics of the open flow method of determination, and their relation to estimates of thermoregulatory ability. Comp. Biochem. Physiol. 19, 445–457. doi: 10.1016/0010-406X(66)90153-8
Lenth, R. V. (2022). Emmeans: Estimated marginal means (aka least-squares means). R Package, version 1.8.1-1.
Lezama-García, K., Mota-Rojas, D., Martínez-Burnes, J., Villanueva-García, D., Domínguez-Oliva, A., Gómez-Prado, J., et al. (2022). Strategies for hypothermia compensation in Altricial and Precocial newborn mammals and their monitoring by infrared thermography. Vet. Sci. 9:246. doi: 10.3390/vetsci9050246
Luna, F., Antenucci, C. D., Bozinovic, F., Vassallo, A. I., and Antenucci, C. D. (2015). “Macrophysiological patterns in the energetics of Caviomorph rodents: implications in a changing world” in Biology of Caviomorph Rodents: Diversity and Evolution Buenos Aires. SAREM Series A - Mammalogical Research (Argentina: SAREM), 245–272.
Luna, F., and Antinuchi, C. D. (2007). Energetics and thermoregulation during digging in the rodent tuco-tuco (Ctenomys talarum). Comp. Biochem. Physiol. A Mol. Integr. Physiol. 146, 559–564. doi: 10.1016/j.cbpa.2005.12.025
Luna, F., Antinuchi, C. D., and Busch, C. (2002). Digging energetics in the south American rodent Ctenomys talarum (Rodentia, Ctenomyidae). Can. J. Zool. 80:6. doi: 10.1139/z02-201
Luna, F., Okrouhlík, J., McKechnie, A. E., Bennett, N. C., and Šumbera, R. (2021). Non-shivering thermogenesis in four species of African mole-rats differing in their sociality. J. Zool. 315, 58–68. doi: 10.1111/jzo.12892
Luna, F., Roca, P., Oliver, J., and Antenucci, C. D. (2012). Maximal thermogenic capacity and non-shivering thermogenesis in the south American subterranean rodent Ctenomys talarum. J. Comp. Physiol. B 182, 971–983. doi: 10.1007/s00360-012-0675-6
Luna, F., Sastre-Serra, J., Oliver, J., and Antenucci, C. D. (2019). Thermogenic capacity in subterranean Ctenomys: species-specific role of thermogenic mechanisms. J. Therm. Biol. 80, 164–171. doi: 10.1016/j.jtherbio.2019.01.012
MacArthur, R. A., and Humphries, M. M. (1999). Postnatal development of thermoregulation in the semiaquatic muskrat (Ondatra zibethicus). Can. J. Zool. 77:9.
Malizia, A. I., and Busch, C. (1991). Reproductive parameters and growth in the fossorial rodent Ctenomys talarum (Rodentia: Octodontidae). Mammalia 55:293. doi: 10.1515/mamm.1991.55.2.293
Malizia, A. I., Zenuto, R. R., and Busch, C. (1995). Demographic and reproductive attributes of dispersers in two populations of the subterranean rodent Ctenomys talarum (tuco-tuco). Can. J. Zool. 73, 732–738. doi: 10.1139/z95-085
McClure, P. A., and Randolph, J. C. (1980). Relative allocation of energy to growth and development of Homeothermy in the eastern wood rat (Neotoma floridana) and hispid cotton rat (Sigmodon hispidus). Ecol. Monogr. 50, 199–219. doi: 10.2307/1942479
McNab, B. K. (1966). The metabolism of Fossorial rodents: a study of convergence. Ecology 47, 712–733. doi: 10.2307/1934259
Morrison, S. F., Ramamurthy, S., and Young, J. B. (2000). Reduced rearing temperature augments responses in sympathetic outflow to Brown adipose tissue. J. Neurosci. 20, 9264–9271. doi: 10.1523/JNEUROSCI.20-24-09264.2000
Naya, D. E., Spangenberg, L., Naya, H., and Bozinovic, F. (2013). Thermal conductance and basal metabolic rate are part of a coordinated system for heat transfer regulation. Proc. R. Soc. B Biol. Sci. 280:20131629. doi: 10.1098/rspb.2013.1629
Nespolo, R. F., Solano-Iguaran, J. J., and Bozinovic, F. (2017). Phylogenetic analysis supports the aerobic-capacity model for the evolution of Endothermy. Am. Nat. 189, 13–27. doi: 10.1086/689598
Newkirk, K. D., Silverman, D. A., and Wynne-Edwards, K. E. (1995). Ontogeny of thermoregulation in the Djungarian hamster (Phodopus campbelli). Physiol. Behav. 57, 117–124. doi: 10.1016/0031-9384(94)00220-Y
Nowack, J., Giroud, S., Arnold, W., and Ruf, T. (2017). Muscle non-shivering thermogenesis and its role in the evolution of Endothermy. Front. Physiol. 8:889. doi: 10.3389/fphys.2017.00889
Oelkrug, R., Polymeropoulos, E. T., and Jastroch, M. (2015). Brown adipose tissue: physiological function and evolutionary significance. J. Comp. Physiol. B 185, 587–606. doi: 10.1007/s00360-015-0907-7
Pinheiro, J., and Bates, D., R Core Team (2022). Nlme: Linear and nonlinear mixed effects models. R Package, version 3.1-159.
Porras, A., Penas, M., Fernandez, M., and Benito, M. (1990). Development of the uncoupling protein in the rat brown-adipose tissue during the perinatal period. Its relationship with the mitochondrial GDP-binding and GDP-sensitive ion permeabilities and respiration. Eur. J. Biochem. 187, 671–675. doi: 10.1111/j.1432-1033.1990.tb15352.x
Puigserver, P., Lladó, I., Palou, A., and Gianotti, M. (1991). Evidence for masking of brown adipose tissue mitochondrial GDP-binding sites in response to fasting in rats made obese by dietary manipulation. Effects of reversion to standard diet. Biochem. J. 279, 575–579. doi: 10.1042/bj2790575
R Core Team (2021). R: A language and environment for statistical computing. R Found. Stat. Comput. Available at: http://www.R-project.org/
R Studio Team (2020). R studio: Integrated development for R. Boston, MA: RStudio, PBC Available at: http://www.rstudio.com/
Reig, O. A., Busch, C., Ortells, M. O., and Contreras, J. R. (1990). “An overview of evolution, systematics, population biology, cytogenetics, molecular biology, and speciation in Ctenomys” in Evolution of Subterranean Mammals at the Organismal and Molecular Levels. eds. E. Nevo and O. A. Reig (New York: Wiley-Liss), 71–96.
Rezende, E. L., Bozinovic, F., and Garland, T. (2004). Climatic adaptation and the evolution of basal and maximum rates of metabolism in rodents. Evolution 58, 1361–1374. doi: 10.1111/j.0014-3820.2004.tb01714.x
Robertson, C. E., and McClelland, G. B. (2019). Developmental delay in shivering limits thermogenic capacity in juvenile high-altitude deer mice (Peromyscus maniculatus). J. Exp. Biol. 222:963. doi: 10.1242/jeb.210963
Robertson, C. E., Tattersall, G. J., and McClelland, G. B. (2019). Development of homeothermic endothermy is delayed in high-altitude native deer mice (Peromyscus maniculatus). Proc. R. Soc. B Biol. Sci. 286:20190841. doi: 10.1098/rspb.2019.0841
Rosenmann, M., and Morrison, P. (1974). Maximum oxygen consumption and heat loss facilitation in small homeotherms by he-O2. Am. J. Physiol. Leg Content 226, 490–495. doi: 10.1152/ajplegacy.1974.226.3.490
Sant Anna, G. M., and Mortola, J. P. (2003). Thermal and respiratory control in young rats exposed to cold during postnatal development. Comp. Biochem. Physiol. A Mol. Integr. Physiol. 134, 449–459. doi: 10.1016/S1095-6433(02)00321-5
Shefer, V. I., and Talan, M. I. (1998). The effect of exercise training in cold on shivering and nonshivering thermogenesis in adult and aged C57BL/6J mice. Exp. Gerontol. 33, 467–476. doi: 10.1016/S0531-5565(98)00009-6
Spiers, D. E., and Adair, E. R. (1986). Ontogeny of homeothermy in the immature rat: metabolic and thermal responses. J. Appl. Physiol. 60, 1190–1197. doi: 10.1152/jappl.1986.60.4.1190
Symonds, M. E. (2013). Brown adipose tissue growth and development. Scientifica 2013, 1–14. doi: 10.1155/2013/305763
Symonds, M. E., Pope, M., and Budge, H. (2012). Adipose tissue development during early life: novel insights into energy balance from small and large mammals. Proc. Nutr. Soc. 71, 363–370. doi: 10.1017/S0029665112000584
Thompson, S. D. (1992). “Gestation and lactation in small mammals: basal metabolic rate and the limits of energy use” in Mammalian Energetics: Interdisciplinary Views of Metabolism and Reproduction. eds. T. E. Tomasi and T. H. Horton (Ithaca, NY: Comstock Pub. Associates), 213–259.
Vassallo, A. I. (2006). Acquisition of subterranean habits in tuco-tucos (Rodentia, Caviomorpha, Ctenomys): role of social transmission. J. Mammal. 87, 939–943. doi: 10.1644/05-MAMM-A-384R2.1
Velotta, J. P., Robertson, C. E., Schweizer, R. M., McClelland, G. B., and Cheviron, Z. A. (2020). Adaptive shifts in gene regulation underlie a developmental delay in thermogenesis in high-altitude deer mice. Mol. Biol. Evol. 37, 2309–2321. doi: 10.1093/molbev/msaa086
Vleck, D. (1979). The energy cost of burrowing by the pocket gopher Thomomys bottae. Physiol. Zool. 52, 122–136. doi: 10.1086/physzool.52.2.30152558
Wade, G. N., and Schneider, J. E. (1992). Metabolic fuels and reproduction in female mammals. Neurosci. Biobehav. Rev. 16, 235–272. doi: 10.1016/S0149-7634(05)80183-6
Webb, D. R., Fullenwider, J. L., McClure, P. A., Profeta, L., and Long, J. (1990). Geometry of maternal-offspring contact in two rodents. Physiol. Zool. 63, 821–844. doi: 10.1086/physzool.63.4.30158179
Wharton, D. C., and Tzagoloff, A. (1967). “Cytochrome oxidase from beef heart mitochondria” in Methods in Enzymology (Amsterdam: Elsevier), 245–250.
White, R. B., Biérinx, A.-S., Gnocchi, V. F., and Zammit, P. S. (2010). Dynamics of muscle fibre growth during postnatal mouse development. BMC Dev. Biol. 10:21. doi: 10.1186/1471-213X-10-21
Withers, P. C. (1977). Measurement of VO2, VCO2, and evaporative water loss with a flow-through mask. J. Appl. Physiol. 42, 120–123. doi: 10.1152/jappl.1977.42.1.120
Withers, P. C., Cooper, C. E., Maloney, S. K., Bozinovic, F., and Cruz-Neto, A. (2016). Ecological and Environmental Physiology of Mammals. Oxford: Oxford University Press.
Wunder, B. A., and Gettinger, R. D. (1996). “Effects of body mass and temperature acclimation on the nonshivering thermogenic response of small mammals” in Adaptations to the Cold: Tenth International Hibernation Symposium (Armidale: University of New England Press), 131–139.
Zenuto, R. R., Antinuchi, C. D., and Busch, C. (2002). Bioenergetics of reproduction and pup development in a subterranean rodent (Ctenomys talarum). Physiol. Biochem. Zool. 75, 469–478. doi: 10.1086/344739
Keywords: thermoregulation, non-shivering thermogenesis, postnatal development, maximum metabolism, Ctenomys
Citation: Luna F, Sastre-Serra J, Oliver J and Antenucci CD (2023) Growing underground: Development of thermogenesis in pups of the fossorial rodent Ctenomys talarum. Front. Ecol. Evol. 11:1120415. doi: 10.3389/fevo.2023.1120415
Edited by:
Enrico Lunghi, University of L'Aquila, ItalyReviewed by:
Daniel Galiano, Universidade Federal da Fronteira Sul, BrazilPatricia Tachinardi, University of São Paulo, Brazil
Copyright © 2023 Luna, Sastre-Serra, Oliver and Antenucci. This is an open-access article distributed under the terms of the Creative Commons Attribution License (CC BY). The use, distribution or reproduction in other forums is permitted, provided the original author(s) and the copyright owner(s) are credited and that the original publication in this journal is cited, in accordance with accepted academic practice. No use, distribution or reproduction is permitted which does not comply with these terms.
*Correspondence: Facundo Luna, Zmx1bmFAbWRwLmVkdS5hcg==