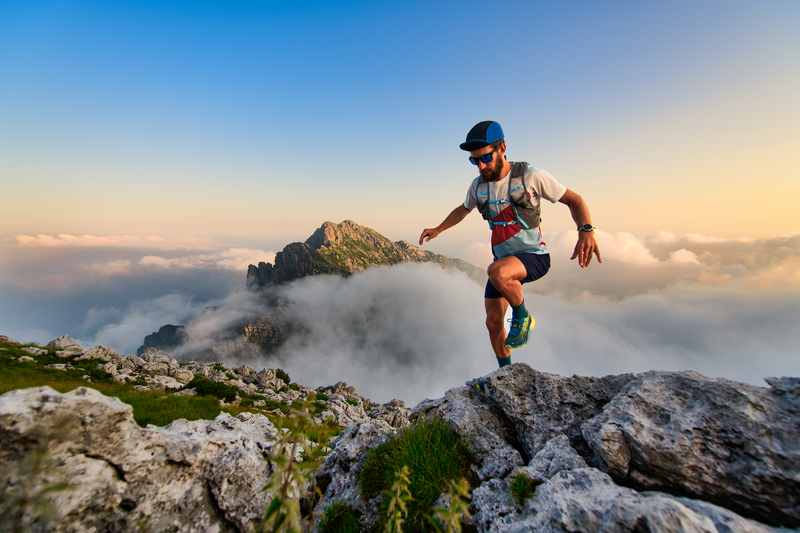
95% of researchers rate our articles as excellent or good
Learn more about the work of our research integrity team to safeguard the quality of each article we publish.
Find out more
ORIGINAL RESEARCH article
Front. Ecol. Evol. , 21 February 2023
Sec. Population, Community, and Ecosystem Dynamics
Volume 11 - 2023 | https://doi.org/10.3389/fevo.2023.1106122
This article is part of the Research Topic The Role of Soil Mesofauna as Indicators of Sustainable Ecosystem Management Plans View all 5 articles
Introduction: The biomass and nutrient allocation strategies in plants are fundamental for predicting carbon storage and mineral and nutrient cycles in terrestrial ecosystems. However, our knowledge regarding the effects of multiple environmental factors on biomass and nutrient allocation remains limited.
Methods: Here we manipulated soil composition (three levels), arbuscular mycorrhizal fungi inoculation (AMF, five levels), and root-knot nematode inoculation (RKN, two levels) using random block design to reveal the effects of these factors on biomass and nutrient allocation strategies of cherry tomato.
Results and Discussion: Our results showed that biomass and nutrient allocation were affected by soil composition, AMF and RKN individually or interactively. The biomass and nutrient allocation in cherry tomato shows different adaptation strategies responded to the joint action of three factors. The reduction of soil nutrients increased belowground biomass allocation, and aboveground nitrogen and phosphorus concentration. AMF colonization increased aboveground biomass allocation and reproductive investment and promoted aboveground nitrogen and phosphorus inputs. Cherry tomato can mitigate the stress of RKN infection by investing more biomass and nutrients into belowground organs. Our study showed that plants can adjust their survival strategies by changing biomass and nutrient allocation to adapt to variation in soil abiotic and biotic factors. These findings contribute to our understanding of the adaptive processes of plant biomass and nutrient allocation strategies under multiple environmental factors.
The carbon dynamics and nutrient cycling processes in terrestrial ecosystems depend on plant carbon and nutrient economics (Elbasiouny et al., 2022; Jevon and Lang, 2022). Plants allocate carbon and nutrients to their aboveground and belowground organs reasonably to meet their normal growth and reproduction (Cheplick, 2020; Irving and Mori, 2021). In general, the root-shoot ratio (root biomass to aboveground biomass) and reproductive allocation (reproductive organ biomass to total biomass) can be used to determine the resource allocation strategies of plants for vegetative growth and reproduction, which are also key parameters reflecting plant survival strategies (Liu and Li, 2020; Li et al., 2021; Zhou et al., 2021). The resource allocation strategies of plants may change with resource supply changes in environment, which will lead to changes in root-shoot ratio and reproductive investment for plants to adapt to variable conditions (Zhang et al., 2021; Bebre et al., 2022; Meng et al., 2022; Zhou et al., 2022). Therefore, as one of the adaptation strategies to environmental factors, the resource allocation strategy of plants is usually plastic (Xie et al., 2012).
The belowground properties of terrestrial ecosystems (e.g., nutrients, mycorrhizal symbiosis, and soil herbivores) can significantly affect the resource allocation strategies of plants. For instance, plants often improve resource uptake by increasing biomass allocation to roots when soil nutrient supply decreases (Irving and Mori, 2021). In addition, symbionts between roots and arbuscular mycorrhizal fungi (AMF) that are prevalent in soil ecosystems can also alter the allocation of plant carbon and nutrients (Cavagnaro et al., 2014; Salmeron-Santiago et al., 2022; Wang R. et al., 2022; Zhou et al., 2022). Herbivorous nematodes are widely distributed in soil ecosystems worldwide as a member of the soil food web, especially in agricultural ecosystems (Schouteden et al., 2015). The destructive foraging of herbivorous nematodes to plant roots can cause carbon transfer belowground, which directly affects the resource allocation of plants (Poll et al., 2007). Therefore, the effects of biotic and abiotic factors on the carbon pool and mineral or nutrient distribution in soil ecosystems should be an important issue that we need to pay attention to. In fact, plants are routinely subjected to a variety of abiotic and biotic stress factors simultaneously in their natural habitat. The combination of two stresses (abiotic-abiotic or abiotic-biotic) does not always lead to negative impact on plants (Pandey et al., 2017). Some stress combinations negate the effect of each other, leading to a net neutral or positive impact on plants. One stress may also provide endurance to plants against another stress and hence plant growth is not always negatively affected (Pandey et al., 2017). Thus, it is important to fully recognize the impact of combined abiotic and biotic stresses on plants to understand the nature of multiple soil factors interaction.
Plant resource allocation can be theoretically predicted using optimal partitioning theory, which indicates that plants tend to allocate resources to organs that have access to more restricted resources to promote growth (Kobe et al., 2010; Liu and Li, 2020). Although the optimal partitioning theory can well explain the impact of environmental changes on biomass allocation, one of its limitations is that it only assumes the change of one kind of resource, and we are still unclear about the resource allocation strategies of plants under the changes of multiple environmental factors (Gleeson and Good, 2003). Plants may be more inclined to allocate more biomass to the functional organs affected by environmental stress when facing limited soil nutrient resources or are foraged by herbivores, and may regulate the distribution patterns of nitrogen and phosphorus to promote growth and reproduction (Xie et al., 2012; Wang et al., 2019). In general, AMF and herbivorous nematodes have completely opposite ecological effects and show positive and negative effects on plant growth and nutrient uptake (Tikoria et al., 2022; Tran et al., 2022). According to the optimal partitioning hypothesis, it can be predicted that when plants are parasitized by herbivorous nematodes, their root nutrient uptake will be limited, which will lead to an increase in the belowground biomass allocation. The symbiotic benefits of AMF are reflected in the enhancement of nutrient uptake and plant resistance to herbivorous nematodes, which alleviates the resource transfer to the belowground organs caused by herbivorous nematode foraging (Schouteden et al., 2015). Most notably, different AMF species may play different roles in the allocation of plant resources after symbiosis with plants (Lerat et al., 2003). Therefore, we can predict that the allocation of plant biomass and nutrients may be regulated by soil nutrients, AMF species, and herbivorous nematodes. Unfortunately, the complex ecological relationships among multiple factors in soil hinder our comprehensive understanding of plant carbon allocation and nutritional economics.
Recent researches suggest that plants may distinguish between mycorrhizal symbionts and pathogenic symbionts when they coexist on roots, and that plants preferentially allocate photosynthates to mycorrhizal symbionts when they perceive the benefits of mycorrhizal symbionts (Bever et al., 2009; Bell et al., 2022). In this case, AMF may be rewarded more photosynthates by increasing nutrient supply to host plants (Bell et al., 2022). When plants are unable to distinguish two competing root symbionts (AMF and herbivorous nematodes) due to their proximity to each other in the root system and have overlapping ecological niche, this will ultimately lead to restricted carbon allocation to the root system (Albornoz et al., 2022; Bell et al., 2022). Competition for carbon sources and space between AMF and herbivorous nematodes is also reflected in their mutual inhibition during their reproduction and colonization processes (Kesba and Al-Sayed, 2006; Schouteden et al., 2015). It is worth noting that soil resource availability can also affect the stable establishment of symbiosis between AMF and plants, as well as the survival and reproduction of herbivorous nematodes in soil (Schmidt et al., 2011; Sheldrake et al., 2018; Harkes et al., 2020; Pan et al., 2020). However, the effects of soil-AMF-herbivorous nematode interactions on plant carbon and nutrient allocation patterns are still unclear.
In this study, our main objectives were to investigate how plants adapt to nutrients and spatial competition between AMF and herbivorous nematodes under different soil compositions. Our work sheds light on the corresponding biomass and nutrient allocation strategies. According to the optimal partitioning theory and the benefits of AMF symbiosis, we hypothesized: (a) plant biomass and nutrient allocations were individually and interactively affected by soil resource availability, AMF, and herbivorous nematodes; (b) soil nutrients and AMF affect the allocations of aboveground and belowground biomass, with lower soil nutrients increasing plant carbon allocation to belowground organs, while the AMF mutualistic symbionts favors aboveground carbon accumulation and nitrogen and phosphorus uptake; (c) herbivorous nematode infection leads to reallocation of aboveground and belowground biomass of plants, resulting in greater carbon and nutrient allocation to belowground organs. We designed a randomized block experiment in a greenhouse to verify above hypotheses. The biomass and nutrient allocation patterns of plants under different soil nutrient levels, AMF and root-knot nematode inoculation were investigated to verify whether the adaptation strategies of plants were regulated by multiple environmental factors.
We selected dwarf cherry tomato (Solanum lycopersicum var. cerasiforme) as the study plant, which is a relatively common fruit crop in the market. It is a self-compatible variety with the advantages of short height and short growth cycles, and it is very convenient for cultivation (Rahim et al., 2019; Wang L. et al., 2022). The cherry tomato seeds (purchased from Beijing Dongsheng Seed Industry Co., LTD.) were surface-sterilized with 0.5% potassium permanganate for 5 min and 5% sodium hypochlorite for 1 min, and thoroughly rinsed with sterile water. Then, seeds were placed in petri dishes with moist filter paper, and germinated in a 25°C constant light incubator (humidity 60%) to obtain cherry tomato seedlings with two cotyledons.
The soil substrate was experimentally prepared by changing the ratio between peat soil and sand for the purpose to provide a nutrient gradient. In this study, three soil composition treatments were set as follows: 1:1, 1:2 and 1:3 (peat soil: sand, volume). The soil substrate was sterilized subsequently at 121°C for 2 h twice (with an interval of 3 days, 4 h in total), and then was put into the sterilized pots (diameter: 16.5 cm, height: 12.5 cm) after natural cooling, with 2 l of soil substrate in each pot. The nutrient contents of the three soil composition treatments after sterilization were measured. The contents of organic matter, nitrogen (total nitrogen and available nitrogen), phosphorus (total phosphorus and available phosphorus) and potassium (available potassium) in the three soil composition treatments decreased with the decrease of peat proportion (Supplementary Table S1).
Three species of arbuscular mycorrhizal fungi (AMF) (Funnelliformis mosseae, Rhizophagus intraradices, and Glomus versiforme) were used as materials for fungal inoculation in this study. Three AMF agents were purchased from the Bank of Arbuscular Mycorrhizal Fungi in China (BGC) (Supplementary Table S2). Three AMF strains containing 25 spores per gram of inoculant were obtained by dilution using sterilized sand. In order to better reveal the joint interaction mechanism of multiple AMF species, three AMF strains were mixed equally by weight and evenly to get a mixture containing three species of AMF, which was used for AMF mixture inoculation treatment. The total spore density of the mixture treatment was also 25 mixed spores per gram mixture. Four AMF agents containing the same spore density were obtained to ensure that the number of AMF spores initially inoculated was consistent.
Meloidogyne incognita, which acts as a common root-knot nematode (RKN) and is known to have a strong ability to infect and reproduce on tomato roots, was used in this study. The second-stage juveniles (J2) of a pure population of M. incognita were provided by the Northeast Institute of Geography and Agroecology, Chinese Academy of Sciences.
A three-factor completely randomized block design was conducted in this study. Soil composition [three levels: 1:1, 1:2, and 1:3 (volume ratio, peat: sand)], AMF [five levels: no inoculation (CK), F. mosseae (Fm), R. intraradices (Ri), G. versiforme (Gv) and mixed inoculum (Ma)] and root-knot nematodes [two levels: inoculated with RKN (+RKN) and no RKN (−RKN)] were used as the main factors to explore the response strategies of the biomass and nutrient allocation of plant under the three factors individually or interactively. There were 30 treatment combinations (3 × 5 × 2) in this study together, and 6 replicates per treatment combination for a total of 180 pots.
According to the experimental design, 40 g (containing 1,000 spores) corresponding AMF agent was added to the soil substrate in pot belong to the corresponding treatments. After moistening the soil substrate with pure water, we gently placed a cherry tomato seedling with two cotyledons on the AMF agent to ensure that the root system of the seedling was fully exposed to the AMF agent. Then a small amount of soil substrate was used to cover the fungus and seedling roots and keep the stems and cotyledons were exposed above the surface of the soil substrate, and a small amount of pure water was sprayed to keep the substrate around the seedling roots moist. In the control treatment, 40 ml water filtrate with non-sterilizing AMF agent (filtered by a 20 μm filter) and sterilizing AMF agent in equal amount were added. All the pots were placed in the greenhouse for tomato seeding culture after AMF inoculation.
Seedlings inoculated with AMF (including the control treatment) were cultured in greenhouse for 35 days and then the half of each treatment combination was inoculated with RKN. Four holes (2 cm depth) were dug evenly at a distance of 1.5 cm away from the stem of seedling to ensure that the root system could be exposed. Then, the RKN suspension containing 2000 J2 was evenly injected into the four holes around the stem of each seedling. For the other half treatment combination, no nematode was inoculated, and only the same amount of nematode filtrate was inoculated (i.e., the same volume of nematode-containing suspension was sieved through 500 mesh).
All the plants were randomly arranged in the greenhouse for cultivation again after RKN inoculation. The photoperiod of the greenhouse cultivation was 12 h/12 h (light/dark), the light quantum flux density was 550–600 μmol/m2s, the temperature was 25°C, and the humidity was 60 ± 10%. The seedlings were checked every day and watered (purified water) to keep the soil moist. The positions of plants were randomly changed every week. The plants were cultured for 85 days after inoculation with RKN, for a total of 120 days of cultivation in greenhouse.
Plants were harvested on the 120th day when most fruits (about 95%) were ripe. We used scissors to separate the aboveground and belowground parts of the plants. The aboveground part was separated to the stems, leaves, fruits and then packed into paper envelopes. Roots were carefully removed from the soil substrate and the fresh weight was measured after they were gently washed and dried with absorbent paper. Finally, the stems, leaves, roots and fruits of all plants were put into a drying oven at 80°C to dry for 48 h to determine their dry weight. The total biomass was defined as the total dry weight of stems, leaves, roots, and fruits of each plant. The root-shoot ratio and reproductive investment were also calculated. The root-shoot ratio was the ratio of the dry weight of roots to the dry weight of aboveground parts (stems, leaves, and fruits). Reproductive investment (%) was the percentage of the fruit biomass to the total biomass of plant (calculated by dry weight).
After the dried plant samples were crushed and sieved (100 mesh), nitrogen and phosphorus concentrations in the aboveground (stems and leaves) and belowground (roots) parts of the plants were determined using an elemental analyzer (EA3100, Italy) and molybdate colorimetry, respectively. Phosphates were extracted by digesting 0.2 g sample with 5 ml 1.84 g/ml H2SO4 and 6 ml 30% H2O2, then the neutralized extracts were transferred and 5 ml molybdenum antimony anti indicator was added. Finally, the absorbance value was measured at 700 nm via automated molybdate colorimetry using Shimadzu UV-1240 ultraviolet spectrophotometer to determine the concentrations of nitrogen and phosphorus in aboveground and belowground parts (Sun et al., 2022).
The shapiro–wilk test (at 0.05) was used to explore the normality of residuals, and the Bartlett test was used to detect the homogeneity of variances before analysis. All analyzed data conform to normal distribution and homogeneity of variance after outliers were eliminated. We explored the effects of soil composition, AMF, and RKN and their interactions on biomass allocation and nutrient uptake using a general model of three-way ANOVA. Duncan’s test was used for post hoc multiple comparisons. We also used independent sample t-tests to determine the differences in the above parameters between treatments with or without AMF inoculation and treatments with and without RKN addition at each soil composition (including all controls). Differences were considered significant if p < 0.05. Principal component analysis (PCA) was used to explore the relationship between plant trait variables in interaction effects of three factors after the data were normalized. All statistical analyses were performed using SPSS (25.0) (SPSS Inc., Chicago, IL, United States).
The total biomass was significantly affected by soil composition, AMF and RKN (Table 1). The total biomass significantly decreased with the soil nutrient reduction (the 1:2 and 1:3 treatment, respectively, decreased by 35.26% and 39.60% compared with 1:1 treatment) or RKN infection (−18.78%), whereas only Gv inoculation significantly increased the total biomass (+6.85%) (Figure 1A). There were significant interaction effects between soil composition and AMF and between soil composition and RKN on the total biomass (Table 1). The interaction of soil nutrient reduction either with AMF inoculation, or with RKN infection significantly decreased the total biomass (Figure 2A).
Table 1. Effects of soil composition, AMF, and RKN and their interactions on total biomass and biomass allocation in cherry tomato.
Figure 1. Single treatment effects of three factors. Soil composition, AMF inoculation and RKN infection and their mean effects on (A) total biomass, (B) root-shoot ratio, and (C) reproductive investment in cherry tomato. The points represent data. Different letters indicate significant differences by Duncan test (p < 0.05) among different treatments. The broken lines in the boxplot were the connections between means. The bold line within each boxplot represents the mean value and whiskers represent outliers (1.5 times the SD).
Figure 2. Interactive effects of three factors. Soil composition, AMF inoculation, RKN infection and their interactive effects on (A) total biomass, (B) root-shoot ratio, (C) reproductive investment, (D) aboveground nitrogen concentration, (E) belowground nitrogen concentration, (F) aboveground phosphorus concentration and (G) belowground phosphorus concentration in cherry tomato. Different letters indicate significant differences by Duncan test (p < 0.05) among different treatments. The whiskers in the bar graph represent the standard errors (SE).
The results showed that the root-shoot ratio was significantly affected by soil composition, AMF and RKN (Table 1). The root-shoot ratio was significantly increased with the reduction of peat soil ratio in the soil substrate (the ratio of 1:2 was the highest (+88.54%) and followed by 1:3 (+37.11%) compared with 1:1) and it also significantly increased with the RKN infection (+51.70%). Conversely, the inoculation with Ri (−23.01%), Gv (−25.30%) and Ma (−27.50%) significantly reduced the root-shoot ratio (Figure 1B). All interaction combinations of soil composition, AMF and RKN also significantly affected the root-shoot ratio (Table 1). The root-shoot ratio significantly increased under the combined action of AMF and RKN with the reduction of nutrients, and indicated that the biomass tends to be allocated to the belowground organs (Figure 2B).
Reproductive investment was significantly affected by soil composition and AMF (Table 1). Reproductive investment when soil composition was 1: 2 was significantly lower than that at the other two ratios, and Ma inoculation significantly increased reproductive investment (+22.56%). In addition, RKN infection had no significant effect on reproductive investment (Figure 1C). The interactions between soil composition and AMF and between soil composition and RKN had significantly effects on reproductive investment (Table 1). The reproductive investments were higher when the soil composition was 1:1 or 1:3 than that of 1:2 in RKN infected plants (Figure 2C).
The aboveground nitrogen (N) concentration was significantly affected by soil composition and AMF (Table 2). The aboveground N concentration when the soil composition was 1:3 was significantly higher than that at the other two ratios, and inoculated with Ri (+18.85%), Ma (+33.17%) and Gv (+38.88%) also significantly increased the aboveground N concentration. Furthermore, aboveground N concentration was not significantly affected whether RKN infection or not (Figure 3A). There were significant interactions between soil composition and AMF, and among soil, AMF and RKN (Table 2). The aboveground N concentration significantly increased with the soil nutrient reduction after AMF inoculation and it tended to increase with nutrient reduction under the combined action of AMF and RKN (Figure 2D).
Table 2. Effects of soil composition, AMF, and RKN and their interactions on nitrogen and phosphorus concentration.
Figure 3. Single treatment effects of three factors. Soil composition, AMF inoculation and RKN infection and their mean effects on (A) aboveground and belowground nitrogen concentration and (B) aboveground and belowground phosphorus concentration in cherry tomato. Different lower-case letters indicate significant differences between the aboveground nutrient concentrations of different treatments, and different capital letters indicate significant differences between the belowground nutrient concentrations of different treatments (p < 0.05). The whiskers represent the standard errors (SE).
Soil composition, AMF and RKN had significant effects on the belowground N concentration (Table 2). The belowground N concentration significantly decreased with the soil nutrient reduction, while it significantly increased with inoculation of Fm (+7.65%), Ma (+7.98%) and Gv (+12.46%) or RKN infection (+31.05%) (Figure 3A). The interactions of all cross combinations of the three factors had significant effects on the belowground N concentration (Table 2). The belowground N concentration significantly decreased with soil nutrient reduction when AMF colonized or AMF coexisted with RKN. On the contrary was the belowground N concentration increased at soil composition was 1:1 in the AMF and RKN interactions (Figure 2E).
The aboveground phosphorus (P) concentration was significantly affected by soil composition and AMF (Table 2). The aboveground P concentration increased significantly with the soil nutrient reduction (the 1:2 and 1:3 treatment, respectively, increased by 60.04 and 105.74% compared with 1:1 treatment) and inoculation of Fm (+39.46%) and Ma (+76.70%), whereas it was not affected by RKN infection (Figure 3B). The aboveground P concentration was also significantly affected by the interaction between soil composition and AMF, and the combined action of soil composition, AMF and RKN (Table 2). The aboveground P concentration in 1:3 soil composition treatment was significantly increased than 1:1 composition treatment no matter whether AMF was inoculated or not, and that is also true for the interaction of AMF and RKN (Figure 2F).
The results showed that the belowground P concentration was significantly affected by AMF and RKN (Table 2). Ri (−12.52%) and Gv (−18.76%) inoculation significantly reduced the belowground P concentration, while RKN infection significantly increased the belowground P concentration (+7.33%) (Figure 3B). Similarly, the interaction of all the cross combinations of the three factors had significant effect on the belowground P concentration (Table 2). The belowground P concentration of 1:3 soil composition treatment was significantly higher than that of 1:1 soil composition, and the belowground P concentration decreased significantly when AMF was inoculated under 1:3 soil composition treatment. The belowground P concentration of 1:3 soil composition treatment was significantly decreased than that of 1:2 soil composition when only RKN was added (Figure 2G).
N and P concentrations showed different responses to AMF inoculation and RKN infection under different soil composition conditions. So, to understand the effects of soil composition, AMF inoculation and RKN infection on distribution patterns of N and P, N and P concentrations (per gram dry biomass) in aboveground and belowground tissues were measured. The results showed that the differences of N and P concentrations were affected by soil composition, AMF and RKN. Soil nutrient reduction and AMF inoculation significantly promoted the transfer of N and P into aboveground tissues, and increased the concentrations of N and P in aboveground tissues, while RKN infection resulted in allocation of N into belowground tissues. The results also showed that plants allocated more N and P to the aboveground parts under the interaction of three factor combinations (Figures 2D–G; Supplementary Figure S1).
Principal component analysis (PCA) showed that PC1 and PC2 accounted for 53.7% of the total variance under the combined action of soil composition, AMF colonization and RKN infection. PC1 captured 27.6% of the total variation and had a greater linear association with total biomass, root-shoot ratio, reproductive investment and aboveground P concentration. On the other hand, aboveground P and N concentration, root-shoot ratio and reproductive investment excelled on PC2 that corresponds to 26.1% of the total variation (Figure 4). Total biomass was significantly positively correlated with belowground N and P concentrations, but significantly negatively correlated with aboveground N and P concentrations. We found that the root-shoot ratio was not regulated by the total biomass. Moreover, there were no significant relationships between root-shoot ratio or reproductive investment and P or N concentration (Figure 4). PCA based on the three factors showed significant differences between the three soil compositions or two AMF treatments (inoculated or not), while there was no separation between RKN infection and no RKN infection treatments (Figure 4; Supplementary Figure S2).
Figure 4. Ordination and classification of phenotypic traits of cherry tomato under the interaction of soil composition, AMF colonization and RKN infection based on principal component analysis (PCA). The points represent data. The arrows represent the original variables and the circles were 95% confidence ellipses. The traits included total biomass (TB), root-shoot ratio (RS), reproductive investment (RI), aboveground nitrogen concentration (AN), aboveground phosphorus concentration (AP), belowground nitrogen concentration (BN) and belowground phosphorus concentration (BP) (values were centered and standardized).
Our results showed that the total biomass was significantly affected by soil composition, AMF colonization and RKN infection, among which soil was the dominant factor, and its interaction with AMF or RKN also could affect the total biomass. These results indicated that the total biomass accumulation was dependent on the availability of soil nutrients on the one hand, and beneficial fungi and herbivorous nematodes in soil also played a regulatory role in the total biomass accumulation. The total biomass decreased with the decrease of soil nutrients and RKN infection. This is understandable because in natural ecosystems, the promotion of plant net primary productivity and biomass accumulation require an increase in the supply of nutrients (nitrogen and phosphorus, etc.) (Cleveland et al., 2013). Consequently, the decrease of soil nutrient availability directly inhibited the increase of plant primary productivity. Meanwhile, the feeding of RKN on roots and the obstruction of root nutrient transport due to RKN parasitism significantly reduced plant nutrient uptake and photosynthate accumulation, finally resulting in the inhibition of biomass accumulation (Sharma and Sharma, 2019; Cunha et al., 2022). In contrast, AMF inoculation can promote plant growth and biomass accumulation, improve net primary productivity and increase carbon fixation in the ecosystem, but this benefit varies with AMF species (Martignoni et al., 2021). Therefore, as we hypothesized, soil nutrient availability, AMF and RKN have direct effects on ecosystem carbon pools, and it can be predicted that increased soil nutrient availability and AMF colonization can improve carbon storage in plants. However, RKN infection may reduce the benefits of AMF colonization, thus the carbon fixation capacity of plants is full of uncertainty due to the complex interactions of soil factors. It is well known that the interaction and feedback between plants and belowground soil resources, including biota, is the core of maintaining the function and sustainability of terrestrial ecosystems (Chen et al., 2022a,b; Chen Q. L. et al., 2022). We found a stronger interactive effect among soil conditions, symbiotic fungus and parasitic nematodes on biomass allocation, furthermore, they even may play the opposite role. So, we cannot ignore the different benefits of biotic and abiotic factors in soil when considering biomass carbon dynamics in terrestrial ecosystems.
Our results indicated that root-shoot ratio and reproductive investment underwent plastic changes in response to soil composition change, AMF colonization and RKN infection. The root-shoot ratio reflects the trade-off strategy in the process of plant biomass allocation. Plants usually allocate more resources to aboveground organs for growth in suitable habitats and more resources to belowground organs for survival in disturbed habitat, which leads to the huge difference in plant root-shoot ratio between these two habitats (Robinson et al., 2010). It was consistent with our results that the root-shoot ratio increased significantly after nutrient reduction. One explanation for the increase of root-shoot ratio is that plants allocate more biomass to the root system to improve the ability of nutrient foraging (Portsmuth and Niinemets, 2007; Irving and Mori, 2021). Another explanation is that in addition to maintaining root growth and older root respiration, plants allocate more carbon belowground to maintain mycorrhizal symbiosis and provide more carbon for RKN consumption (Robin et al., 2018). This response pattern to rhizosphere organisms is especially obvious under low nutrient condition. If plants do not have enough nutrients to support aboveground photosynthesis, increasing the root-shoot ratio may be a good way for plants to balance their carbon budget (Irving and Mori, 2021).
In addition, we also verified that the existence of mycorrhizal symbiosis alleviated the excessive resource investment to the belowground organs (roots) of plant, so that more resources could be used to improve aboveground photosynthesis (Wright et al., 1998). RKN infection had a negative effect on the nutrient uptake of roots and resulted in an increase in the root-shoot ratio. This result suggested that plants will invest more photosynthate to belowground roots to alleviate the damage of root function caused by RKN (Poll et al., 2007). Thus, AMF and RKN show completely opposite ecological effects on the belowground carbon pool of ecosystem (Figure 5).
Figure 5. Effects of reduced soil nutrients, AMF inoculation and RKN infestation on carbon, nitrogen and phosphorus allocation patterns in cherry tomato. C represents biomass carbon allocation; N represents nitrogen allocation and P represents phosphorus allocation. The arrow indicates the input direction. EH means AMF extraradical hyphae. Galls means RKN galls. (A) Root AMF colonization promoted the allocation of C, N, and P to the aboveground organs, while RKN infection induced the input of C, N, and P to the belowground organs. (B) Under the interaction of soil nutrients, arbuscular mycorrhizal and RKN, nitrogen and phosphorus are preferentially allocated to the aboveground organs to meet the needs of photosynthesis and reproduction, while carbon is mainly input to the belowground organs to alleviate the nutrient limitation and nutrient uptake block caused by RKN and meet the carbon demand of root symbionts.
It is worth noting that the resource allocation trade-off between reproductive organs and vegetative organs is also an important process for plants to adapt to habitat changes. That the reproductive investment decreased firstly and then increased with the decrease of soil nutrients was consistent with our expectations. It is easy to understand that the higher resource availability in soil can meet the investment demand of plants in reproduction. Plants will also tend to increase the investment of reproduction under low nutrient conditions to ensure the survival of more offspring and to maintain the regeneration of their population. Although studies have shown that plants in nutrient constrained habitats tend to invest less resources in sexual reproduction, mainly because of the high cost for sexual reproduction (Fujita et al., 2014). However, we found that cherry tomato can improve reproductive investment under low nutrient conditions. One possible reason is that plants increase resource investment in reproduction to ensure their reproductive success under the joint action of AMF inoculation and RKN infection.
Mycorrhizal symbionts can significantly increase the reproductive investment of the host plant, which can be attributed to the nutrient supply (especially the aboveground phosphorus supply) to the host plant by the mycorrhizal symbionts (Ramírez-Flores et al., 2019). Importantly, AMF can facilitate phosphorus acquisition by plants under resource-constrained conditions and reduce the belowground resource investment of host plant, resulting in a higher benefit/return to aboveground biomass (reproduction and photosynthesis) (Qi et al., 2022). Our results suggested that soil nutrient differences could determine the belowground carbon storage capacity of plant and it was also affected by different ecological factors such as mycorrhizal symbiosis and RKN infection. In response to biotic and abiotic factors, plants show the appropriate biomass allocation strategy to the aboveground and belowground organs, which not only meets the needs of their growth and survival, but also provides resources for maintaining population regeneration.
This study found that the allocation patterns of N and P were affected by soil compositions, AMF colonization and RKN infection. The results suggested that the reasonable allocation of N and P may be an important way for plants to adapt to variable environmental conditions. We found that N and P were largely transferred to the aboveground organs when the soil nutrient declined, which may be an adaption strategy to ensure plant survival (Figure 5; Supplementary Figure S1). Because more nutrients are allocated to the aboveground organs of plants, it is beneficial to meet the photosynthetic needs of leaves and reproductive resource demand, and to improve their fitness under adverse conditions (Shen et al., 2020). We also found that AMF symbiosis had positive effects on N concentration and aboveground P concentration of cherry tomato, but had negative effects on belowground P concentration, and it is worth noting that these effects varied with AMF species (Figures 3, 5). Although some studies suggested that AMF colonization is beneficial to the uptake of N and P by host plants (Gojon, 2022; Ma et al., 2022), AMF may also cause damage to host plant growth and development by reducing P uptake (Smith et al., 2004). The benefits of AMF symbiosis on nutrient uptake may be influenced by soil nutrient availability and other factors individually or in combination. For example, when soil nutrient availability is relatively high, the cost of P uptake through extracorporeal hyphae will be higher than that through roots, so that plants can meet nutrient uptake without the help of mycorrhiza and do not need additional carbon investment to mycorrhiza (Raven et al., 2018; Wang, R., et al., 2022).
RKN infection mainly occurs in the belowground roots. According to the optimal partitioning theory, plants will transfer more resources belowground to compensate for the adverse effects caused by nutrient uptake inhibition in root due to RKN infection. Our results are consistent with these expectations. Moreover, plants allocate more N and P to the belowground organs under the stress of RKN feeding (Figure 3; Supplementary Figure S1). One possible reason is that root exudates may increase under the action of rhizosphere organisms, which not only meet the maintenance of root function, but also promote mycorrhizal colonization and improve the ability of root resistance to RKN (Wieder et al., 2015; Branco et al., 2022). Our study specifically demonstrated that cherry tomatoes tend to build up higher N and P concentrations in the aboveground organs to preferentially maintain photosynthesis and reproduction under the combined interactions of soil nutrient reduction, mycorrhizal colonization and RKN infection (Figure 5). In summary, plants may better adapt to environmental changes by altering the biomass or nutrient allocations, which are also affected by soil nutrients, mycorrhizal colonization and RKN infection.
Although the increase of N and P concentrations in plants is beneficial to the accumulation and allocation of plant biomass (Yan et al., 2016; Yue et al., 2021). We found that the biomass accumulation and allocation did not seem to be positively regulated by nutrient concentrations when AMF colonization and RKN infection occurred. This suggests that the biomass allocation of plants under the combined effects of soil, AMF and RKN may be the result of comprehensive adaptation, rather than being directly regulated by nutrients. It is now increasingly accepted that mycorrhizal colonization is not necessarily associated with plant growth and P uptake, as in some cases plant growth restriction can be attributed to AMF colonization (Schouteden et al., 2015; Frew et al., 2018). Nutrients and other factors can jointly regulate the process of plant biomass allocation, and the effect of nutrients on biomass allocation may also be regulated by other environmental variables (Yue et al., 2021). This suggests that we need to consider multiple factors in understanding the allocation patterns of plant biomass.
Our results showed that cherry tomato can flexibly adjust biomass allocation and nutrient allocation to different organs in response to changes in soil composition, mycorrhizal symbiosis, and herbivorous nematode foraging. We found that plants have different patterns of biomass and nutrient allocation in response to environmental changes. In particular, the reproductive investment of plants will also change accordingly in response to soil nutrients, AMF and RKN, so as to achieve the purpose of successful reproduction and population regeneration under the combined effect of multiple factors. As a key period of biomass allocation and conversion in plant life history, reproductive investment should be paid more attention in future studies. More importantly, this study provides us with a broader perspective to understand adaptation strategies of plant resource allocation when multiple complex ecological effects coexist in soil. Fully understanding the adaptation strategies of plant biomass and nutrient allocation to complex ecological relationships in soil will help us better predict the contribution of plants to carbon fixation and element cycling in terrestrial ecosystems.
The raw data supporting the conclusions of this article will be made available by the authors, without undue reservation.
ZT and LW: conceptualization. LW: methodology and investigation. LW, XC, and ZT: software and formal analysis. LW: writing-original drafts. ZT, XY, CW, and PG: writing-review and editing. ZT: funding acquisition. All authors contributed to the article and approved the submitted version.
This work was supported by grants from the National Natural Science Foundation of China (31960228) and the Fundamental Research Funds for the Central Universities (2412022ZD055).
The authors thank Junyuan Wang for her assistance in the greenhouse work, and thank Di Zhang for his help with the drawing.
The authors declare that the research was conducted in the absence of any commercial or financial relationships that could be construed as a potential conflict of interest.
All claims expressed in this article are solely those of the authors and do not necessarily represent those of their affiliated organizations, or those of the publisher, the editors and the reviewers. Any product that may be evaluated in this article, or claim that may be made by its manufacturer, is not guaranteed or endorsed by the publisher.
The Supplementary material for this article can be found online at: https://www.frontiersin.org/articles/10.3389/fevo.2023.1106122/full#supplementary-material
Albornoz, F. E., Prober, S. M., Ryan, M. H., and Standish, R. J. (2022). Ecological interactions among microbial functional guilds in the plant-soil system and implications for ecosystem function. Plant Soil 476, 301–313. doi: 10.1007/s11104-022-05479-1
Bebre, I., Marques, I., and Annighöfer, P. (2022). Biomass allocation and leaf morphology of saplings grown under various conditions of light availability and competition types. Plan. Theory 11:305. doi: 10.3390/plants11030305
Bell, C. A., Magkourilou, E., Urwin, P. E., and Field, K. J. (2022). Disruption of carbon for nutrient exchange between potato and arbuscular mycorrhizal fungi enhanced cyst nematode fitness and host pest tolerance. New Phytol. 234, 269–279. doi: 10.1111/nph.17958
Bever, J. D., Richardson, S. C., Lawrence, B. M., Holmes, J., and Watson, M. (2009). Preferential allocation to beneficial symbiont with spatial structure maintains mycorrhizal mutualism. Ecol. Lett. 12, 13–21. doi: 10.1111/j.1461-0248.2008.01254.x
Branco, S., Schauster, A., Liao, H. L., and Ruytinx, J. (2022). Mechanisms of stress tolerance and their effects on the ecology and evolution of mycorrhizal fungi. New Phytol. 235, 2158–2175. doi: 10.1111/nph.18308
Cavagnaro, R. A., Oyarzabal, M., Oesterheld, M., and Grimoldi, A. A. (2014). Screening of biomass production of cultivated forage grasses in response to mycorrhizal symbiosis under nutritional deficit conditions. Grassl. Sci. 60, 178–184. doi: 10.1111/grs.12057
Chen, Q. L., Hu, H. W., Zhu, D., Zhu, Y. G., and He, J. Z. (2022). Calling for comprehensive explorations between soil invertebrates and arbuscular mycorrhizas. Trends Plant Sci. 27, 793–801. doi: 10.1016/j.tplants.2022.03.005
Chen, K., Kleijn, D., Scheper, J., and Fijen, T. P. M. (2022a). Additive and synergistic effects of arbuscular mycorrhizal fungi, insect pollination and nutrient availability in a perennial fruit crop. Agric. Ecosyst. Environ. 325:107742. doi: 10.1016/j.agee.2021.107742
Chen, K., Scheper, J., Fijen, T. P. M., and Kleijn, D. (2022b). Potential tradeoffs between effects of arbuscular mycorrhizal fungi inoculation, soil organic matter content and fertilizer application in raspberry production. PLoS One 17:e0269751. doi: 10.1371/journal.pone.0269751
Cheplick, G. P. (2020). Life-history variation in a native perennial grass (Tridens flavus): reproductive allocation, biomass partitioning, and allometry. Plant Ecol. 221, 103–115. doi: 10.1007/s11258-019-00996-z
Cleveland, C. C., Houlton, B. Z., Smith, W. K., Marklein, A. R., Reed, S. C., Parton, W., et al. (2013). Patterns of new versus recycled primary production in the terrestrial biosphere. Proc. Natl. Acad. Sci. U. S. A. 110, 12733–12737. doi: 10.1073/pnas.1302768110
Cunha, H. F. V., Andersen, K. M., Lugli, L. F., Santana, F. D., Aleixo, I., Moraes, A. M., et al. (2022). Direct evidence for phosphorus limitation on Amazon forest productivity. Nature 608, 558–562. doi: 10.1038/s41586-022-05085-2
Elbasiouny, H., El-Ramady, H., Elbehiry, F., Rajput, V. D., Minkina, T., and Mandzhieva, S. (2022). Plant nutrition under climate change and soil carbon sequestration. Sustainability 14:914. doi: 10.3390/su14020914
Frew, A., Powell, J. R., Glauser, G., Bennett, A. E., and Johnson, S. N. (2018). Mycorrhizal fungi enhance nutrient uptake but disarm defenses in plant roots, promoting plant-parasitic nematode populations. Soil Biol. Biochem. 126, 123–132. doi: 10.1016/j.soilbio.2018.08.019
Fujita, Y., Venterink, H. O., Van Bodegom, P. M., Douma, J. C., Heil, G. W., Hölzel, N., et al. (2014). Low investment in sexual reproduction threatens plants adapted to phosphorus limitation. Nature 505, 82–86. doi: 10.1038/nature12733
Gleeson, S. K., and Good, R. E. (2003). Root allocation and multiple nutrient limitation in the New Jersey pinelands. Ecol. Lett. 6, 220–227. doi: 10.1046/j.1461-0248.2003.00416.x
Gojon, A. (2022). Nitrogen acquisition in arbuscular mycorrhizal symbioses: a step into the real world. J. Plant Physiol. 271:153646. doi: 10.1016/j.jplph.2022.153646
Harkes, P., Van Steenbrugge, J. J. M., Van Den Elsen, S. J. J., Suleiman, A. K. A., De Haan, J. J., Holterman, M. H. M., et al. (2020). Shifts in the active rhizobiome paralleling low Meloidogyne chitwoodi densities in fields under prolonged organic soil management. Front. Plant Sci. 10:1697. doi: 10.3389/fpls.2019.01697
Irving, L. J., and Mori, S. (2021). Effects of light, N and defoliation on biomass allocation in Poa annua. Plan. Theory 10:1783. doi: 10.3390/plants10091783
Jevon, F. V., and Lang, A. K. (2022). Tree biomass allocation differs by mycorrhizal association. Ecology 103:e3688. doi: 10.1002/ecy.3688
Kesba, H. H., and Al-Sayed, A. S. A. (2006). Interactions of three species of plant-parasitic nematodes with arbuscular mycorrhizal fungus, Glomus macrocarpus, and their effect on grape biochemistry. Nematology 7, 945–952. doi: 10.1163/156854105776186406
Kobe, R. K., Iyer, M., and Walters, M. B. (2010). Optimal partitioning theory revisited: nonstructural carbohydrates dominate root mass responses to nitrogen. Ecology 91, 166–179. doi: 10.1890/09-0027.1
Lerat, S., Lapointe, L., Piché, Y., and Vierheilig, H. (2003). Strains colonizing barley roots. Can. J. Bot. 81, 886–889. doi: 10.1139/b03-070
Li, S., Liu, J., Li, J., Deng, Y., Chen, J., Wang, J., et al. (2021). Reproductive strategies involving biomass allocation, reproductive phenology and seed production in two Asteraceae herbs growing in karst soil varying in depth and water availability. Plant Ecol. 222, 737–747. doi: 10.1007/s11258-021-01141-5
Liu, Y., and Li, Z. (2020). Effects of water addition on reproductive allocation of dominant plant species in Inner Mongolia steppe. Front. Plant Sci. 11:555743. doi: 10.3389/fpls.2020.555743
Ma, J., Wang, W., Yang, J., Qin, S., Yang, Y., Sun, C., et al. (2022). Mycorrhizal symbiosis promotes the nutrient content accumulation and affects the root exudates in maize. BMC Plant Biol. 22:64. doi: 10.1186/s12870-021-03370-2
Martignoni, M. M., Garnier, J., Zhang, X., Rosa, D., Kokkoris, V., Tyson, R. C., et al. (2021). Co-inoculation with arbuscular mycorrhizal fungi differing in carbon sink strength induces a synergistic effect in plant growth. J. Theor. Biol. 531:110859. doi: 10.1016/j.jtbi.2021.110859
Meng, B., Li, J., Yao, Y., Nippert, J. B., Williams, D. G., Chai, H., et al. (2022). Soil N enrichment mediates carbon allocation through respiration in a dominant grass during drought. Funct. Ecol. 36, 1204–1215. doi: 10.1111/1365-2435.14033
Pan, S., Wang, Y., Qiu, Y., Chen, D., Zhang, L., Ye, C., et al. (2020). Nitrogen-induced acidification, not N-nutrient, dominates suppressive N effects on arbuscular mycorrhizal fungi. Glob. Chang. Biol. 26, 6568–6580. doi: 10.1111/gcb.15311
Pandey, P., Irulappan, V., Bagavathiannan, M. V., and Senthil-Kumar, M. (2017). Impact of combined abiotic and biotic stresses on plant growth and avenues for crop improvement by exploiting physio-morphological traits. Front. Plant Sci. 8:537. doi: 10.3389/fpls.2017.00537
Poll, J., Marhan, S., Haase, S., Hallmann, J., Kandeler, E., and Ruess, L. (2007). Low amounts of herbivory by root-knot nematodes affect microbial community dynamics and carbon allocation in the rhizosphere. FEMS Microbiol Ecol. 62, 268–279. doi: 10.1111/j.1574-6941.2007.00383.x
Portsmuth, A., and Niinemets, Ü. (2007). Structural and physiological plasticity in response to light and nutrients in five temperate deciduous woody species of contrasting shade tolerance. Funct. Ecol. 21, 61–77. doi: 10.1111/j.1365-2435.2006.01208.x
Qi, S., Wang, J., Wan, L., Dai, Z., Da Silva Matos, D. M., Du, D., et al. (2022). Arbuscular mycorrhizal fungi contribute to phosphorous uptake and allocation strategies of Solidago canadensis in a phosphorous-deficient environment. Front. Plant Sci. 13:831654. doi: 10.3389/fpls.2022.831654
Rahim, M. A., Rabbi, A. Z., Afrin, K. S., Jung, H. J., and Nou, I. S. (2019). Differential expression pattern of lignin biosynthetic genes in dwarf cherry tomato (Solanum lycopersicum var. cerasiforme). Plant Breed. Biot. 7, 229–236. doi: 10.9787/PBB.2019.7.3.229
Ramírez-Flores, M. R., Bello-Bello, E., Rellán-Álvarez, R., Sawers, R. J. H., and Olalde-Portugal, V. (2019). Inoculation with the mycorrhizal fungus Rhizophagus irregularis modulates the relationship between root growth and nutrient content in maize (Zea mays ssp. mays L.). Plant Direct 3:e00192. doi: 10.1002/pld3.192
Raven, J. A., Lambers, H., Smith, S. E., and Westoby, M. (2018). Costs of acquiring phosphorus by vascular land plants: patterns and implications for plant coexistence. New Phytol. 217, 1420–1427. doi: 10.1111/nph.14967
Robin, A. H. K., Irving, L. J., Khaembah, E. N., and Matthew, C. (2018). Modelling carbon fluxes as an aid to understanding perennial ryegrass (Lolium perenne) root dynamics. Agronomy 8:236. doi: 10.3390/agronomy8110236
Robinson, D., Davidson, H., Trinder, C., and Brooker, R. (2010). Root-shoot growth responses during interspecific competition quantified using allometric modelling. Ann. Bot. London 106, 921–926. doi: 10.1093/aob/mcq186
Salmeron-Santiago, I. A., Martínez-T, R. M., Valdez-Alarcón, J. J., Pedraza-Santos, M. E., Santoyo, G., Pozo, M. J., et al. (2022). An updated review on the modulation of carbon partitioning and allocation in arbuscular mycorrhizal plants. Microorganisms 10:75. doi: 10.3390/microorganisms10010075
Schmidt, B., Gaşpar, S., Camen, D., Ciobanu, I., and Sumălan, R. (2011). Arbuscular mycorrhizal fungi in terms of symbiosis-parasitism continuum. Commun. Agr. Appl. Biol. Sci. 76, 653–659.
Schouteden, N., De Waele, D., Panis, B., and Vos, C. M. (2015). Arbuscular mycorrhizal fungi for the biocontrol of plant-parasitic nematodes: a review of the mechanisms involved. Front. Microbiol. 6:1280. doi: 10.3389/fmicb.2015.01280
Sharma, I. P., and Sharma, A. K. (2019). Mycorrhizal colonization and phosphorus uptake in presence of PGPRs along with nematode infection. Symbiosis 77, 185–187. doi: 10.1007/s13199-018-0576-x
Sheldrake, M., Rosenstock, N. P., Mangan, S., Revillini, D., Sayer, E. J., Olsson, P. A., et al. (2018). Responses of arbuscular mycorrhizal fungi to long-term inorganic and organic nutrient addition in a lowland tropical forest. ISME J. 12, 2433–2445. doi: 10.1038/s41396-018-0189-7
Shen, K. P., Cornelissen, J. H. C., Wang, Y. J., Wu, C. B., He, Y. J., Ou, J., et al. (2020). AM fungi alleviate phosphorus limitation and enhance nutrient competitiveness of invasive plants via mycorrhizal networks in karst areas. Front. Ecol. Evol. 8:125. doi: 10.3389/fevo.2020.00125
Smith, S. E., Smith, F. A., and Jakobsen, I. (2004). Functional diversity in arbuscular mycorrhizal (AM) symbioses: the contribution of the mycorrhizal P uptake pathway is not correlated with mycorrhizal responses in growth or total P uptake. New Phytol. 162, 511–524. doi: 10.1111/j.1469-8137.2004.01039.x
Sun, Y. F., Li, Y. W., Lu, X. M., Wang, Y., and Bai, Y. F. (2022). Contrasting effects of arbuscular mycorrhizal fungi on nitrogen uptake in Leymus chinensis and Cleistogenes squarrosa grasses, dominants of the inner Mongolian steppe. Plant Soil 475, 395–410. doi: 10.1007/s11104-022-05375-8
Tikoria, R., Kaur, A., and Ohri, P. (2022). Potential of vermicompost extract in enhancing the biomass and bioactive components along with mitigation of Meloidogyne incognita-induced stress in tomato. Environ. Sci. Pollut. Res. 29, 56023–56036. doi: 10.1007/s11356-022-19757-z
Tran, C. T. K., Watts-Williams, S. J., Smernik, R. J., and Cavagnaro, T. R. (2022). Arbuscular mycorrhizas increased tomato biomass and nutrition but did not affect local soil P availability or 16S bacterial community in the field. Sci. Total Environ. 819:152620. doi: 10.1016/j.scitotenv.2021.152620
Wang, L., Chen, X., Du, Y. Q., Zhang, D., and Tang, Z. H. (2022). Nutrients regulate the effects of arbuscular mycorrhizal fungi on the growth and reproduction of cherry tomato. Front. Microbiol. 13:843010. doi: 10.3389/fmicb.2022.843010
Wang, B., Gong, J. R., Zhang, Z. H., Yang, B., Liu, M., Zhu, C. C., et al. (2019). Nitrogen addition alters photosynthetic carbon fixation, allocation of photo assimilates, and carbon partitioning of Leymus chinensis in a temperate grassland of Inner Mongolia. Agric. For. Meteorol. 279:107743. doi: 10.1016/j.agrformet.2019.107743
Wang, R., Lu, J., Jiang, Y., and Dijkstra, F. A. (2022). Carbon efficiency for nutrient acquisition (CENA) by plants: role of nutrient availability and microbial symbionts. Plant Soil 476, 289–300. doi: 10.1007/s11104-022-05347-y
Wieder, W. R., Cleveland, C. C., Smith, W. K., and Todd-Brown, K. (2015). Future productivity and carbon storage limited by terrestrial nutrient availability. Nat. Geosci. 8, 441–444. doi: 10.1038/ngeo2413
Wright, D. P., Read, D. J., and Scholes, J. D. (1998). Mycorrhizal sink strength influences whole plant carbon balance of Trifolium repens L. Plant Cell Environ. 21, 881–891. doi: 10.1046/j.1365-3040.1998.00351.x
Xie, J., Tang, L., and Wang, Z. (2012). Distinguishing the biomass allocation variance resulting from ontogenetic drift or acclimation to soil texture. PLoS One 7:e41502. doi: 10.1371/journal.pone.0041502
Yan, B., Ji, Z., Fan, B., Wang, X., He, G., Shi, L., et al. (2016). Plants adapted to nutrient limitation allocate less biomass into stems in an arid-hot grassland. New Phytol. 211, 1232–1240. doi: 10.1111/nph.13970
Yue, K., Fornara, D. A., Li, W., Ni, X., Peng, Y., Liao, S., et al. (2021). Nitrogen addition affects plant biomass allocation but not allometric relationships among different organs across the globe. J. Plant Ecol. 14, 361–371. doi: 10.1093/jpe/rtaa100
Zhang, J., Huang, W., and Ding, J. (2021). Phenotypic plasticity in resource allocation to sexual trait of alligatorweed in wetland and terrestrial habitats. Sci. Total Environ. 757:143819. doi: 10.1016/j.scitotenv.2020.143819
Zhou, L., Yan, W., Sun, X., Shao, J., Zhang, P., Zhou, G., et al. (2021). Regulation of climate, soil and hydrological factors on macrophyte biomass allocation for coastal and inland wetlands in China. Sci. Total Environ. 774:145317. doi: 10.1016/j.scitotenv.2021.145317
Keywords: biomass allocation, nutrient uptake, biotic and abiotic factors, adaptation strategies, physiological response
Citation: Wang L, Chen X, Yan X, Wang C, Guan P and Tang Z (2023) A response of biomass and nutrient allocation to the combined effects of soil nutrient, arbuscular mycorrhizal, and root-knot nematode in cherry tomato. Front. Ecol. Evol. 11:1106122. doi: 10.3389/fevo.2023.1106122
Received: 23 November 2022; Accepted: 01 February 2023;
Published: 21 February 2023.
Edited by:
Nicolas Schtickzelle, Université Catholique de Louvain, BelgiumReviewed by:
Xie Jiangbo, Zhejiang Agriculture &Forestry University, ChinaCopyright © 2023 Wang, Chen, Yan, Wang, Guan and Tang. This is an open-access article distributed under the terms of the Creative Commons Attribution License (CC BY). The use, distribution or reproduction in other forums is permitted, provided the original author(s) and the copyright owner(s) are credited and that the original publication in this journal is cited, in accordance with accepted academic practice. No use, distribution or reproduction is permitted which does not comply with these terms.
*Correspondence: Zhanhui Tang, ✉ dGFuZ3poNzg5QG5lbnUuZWR1LmNu
Disclaimer: All claims expressed in this article are solely those of the authors and do not necessarily represent those of their affiliated organizations, or those of the publisher, the editors and the reviewers. Any product that may be evaluated in this article or claim that may be made by its manufacturer is not guaranteed or endorsed by the publisher.
Research integrity at Frontiers
Learn more about the work of our research integrity team to safeguard the quality of each article we publish.