- 1Fisheries Department, Faculty of Natural Resources, University of Guilan, Sowmeh Sara, Iran
- 2Universidad de Buenos Aires, CONICET, Instituto de Biodiversidad y Biología Experimental y Aplicada (IBBEA), Facultad de Ciencias Exactas y Naturales, Departamento de Biodiversidad y Biología Experimental, Laboratorio de Biología de la Reproducción y el Crecimiento de Crustáceos Decápodos, Ciudad Universitaria, Buenos Aires, Argentina
Anthropogenic noise in marine and freshwater environments has increased dramatically, with a range of negative impacts and detrimental consequences on many aquatic animals across taxa. Benthic organisms, including many invertebrates, can sense underwater sounds, yet the responses they trigger in these organisms have received little attention. We conducted two laboratory-based experiments to investigate the effect of underwater sound playback on the movement behavior and feeding performance of the red cherry shrimp Neocaridina davidi as a model of freshwater decapod. Movement speed decreased significantly upon opening the divider in both the sound and control treatments. However, there were no significant sound-dependent changes overall between the control and sound treatments. The spatial distribution of shrimp in response to the sound treatment showed significant changes; shrimp spent more time at the farthest one-third position from the sound source. Feeding latency (latency to find food) also increased in the sound treatment compared to the control. Moreover, in terms of the number of successes and failures in finding the food source in the control treatment, significantly more shrimp succeeded in finding the food source. The number of revisits to the food source decreased in the sound treatment compared to control and more shrimp were significantly distracted in the sound treatment. Our study highlights the potential for human-made sound to impact on crustacean activity. Thus, they are prone to the impacts of anthropogenic noise, causing negative impacts on their movement-swimming activities, and feeding behavior. Behavioral changes observed, namely altered feeding and locomotory behavior may have wider-reaching negative effects, including detrimental impacts on animal fitness.
1 Introduction
Over recent decades, environmental pollution caused by human activities has altered terrestrial and aquatic habitats globally (Kight and Swaddle, 2011). Aquatic animals use signals and exploit cues to explore their habitat and perform various biological activities (Kight and Swaddle, 2011). In aquatic habitats, anthropogenic noise (Kunc et al., 2015; Peng et al., 2015) have quite diversely changed acoustic characteristics of the environment globally, and subsequently encountered many species under threat of extinction. The ability to exploit such habitat information is crucial and a prerequisite for survival. Interference with these sensory systems due to introducing novel stimuli may be detrimental to their feeding efficiency success, communication with conspecifics, locating prey, avoiding predators, and courtship performances (Dominoni et al., 2020). Sound, as an informative sensory stimulus, is a key signal in the aquatic environment and attenuates less over the same distance in water than in air (Slabbekoorn et al., 2010). Sound can therefore be propagated over long distances in aquatic habitats (Hawkins and Myrberg, 1983). Sound pollution is omnipresent in aquatic habitats and may affect large areas. It therefore may negatively affect aquatic species not only at the individual level, but also at an environment level changing species interactions (Slabbekoorn et al., 2010; Kunc et al., 2016; Shannon et al., 2016; De Jong et al., 2018; Slabbekoorn et al., 2019).
Anthropogenic noise has a wide range of impacts and cascading effects at variety of trophic levels, that can reverberate at community levels and increase soundscape complexity in aquatic ecosystems (Slabbekoorn et al., 2010; Shafiei Sabet et al., 2016c; Kok et al., 2023; Rojas et al., 2023). Aquatic animals need to optimize time allocation with behaviors and enhance activity performance in behaviors that are crucial for their success in survival and reproduction. Feeding activity in animals is a complex process which involves several sequences, and behavioral choices such as when, where, what and how to feed (Galef and Giraldeau, 2001). Noise could play a prominent role and act negatively as a stressor in a wide range of animals (i.e. Wright et al., 2007b; Williams et al., 2015). Acoustic events and elevation in ambient noise levels may influence a range of feeding activates among species and consequently compromise feeding performance in aquatic animals (Purser and Radford, 2011; Wale et al., 2013; Voellmy et al., 2014). Noise could act as a distracting stimulus, diverting an individual’s limited attention from their primary tasks to the noise stimuli now present in the environment (Chan and Blumstein, 2011). This could impair foraging success if suitable feeding items are detected less frequently or at lower finding speed, are assessed with lower attention, or if food items are captured with more discrimination and handling errors (Purser and Radford, 2011). Moreover, acoustic stimulus can play an important role as habitat-specific cues in orientation through heterogeneous habitats (Huijbers et al., 2012). Noise could cause spatial distribution of aquatic animals. Fishes that live in the water column and are motile have some choice to adopt and select where they live and they can show spatial preferences in a way when they are in noisy areas (Holles et al., 2013; Shafiei Sabet et al., 2016a). They may move into quieter areas to avoid potential impacts of elevated noise levels on their feeding activities.
There are well documented research outputs and assessments on how anthropogenic noise affects marine mammals (Wright et al., 2007a; Nowacek et al., 2007; Southall et al., 2008) and marine fish species (Bruintjes et al., 2015; Simpson et al., 2016; Herbert-Read et al., 2017). However, there has been limited attention paid to the potential impacts of anthropogenic noise on aquatic invertebrates (Wale et al., 2013; Roberts et al., 2015; Williams et al., 2015; Roberts et al., 2016). Although invertebrates are quite diverse, important for all trophic levels, and have a relatively large abundance in marine and freshwater environments (Morley et al., 2014; Solan et al., 2016), their capability to detect and potentially exploit sound is relatively unknown. Marine decapods play an important role in top-down control of prey populations and can significantly impact community structure in aquatic habitats (Bell and Coull, 1978; Reise, 1979). Yet, the potential effects of anthropogenic noise on freshwater decapods and their role in regulating top-down and button-up cascading impacts in aquatic food webs and predator–prey interaction consequences are unknown (Azarm-Karnagh et al., 2023).
The red cherry shrimp (Neocaridina davidi) is a tiny and gregarious benthic freshwater invertebrate species of shrimp with a wide distribution, occurring from Southeast Asia to Europe (Liang, 2004; Wowor et al., 2004; Klotz et al., 2013; Onuki and Fuke, 2022). Although this species is an ornamental crustacean and provides an important link between trophic levels and food webs (Kelly et al., 2012; Weber and Traunspurger, 2016), its behavioral responses to stress stimuli are still poorly understood. In contrast to marine habitats, given the broad-ranging diet of the red cherry shrimp, it is likely that this species also influences food webs in freshwater habitats. However, there is currently a general lack of knowledge concerning how anthropogenic noise may affect their biological and ecological performance.
In the current study, two experiments were conducted to analyse the effect of sound playbacks on the locomotor behavior and feeding activities of the red cherry shrimp under laboratory conditions. In the first experiment we evaluated the initial releasing movement speed, spatial distribution, food-finding latency (the time to find the food source), the number of successes and failures in finding the food source, and the number of revisits to the food source in response to ambient noise conditions (control treatment) and an elevated generated sound (anthropogenic) level. In the second experiment we investigated sound-related feeding distraction in response to ambient noise conditions as a control treatment and an elevated generated sound level.
2 Material and methods
2.1 Study animals and husbandry
Subjects were 140 (70 females; 70 males) red cherry shrimp (Neocaridina davidi). All shrimp were purchased from a local aquatic pet dealer in Rasht city, Iran. Due to the COVID-19 pandemic and social distance limitations at University of Guilan, all experiments were run at the home of SAK. For acclimation to the new tank conditions, the animals were transferred to a glass holding tank (see Figure S1 in the Supplementary Materials) (48 × 28 × 32 cm and 4 mm wall thickness) for one week equipped with a 150 watts heater, sponge biofilter, Java moss, natural plant, a 12 watts LED light, and sandy bed and plant soil. Water temperature was kept at 26 ± 1°C, aeration 5 L/min, and the light period was 14 D: 10 L. Shrimp were fed every 12 hours with Spirulina algae tablets, with any excess cleared from the holding tank during tank maintenance no more than 4 h after feeding. Although there was a constant water change within the holding tank, 25% of water was removed by siphon with excess food and waste; this water was replaced by normal tank flow-through. Water changes and the flow-through system ensured the maintenance of constant oxygen levels and the removal of any metabolic waste materials. The physical and chemical conditions, such as ambient acoustics, water temperature, water changes, for both tanks (holding and experimental), were similar. The sex ratio of shrimp was 1:1, and all mature shrimp had a body length ranging from 2 to 2.5 cm.
2.2 General experimental design
Both experiments were conducted in a tank (40 × 30 × 30 cm) with 4 mm thick walls, two divider plates, and a dewatering surface of 20 cm. Water temperature, pH, total dissolved solids (TDS), light intensity, and NO3−, NO2−, NH4+ were measured (see Table S1 in the Supplementary Materials). Light intensity was recorded daily at the beginning and end of each treatment. Light intensity was measured 20 cm above the water level and in the bottom-middle of the tank. The other parameters were measured daily before each treatment. In each experiment, 35 shrimp were used for each treatment with an alternation of both males and females. The playback speaker was placed behind a vertical partition 6 cm from one short side of the tank. The experimental tank was placed on a 5 cm thick foam pipe lagging to minimize sound transfer and its sides were covered with opaque black paint to minimize distraction from visual reflections.
2.3 Sound characteristics
The sounds used for both experiments in this study were created using specialized software (Audacity-win-vr 2.3.1), based on Shafiei Sabet et al. (2015). Two synthetic acoustic stimuli including elevated sound treatment and silent playback control hereafter ambient noise treatment (20 min duration each treatment) were used in these experiments, presenting frequencies that fall within the likely hearing range of the red cherry shrimp. The ambient noise frequency (control) was 400 to 2000 Hz with an intensity of 96.54 ± 1 dB in WAV format. For the elevated sound treatment, a sound with a continuous pattern (Broad band white noise) and frequency of 400 to 2000 Hz, intensity of 110.40 ± 1, and in WAV format was used (For acoustic stimuli validation see Figure 1 and Supplementary Material). A 30-watt hand-built underwater speaker with a frequency of 0 to 3000 Hz, a model laptop (Lenovo-V110), an amplifier, and a 12 V sealed battery were used to supply the required power. The frequency range of auditory sensitivity for invertebrates varies (Morley et al., 2014; Solé et al., 2023) and there are no data for the red cherry shrimp. However, we expected their sensitivity to overlap the lower frequency range of fish hearing and our current stimuli (see Figure 1). We randomized the order of two trials per individual for the sound treatment and one control to avoid the effect of treatment being confounded by an order effect.
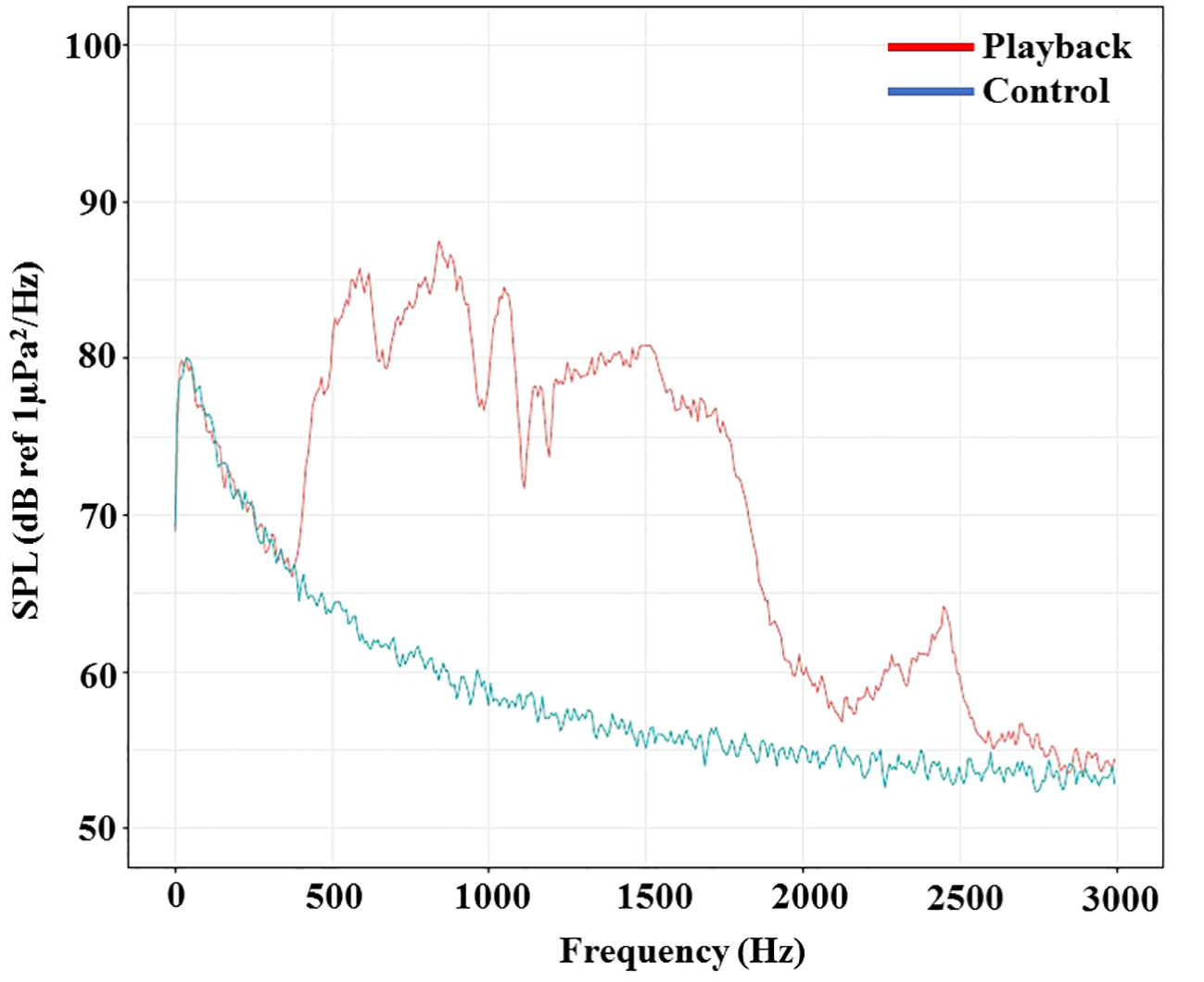
Figure 1 Mean power spectrum (Power Spectral Density; PSD) of 5 s recordings of all sound (red line) and experimental tank ambient (blue line) stimuli. Sound Pressure Level expressed in dB re 1 µPa2/Hz (Welch’s method, Hann window, FFT length 1024). (For interpretation of the references to colour in this figure legend, the reader is referred to the web version of this article). The recordings were made in water depth 3 cm and 3 cm far from the right aquarium wall (longitude plane view).
2.4 Sound measurement and analysis
The sound emitted in the experimental tank environment was measured using a sound measuring device (TASCAM-DR-100MK2) equipped with a calibrated hydrophone by a specialized standard method (Shafiei Sabet et al., 2015). The sound data were imported into R (RStudio Team, 2020; v.3.6.3) for analysis under specialized acoustic programming and the sound intensity (dB ref 1 µPa), frequency (Hz), sensitivity and sample rate were measured (see Figure S2 in the Supplementary Materials). Graph of sound pressure level or SPL per unit (dB ref 1µPa2/Hz) were drawn by the R software (The R Foundation for Statistical Computing, Vienna, Austria, http://www.r-project.org) using packages: devtools and ggplots2 and also a custom-made R-package. (Figure 1).
2.5 Analysis of behavior
Following the one-week acclimation period, the behaviors of the red cherry shrimp (video recordings) were analyzed using Logger Pro® software (Vernier Software & Technology, Beaverton, OR, USA, version 3.14.1). To analyze behavioral observations in all trials, the temporal resolution of the recorded videos were reduced to 1 frame per second (due to the slow movement of the shrimp and the ease of recording the movement points and the movement speed and spatial distribution. All processing behavioral data were done without the audio track to ensure the treatment sequence was blind for the observer (SAK).
2.6 First experiment: movement speed, spatial distribution, feeding latency, food finding success and number of times the shrimp revisited the food source
In the first experiment, before starting the main experiments, shrimp (n = 70; 35 individuals per treatment) were denied access to food for 48 hours in the holding tank. Then, in order to acclimate to the experiment tank (see Figure S3 in the Supplementary Materials), 1 hour before the test, they were individually transferred to the starting area behind a divider plate. All transferring procedures were gentle and remained the same for all trials. At this time, the food source was added to the other side of the divider plate. In the ambient noise treatment, the ambient noise was played (20 minutes duration) at the same time as the divider plate was removed (2 cm upwards from bottom of the aquarium, with minimal physical movement of the divider plate so that less stress is applied to the shrimp and it can pass through it). In the sound treatment (20 minutes duration; the same duration as the ambient noise treatment), the sound file was played at the same time as the divider plate was removed.
For both treatments, to use the same protocol in behavioral analysis, each shrimp was given a maximum of 10 minutes to find the food source and shrimp that did not find the food source were excluded from the trials and further analysis because of a potential difference in food-finding motivation. Each shrimp was used in a trial once. Between trials we syphoned the aquarium to clean it. For all trials, the divider plate was always removed at the same time as the acoustic treatment was started (20 minutes of sound/control treatment) (see Figures 2A, B).
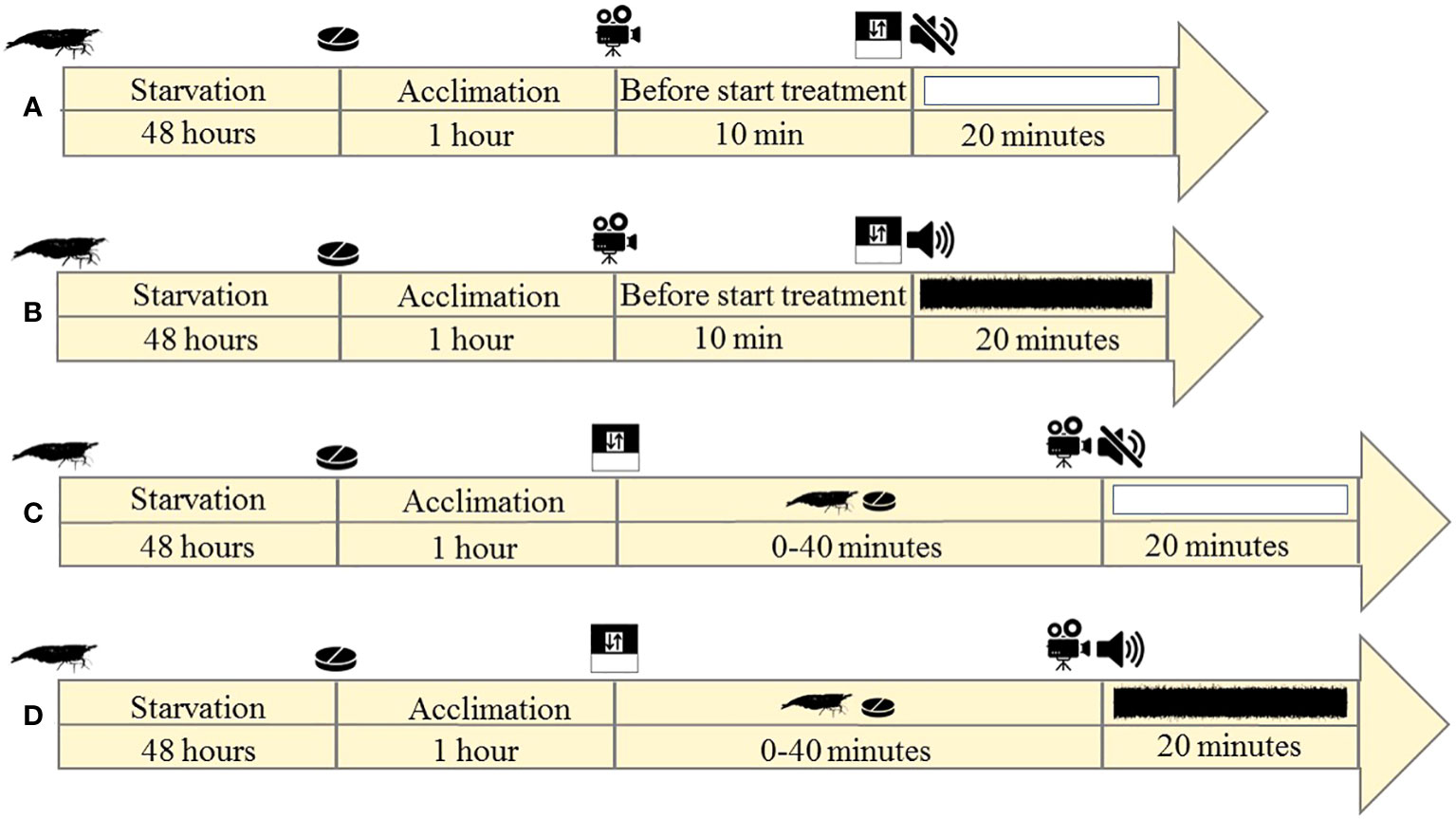
Figure 2 Timeline view of first (A, B) and second (C, D) experiments in (A, C) control and (B, D) sound treatments. the shrimp,
food source,
opening divider,
start filming,
ambient noise exposure,
elevated sound exposure.
The behavioral indicators measured are described as follows: Movement speed: Mean distance moved by the center point of the subject per unit time was calculated from the last 10 mins before the divider plate was removed to 10 mins immediately after the divider plate was removed with onset of either control or sound playback. Spatial distribution: We calculated spatial preference in terms of time spent (seconds) by the shrimp in different areas of the aquarium. The mean attendance of individuals in the specified area (left one-third and right one-third of the tank horizontally) was calculated from the last 10 mins before the divider plate was removed to 10 mins immediately after the divider plate was removed with onset of either control or sound playback (see Figures 3A, B). Food-finding success: We calculated number of individuals that successfully found the food source during 10 minutes in each treatment. Feeding latency (latency to find food): We calculated mean time to find the food source during 10 minutes in each treatments. Number of times the shrimp revisited the food source: We calculated the number of times the shrimp revisited the food source within each 20 minute observation period (from the last 10 mins before the divider plate was removed to 10 mins immediately after the divider plate was removed) (i.e. Wale et al., 2013) (see Table S2 in the Supplementary Materials).
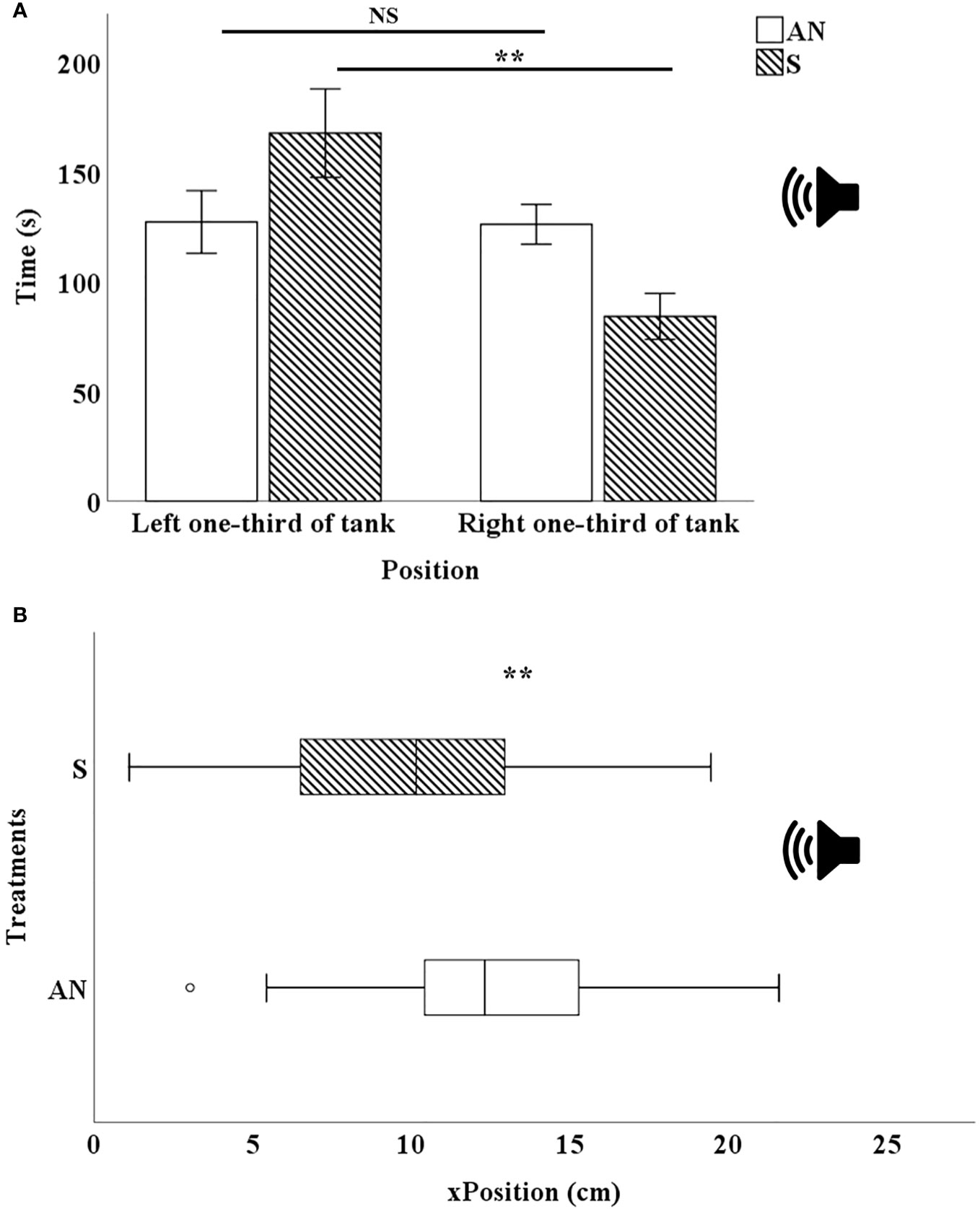
Figure 3 (A) Mean ± SE time–place budget distribution of Neocaridina davidi. In sound condition the distribution of shrimp significantly increased in the left one-third of the tank (the point farthest from the speaker). (B) Mean ± SE spatial distribution in horizontal (x) position in AN: Ambient Noise and S: Sound conditions, position of underwater speaker in tank. NS: Not Significant, **P<0.01.
2.7 Second experiment: feeding distraction
In the second experiment, before the start of experiments, shrimp (n = 70; 35 individuals per treatment) were denied access to food for 48 hours in the holding tank (see Figure S4 in the Supplementary Materials). For both experiments, the animal size and sex ratio, feeding regime and feed restriction period were the same to avoid any confounding factors affecting behaviors unintentionally. In the control treatment, after 1 hour acclimation behind the divider plate in the experiment tank, the divider plate was removed and shrimp were allowed to search for food without playing any sound treatments. As soon as the shrimp found the food and started feeding, the control treatment (ambient noise) was played for 20 minutes and each reaction was recorded in real time by the camcorder (Figure 2C). In the same manner for the sound playback treatment, after 1 hour of acclimation behind the divider plate in the experimental aquarium, the divider plate was removed and shrimp were allowed to search for food, yet without any sound treatment playbacks (Figure 2D). As soon as the shrimp found the food and started feeding, the sound treatment was played for 20 minutes and each reaction was recorded in real time by the camcorder. A feeding distraction event is defined as a shrimp stopping eating, freezing and moving away from the food source (was calculated for 10 minutes) (Wale et al., 2013). The number of shrimp whose feeding performance was disturbed versus undisturbed was recorded in each of the control and sound treatments.
2.8 Statistical analysis
Statistical analysis of behavioral data was performed using SPSS-25 and Excel-2019 software. Kolmogorov-Smirnov test and Levene’s tests were used to test the normality and variability of the data. For movement speed, paired sample t-test was used. A single sample t-test with a reference mean of 12.5 was performed for the position of the left/right one-third of the tank. Because the food partition is 25 cm (as shown in Figure S3), the theoretical mean representing a random choice (null hypothesis) between the left and right sides of the partition is 12.5 cm. The 0.05 level is often the alpha level, used as a benchmark for the significance of statistical tests. For the x-position spatial distribution, the number of revisiting and feeding latency (latency to find food), independent two sample t-test was used at the level of 0.05. Chi-square test of independence was also used for food-finding and feeding distraction.
3 Results
3.1 First experiment
3.1.1 Movement speed
As soon as the divider was opened, the initial movement speed in the sound and control treatments, showed a significant decrease (P<0.001, df=34) (Figure 4A). However, there were no significant changes between averaged total movement speed for the control and sound treatments (Figure 4B) (P>0.05, df=34).
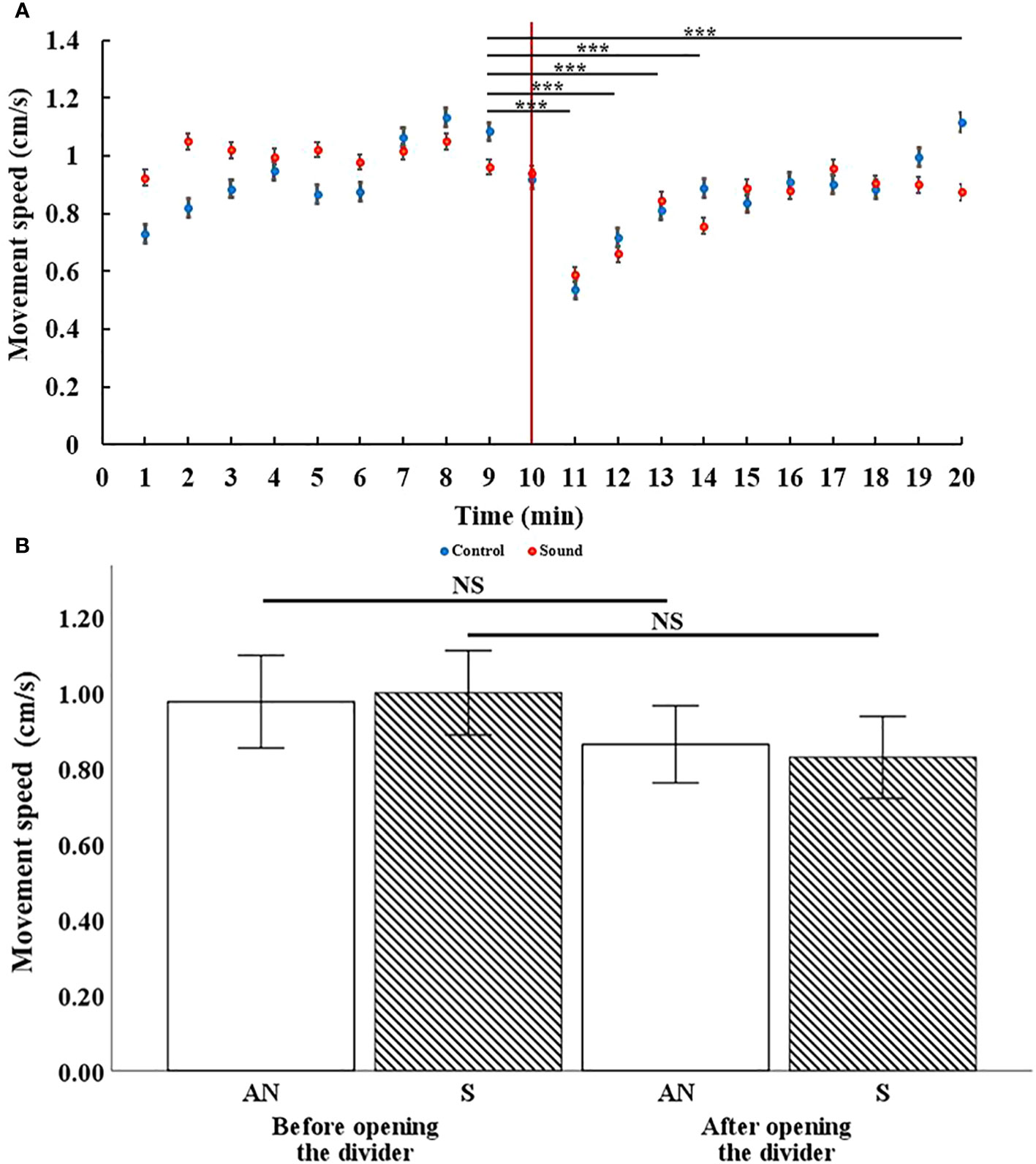
Figure 4 (A) Movement speed in control (AN) and sound (S) treatments changed (decreased significantly) as soon as removing the divider plate and simultaneously onset of treatments. As soon as opening the divider significantly movement speed reduced. ***P<0.001. The vertical red line at the 10-minute time point represent the instant moment of removing the divider plate and simultaneously onset of treatments. (B) Mean ± SE movement speed (cm/s) for each sound treatment; ambient noise condition as control (AN) and sound treatment (S) for 35 red cherry shrimp (averaged mean total 10 minutes from before of removing the divider plate and simultaneously onset of treatments to the first 10 minutes of removing the divider plate and simultaneously onset of treatments. Red cherry shrimp movement speed did not change with sound conditions among treatments. NS: Not Significant.
3.1.2 Spatial distribution
Shrimp in the control treatment were distributed equally in the available space before opening the divider. After opening the divider and allowing the shrimp to enter the new environment they were again equally distributed throughout the whole space (Figures 5A, B). In the sound treatment, before opening the divider, the shrimp were equally distributed in the available space, but when the divider was opened and the shrimp were exposed to the sound, they dispersed to the left of the tank (farthest point from the sound source) (Figures 5C, D).
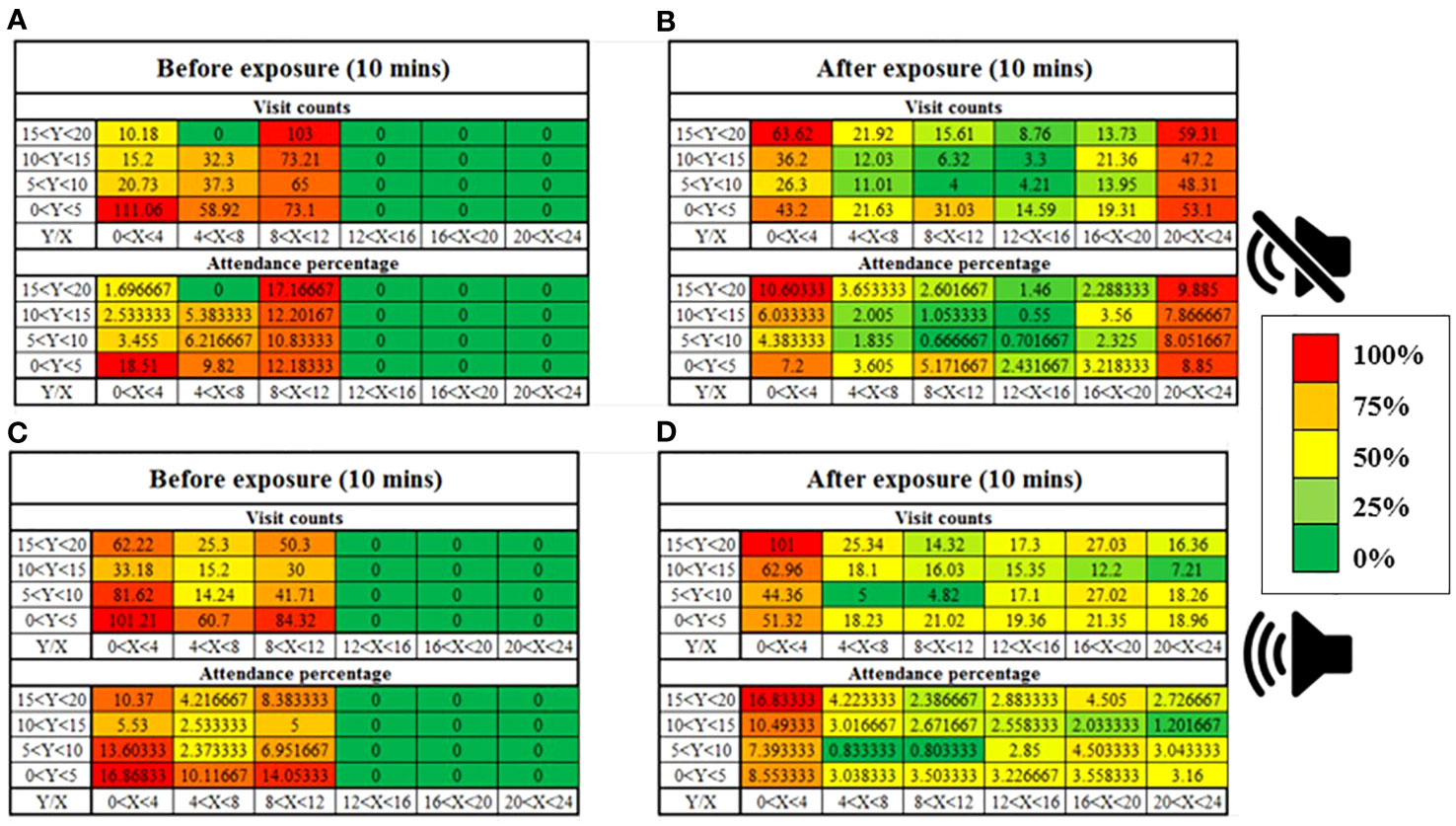
Figure 5 Cluster heatmap showing the spatial occupancy in terms of time spent in movement (expressed in seconds) by the shrimp among the aquarium cells. On the right wall, the location of the underwater sound speaker is presented. (A, B) Control condition. (C, D) Sound condition.
Figures 3A, B, shows the spatial distribution time of 70 shrimp in the right third of the tank (near the sound source) and the left third of the tank (away from the sound source). In the control treatment, the distribution of shrimp on both sides of the tank was the same and no significant changes were observed (df=69, P=0.391). In the sound treatment, the horizontal distribution of shrimp on the left side of the tank (the farthest distance from the sound source) significantly increased (df=69, P=0.003).
3.1.3 Feeding activities
In the sound treatment, individuals found the food source significantly later than the control treatment (Figure 6A, df=57, P=0.005). One individual in the control treatment and 9 in the sound treatment were not successful in finding the food source during the entire 10 minutes. The number of revisits to the food source was significantly reduced in the sound treatment (Figure 6B, df=68, P <0.0001), and significantly more individuals succeeded in finding the food source in the control treatment compared to the sound treatment (Figure 6C, df=1, P=0.006).
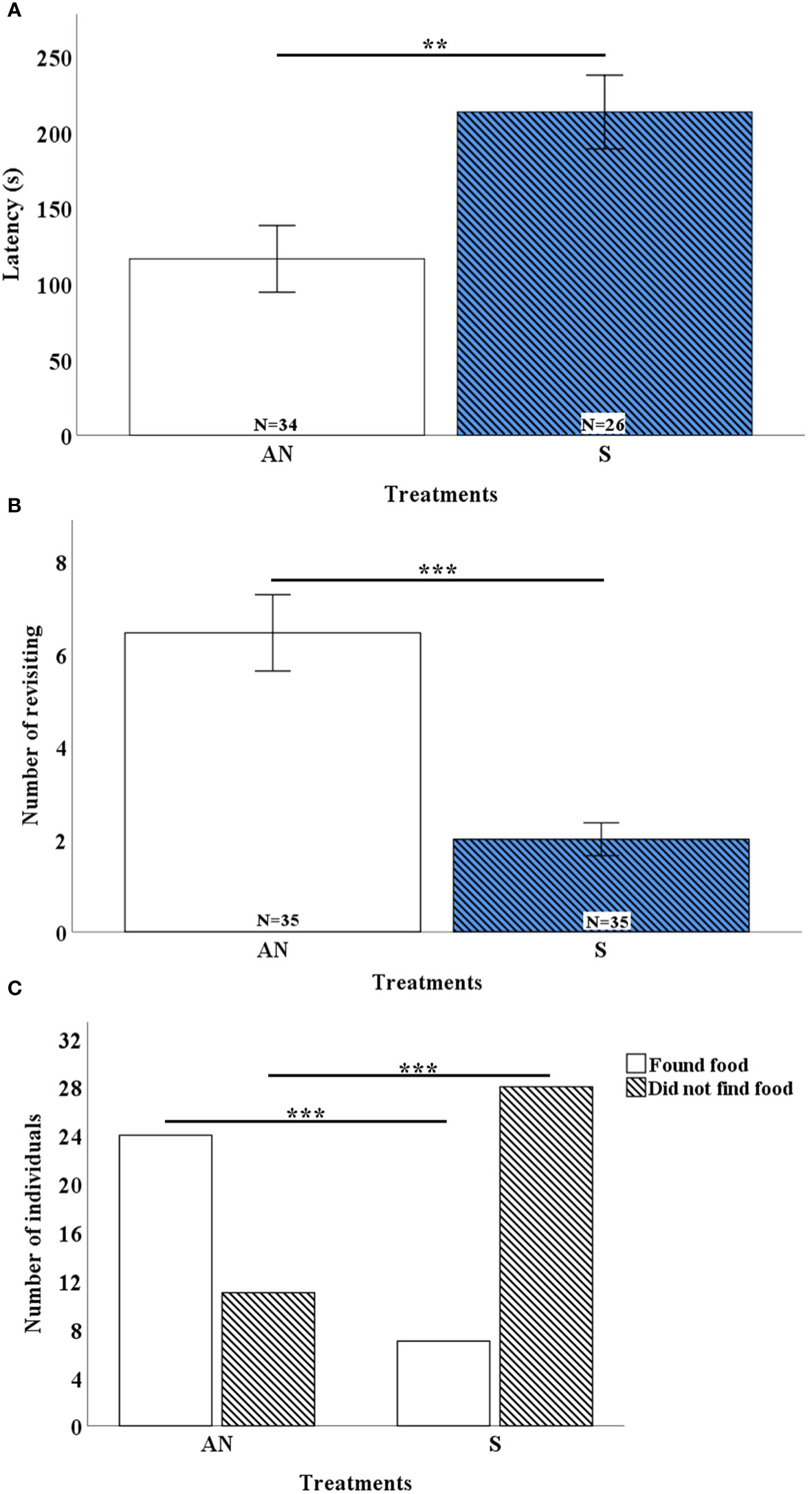
Figure 6 (A) Mean ± SE of the time of finding food source in AN: Ambient Noise and S: Sound conditions (N=35). In sound condition the time of finding food significantly increased. (B) Mean ± SE of the number of revisiting to food source in AN: Ambient Noise and S: Sound conditions (N=35). In sound condition the number of revisiting to food source significantly decreased. (C) The number of individuals that found/not found food source for 10 minutes in AN: Ambient Noise and S: Sound conditions (N=35). In sound condition significantly more shrimp did not find food source. NS: Not Significant, **P<0.01, ***P<0.001.
3.2 Second experiment
3.2.1 Feeding distraction
In the sound treatment significantly more individuals (df=1, P=0.0001) were distracted during feeding than in the control treatment (Figure 7).
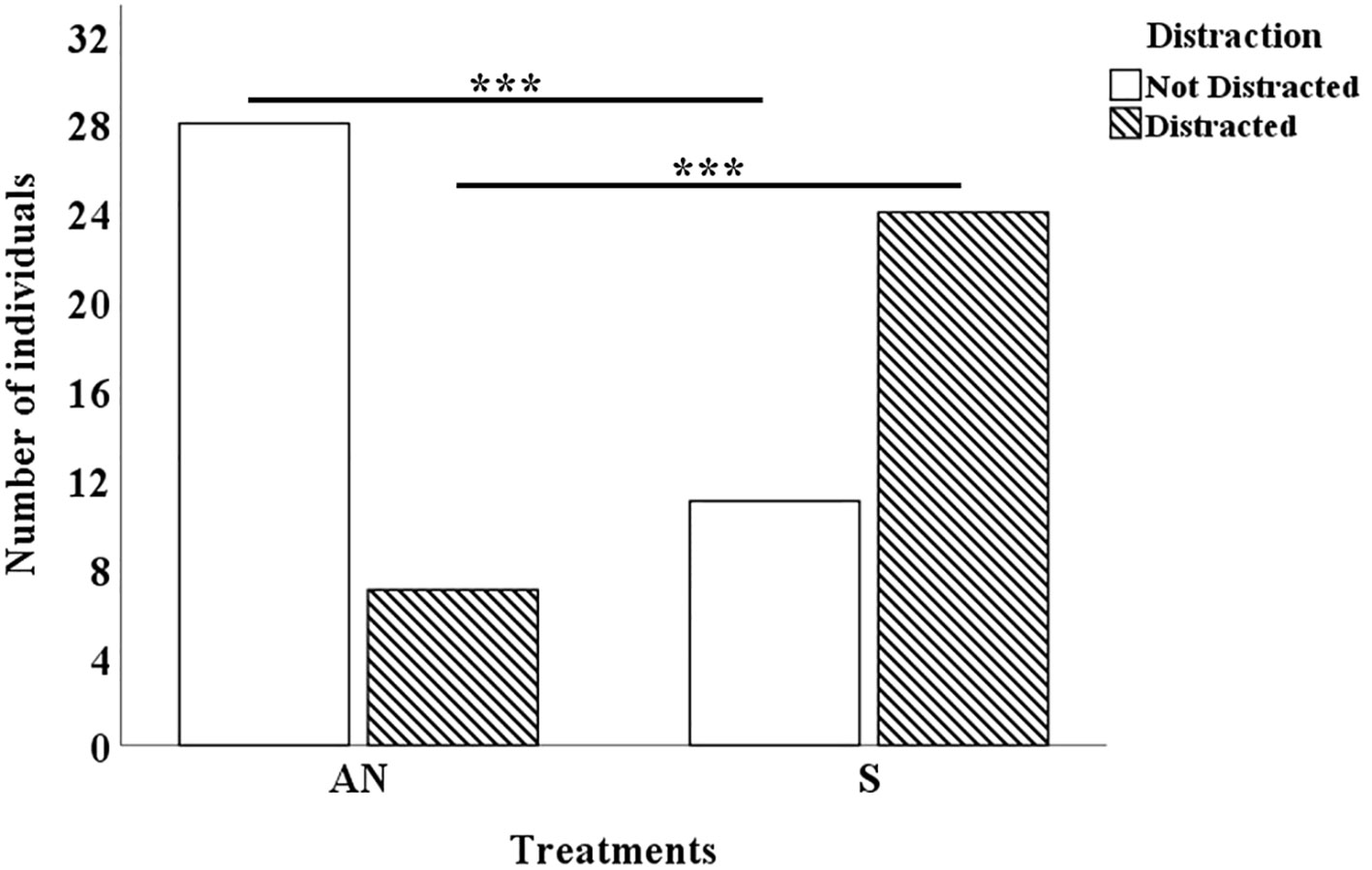
Figure 7 The number of Neocaridina davidi that distracted/not distracted from food source for 10 minutes in AN: Ambient Noise and S: Sound conditions (N=35). In sound condition significantly more shrimp distracted in feeding. ***P<0.001.
4 Discussion
The present study highlights that invertebrates are likely to perceive sound and be susceptible to the impact of sound as a source of stress. This makes them important candidates for further investigation into the effects of anthropogenic noise pollution. Experimental sound playback resulted in a variety of behavioral changes in the red cherry shrimp, some of which have been reported in other invertebrates and some appear to be species-specific. Our results are the first to demonstrate that freshwater decapods can be affected by acoustic stimuli. Sound treatment compromised movement and feeding activity and this is consistent with previous research in crustaceans (Filiciotto et al., 2014; Sal Moyano et al., 2021). In controlled tank-based experiments, it has been shown that sound affects the behavior of feeding crabs and disrupts their feeding performance (Wale et al., 2013). Moreover, in other taxa (e.g. fishes) recent studies have shown that acoustic stimuli disrupt feeding performance (Purser and Radford, 2011; Voellmy et al., 2014; Shafiei Sabet et al., 2015; Magnhagen et al., 2017; Mickle and Higgs, 2018).
4.1 Impact of sound on the movement and locomotor behavior of shrimp
Upon opening the divider plate, the movement speed decreased in the control and sound treatments; however, there was no difference between total movement speed for the control and sound treatments. This change may be due to the stress of facing the new environment as shrimp in both treatments displayed the same pattern of reducing their movement speed (Shafiei Sabet et al., 2019). The effects of anthropogenic noise on movement and swimming behavior of crustaceans and fish is well reported (Sarà et al., 2007; De Vincenzi et al., 2015; Filiciotto et al., 2016; Shafiei Sabet et al., 2016a; Shafiei Sabet et al., 2016b; Jimenez et al., 2020; Sal Moyano et al., 2021). Roberts et al. (2016) found that exposure to anthropogenic substrate-born vibrations, similar to blasting and pile driving, reduced locomotion in adult hermit crabs. Solan et al. (2016) also reported a reduction of locomotor activity when the Norwegian lobster was exposed to continuous and impulsive sound. Snitman et al. (2022) reported that the crab species, Neohelice granulata, had reduced locomotory activity when exposed to diverse sound. The reduced locomotory and movement speed could be related to a distraction or confusion effect. It has been shown that Caribbean hermit crabs in response to motor boat sounds were more inactive and allowed a simulated predator to approach closer before their retracting movement. The crabs reallocated finite attention to the distracting acoustic stimuli and were prevented from responding to an approaching threat (Chan et al., 2010). In earthworms, it has been shown that substrate vibrational sounds mask the vibrational cues of approaching foraging moles, making earthworms more prone to predation risks in ensonified areas (Dominoni et al., 2020). Moreover, earthworms may not be able to discriminate between the subterranean waves from anthropogenic sources and vibrations coming from approaching predators (Dominoni et al., 2020). Thus, sound pollution can potentially have multiple impacts on locomotor activities and threat assessment in invertebrates. Other invertebrate studies have shown that acoustic stimuli can have multiple negative effects (Edmonds et al., 2016; Carroll et al., 2017). Finding a mate or detecting a predator has direct effects on reproductive success and survival in animals (Dall et al., 2005; Kight and Swaddle, 2011; Kunc et al., 2016; Shannon et al., 2016; Dominoni et al., 2020). If shrimp spend more of their time budget finding food or shift their attention from foraging areas because of anthropogenic noise, it may detrimentally alter other important biological activities such as courtship and finding a mate to increase their reproductive success or detecting approaching predators to survive in their habitats. These impacts can induce alterations not only on individuals but also can affect populations and ecosystems, especially when anthropogenic noise impacts keynote predators and functionally important habitat forming species. This disruption may have knock-on effects on important ecological and biological processes such as species abundance, survivorship, and the reproductive success of aquatic organisms (Slabbekoorn et al., 2010; Williams et al., 2015; Shafiei Sabet et al., 2016a; Shafiei Sabet et al., 2016b).
In the control treatment, no difference was observed in the horizontal spatial distribution between individuals, but in the sound treatment, the horizontal distribution of shrimp significantly increased when comparing the point nearest the underwater sound source with the furthest away. This spatial displacement due to sound exposure may have potentially negative impacts on the abundance of invertebrates (e.g. earthworm) (Velilla et al., 2021). The authors reported that earthworm abundance decreased with increasing vibratory noise. Similar behavior has been reported in other species that have been exposed to annoying or disturbing sounds (De Vincenzi et al., 2015; Shafiei Sabet et al., 2016a; Shafiei Sabet et al., 2016b). In mobile species such as the red cherry shrimp, locomotion is an important link between the behavior of individuals and ecological processes (Herrnkind, 1983; Spanier et al., 1988; Lawton and Lavalli, 1995). Therefore, any impacts of anthropogenic noise on their locomotor activities may have detrimental consequences on their other biological activities such as foraging or anti-predator behavior. Although, the impact of acoustic stimuli on the spatial distribution of red cherry shrimp is not fully understood, in other species there are indications that anthropogenic noise affects swimming activities and spatial displacement. Shafiei Sabet et al. (2016a) reported that sound treatment caused various behavioral changes in both the spatial distribution and swimming behavior of zebrafish within the treatment tank. Sound playback led to more freezing and less time spent near the active speaker. However, this behavioral response to sound exposure contrasts what was observed in another study on zebrafish under laboratory conditions (Neo et al., 2015). Shafiei Sabet et al. (2016b) compared zebrafish and Lake Victoria cichlids, the former being sensitive to lower absolute thresholds and wider spectral ranges. It is likely that consistent with our study, experimental sound playback induced a significant reduction in movement speed in the first few minutes of exposure for both species under captive conditions. Neither species showed spatial shifts away from the active speaker in the horizontal plane (Shafiei Sabet et al., 2016b). We reported non-acoustic dependent changes in the movement behavior of the shrimp. Opening the divider plate caused a non-auditory behavioral response by reducing the instant movement speed of shrimp, which may be an anti-predator response and likely improves their survival by lowering visual visibility. Another assumption would be cross sensory interference (Hubert et al., 2021): acoustic stimuli may interfere with their visual activities and food detecting performance (Solé et al., 2023). It is more likely that our sound playbacks caused negative effects on olfactory-mediated food finding behavior of shrimp (Hubert et al., 2021; Solé et al., 2023). However, we argue that our experimental design does not allow us to conclude the effect of sound playback on the movement speed of tested animals due to sound playback of treatments starting immediately after the divider plate was removed. When interpreting behavioral responses, it is important to take into account that all observed behavioral changes cannot necessarily always be uniquely attributed to the sound playbacks and methodological approaches across other sensory modalities may also need to be considered. In the present analysis, the experimental set up was successful and able to explore direct assessment of the effects of acoustic stimuli on the horizontal movement of the shrimp. However, acoustics artifacts due to using underwater sound source in a fish tank provide complex sound fields (Parvulescu, 1967; Akamatsu et al., 2002; Rogers et al., 2016). In our recent studies (Neo et al., 2015; Shafiei Sabet et al., 2016c) we did not find any spatial displacement related to sound treatments in captivity. The results of this study indicate that the red cherry shrimp show sound-dependent spatial avoidance and avoid the area closest to the sound source. Particle motion components of the experimental set up were not able to be measured, which makes it likely that the behavioral observations were in response to particle motion component rather than sound pressure (Popper and Hawkins, 2019; Hawkins et al., 2021).
4.2 Acoustic stimuli compromised feeding performance in shrimp
In the present study, feeding latency (latency to find food), in shrimp significantly increased in the sound treatment compared to the control. This delay in finding the food source seems to be due to stress and confusion caused by sound playback, which agrees with previous studies using crustaceans and fish (Hughes et al., 2014; Voellmy et al., 2014; Gendron et al., 2020). Elevated levels of sound in the present study had negative effects on the feeding activity and performance (e.g. feeding latency and food finding) in shrimp under laboratory conditions. More individuals successfully found and consumed the food source in the control treatment than the sound treatment, which is consistent with the findings of Voellmy et al. (2014) and confirms the effect of sound on feeding distraction in both an invertebrate (shrimp) and vertebrate (fish species) respectively. These observations suggest that anthropogenic noise may cause similar impacts on foraging activities in animals under laboratory conditions. It is important to consider that the findings of the present study should not be interpreted for wild animals and fish in field conditions. Various studies have shown that food-deprivation influences behavior including foraging; food-deprived three-spined sticklebacks were more likely to initiate predator inspection visits and had higher feeding rates than well-fed shoal mates (Godin and Crossman, 1994).
The number of revisits to the food source also significantly decreased when exposed to sound. Such changes in feeding behavior have not been reported previously. This study showed that anthropogenic noise pollution influences the behavioral activity of the red cherry shrimp. Currently, no data on the sensitivity of red cherry shrimp to acoustic signals is available; however, the results of the present study indicate that N. davidi may perceive all or part of the acoustic stimuli projected within the wider band-width of their underwater audible rasps characterized by signals with most of energy concentrated in the 4–2000 Hz range, with the peak frequency of 700 Hz and peak amplitude of 120 dB re 1 lPa (Buscaino et al., 2011). Filiciotto et al. (2014) found that lobsters exposed to boat sounds had higher mobility and moving state values compared to lobsters in the control treatment. Shafiei Sabet et al. (2019) found no significant changes in behavioral parameters of Daphnia magna while Sal Moyano et al. (2021) evaluated the effects of biological and anthropogenic acoustic signals on the orientation response of different stages (megalopae and juveniles) of 4 brachyuran crabs species. C. angulatus megalopae and juveniles responded positively towards crustacean signals, while juveniles responded negatively towards fish sounds. N. granulata juveniles orientated negatively towards crustacean, motorboat and fish signals while C. altimanus and L. uruguayensis juveniles did not respond to fish signals (Sal Moyano et al., 2021). These findings support the idea that invertebrates potentially can be distracted and impress by anthropogenic noise and highlight the role of acoustic stimuli on feeding performance and prey–predator relationships.
4.3 Do acoustic stimuli compromise food source assessment and attention in shrimp?
In the sound treatment, significantly more individuals were distracted in feeding compared to the control treatment. Similar results are reported in other species (Wale et al., 2013; Hughes et al., 2014; Voellmy et al., 2014; Gendron et al., 2020; Hastie et al., 2021). Wale et al. (2013) argued that crabs exposed to ship noise were more likely to suspend feeding than those exposed to ambient noise. McLaughlin and Kunc (2015) found that cichlids exposed to anthropogenic noise showed an increase in sheltering accompanied by a decrease in foraging. Their results highlight the multiple negative effects of an environmental stressor on an individual’s behavior. However, there are studies that show no effects on foraging behavior and locomotion on crustaceans after sound exposure (Hubert et al., 2021; Solé et al., 2023). Hubert et al. (2021) found no overall effect of boat sound on food finding success and efficacy, foraging duration or walking distance in shore crabs. This may be due to differences in characteristics (range of frequencies/exposure intensity levels) of provided acoustic stimuli or species specific behavioral properties. The observed variable responses in foraging and locomotion behavior may reflect the differences in experimental set up arena such as size and shape (rectangular/maze) of aquariums and the location of the underwater speaker projection (side, upper, button).
There are several mechanisms to explain how anthropogenic noise affects behavior: increasing stress (Smith et al., 2004), masking relevant biological cues (Hawkins and Chapman, 1975), distracting of attention (Dukas, 2004; Chan et al., 2010), physically damaging sensory system and internal organs (McCauley et al., 2003) and injury or hearing loss in some species (Popper et al., 2005; Halvorsen et al., 2012). These could be operating separately or simultaneously, and in many cases, it is difficult to determine which of these is most relevant, especially in the present study. Wale et al. (2013) showed that the ability of crabs to find food items was not impaired by the playback of ship noise compared to ambient noise. Individuals exposed to ship noise were more likely to suspend feeding than those exposed to ambient noise. Hughes et al. (2014) showed that the sounds made by predatory fish can affect the feeding behavior of crabs. In the presence of the sound of predatory fish in the frequency range of 10 to 1600 Hz and the sound intensity of 146 to 116 dB, the amount of crab feeding from bivalves was significantly reduced. Although it needs further research, shrimp that do not feed properly because of acute and/or chronic exposure to sound could be affected in their feeding performance, growth, size at sexual maturity, mate selection, and brooding eggs behavior, thus affecting the whole life cycle and the species fitness and their role in the trophic webs.
Our results provide important insights into the effects of sound on this species at individual level under laboratory conditions. Shrimp spent more time away from the active speaker suggesting that they were able to detect the acoustic stimuli and further research measuring both sound pressure and particle motion components is recommended (Azarm-Karnagh et al., 2023). Anthropogenic noise decreased food finding success and the number of revisits to the food source, increased feeding latency (latency to find food), and distraction. Overall, the findings of the present study highlight the negative impacts of anthropogenic noise on the behavior of shrimp that could impair their foraging performance and potentially have cascading effects on their survival. It is likely that anthropogenic noise impacts on freshwater aquatic species across ecological levels are underestimated. Based on our current findings on a freshwater invertebrate species (Neocaridina davidi) and recent studies (Rojas et al., 2021; Fernandez-Declerck et al., 2023) on invasive freshwater fish species (the round goby Neogobius melanostomus) and the invasive pumpkinseed sunfish (Lepomis gibbosus), anthropogenic noise could potentially impact on a variety of ecologically important species, as well as on invasive species, and cause sound-dependent behavioral changes. Empirical data is however scarce and more indoor and outdoor studies are needed to provide a clearer understanding of anthropogenic noise trophic impacts and their potential ecological consequences on freshwater (in)vertebrates.
5 Conclusions
The current study examined the effect of sound playbacks on the movement behavior and feeding performance on shrimp. Short-term exposure to sound changed some behavioral patterns in N. davidi. The simulated sound in laboratory conditions influenced the behavior of the animals, mainly in terms of spatial distribution (avoiding the sound source), feeding latency (latency to find food), finding-food successfully, number of revisits to the food source and feeding distraction. We found no evidence that the movement behavior was affected by the sound. As new information becomes available, there is a need to change and develop the sound exposure criteria and implement updates of invertebrates’ perception with regard to the acoustic environment. The sound field metrics used currently are often inappropriate as they are expressed in terms of sound pressure and potential impacts on invertebrates, whereas all fishes and most invertebrates are able to perceive and detect particle motion components (Popper and Hawkins, 2019; Hawkins et al., 2021). Thus, there is a need to explore anthropogenic noise effects on invertebrates in terms of sound pressure levels, particle motion components and substrate vibrations. To assess anthropogenic noise more comprehensively in the next experiments and enable a better understanding of noise impacts on crustaceans, further research should consider analysis of particle motion components and substrate vibrations. Furthermore, experiments should be performed to assess the animal responses in nature where the acoustic field is not influenced by laboratory conditions.
We suggest that to reduce behavioral biases and assess more explicitly anthropogenic noise effects on animals in captivity, welfare issues (e.g. animal’s stock density, vegetation, substrate and aeration in holding tanks) and physiological factors (e.g. level of hunger, starvation period before running experiments) are needed to be considered (Hubert et al., 2018; Hubert et al., 2021; Hubert et al., 2023). To support the development of this field and contribute to open science we further recommend that experimental protocols/designs should be reported in published papers. Longer-term complementary studies under laboratory and field conditions should examine whether the results reported in this work are relevant for the potential chronic effects of sound on populations and on ecological communities, providing and implementing innovative tools for the measure of acoustic pollution.
Data availability statement
The raw data supporting the conclusions of this article will be made available by the authors, without undue reservation. Further inquiries can be directed to the corresponding author.
Ethics statement
Shrimp did not show any sign of anxiety and no sign of adverse effects of the experimental conditions. We adhered to the Guidelines for the treatment of animals in behavioral research and teaching (ASAB, 2023). There are no legal requirements for studies involving crustaceans in Iran. The study was conducted in accordance with the local legislation and institutional requirements.
Author contributions
SA-K: investigation, data curation, visualization, formal analysis, and writing – original draft preparation. LLG: conceptualization, methodology, supervision, and writing – reviewing and editing. SSS: conceptualization, methodology, supervision, project administration, funding acquisition, and writing – reviewing and editing. All authors contributed to the article and approved the submitted version.
Funding
This work was funded by the Iranian Ministry of Science, Research and Technology, University of Guilan with the grant number 981511160005.
Acknowledgments
We thank Jeroen Hubert for his help and feedback to update the R scripts for the fish tank acoustics analysis. We would like to thank Dr Ellen Williams, who assisted with manuscript editing. SSHS has been supported as a member by the International Society for Applied Ethology.
Conflict of interest
The authors declare that the research was conducted in the absence of any commercial or financial relationships that could be construed as a potential conflict of interest.
Publisher’s note
All claims expressed in this article are solely those of the authors and do not necessarily represent those of their affiliated organizations, or those of the publisher, the editors and the reviewers. Any product that may be evaluated in this article, or claim that may be made by its manufacturer, is not guaranteed or endorsed by the publisher.
Supplementary material
The Supplementary Material for this article can be found online at: https://www.frontiersin.org/articles/10.3389/fevo.2023.1091314/full#supplementary-material
References
Akamatsu T., Okumura T., Novarini N., Yan H. Y. (2002). Empirical refinements applicable to the recording of fish sounds in small tanks. J. Acoust. Soc Am. 112, 3073e3082. doi: 10.1121/1.1515799
ASAB (2023). Guidelines for the treatment of animals in behavioural research and teaching. Anim. Behav. 135, I–X. doi: 10.1016/j.anbehav.2022.09.006
Azarm-Karnagh S., López Greco L., Shafiei Sabet S. (2023). “Anthropogenic noise impacts on invertebrates: case of freshwater red cherry shrimp (Neocaridina davidi),” in The Effects of Noise on Aquatic Life. Eds. Popper A. N., Sisneros J., Hawkins A. D., Thomsen F. (Cham: Springer). doi: 10.1007/978-3-031-10417-6_151-1
Bell S. S., Coull B. C. (1978). Field evidence that shrimp predation regulates meiofauna. Oecologia 35 (2), 141–148. doi: 10.1007/BF00344727
Bruintjes R., Purser J., Everley K. A., Mangan S., Simpson S. D., Radford A. N. (2015). Rapid recovery following short-term acoustic disturbance in two fish species. R. Soc Op. Sci. 3 (1), 1-13. doi: 10.1098/RSOS.150686
Buscaino G., Filiciotto F., Gristina M., Buffa G., Bellante A., Maccarrone V., et al. (2011). Defensive strategies of European spiny lobster Palinurus elephas during predator attack. Mar. Ecol. Prog. 423, 143–154. doi: 10.3354/meps08957
Carroll A. G., Przeslawski R., Duncan A., Gunning M., Bruce B. (2017). A critical review of the potential impacts of marine seismic surveys on fish & invertebrates. Mar. Poll. Bull. 114 (1), 9–24. doi: 10.1016/j.marpolbul.2016.11.038
Chan A. A. Y. H., Blumstein D. T. (2011). Attention, noise, and implications for wildlife conservation and management. App. Anim. Behav. Sci. 131 (1-2), 1–7. doi: 10.1016/J.APPLANIM.2011.01.007
Chan A. A. Y. H., Giraldo-Perez P., Smith S., Blumstein D. T. (2010). Anthropogenic noise affects risk assessment and attention: the distracted prey hypothesis. Biol. Lett. 6 (4), 458–461. doi: 10.1098/RSBL.2009.1081
Dall S. R. X., Giraldeau L. A., Olsson O., McNamara J. M., Stephens D. W. (2005). Information and its use by animals in evolutionary ecology. Trends Ecol. Evol. 20 (4), 187–193. doi: 10.1016/J.TREE.2005.01.010
De Jong K., Amorim M. C. P., Fonseca P. J., Fox C. J., Heubel K. U. (2018). Noise can affect acoustic communication and subsequent spawning success in fish. Environ. pollut. 237, 814–823. doi: 10.1016/J.ENVPOL.2017.11.003
De Vincenzi G., Maccarrone V., Filiciotto F., Buscaino G., Mazzola S. (2015). Behavioural responses of the European Spiny lobster, Palinurus elephas (Fabricius 1787), to conspecific and synthetic sounds. Crustaceana 88 (5), 523–540. doi: 10.1163/15685403-00003430
Dominoni D. M., Halfwerk W., Baird E., Buxton R. T., Fernández-Juricic E., Fristrup K. M., et al. (2020). Why conservation biology can benefit from sensory ecology. Nat. Ecol. Evol. 4 (4), 502–511. doi: 10.1038/S41559-020-1135-4
Dukas R. (2004). Causes and consequences of limited attention. Brain. Behav. Evol. 63 (4), 197–210. doi: 10.1159/000076781
Edmonds N. J., Firmin C. J., Goldsmith D., Faulkner R. C., Wood D. T. (2016). A review of crustacean sensitivity to high amplitude underwater noise: Data needs for effective risk assessment in relation to UK commercial species. Mar. pollut. Bull. 108 (1-2), 5–11. doi: 10.1016/J.MARPOLBUL.2016.05.006
Fernandez-Declerck M., Rojas E., Prosnier L., Teulier L., Dechaume-Moncharmont F. X., Médoc V. (2023). Adding insult to injury: anthropogenic noise intensifies predation risk by an invasive freshwater fish species. Biol. Invasions 25 (9), 2775–2785. doi: 10.1007/s10530-023-03072-w
Filiciotto F., Vazzana M., Celi M., Maccarrone V., Ceraulo M., Buffa G., et al. (2016). Underwater noise from boats: Measurement of its influence on the behaviour and biochemistry of the common prawn (Palaemon serratus, Pennant 1777). J. Exp. Mar. Biol. 478, 24–33. doi: 10.1016/j.jembe.2016.01.014
Filiciotto F., Vazzana M., Celi M., Maccarrone V., Ceraulo M., Buffa G., et al. (2014). Behavioural and biochemical stress responses of Palinurus elephas after exposure to boat noise pollution in tank. Mar. Pollut. Bull. 84 (1-2), 104–114. doi: 10.1016/j.marpolbul.2014.05.029
Galef B. G. Jr., Giraldeau L. A. (2001). Social influences on foraging in vertebrates: causal mechanisms and adaptive functions. Anim. Behav. 61 (1), 3–15. doi: 10.1006/anbe.2000.1557
Gendron G., Tremblay R., Jolivet A., Olivier F., Chauvaud L., Winkler G., et al. (2020). Anthropogenic boat noise reduces feeding success in winter flounder larvae (Pseudopleuronectes americanus). Environ. Biol. Fishes. 103 (9), 1079–1090. doi: 10.1007/s10641-020-01005-3
Godin J. J., Crossman S. L. (1994). Hunger-dependent predator inspection and foraging behaviours in the three spine stickleback (Gasterosteus aculeatus) under predation risk. Behav. Ecol. Sociobiol. 34, 359–366. doi: 10.1007/BF00197006
Halvorsen M. B., Casper B. M., Matthews F., Carlson T. J., Popper A. N. (2012). Effects of exposure to pile-driving sounds on the lake sturgeon, Nile tilapia and hogchoker. Proc. R. Soci. B: Biol. Sci. 279 (1748), 4705–4714. doi: 10.1098/RSPB.2012.1544
Hastie G. D., Lepper P., McKnight J. C., Milne R., Russell D. J. F., Thompson D. (2021). Acoustic risk balancing by marine mammals: anthropogenic noise can influence the foraging decisions by seals. J. Appl. Ecol. 58 (9), 1854–1863. doi: 10.1111/1365-2664.13931
Hawkins A. D., Chapman C. J. (1975). Masked auditory thresholds in the cod, Gadus morhua. J. Comp. Physiol. A 103, 209–2226. doi: 10.1007/BF00617122
Hawkins A. D., Hazelwood R. A., Popper A. N., Macey P. C. (2021). Substrate vibrations and their potential effects upon fishes and invertebrates. J. Acoust. Soc Am. 149 (4), 2782–2790. doi: 10.1121/10.0004773
Hawkins A. D., Myrberg A. A. (1983). Hearing and sound communication under water. Bioacoustics, a comparative approach (London: Academic Press), 347–405.
Herbert-Read J. E., Kremer L., Bruintjes R., Radford A. N., Ioannou C. C. (2017). Anthropogenic noise pollution from pile-driving disrupts the structure and dynamics of fish shoals. Proc. R. Soc B: Biol. Sci. 284 (1863), 1-9. doi: 10.1098/RSPB.2017.1627
Herrnkind W. F. (1983). “Movements patterns and orientation,” in The Biology of Crustacea, vol. Vol. 7. Behav. Ecol . Eds. Vernberg F. J., Vernberg W. B. (New York, NY: Academic Press), 41–105.
Holles S., Simpson S. D., Radford A. N., Berten L., Lecchini D. (2013). Boat noise disrupts orientation behaviour in a coral reef fish. Mar. Ecol. Prog. Ser. 485, 295–300. doi: 10.3354/meps10346
Hubert J., Campbell J., van der Beek J. G., den Haan M. F., Verhave R., Verkade L. S., et al. (2018). Effects of broadband sound exposure on the interaction between foraging crab and shrimp–A field study. Environ. Pollut. 243, 1923–1929. doi: 10.1016/j.envpol.2018.09.076
Hubert J., van Bemmelen J. J., Slabbekoorn H. (2021). No negative effects of boat sound playbacks on olfactory-mediated food finding behaviour of shore crabs in a T-maze. Environ. pollut. 270, 116184. doi: 10.1016/j.envpol.2020.116184
Hubert J., van der Burg A. D., Witbaard R., Slabbekoorn H. (2023). Separate and combined effects of boat noise and a live crab predator on mussel valve gape behavior. Behav. Ecol. 34 (3), 495–505. doi: 10.1093/beheco/arad012
Hughes A., Mann D. A., Kimbro D. L. (2014). Predatory fish sounds can alter crab foraging behaviour and influence bivalve abundance. Proc. R. Soc B: Biol. Sci. 281 (1788), 1-8. doi: 10.1098/rspb.2014.0715
Huijbers C. M., Nagelkerken I., Lössbroek P. A., Schulten I. E., Siegenthaler A., Holderied M. W., et al. (2012). A test of the senses: fish select novel habitats by responding to multiple cues. Ecology 93 (1), 46–55. doi: 10.1890/10-2236.1
Jimenez V. L., Fakan E. P., McCormick M. I. (2020). Vessel noise affects routine swimming and escape response of a coral reef fish. PloS One 15 (7), e0235742. doi: 10.1371/journal.pone.0235742
Kelly E., Tully O., Browne R. (2012). Effects of temperature and salinity on the survival and development of larval and juvenile Palaemon serratus (Decapoda: Palaemonidae) from Irish waters. J. Mar. Biolog. Assoc. United Kingdom 92 (1), 151–161. doi: 10.1017/S0025315411000415
Kight C. R., Swaddle J. P. (2011). How and why environmental noise impacts animals: An integrative, mechanistic review. Ecol. Lett. 14 (10), 1052–1061. doi: 10.1111/J.1461-0248.2011.01664.X
Klotz W., Miesen F. W., Hüllen S., Herder F. (2013). Two Asian fresh water shrimp species found in a thermally polluted stream system in north Rhine-Westphalia, Germany. Aquat. Invasions 8 (3), 333–339. doi: 10.3391/AI.2013.8.3.09
Kok A., Berkhout B. W., Carlson N. V., Evans N. P., Khan N., Potvin D. A., et al. (2023). How chronic anthropogenic noise can affect wildlife communities. Front. Ecol. Evol. 11, 289. doi: 10.3389/fevo.2023.1130075
Kunc H. P., Lyons G. N., Sigwart J. D., McLaughlin K. E., Houghton J. D. R. (2015). Anthropogenic noise affects behavior across sensory modalities. Am. Nat. 184 (4), E93–E100. doi: 10.1086/677545
Kunc H. P., McLaughlin K. E., Schmidt R. (2016). Aquatic noise pollution: implications for individuals, populations, and ecosystems. Proc. R. Soc B: Biol. Sci. 283 (1836), 1-8. doi: 10.1098/RSPB.2016.0839
Lawton P. K. L., Lavalli N. (1995). “Postlarval, juvenile, adolescent, and adult ecology,” in Biology of the Lobster Homarus americanus. Ed. Factor J. R. (San Diego, CA: Academic Press), 47–88.
Liang X. Q. (2004). Fauna Sinica. Invertebrata-Crustacea-Decapoda-Atyidae (Beijing, China: Science Press).
Magnhagen C., Johansson K., Sigray P. (2017). Effects of motorboat noise on foraging behaviour in Eurasian perch and roach: A field experiment. Mar. Ecol. Prog. Ser. 564, 115–125. doi: 10.3354/meps11997
McCauley R. D., Fewtrell J., Popper A. N. (2003). High intensity anthropogenic sound damages fish ears. J. Acoust. Soc Am. 113, 1–5. doi: 10.1121/1.1527962
McLaughlin K. E., Kunc H. P. (2015). Changes in the acoustic environment alter the foraging and sheltering behaviour of the cichlid Amititlania nigrofasciata. Behav. Processes 116, 75–79. doi: 10.1016/j.beproc.2015.04.012
Mickle M. F., Higgs D. M. (2018). Integrating techniques: A review of the effects of anthropogenic noise on freshwater fish. Can. J. Fish. Aquat. Sci. 75 (9), 1534–1541. doi: 10.1139/cjfas-2017-0245
Morley E. L., Jones G., Radford A. N. (2014). The importance of invertebrates when considering the impacts of anthropogenic noise. Proc. R. Soc B: Biol. Sci. 281 (1776), 1-8. doi: 10.1098/RSPB.2013.2683
Neo Y. Y., Parie L., Bakker F., Snelderwaard P., Tudorache C., Schaaf M., et al. (2015). Behavioral changes in response to sound exposure and no spatial avoidance of noisy conditions in captive zebrafish. Front. Behav. Neurosci. 9, 28. doi: 10.3389/fnbeh.2015.00028
Nowacek D. P., Thorne L. H., Johnston D. W., Tyack P. L. (2007). Responses of cetaceans to anthropogenic noise. Mamm. Rev. 37 (2), 81–115. doi: 10.1111/J.1365-2907.2007.00104.X
Onuki K., Fuke Y. (2022). Rediscovery of a native freshwater shrimp, Neocaridina denticulata, and expansion of an invasive species in and around Lake Biwa, Japan: genetic and morphological approach. Conserv. Genet. 23 (5), 967–980. doi: 10.1007/s10592-022-01467-1
Parvulescu A. (1967). “The acoustics of small tanks,” in Marine Bio- Acoustics. Ed. Tavolga W. N. (Oxford: Pergamon Press).
Peng C., Zhao X., Lio G. (2015). Noise in the sea and its impact on marine organisms, International Int. J. Environ. Res. Public Health 12, 12304–12323. doi: 10.3390/ijerph121012304
Popper A. N., Hawkins A. D. (2019). An overview of fish bioacoustics and the impacts of anthropogenic sounds on fishes. The Journal of the Acoustical Society of America 143 (1), 470–488. doi: 10.1111/JFB.13948
Popper A. N., Smith M. E., Cott P. A., Hanna B. W., MacGillivray A. O., Austin M. E., et al. (2005). Effects of exposure to seismic airgun use on hearing of three fish species. J. Acoust. Soc Am. 117 (6), 3958. doi: 10.1121/1.1904386
Purser J., Radford A. N. (2011). Acoustic noise induces attention shifts and reduces foraging performance in three-spined sticklebacks (Gasterosteus aculeatus). PloS One 6 (2), e17478. doi: 10.1371/JOURNAL.PONE.0017478
Reise K. (1979). Moderate predation on meiofauna by the macrobenthos of the Wadden Sea. Helgoländer Wissenschaftliche. Meeresuntersuchungen. 32 (4), 453–465. doi: 10.1007/BF02277989
Roberts L., Cheesman S., Breithaupt T., Elliott M. (2015). Sensitivity of the mussel Mytilus edulis to substrate-borne vibration in relation to anthropogenically generated noise. Mar. Ecol. Prog. Ser. 538, 185–195. doi: 10.3354/MEPS11468
Roberts L., Cheesman S., Elliott M., Breithaupt T. (2016). Sensitivity of Pagurus bernhardus (L.) to substrate-borne vibration and anthropogenic noise. J. Exp. Mar. Biol. 474, 185–194. doi: 10.1016/j.jembe.2015.09.014
Rogers P. H., Hawkins A. D., Popper A. N., Fay R. R., Gray M. D. (2016). Parvulescu revisited: Small tank acoustics for bioacousticians. Adv. Exp. Med. Biol. 875, 933–941. doi: 10.1007/978-1-4939-2981-8_115/COVER
Rojas E., Gouret M., Agostini S., Fiorini S., Fonseca P., Lacroix G., et al. (2023). From behaviour to complex communities: Resilience to anthropogenic noise in a fish-induced trophic cascade. Environ. Pollut. 335, 122371. doi: 10.1016/j.envpol.2023.122371
Rojas E., Thévenin S., Montes G., Boyer N., Médoc V. (2021). From distraction to habituation: Ecological and behavioural responses of invasive fish to anthropogenic noise. Freshw. Biol. 66 (8), 1606–1618. doi: 10.1111/fwb.13778
RStudio Team (2020). RStudio: Integrated Development for R. (Boston, MA: PBC). Available at: http://www.rstudio.com/.
Sal Moyano M. P., Ceraulo M., Hidalgo F. J., Luppi T., Nuñez J., Radford C. A., et al. (2021). Effect of biological and anthropogenic sound on the orientation behavior of four species of brachyuran crabs. Mar. Ecol. Prog. Ser. 669, 107–120. doi: 10.3354/meps13739
Sarà G., Dean J. M., D’Amato D., Buscaino G., Oliveri A., Genovese S., et al. (2007). Effect of boat noise on the behaviour of bluefin tuna Thunnus thynnus in the Mediterranean Sea. Mar. Ecol. Prog. Ser. 331, 243–253. doi: 10.3354/MEPS331243
Shafiei Sabet S., Karnagh S. A., Azbari F. Z. (2019). Experimental test of sound and light exposure on water flea swimming behaviour. Proc. Meet. Acoust. 38, 010015. doi: 10.1121/2.0001270
Shafiei Sabet S., Neo Y. Y., Slabbekoorn H. (2015). The effect of temporal variation in sound exposure on swimming and foraging behaviour of captive zebrafish. Anim. Behav. 107, 49–60. doi: 10.1016/j.anbehav.2015.05.022
Shafiei Sabet S., Neo Y. Y., Slabbekoorn H. (2016c). “Impact of anthropogenic noise on aquatic animals: from single species to community-level effects,” in The effects of noise on aquatic life II (New York: Springer), 957–961.
Shafiei Sabet S., Van Dooren D., Slabbekoorn H. (2016a). Son et lumière: Sound and light effects on spatial distribution and swimming behavior in captive zebrafish. Environ. Poll. 212, 480–488. doi: 10.1016/j.envpol.2016.02.046
Shafiei Sabet S., Wesdorp K., Campbell J., Snelderwaard P., Slabbekoorn H. (2016b). Behavioural responses to sound exposure in captivity by two fish species with different hearing ability. Anim. Behav. 116, 1–11. doi: 10.1016/j.anbehav.2016.03.027
Shannon G., McKenna M. F., Angeloni L. M., Crooks K. R., Fristrup K. M., Brown E., et al. (2016). A synthesis of two decades of research documenting the effects of noise on wildlife. Biol. Rev. 91 (4), 982–1005. doi: 10.1111/BRV.12207
Simpson S. D., Radford A. N., Nedelec S. L., Ferrari M. C. O., Chivers D. P., McCormick M. I., et al. (2016). Anthropogenic noise increases fish mortality by predation. Nat. Commun. 7 (1), 1–7. doi: 10.1038/ncomms10544
Slabbekoorn H., Bouton N., van Opzeeland I., Coers A., ten Cate C., Popper A. N. (2010). A noisy spring: the impact of globally rising underwater sound levels on fish. Trends Ecol. Evol. 25 (7), 419–427. doi: 10.1016/J.TREE.2010.04.005
Slabbekoorn H., Dalen J., de Haan D., Winter H. V., Radford C., Ainslie M. A., et al. (2019). Population-level consequences of seismic surveys on fishes: An interdisciplinary challenge. Fish Fish. 20 (4), 653–685. doi: 10.1111/FAF.12367
Smith M. E., Kane A. S., Popper A. N. (2004). Noise-induced stress response and hearing loss in goldfish (Carassius auratus). J. Exp. Biol. 207, 427–435. doi: 10.1242/jeb.00755
Snitman S. M., Mitton F. M., Marina P., Maria C., Giuseppa B., Gavio M. A., et al. (2022). Effect of biological and anthropogenic habitat sounds on oxidative stress biomarkers and behavior in a key crab species. Comp. Biochem. Physiol. C: Toxicol. Pharmacol. 257, 1–42. doi: 10.1016/j.cbpc.2022.109344
Solan M., Hauton C., Godbold J. A., Wood C. L., Leighton T. G., White P. (2016). Anthropogenic sources of underwater sound can modify how sediment-dwelling invertebrates mediate ecosystem properties. Sci. Rep. 6 (1), 1–9. doi: 10.1038/srep20540
Solé M., Kaifu K., Mooney T. A., Nedelec S. L., Olivier F., Radford A. N., et al. (2023). Marine invertebrates and noise. Front. Mar. Sci. 10, 185. doi: 10.3389/fmars.2023.1129057
Southall B. L., Bowles A. E., Ellison W. T., Finneran J. J., Gentry R. L., Greene C. R., et al. (2008). Marine mammal noise-exposure criteria: Initial scientific recommendations. Bioacoustics 17 (1-3), 273–275. doi: 10.1080/09524622.2008.9753846
Spanier E., Tom M., Pisants S., Almong G. (1988). Seasonality and shelter selection by the slipper lobate Scyllarides latus in the Southeastern mediterranean. Mar. Ecol. Prog. Ser. 42, 247–255. doi: 10.3354/meps042247
Velilla E., Collinson E., Bellato L., Berg M. P., Halfwerk W. (2021). Vibrational noise from wind energy-turbines negatively impacts earthworm abundance. Oikos 130 (6), 844–849. doi: 10.1111/OIK.08166
Voellmy I. K., Purser J., Flynn D., Kennedy P., Simpson S. D., Radford A. N. (2014). Acoustic noise reduces foraging success in two sympatric fish species via different mechanisms. Anim. Behav. 89 (March), 191–198. doi: 10.1016/j.anbehav.2013.12.029
Wale M. A., Simpson S. D., Radford A. N. (2013). Noise negatively affects foraging and antipredator behaviour in shore crabs. Anim. Behav. 86 (1), 111–118. doi: 10.1016/j.anbehav.2013.05.001
Weber S., Traunspurger W. (2016). Influence of the ornamental red cherry shrimp Neocaridina davidi (Bouvier 1904) onfreshwater meiofaunal assemblages. Limnologica 59 (June 2016), 155–161. doi: 10.1016/j.limno.2016.06.001
Williams R., Wright A. J., Ashe E., Blight L. K., Bruintjes R., Canessa R., et al. (2015). Impacts of anthropogenic noise on marine life: Publication patterns, new discoveries, and future directions in research and management. Ocean Coast. Manage. 115, 17–24. doi: 10.1016/J.OCECOAMAN.2015.05.021
Wowor D., Cai Y., Ng P. K. L. (2004). “Crustacean: decapoda: caridea,” in The Freshwater Invertebrates of Malaysia and Singapore. Eds. Yule C., Yong H. S. (Kuala Lumpur: Malaysian Academy of Sciences), 337–357.
Wright A. J., Soto N. A., Baldwin A. L., Bateson M., Beale C. M., Clark C., Martin V. (2007a). Do marine mammals experience stress related to anthropogenic noise? Inter. J. Comp. Psycho. 20 (2), 274–316. doi: 10.46867/ijcp.2007.20.02.01
Keywords: behavior, ornamental shrimp, feeding performance, noise pollution, movement activities, invasive species
Citation: Azarm-Karnagh S, López Greco L and Shafiei Sabet S (2023) Annoying noise: effect of anthropogenic underwater noise on the movement and feeding performance in the red cherry shrimp, Neocaridina davidi. Front. Ecol. Evol. 11:1091314. doi: 10.3389/fevo.2023.1091314
Received: 06 November 2022; Accepted: 31 October 2023;
Published: 19 December 2023.
Edited by:
Chris Templeton, Western Washington University, United StatesReviewed by:
Felipe N. Moreno-Gómez, Universidad Católica del Maule, ChileMarta Solé, BarcelonaTech (UPC), Spain
Copyright © 2023 Azarm-Karnagh, López Greco and Shafiei Sabet. This is an open-access article distributed under the terms of the Creative Commons Attribution License (CC BY). The use, distribution or reproduction in other forums is permitted, provided the original author(s) and the copyright owner(s) are credited and that the original publication in this journal is cited, in accordance with accepted academic practice. No use, distribution or reproduction is permitted which does not comply with these terms.
*Correspondence: Saeed Shafiei Sabet, cy5zaGFmaWVpLnNhYmV0QGd1aWxhbi5hYy5pcg==