- 1Laboratory of Fundamental and Applied Research in Chemical Ecology, Institute of Biology, University of Neuchâtel, Neuchâtel, Switzerland
- 2Neuchâtel Plateform of Analytical Chemistry, University of Neuchâtel, Neuchâtel, Switzerland
Cotton (Gossypium hirsutum) stores defensive compounds in glands covering its leaves and other tissues. The density and the chemical filling of these glands increase systematically in developing leaves in response to herbivory on older leaves. Cotton seedlings are known to respond more strongly to actual caterpillar herbivory than to mere physical damage. It is not clear whether this amplified response is linked to insect-derived elicitors or difference in damage properties. To investigate this, we assessed the effect of repeated artificial damage without and with application of regurgitant from Spodoptera exigua caterpillars. Repeated mechanical damage led to a systemic increase of gland density, gland size, and content of defensive terpenes, with no detectable additional elicitation upon regurgitant treatment. Dual choice feeding assays further showed that defense induction triggered by just physical damage made newly developing leaves far less palatable to S. exigua larvae as compared to leaves from undamaged seedlings, whereas they did not distinguish between leaves from damaged plants treated with or without regurgitant. Our study confirms that the systemic induction of cotton glands is an unspecific response to physical damage, although cotton is known to respond to caterpillar-associated elicitors for other defensive traits. Cotton glands induction can be readily visualized under modest magnification, making the experiments described in this study highly suited to teach chemical ecology and aspects of plant defense theory in practical classes.
1. Introduction
Plants produce a variety of volatile and non-volatile compounds to protect themselves from insect herbivores. Cotton (Gossypium sp.) stores defensive chemicals in glands, visible to the naked eye as small black dots present on the surface of its tissues (Hagenbucher et al., 2013). These structures provide crucial protection against herbivores. Cotton mutants without glands suffer more damage from various insects (Bottger et al., 1964; Jenkins et al., 1966; Lukefahr et al., 1966). For instance, Spodoptera exigua larvae preferentially feed and perform better on glandless cotton than glanded cotton (Bottger et al., 1964; McAuslane and Alborn, 1998; Agrawal and Karban, 2000). The strong defensive effect of glands is associated with a group of related terpenoid aldehydes with insecticidal activity, which notably include gossypol and heliocides (Hagenbucher et al., 2013). Cotton glands also contain several volatile monoterpenes and sesquiterpenes (Elzen et al., 1985). These volatile organic compounds (VOCs) are emitted at relatively low levels by healthy plants, but rupturing of the glands by herbivores results in their immediate release (Loughrin et al., 1994; McCall et al., 1994). Gland-stored VOCs have direct detrimental effects on the development of lepidopteran larvae (Gunasena et al., 1988; Zhang et al., 2022) and they may repel oviposition by adult moths and colonization by piercing-sucking insects (Jenkins et al., 1966; Zhang et al., 2020). They also are attractive to certain parasitoid wasps (Elzen et al., 1984, 1986; Zhang et al., 2020), suggesting that they may be involved in indirect defense as well.
For various plants it is known that they perceive herbivore attacks and respond by mobilizing additional defenses (Felton and Tumlinson, 2008). This is also the case for cotton plants; in response to herbivory, young immature leaves overall produce higher concentrations of defense compounds (Rose et al., 1996; McAuslane et al., 1997; McAuslane and Alborn, 1998; Agrawal and Karban, 2000; Bezemer et al., 2004; Anderson and Agrell, 2005; Opitz et al., 2008; Eisenring et al., 2017). The increased concentrations of these gland-stored compounds are a result of additional glands being formed, but also of an increased filling of the glands: they accumulate more terpenoid aldehydes and volatile terpenes (McAuslane et al., 1997; Opitz et al., 2008). The magnitude of this systemic induction depends on damage intensity (Agrawal and Karban, 2000; Opitz et al., 2008; Eisenring et al., 2017).
Plants defenses are induced upon recognition of physical injury though damage-associated molecular patterns (DAMPs), as well as the specific detection of a feeding insect through herbivore-associated molecular patterns (HAMPs) (Heil, 2009; Acevedo et al., 2015; Duran-Flores and Heil, 2016; Erb and Reymond, 2019). HAMPs are notably present in the regurgitant of lepidopteran larvae, but such herbivore-specific elicitors are also known for grasshoppers (Alborn et al., 2007), sucking-piercing insects (Yang et al., 2014; Shangguan et al., 2018) and insect eggs (Stahl et al., 2020). Several studies strongly suggest that Upland cotton plants (Gossypium hirsutum) can also detect HAMPs and can distinguish physical wounding alone from caterpillar damage. For instance, treatment of artificial wounds with regurgitant of S. exigua, Spodoptera litura and Helicoverpa armigera results in a transcriptional, metabolic and hormonal response distinct from that of wounding alone (Zebelo et al., 2017; Si et al., 2020). Also, both physical damage and larvae-derived factors – yet unidentified – are known to play a role in G. hirsutum induced systemic volatile emissions, with some compounds released in greater amounts when S. exigua regurgitant is added to artificially inflicted wounds (Paré and Tumlinson, 1997; Arce et al., 2021). Whether systemic gland induction is also triggered by HAMPs is not clear. Repeated mechanical damage visibly induces glands and increases their chemical content, but induction is lower than with real herbivory by Spodoptera littoralis (Opitz et al., 2008). This difference could be due to insect-derived elicitors, but also to a difference in frequency and extent of the physical damage (Opitz et al., 2008; Wu and Baldwin, 2009). Disentangling the effects of physical and insect-associated chemical cues in defense induction can be achieved through artificial damage and application of larvae regurgitant (Bricchi et al., 2010).
Hence, the aim of this study was to investigate the relative effects of repeated physical injury and insect-derived elicitors on the systemic induction of G. hirsutum glands. In addition, we describe a simple experimental protocol for cotton defense induction that can be used as model for hands-on teaching. We assessed the effect of repeated artificial mechanical damage without and with application of S. exigua regurgitant on leaf concentration of gland-stored terpenoid aldehydes and volatile terpenes, as well as on gland density and size. Gland size was used as a proxy to estimate overall gland filling. The true defensive effect of the induction was determined through feeding choice assays with S. exigua larvae. We used a repeated-damage method and regurgitant application similar to those performed by Arce et al. (2021). Their work explored the involvement of both DAMPs and HAMPs from regurgitant of S. exigua in the induction of systemic volatile emissions in G. hirsutum seedlings. Here we showed that repeated mechanical damage induces a visible systemic increase in gland density and filling, with no apparent additional effect of regurgitant application. The unspecific but characteristic effect of physical damage on gland production in G. hirsutum makes the described experiments ideal for teaching the chemical ecology of plant defenses to students at different levels.
2. Materials and methods
2.1. Plants
Seeds of Gossypium hirsutum L., variety STAM 59A, which is commonly cultivated in Africa, were provided by CIRAD (French agricultural research and cooperation organization, France). We soaked the seeds in water at 24°C in the dark overnight and then sowed them individually in plastic pots (h: 8.5 cm, d: 6 cm), filled with soil (universal potting soil, Ricoter, Switzerland). Seedlings were kept in phytotrons (GroBanks CLF Plant Climatics, Germany, 16 h light, 28°C, 65 μmol m−2 s−1 and 8 h dark, 25°C) until they were used for experiments, at the age of 3 weeks. They were watered every 3 days and fertilized with 30 mL of fertilizer (3.3 mL/L, Capito, 7-3-6 NPK, with oligo-elements) once a week. The day before the first damage treatment, we moved the plants to an experimental room under LED lights (SLT-EDK 6400K, Edkfarm, China, 200 μmol m−2 s−1, 16 h:8 h light/dark, 26 ± 3°C) where they were kept until measurements were made. To minimize potential signaling through induced volatile emissions plants with the different treatments were separated from each other by ~1 m. Their position was changed every day.
2.2. Insects and regurgitant collection
Spodoptera exigua (Hübner; Lepidoptera; Noctuidae) is a cosmopolitan generalist pest that feeds on many crops, including cotton. Its rearing and collection of regurgitant were performed as described by Arce et al. (2021) and according to Turlings et al. (1993). Caterpillars were reared from eggs supplied by Entocare (Wageningen, Netherlands), on wheat germ-based artificial diet (Frontier Scientific Services, Newark, United States). Regurgitant was collected from third to fourth instar larvae, that had been fed on cotton leaves for 24 h. We triggered regurgitation by gently pressing the caterpillars’ body toward the head. The regurgitant was collected with a pipette and stored in Eppendorfs protected from light and kept on ice. After each collection, Eppendorfs with regurgitant were kept at −80°C until use.
2.3. Induction by simulating herbivory through artificial mechanical damage
To dissociate the effect of physical damage and the effect of S. exigua regurgitant, we simulated chewing herbivory by mechanically damaging cotton seedlings without and with applying regurgitant to the wounds. Controls consisted of undamaged plants. Seedlings used had two true leaves and were starting to develop the third one. The earliest stage used were plants whose third leaves just started to emerge (Supplementary Figure S1A) and the latest stage were plants with small third leaves that started to expand, but had not flattened yet (Supplementary Figure S1B). We used the same procedure and timing of damage that was used by Arce et al. (2021) for their analysis of systemically induced volatile emissions. First and second leaves were damaged with serrated forceps a total of four times, over two consecutive days (mornings and evenings). Each damage event consisted of applying the forceps three times with strong manual pressure on each side of a leaf (~1 cm2 per leaf side). Right after damaging the leaves, 10 μL of regurgitant were spread on the wounded surfaces (~5 μL per leaf side). A different batch of regurgitant was used every one to two damage events. We started damaging the apical part of the first leaf (Figure 1A), then its more basal part. The second leaf was damaged in the same way on the second day (Figure 1B). Seven days after damage began, the third leaves were collected for measurements and for choice feeding assays.
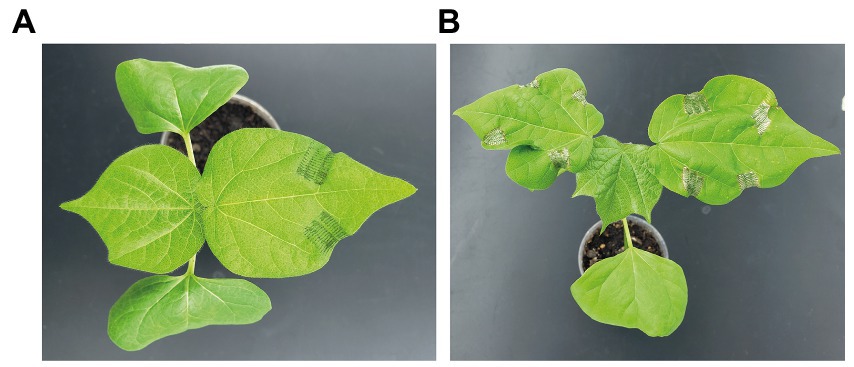
Figure 1. Pictures illustrating the process of induction through repeated mechanical damage, without and with application of Spodoptera exigua regurgitant. Plants were damaged four times over two consecutive days. (A) Seedling of Gossypium hirsutum right after the first damage treatment: the first leaf was damaged on two sides of its apical part. (B) Seedling of G. hirsutum the day after the last damage treatment: both first and second leaves were damaged on each side of their apical and basal part.
2.4. Gland density
Third leaves (n = 18 per damage treatment) were collected and their abaxial surface was directly photographed. Pictures were used to count glands manually using the Multipoint tool of the Fiji distribution of ImageJ software (Schindelin et al., 2012). We counted all the glands present in a surface of 1 × 1 cm selected in the upper center of the leaves. Leaves photographed for gland density measurement were then flash-frozen for subsequent terpenes analysis or used for the first experimental repetition of the choice feeding assays.
2.5. Gland size
We measured gland area (n = 11–12 per damage treatment) to estimate gland size, as we could not measure their volume. Gland size was used as a proxy to estimate overall gland filling. For this, pictures of the central part of the abaxial surface were taken under a stereoscope with a magnification of 6.3×. These pictures were taken after the leaves were used for the third replication of the feeding choice assays, were caterpillars ate a maximum of 4.3% of the leaves. This left sufficient space to measure gland size. Using Fiji, pictures were converted to 8-bit. We then used the Threshold tool to select glands and pictures were converted to mask (Binary ➔ Convert to mask). Analyze Particles was used to measure simultaneously the area of each gland, excluding glands touching the edges of the images. This represented between 20 and 96 glands per leaf. Areas were averaged into a single value for each leaf.
2.6. Gland-stored compounds
Third leaves (n = 6 per damage treatment) were flash-frozen in liquid nitrogen and stored at −80°C until they were ground into powder.
2.6.1. Terpenoid aldehydes
Fifty milligram of frozen leaf powder were extracted with 80 μL acetonitrile. Samples were homogenized with five to six glass beads (1.25–1.65 mm diameter) in a mixer mill for 3 min at 30 Hz (TissueLyser II, Qiagen, Germany). They were then centrifuged for 5 min at 14,000 rpm. The recovered supernatant was centrifuged a second time before being transferred to amber glass vials. Samples were directly analyzed through ultra-performance liquid chromatography with diode array detection (UPLC-DAD, Ultimate 3000 Dionex, Thermo Fisher Scientific, MA, United States). DAD detector was set at 288 ± 2 nm. 5 μL of samples were injected onto an ACQUITY UPLC® BEH C18 column (2.1 × 100 mm, 1.7 μm; Waters, MA, United States). Flow rate was held constant at 0.45 mL/min and the temperature was kept at 30°C. The mobile phase A consisted of 0.05% formic acid in MilliQ water (18 Ω) and the mobile phase B of 0.05% formic acid in acetonitrile (HiPerSolv, VWR Chemicals®, France). B increased from 45% to 90% in 8 min, then to 100% in 0.5 min, held at 100% for 2.5 min, which was followed by re-equilibration at 45% B for 3.5 min. Gossypol and heliocides (grouped together) were identified by their retention time. Quantification was based on linear regression from six calibration points (5–250 μg/mL) in gossypol equivalents.
2.6.2. Volatile terpenes
We used the same procedure as described by Clancy et al. (2023). 100 mg of frozen leaf powder were extracted with 1 mL of hexane. Samples were vortexed and kept at 4°C for 24 h for the leaf material to sediment. We recovered 800 μL of hexane and added 8 μL of nonyl acetate as internal standard. Samples were directly analyzed by gas chromatography coupled with mass spectrometry (GC Agilent 8890A and MSD Agilent 5977B) (20 ng/μL). 1.5 μL of samples were injected (inlet at 250°C) in splitless mode onto an Agilent HP-5MS column (30 m × 250 μm × 0.25 μm). We used helium as carrier gas, with a stable flow rate of 0.9 mL/min. Temperature ramped from 40°C to 130°C at 15°C min−1, then 60°C min−1 to 190°C and 30°C min−1 to 290°C, where it was held for 0.5 min. Peaks were integrated from the Total Ion Chromatograms. Terpenes were identified by comparison of mass spectra with the NIST 17 mass spectral library, and had been previously identified for cotton with authentic standards. We quantified monoterpenes with the relative response factor of α-pinene and sesquiterpenes based on β-caryophyllene.
2.7. Dual choice feeding assays
We conducted dual choice feeding assays to investigate the relative defensive effects of induction by physical damage and elicitation by regurgitant. S. exigua larvae were offered pairs of leaves of the three possible combinations of damage treatment (n = 14–16 for each combination). Late third to early fourth instars were fed with cotton for 24 h and starved for 3 h before each choice assay. Leaves were placed in pairs in transparent Petri dishes of 14 cm diameter, with their petioles wrapped in wet cotton wool to prevent desiccation. Because gland-associated traits vary with leaf area (McAuslane et al., 1997; Opitz et al., 2008), leaves of similar size were paired (Supplementary Figure S2). We released a single larva in the center of each dish. Petri dishes were placed under artificial light in random position and orientation. The assay was replicated three times with n = 5–6 for the first and third repetition and n = 3–4 for the second one. Data of the three repetitions were analyzed together. Because of difference in feeding between the three repetitions, caterpillars fed for 10 h (10:30 to 20:30) the first repetition and for 24 h (12:00 to 12:00 + 1D) the second and third repetitions. Leaf surface consumed was analyzed from pictures using Fiji.
2.8. Statistical analyses
Statistical analyses were performed with R 4.2.2 (R Core Team, 2022). A threshold of α = 0.05 was used. We plotted predictions from the statistic models and their 95% confidence intervals with the function ggeffect() of the package ggeffects (v. 1.1.3, Lüdecke, 2018) and ggplot2 (v. 3.3.6, Wickham, 2016).
2.8.1. Gland density, gland size and gland-stored compounds
Effect of damage treatment on gland density, gland size and leaf concentrations of gland-stored compounds was tested by analysis of covariance with the package car (v. 3.1.1, Fox and Weisberg, 2019), followed by pairwise comparisons (Tuckey contrasts) with the function glht() of the package multcomp, with multiplicity adjustment of p-values (single-step method; v. 1.4.20, Hothorn et al., 2008). Leaf area was used as covariate in all analyses. Gland density was analyzed with a negative binomial generalized linear model (GLM), gland area with a linear model (LM) and leaf concentrations of the different compounds with either gamma GLMs with log link function or LMs, depending on data distribution. LMs were performed with the base stats package. Gamma and negative binomial GLMs were performed with the package MASS (v. 7.3.58.1, Venables and Ripley, 2002).
2.8.2. Dual choice feeding assays
Welch’s paired t-tests were used to check that there was no difference in size between the leaves paired. Within each combination of damage treatment, difference in leaf consumption was tested with a generalized linear mixed-effect model (GLMM) including damage treatment and experimental repetition as fixed effects and larval identity as random intercept. A Tweedie model with log link function was used, from the package glmmTMB (v. 1.1.4, Brooks et al., 2017). To test whether there were differences in the strength of preference/deterrence between combinations, we performed a Tweedie GLM with probit link function on the proportion of leaf eaten from one of the damage treatments.
3. Results
3.1. Relationship of leaf area with gland density and filling
Both gland density and gland size depended on leaf area (Figure 2). While gland density decreased with leaf area, gland size increased. Gossypol leaf concentration decreased with leaf size, but there was no significant relationship for heliocides content (Supplementary Table S1). Similarly, volatile terpenes concentrations either decreased with leaf size or showed no significant correlation (Supplementary Table S1).
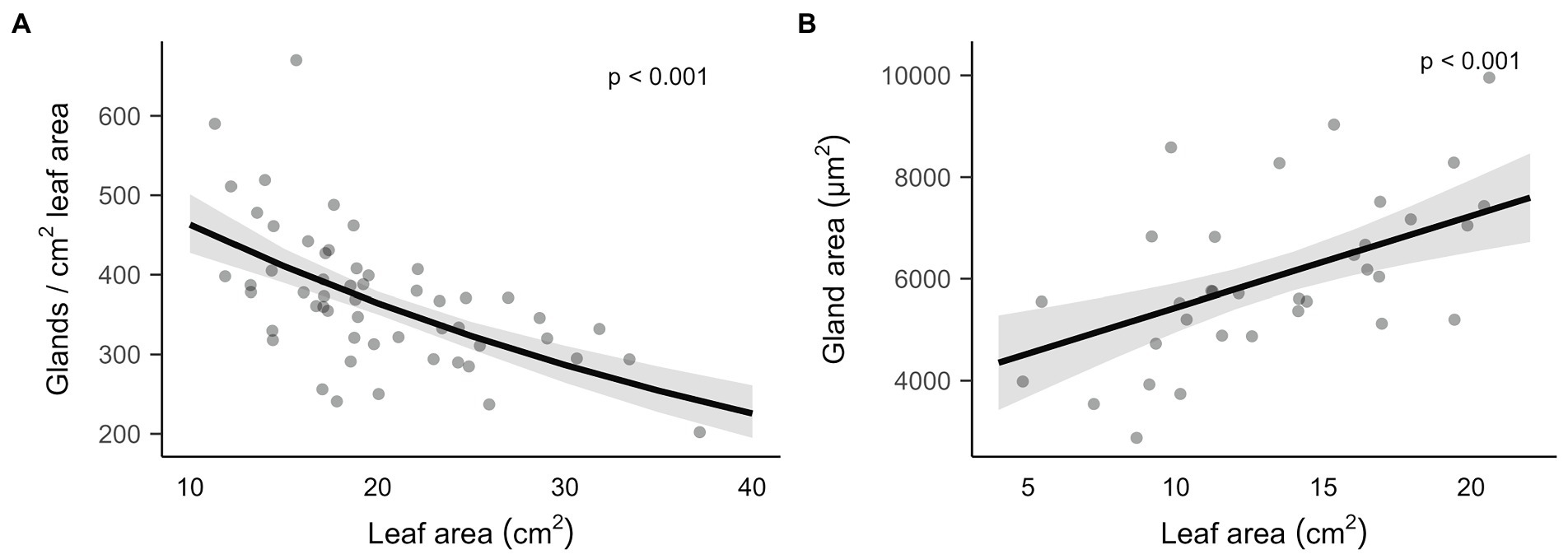
Figure 2. Relationship of leaf area with gland density and gland size (area). Traits were measured in the third leaves of Gossypium hirsutum seedlings. Plots represent together plants that were undamaged, mechanically damaged (Damaged) and mechanically damaged with application of Spodoptera exigua regurgitant (Dam. + regurgitant). Effect of leaf area was indeed independent of the effect of damage treatment (Gland density: χ2(2,48) = 0.97, p = 0.62, Gland size: F(2,28) = 0.01, p = 0.99). (A) Predicted relationship and 95% CI between leaf area and gland density, estimated from a negative binomial GLM containing leaf area as covariate and damage treatment as factor. Leaf area was significant at α = 0.05, with n = 54, χ2(1,50) = 47.84 and p = 4.62·10−12. (B) Predicted relationship and 95% CI between leaf area and gland size, estimated from a LM containing leaf area as covariate and damage treatment as factor. Leaf area was significant at α = 0.05, with n = 34, F(1,30) = 16.32 and p = 3.42·10−4.
3.2. Induction of gland density and size
Damaged cotton seedlings increased their gland density and gland size, whether regurgitant was applied to their wounds or not (Figure 3). A week after damage began, the third leaves of mechanically damaged plants had on average 27% more glands per cm2 than leaves of undamaged plants (Figure 3A). In addition, their glands were on average 41% larger (Figure 3B). For both traits, there was no detectable difference between plants that received damage only or damage with regurgitant application.
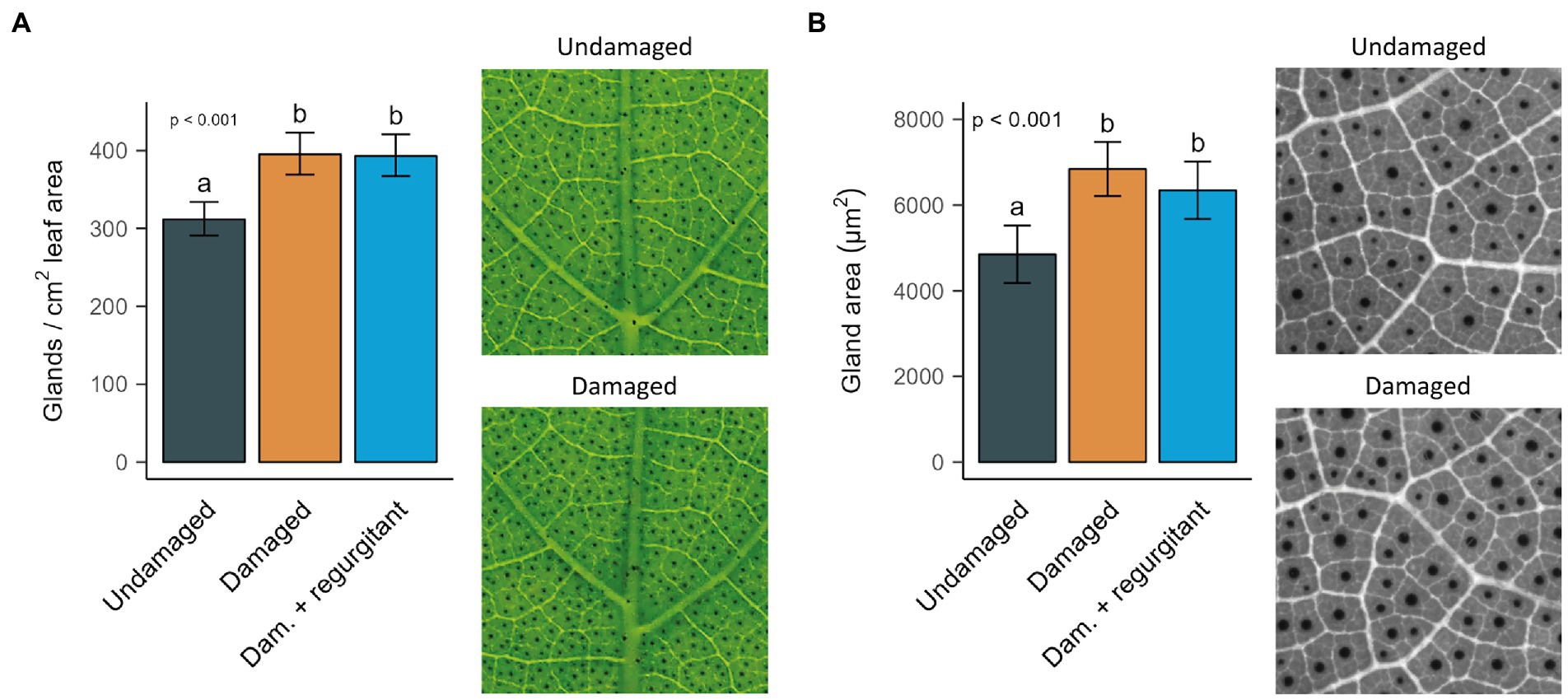
Figure 3. Effect of mechanical damage and application of Spodoptera exigua regurgitant on gland density and gland size (area). Traits were measured in the third leaves of Gossypium hirsutum seedlings that were undamaged, mechanically damaged (Damaged) and mechanically damaged with application of S. exigua regurgitant (Dam. + regurgitant). Plants were damaged on their first and second leaves while the third leaf was emerging. Leaves were collected a week after damage began. (A) Predicted means and 95% CI of gland density for an average leaf area of 20 cm2. Damage treatment (n = 18) was tested with a negative binomial GLM with leaf area as covariate. It was significant at α = 0.05, with χ2(2,50) = 30.77 and p = 2.08·10−9. Different letters indicate a significant difference between types of damage treatment, as tested by pairwise comparisons. Pictures are examples of leaves of similar size from undamaged and mechanically damaged plants that were used to measure the number of glands in a 1 × 1 cm surface. (B) Predicted means and 95% CI of gland area for an average leaf area of 13.3 cm2. Damage treatment (n = 10–13) was tested with a LM with leaf area as covariate. It was significant at α = 0.05, with F(2,30) = 10.19 and p = 3.42·10−4. Different letters indicate a significant difference between types of damage treatment, as tested by pairwise comparisons. Pictures are examples of leaves of similar size from undamaged and mechanically damaged plants that were used to measure the areas of glands in a 3,000 × 3,000 μm surface.
3.3. Induction of terpenoid aldehydes leaf concentration
We quantified gossypol and heliocides. Their leaf concentration followed the same pattern as gland density and gland size: damaged plants accumulated more of these compounds than undamaged plants, regardless of regurgitant application (Figure 4; Supplementary Table S1). A week after the start of induction, the third leaves of mechanically damaged plants had more than doubled their gossypol content, with an average increase of 128%. Heliocides increased by 86%. There was again no observable difference in their concentrations between mechanical damage alone and mechanical damage with regurgitant application.
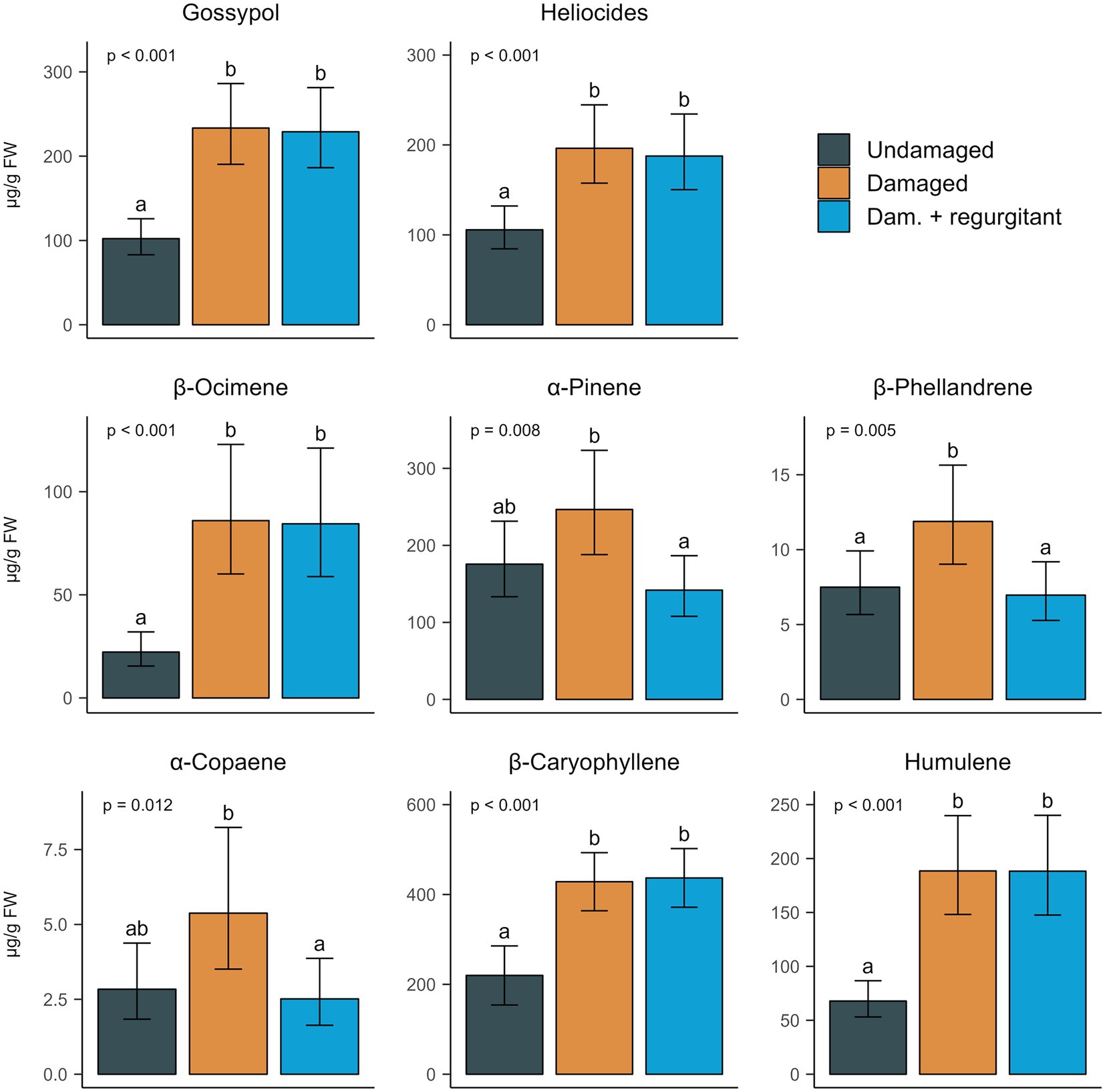
Figure 4. Effect of mechanical damage and application of Spodoptera exigua regurgitant on the leaf concentrations of terpenoid aldheydes and volatile terpenes. Terpenoid aldheydes (gossypol and heliocides) and volatile terpenes (from β-ocimene to humulene) were extracted from the third leaves of Gossypium hirsutum seedlings that were, from left to right: undamaged, mechanically damaged (Damaged) and mechanically damaged with application of S. exigua regurgitant (Dam. + regurgitant). Plants were damaged on their first and second leaves while the third leaf was emerging. Leaves were collected a week after damage began. Damage treatment (n = 6) was tested with gamma GLMs (log link function) or LMs, with leaf area as covariate at α = 0.05. Plots represent predicted means and 95% CI of analytes concentration [μg per g of fresh weight (FW)], for an average leaf area of 18.3 cm2. Different letters indicate a significant difference between types of damage treatment, as tested by pairwise comparisons. Details on statistics are in Supplementary Table S1.
3.4. Induction of volatile terpenes leaf concentration
Ten monoterpenes and nine sesquiterpenes were quantified from leaf extracts (Supplementary Table S1). The leaf concentration of all monoterpenes and six sesquiterpenes was significantly affected by damage treatment (Supplementary Table S1). Several compounds showed similar patterns to that observed for the terpenoid aldehydes: leaf accumulation increased with wounding, with no distinct differences between physical damage only and damage with regurgitant. This was the case for the sesquiterpenes β-caryophyllene, humulene, β-elemene, α-guiaene and γ-bisabolene, and the monoterpene β-ocimene (Figure 4; Supplementary Table S1). β-ocimene levels were most affected by induction, with a nearly fourfold increase for both types of damage. The other monoterpenes also increased with mechanical damage (e.g., β-phellandrene) or tended to do so (e.g., α-pinene), but to a lesser extent than β-ocimene or the above mentioned sesquiterpenes (Figure 4; Supplementary Table S1). Unexpectedly, plants treated with regurgitant did not accumulate more of these monoterpenes: leaf concentrations were similar to those of undamaged plants and significantly lower than with mechanical damage alone. This was also the case of the sesquiterpene α-copaene (Figure 4; Supplementary Table S1).
3.5. Dual choice feeding assays
Spodoptera exigua larvae were offered pairs of leaves of all three possible combinations of damage treatment. Larvae preferred leaves of undamaged plants to those of induced plants, regardless of regurgitant application (Figure 5). However, their preference for uninduced leaves was stronger when the choice was with leaves from plants treated with regurgitant than with leaves from plants that were damaged only (Supplementary Figure S3). Larvae did not distinguish between leaves of plants mechanically damaged only and those damaged with regurgitant application (Figure 5).
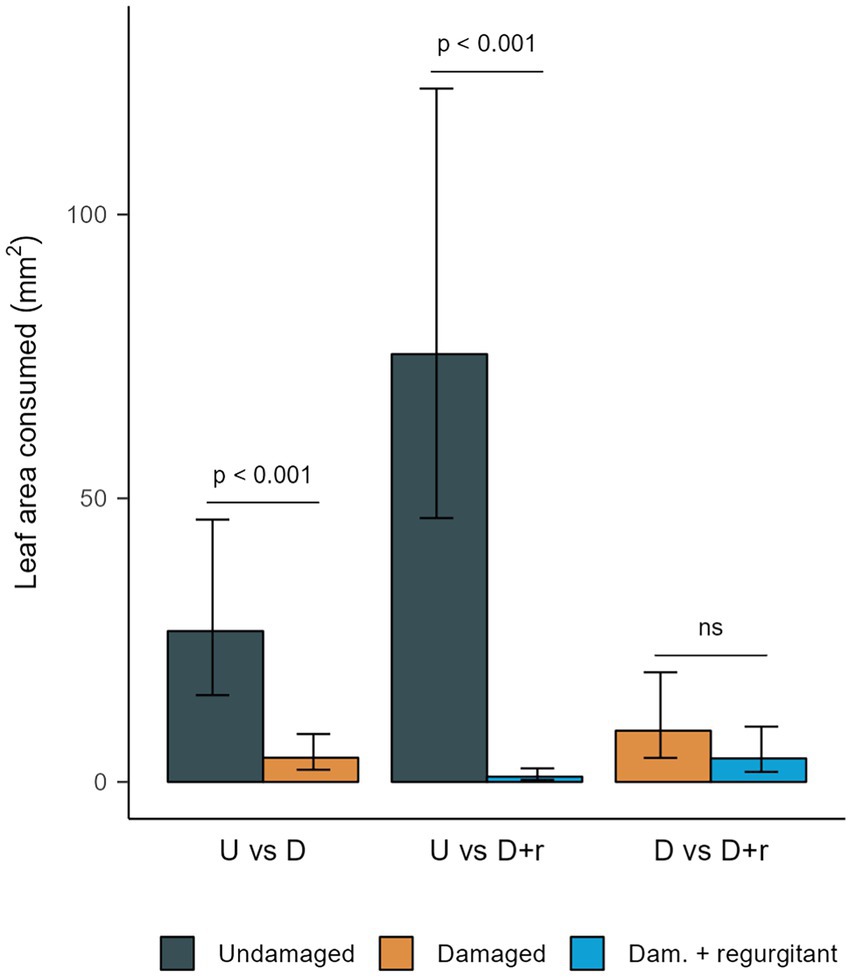
Figure 5. Leaf area consumed by Spodoptera exigua larvae in dual choice feeding assays. Late third to early fourth instar larvae were offered pairs of third leaves of Gossypium hirsutum seedlings that were undamaged (U), mechanically damaged (Damaged; D) or mechanically damaged with application of S. exigua regurgitant (Dam. + regurgitant; D + r). Plants were damaged on their first and second leaves while the third leaf was emerging. Leaves were used for the assay a week after damage began. Leaves were paired in all possible combinations of damage treatment. A single larva was used for each pair of leaves. Within each combination of damage treatment, we compared leaf consumption with a Tweedie GLMM (log link function), with larval identity set as random factor. Data from three independent repetitions were analyzed together, with repetition set as fixed factor. Predicted means and 95% CI are plotted. Leaf area consumed by larvae was different between leaves of undamaged and mechanically damaged plants (U vs. D; n = 16 larvae) at α = 0.05, with, χ2(1,25) = 22.48 and p = 2.13·10−6. Consumption was different between leaves of undamaged plants and plants mechanically damaged with regurgitant application (U vs. D + r; n = 15 larvae) at α = 0.05, with, χ2(1,22) = 94.32 and p < 2.2·10−16. There was no significant difference between leaves of plants mechanically damaged and plants mechanically damaged with application of regurgitant (D vs. D + r; n = 14 larvae) at α = 0.05, with, χ2(1,21) = 1.96 and p = 0.16.
4. Discussion
4.1. Relationship of leaf area with gland density and filling
Cotton gland density is highly dependent on leaf area, or age: the larger a leaf grows, the more space there is between the glands that it carries (McAuslane et al., 1997). Therefore, leaf area is an important variable to consider when studying the induction of glands in cotton. As expected, we observed a negative correlation between gland density and leaf area. In contrast, gland size had an opposite relationship, with larger leaves having larger glands to remove. Larger glands suggest that they were likely more filled with chemical content. Gland filling is thus a process occurring during leaf maturation. Mint, for instance, has glands that fill as the leaves age, with monoterpene synthesis peaking in middle-aged leaves (Gershenzon et al., 2000; Turner et al., 2000). Gland-stored compounds differed in their relationship to leaf area, indicating that they likely contributed to gland filling differently and that leaf chemical composition varied with leaf maturation. For instance, gossypol and α-pinene decreased with leaf area, whereas heliocides and β-ocimene stayed at similar levels. Eisenring et al. (2017) described that gossypol decreases with leaf aging, while heliocides remain constant, an effect hypothesized to be due to differences in compound stability and synthesis over time (Bell et al., 1978; Eisenring et al., 2017).
4.2. Systemic induction of glands and their chemical content by repeated mechanical damage
Cotton seedlings responded to repeated mechanical damage on their first and second leaves by accumulating more gossypol, heliocides and volatile terpenes in their developing undamaged third leaves. Volatile terpenes differed in their levels of induction, with β-ocimene showing the strongest increase, followed by some of the sesquiterpenes. These results are in accordance with those of Opitz et al. (2008). They described mechanical damage to increase foliar content of the gland-stored compounds in immature leaves, within the same time frame. They also observed a particularly strong induction of β-ocimene, as well as a stronger response from sesquiterpenes than from monoterpenes. Induction of gland-stored compounds is a result of both greater gland density and increased gland content (McAuslane et al., 1997; Opitz et al., 2008). We found that leaves of mechanically damaged plants had indeed higher gland density and they also had larger glands, suggesting that they were more filled with chemical content. The induction triggered by mechanical damage apparently deterred Spodoptera exigua larvae from feeding: they strongly preferred to feed on leaves of undamaged plants in the dual choice assays.
4.3. No detectable elicitation by Spodoptera exigua regurgitant
Adding S. exigua larvae regurgitant to artificial wounds did not elicit a stronger response than mere physical damage in terms of gland density, gland size and accumulation of gland-stored compounds. In accordance with this, caterpillars fed equally on leaves from plants induced with mechanical damage and leaves from damaged plants with addition of regurgitant. Keeping damage constant, our experiments strongly suggest that systemic induction of gland number and filling is not affected by regurgitant-derived factors. It adds evidence to the hypothesis that systemic induction of cotton glands is an unspecific response to physical damage, as proposed by Opitz et al. (2008).
Using a similar induction method, Arce et al. (2021) observed that the application of S. exigua regurgitant to repeatedly mechanically damaged plants induces a stronger systemic release of some volatile compounds than mechanical damage alone. This contrasts with what we observed for gland induction and implies that involvement of regurgitant-derived HAMPs in eliciting cotton defenses is trait-dependent. Glands provide cotton with a broad-spectrum direct defense: they are not only effective against various insects, but also against pathogens and non-ruminant animals (Hagenbucher et al., 2013). Therefore, the non-specificity in regulating their induction may be adaptive. On the other hand, systemic volatile emissions are involved in the more specific interactions with natural enemies of herbivores and can act as signals between or within plants. In those cases, specificity may be necessary to convey reliable information (Turlings and Erb, 2018). Traits-dependency of the elicitation by HAMPS likely explains why S. exigua had a stronger preference for uninduced leaves when the choice involved leaves from plants treated with regurgitant than when the assay was done with leaves from plants that were only damaged. They were probably deterred by other defenses, such as volatile emissions that are more strongly induced when regurgitant is applied. Indeed, it is known that S. exigua prefers to feed on uninduced glandless plants to induced ones, indicating an effect of other inducible traits than glands on their choice (McAuslane and Alborn, 1998).
Application of regurgitant triggered a reduction in the leaf concentration of some volatile terpenes, to levels similar to undamaged plants. It happened despite a higher gland density than undamaged plants, implying that the additional glands that were formed contained a different blend of compounds. Insect regurgitant can also contain effectors, that inhibit the induction of plant defenses (Acevedo et al., 2015). However, the fact that this suppression is restricted to only some of the gland-stored compounds rather suggests that the differences are due to the reallocation of the substrates used in their synthesis to other inducible traits, such as the systematically emitted VOCs.
4.4. Factors that potentially modulate the effects of mechanical damage and regurgitant on gland induction
The strength of induction by mechanical damage and regurgitant exposure and their relative importance likely depends on several factors. We measured induced traits at a single time point, in a specific leaf and at a specific plant stage. However, there is likely temporal variation in the induction of plant defenses. As mentioned, accumulation of gland-stored compounds depends on leaf development (McAuslane et al., 1997; Eisenring et al., 2017) and plant age is another ontogenic factor to take in account (Anderson et al., 2001; Boege and Marquis, 2005; Quintero and Bowers, 2011). There is also spatial heterogeneity within the plants: the levels of cotton glands induction depend on the position of a leaf relative to the damage (Anderson and Agrell, 2005; Eisenring et al., 2017). Hence, measurements in other leaves, other time points and other plant developmental stages could lead to different outcomes for both the effects of damage alone and addition of regurgitant.
Also, induction of cotton glands depends on damage intensity (Agrawal and Karban, 2000; Opitz et al., 2008; Eisenring et al., 2017). Eisenring et al. (2017) described that in cotton seedlings gland density and terpenoid aldehydes content strongly increase with the surface damaged by herbivores over 2 days, before reaching a plateau around 2.5 cm2. The area that we damaged in this study was much higher, 8 cm2 in total. This might have triggered induction to its full strength. It is possible that elicitation by HAMPs may be visible only if the surface damaged is not high enough to trigger a full response. Another point that should be considered is how resources availability affects a trade-off between allocation to growth vs. defense (Züst and Agrawal, 2017). Increasing nitrogen for instance diminishes the effect of S. exigua herbivory on terpenoid aldehyde production (Chen et al., 2008). Thus, nutrient availability likely impacts how cotton plants integrate both damage and insect-derived cues in their response.
4.5. Cotton as a model for teaching plants chemical ecology
The characteristics of cotton glands make Gossypium hirsutum a great model to teach the chemical ecology of plant defenses against insect herbivores. Cotton glands are a nice example of a constitutive as well as inducible direct defense. The unspecific response of cotton seedlings to physical damage allows to work without the need of having insects available. The timing of the response is ideal for working with classes, as a week can separate damage treatment and measurements. Gland density and gland size can be easily measured, without requirement of chemical analyses. On the other hand, the quantification of terpenoid aldehydes and volatile terpenes can be carried out with more advanced students to introduce HPLC-DAD and GC-MS as analytical tools. The possibility to perform a simple bioassay is also interesting. Choice assays can be readily performed with generalist caterpillars such as S. exigua and Spodoptera littoralis, if available. The above-mentioned factors that could influence induction should be taken in account. With low nitrogen availability, induction could be stronger (Chen et al., 2008), but we advise to use sufficient fertilization to ensure proper plant growth. We indeed observed that undamaged plants had bigger third leaves than damaged plants under a lower fertilization regime (data not shown). This effect on growth was not observed in the present study with well-fertilized plants. Having leaves of similar size makes the interpretation of the choice tests considerably easier. Indeed, if leaves of undamaged plants are bigger, their gland density is lower than damaged plants, making it difficult to disentangle the effect of leaf size and induction on caterpillar feeding behavior.
Data availability statement
The original contributions presented in the study are included in the article/Supplementary material, further inquiries can be directed to the corresponding author.
Author contributions
TCJT and MM designed the study. MM collected, analyzed, and interpreted the data and wrote the first draft of the manuscript. AV developed the method to analyze terpenoid aldehydes and analyzed the samples. All authors contributed to the article and approved the submitted version.
Funding
This work was funded by the Swiss National Science Foundation (grant 315230_185319).
Acknowledgments
We thank the undergraduate students who participated in some of the experiments as part of a practical class, Thierry Brévault of CIRAD for providing the cotton seeds, Carla M. Arce for her advice on the induction method and Mary V. Clancy for helping with the hexane extractions.
Conflict of interest
The authors declare that the research was conducted in the absence of any commercial or financial relationships that could be construed as a potential conflict of interest.
Publisher’s note
All claims expressed in this article are solely those of the authors and do not necessarily represent those of their affiliated organizations, or those of the publisher, the editors and the reviewers. Any product that may be evaluated in this article, or claim that may be made by its manufacturer, is not guaranteed or endorsed by the publisher.
Supplementary material
The Supplementary material for this article can be found online at: https://www.frontiersin.org/articles/10.3389/fevo.2023.1119472/full#supplementary-material
References
Acevedo, F. E., Rivera-Vega, L. J., Chung, S. H., Ray, S., and Felton, G. W. (2015). Cues from chewing insects—the intersection of DAMPs, HAMPs, MAMPs and effectors. Curr. Opin. Plant Biol. 26, 80–86. doi: 10.1016/j.pbi.2015.05.029
Agrawal, A. A., and Karban, R. (2000). Specificity of constitutive and induced resistance: pigment glands influence mites and caterpillars on cotton plants. Entomol. Exp. Appl. 96, 39–49. doi: 10.1046/j.1570-7458.2000.00677.x
Alborn, H. T., Hansen, T. V., Jones, T. H., Bennett, D. C., Tumlinson, J. H., Schmelz, E. A., et al. (2007). Disulfooxy fatty acids from the American bird grasshopper Schistocerca americana, elicitors of plant volatiles. Proc. Natl. Acad. Sci. U. S. A. 104, 12976–12981. doi: 10.1073/pnas.0705947104
Anderson, P., and Agrell, J. (2005). Within-plant variation in induced defence in developing leaves of cotton plants. Oecologia 144, 427–434. doi: 10.1007/s00442-005-0095-3
Anderson, P., Jönsson, M., and Mörte, U. (2001). Variation in damage to cotton affecting larval feeding preference of Spodotera littoralis. Entomol. Exp. Appl. 101, 191–198. doi: 10.1046/j.1570-7458.2001.00903.x
Arce, C. M., Besomi, G., Glauser, G., and Turlings, T. C. (2021). Caterpillar-induced volatile emissions in cotton: the relative importance of damage and insect-derived factors. Front. Plant Sci. 12:709858. doi: 10.3389/fpls.2021.709858
Bell, A. A., Stipanovic, R. D., O’Brien, D. H., and Fryxell, P. A. (1978). Sesquiterpenoid aldehyde quinones and derivatives in pigment glands of Gossypium. Phytochemistry 17, 1297–1305. doi: 10.1016/S0031-9422(00)94578-3
Bezemer, T. M., Wagenaar, R., van Dam, N. M., van Der Putten, W. H., and Wäckers, F. L. (2004). Above- and below-ground terpenoid aldehyde induction in cotton, Gossypium herbaceum, following root and leaf injury. J. Chem. Ecol. 30, 53–67. doi: 10.1023/B:JOEC.0000013182.50662.2a
Boege, K., and Marquis, R. J. (2005). Facing herbivory as you grow up: the ontogeny of resistance in plants. Trends Ecol. Evol. 20, 441–448. doi: 10.1016/j.tree.2005.05.001
Bottger, G., Sheehan, E. T., and Lukefahr, M. (1964). Relation of gossypol content of cotton plants to insect resistance. J. Econ. Entomol. 57, 283–285. doi: 10.1093/jee/57.2.283
Bricchi, I., Leitner, M., Foti, M., Mithöfer, A., Boland, W., and Maffei, M. E. (2010). Robotic mechanical wounding (MecWorm) versus herbivore-induced responses: early signaling and volatile emission in Lima bean (Phaseolus lunatus L.). Planta 232, 719–729. doi: 10.1007/s00425-010-1203-0
Brooks, M. E., Kristensen, K., Van Benthem, K. J., Magnusson, A., Berg, C. W., Nielsen, A., et al. (2017). Glmmtmb balances speed and flexibility among packages for zero-inflated generalized linear mixed modeling. R J. 9, 378–400. doi: 10.3929/ethz-b-000240890
Chen, Y., Schmelz, E. A., Wäckers, F., and Ruberson, J. R. (2008). Cotton plant, Gossypium hirsutum L., defense in response to nitrogen fertilization. J. Chem. Ecol. 34, 1553–1564. doi: 10.1007/s10886-008-9560-x
Clancy, M. V., Mamin, M., Flückiger, G., Quijano-Medina, T., Pérez-Niño, B., Abdala-Roberts, L., et al. (2023). Terpene chemotypes in Gossypium hirsutum (wild cotton) from the Yucatan peninsula, Mexico. Phytochemistry 205:113454. doi: 10.1016/j.phytochem.2022.113454
Duran-Flores, D., and Heil, M. (2016). Sources of specificity in plant damaged-self recognition. Curr. Opin. Plant Biol. 32, 77–87. doi: 10.1016/j.pbi.2016.06.019
Eisenring, M., Meissle, M., Hagenbucher, S., Naranjo, S. E., Wettstein, F., and Romeis, J. (2017). Cotton defense induction patterns under spatially, temporally and quantitatively varying herbivory levels. Front. Plant Sci. 8:234. doi: 10.3389/fpls.2017.00234
Elzen, G. W., Williams, H. J., Bell, A. A., Stipanovic, R. D., and Vinson, S. B. (1985). Quantification of volatile terpenes of glanded and glandless Gossypium hirsutum L. cultivars and lines by gas chromatography. J. Agric. Food Chem. 33, 1079–1082. doi: 10.1021/jf00066a015
Elzen, G., Williams, H., and Vinson, S. (1984). Isolation and identification of cotton synomones mediating searching behavior by parasitoid Campoletis sonorensis. J. Chem. Ecol. 10, 1251–1264. doi: 10.1007/BF00988552
Elzen, G., Williams, H., and Vinson, S. (1986). Wind tunnel flight responses by hymenopterous parasitoid Campoletis sonorensis to cotton cultivars and lines. Entomol. Exp. Appl. 42, 285–289. doi: 10.1111/j.1570-7458.1986.tb01034.x
Erb, M., and Reymond, P. (2019). Molecular interactions between plants and insect herbivores. Annu. Rev. Plant Biol. 70, 527–557. doi: 10.1146/annurev-arplant-050718-095910
Felton, G. W., and Tumlinson, J. H. (2008). Plant–insect dialogs: complex interactions at the plant–insect interface. Curr. Opin. Plant Biol. 11, 457–463. doi: 10.1016/j.pbi.2008.07.001
Fox, J., and Weisberg, S. (2019). An R companion to applied regression. 3rd ed. Thousand Oaks, CA: Sage
Gershenzon, J., McConkey, M. E., and Croteau, R. B. (2000). Regulation of monoterpene accumulation in leaves of peppermint. Plant Physiol. 122, 205–214. doi: 10.1104/pp.122.1.205
Gunasena, G. H., Vinson, S. B., Williams, H. J., and Stipanovic, R. D. (1988). Effects of caryophyllene, caryophyllene oxide, and their interaction with gossypol on the growth and development of Heliothis virescens (F.) (Lepidoptera: Noctuidae). J. Econ. Entomol. 81, 93–97. doi: 10.1093/jee/81.1.93
Hagenbucher, S., Olson, D. M., Ruberson, J. R., Wäckers, F. L., and Romeis, J. (2013). Resistance mechanisms against arthropod herbivores in cotton and their interactions with natural enemies. Crit. Rev. Plant Sci. 32, 458–482. doi: 10.1080/07352689.2013.809293
Heil, M. (2009). Damaged-self recognition in plant herbivore defence. Trends Plant Sci. 14, 356–363. doi: 10.1016/j.tplants.2009.04.002
Hothorn, T., Bretz, F., and Westfall, P. (2008). Simultaneous inference in general parametric models. Biom. J. 50, 346–363. doi: 10.1002/bimj.200810425
Jenkins, J. N., Maxwell, F. G., and Lafever, H. N. (1966). The comparative preference of insects for Glanded and glandless Cottons1. J. Econ. Entomol. 59, 352–356. doi: 10.1093/jee/59.2.352
Loughrin, J. H., Manukian, A., Heath, R. R., Turlings, T. C., and Tumlinson, J. H. (1994). Diurnal cycle of emission of induced volatile terpenoids by herbivore-injured cotton plant. Proc. Natl. Acad. Sci. U. S. A. 91, 11836–11840. doi: 10.1073/pnas.91.25.11836
Lüdecke, D. (2018). Ggeffects: tidy data frames of marginal effects from regression models. J. Open Source Software 3:772. doi: 10.21105/joss.00772
Lukefahr, M., Noble, L., and Houghtaling, J. (1966). Growth and infestation of bollworms and other insects on glanded and glandless strains of cotton. J. Econ. Entomol. 59, 817–820. doi: 10.1093/jee/59.4.817
McAuslane, H. J., and Alborn, H. T. (1998). Systemic induction of allelochemicals in glanded and glandless isogenic cotton by Spodoptera exigua feeding. J. Chem. Ecol. 24, 399–416. doi: 10.1023/A:1022596812049
McAuslane, H. J., Alborn, H. T., and Toth, J. P. (1997). Systemic induction of terpenoid aldehydes in cotton pigment glands by feeding of larval Spodoptera exigua. J. Chem. Ecol. 23, 2861–2879. doi: 10.1023/A:1022575313325
McCall, P. J., Turlings, T. C. J., Loughrin, J., Proveaux, A. T., and Tumlinson, J. H. (1994). Herbivore-induced volatile emissions from cotton (Gossypium hirsutum L.) seedlings. J. Chem. Ecol. 20, 3039–3050. doi: 10.1007/BF02033709
Opitz, S., Kunert, G., and Gershenzon, J. (2008). Increased terpenoid accumulation in cotton (Gossypium hirsutum) foliage is a general wound response. J. Chem. Ecol. 34, 508–522. doi: 10.1007/s10886-008-9453-z
Paré, P. W., and Tumlinson, J. H. (1997). De novo biosynthesis of volatiles induced by insect herbivory in cotton plants. Plant Physiol. 114:7. doi: 10.1016/S0031-9422(97)00442-1
Quintero, C., and Bowers, M. D. (2011). Plant induced defenses depend more on plant age than previous history of damage: implications for plant-herbivore interactions. J. Chem. Ecol. 37, 992–1001. doi: 10.1007/s10886-011-0007-4
R Core Team (2022). R: A language and environment for statistical computing. Vienna, Austria: R Foundation for Statistical Computing.
Rose, U. S., Manukian, A., Heath, R. R., and Tumlinson, J. H. (1996). Volatile semiochemicals released from undamaged cotton leaves (a systemic response of living plants to caterpillar damage). Plant Physiology 111, 487–495.
Schindelin, J., Arganda-Carreras, I., Frise, E., Kaynig, V., Longair, M., Pietzsch, T., et al. (2012). Fiji: an open-source platform for biological-image analysis. Nat. Methods 9, 676–682. doi: 10.1038/nmeth.2019
Shangguan, X., Zhang, J., Liu, B., Zhao, Y., Wang, H., Wang, Z., et al. (2018). A mucin-like protein of planthopper is required for feeding and induces immunity response in plants. Plant Physiol. 176, 552–565. doi: 10.1104/pp.17.00755
Si, H., Liu, H., Sun, Y., Xu, Z., Liang, S., Li, B., et al. (2020). Transcriptome and metabolome analysis reveal that oral secretions from Helicoverpa armigera and Spodoptera litura influence wound-induced host response in cotton. Crop J. 8, 929–942. doi: 10.1016/j.cj.2019.12.007
Stahl, E., Brillatz, T., Queiroz, E. F., Marcourt, L., Schmiesing, A., Hilfiker, O., et al. (2020). Phosphatidylcholines from Pieris brassicae eggs activate an immune response in Arabidopsis. eLife 9:e60293. doi: 10.7554/eLife.60293
Turlings, T. C., and Erb, M. (2018). Tritrophic interactions mediated by herbivore-induced plant volatiles: mechanisms, ecological relevance, and application potential. Annu. Rev. Entomol. 63, 433–452. doi: 10.1146/annurev-ento-020117-043507
Turlings, T. C., McCall, P. J., Alborn, H. T., and Tumlinson, J. H. (1993). An elicitor in caterpillar oral secretions that induces corn seedlings to emit chemical signals attractive to parasitic wasps. J. Chem. Ecol. 19, 411–425. doi: 10.1007/BF00994314
Turner, G. W., Gershenzon, J., and Croteau, R. B. (2000). Distribution of peltate glandular trichomes on developing leaves of peppermint. Plant Physiol. 124, 655–664. doi: 10.1104/pp.124.2.665
Venables, W. N., and Ripley, B. D. (2002). Modern applied statistics with S. 4th ed. New York: Springer.
Wu, J., and Baldwin, I. T. (2009). Herbivory-induced signalling in plants: perception and action. Plant Cell Environ. 32, 1161–1174. doi: 10.1111/j.1365-3040.2009.01943.x
Yang, J.-O., Nakayama, N., Toda, K., Tebayashi, S., and Kim, C.-S. (2014). Structural determination of elicitors in Sogatella furcifera (Horváth) that induce japonica rice plant varieties (Oryza sativa L.) to produce an ovicidal substance against S. furcifera eggs. Biosci. Biotechnol. Biochem. 78, 937–942. doi: 10.1080/09168451.2014.917266
Zebelo, S., Disi, J., Balusu, R., Reeves, B., and Fadamiro, H. (2017). Spodoptera exigua modulates gossypol biosynthesis in cotton Gossypium hirsutum. J. Plant Interact. 12, 121–127. doi: 10.1080/17429145.2017.1298853
Zhang, H., Liu, E., Huang, X., Kou, J., Teng, D., Lv, B., et al. (2022). Characterization of a novel insect-induced sesquiterpene synthase gbtps1 based on the transcriptome of Gossypium barbadense feeding by cotton bollworm. Front. Plant Sci. 13:898541. doi: 10.3389/fpls.2022.898541
Zhang, L., Lu, G., Huang, X., Guo, H., Su, X., Han, L., et al. (2020). Overexpression of the caryophyllene synthase gene GhTPS1 in cotton negatively affects multiple pests while attracting parasitoids. Pest Manag. Sci. 76, 1722–1730. doi: 10.1002/ps.5695
Keywords: cotton, gossypol glands, terpenoid aldehydes, volatile terpenes, constitutive and induced plant defense, mechanical damage, Spodoptera exigua, teaching chemical ecology
Citation: Mamin M, Vallat A and Turlings TCJ (2023) Cotton plants as ideal models for teaching and research on inducible direct plant defenses. Front. Ecol. Evol. 11:1119472. doi: 10.3389/fevo.2023.1119472
Edited by:
Ping Wen, Xishuangbanna Tropical Botanical Garden (CAS), ChinaReviewed by:
Masayoshi Uefune, Meijo University, JapanShanchun Yan, Northeast Forestry University, China
Copyright © 2023 Mamin, Vallat and Turlings. This is an open-access article distributed under the terms of the Creative Commons Attribution License (CC BY). The use, distribution or reproduction in other forums is permitted, provided the original author(s) and the copyright owner(s) are credited and that the original publication in this journal is cited, in accordance with accepted academic practice. No use, distribution or reproduction is permitted which does not comply with these terms.
*Correspondence: Ted C. J. Turlings, ✉ dGVkLnR1cmxpbmdzQHVuaW5lLmNo