- 1Department of Plant, Soil and Microbial Sciences, Michigan State University, East Lansing, MI, United States
- 2USDA Forest Service, Northern Research Station, Delaware, OH, United States
- 3USDA Forest Service, Northern Research Station, Rhinelander, WI, United States
- 4Forestry Division, Wisconsin Department of Natural Resources, Rhinelander, WI, United States
- 5USDA Forest Service, Northern Research Station, Irvine, PA, United States
Climate change represents an existential threat to many forest ecosystems because tree populations are often adapted to local climate means and variability. If tree populations cannot migrate or adapt, they risk becoming increasingly maladapted with climate change. This emerging mismatch underscores the need for climate adaptive management techniques, such as assisted migration of tree species, to help mitigate climate change impacts on forest ecosystems. Although biotic and abiotic factors are known to constrain tree establishment success, the extent to which they may determine the success of assisted migration plantings is poorly understood. Thus, defining the extent to which trees affect—and in turn are affected by local soil environments and microbial communities (i.e., plant-soil feedbacks; hereafter PSFs) remains important for guiding effective climate-adaptive forest management. Our objectives were to synthesize the current state of knowledge about the direction and magnitude of PSF effects on temperate tree species of eastern North America, and to identify key hypotheses important for guiding future research. To accomplish these goals, we conducted a meta-analysis of 26 peer-reviewed publications that addressed our criteria. Our compiled database included 61 tree species and was composed primarily of short-term greenhouse experiments that studied PSF effects by manipulating the soil biota in three ways: (1) soil was previously inoculated by a conspecific or heterospecific tree species (i.e., home vs. away), (2) soil was live or sterilized, or (3) soil was untreated or treated with fungicide. We found that PSF had significant effects on tree growth, with the direction and magnitude of PSF strongly dependent on tree mycorrhizal guild. Arbuscular mycorrhizal tree species grown in live or home soils grew 13–33% less than those in sterile or away soils, while ectomycorrhizal tree species grew 11–44% more in live or home than sterile or away soils. PSF effects were associated with several plant functional traits, including specific leaf area, tissue nitrogen, and specific root length. We provide suggestions on incorporating PSFs into assisted migration trials and outline key knowledge gaps for future research. Our synthesis of context-dependent effects of PSFs on tree performance will help inform management decisions involving assisted migration.
1. Introduction
Climate change is leading to observed shifts in tree species ranges (Parmesan and Yohe, 2003; Fei et al., 2017). However, the unprecedented rate of contemporary climate change, along with the long lifespan and short dispersal distances of many tree species (Rogers et al., 2017), will likely limit the ability of tree populations to continue to respond to climate change via adaptation and migration (Davis and Shaw, 2001; Loarie et al., 2009; Prasad et al., 2020). Maladaptation to local environmental conditions caused by climate change threatens tree species” persistence, forest biodiversity, timber production, and the provisioning of critical ecosystem services globally (Bastin et al., 2019; FAO and UNEP, 2020). One potential management tool for aiding in the migration of populations to new climate conditions is assisted migration, defined as the intentional movement of individuals and populations within (i.e., assisted gene flow; Aitken and Whitlock, 2013) or outside the species' existing range (i.e., assisted colonization; Etterson et al., 2020).
Although there is increasing interest in assisted migration as an adaptation tool (Hoegh-Guldberg et al., 2008; Charles and Stehlik, 2020), any long-distance movement, whether natural or assisted, will expose tree populations to novel variation in edaphic conditions and soil biota (Van Grunsven et al., 2010), and risks disrupting the migrant and resident populations' local adaptation to plant-soil interactions (PSFs) (van der Putten, 2012), which are defined as plant-induced changes to biotic and abiotic soil conditions that affect subsequent plant fitness (Bever, 1994; Bever et al., 1997; Wardle, 2002). Such PSFs have a fundamental role in determining plant abundance (Klironomos, 2002; Mangan et al., 2010; van der Putten et al., 2013; Reinhart et al., 2021), productivity (Maron et al., 2011), invasibility (Klironomos, 2002; Callaway et al., 2004), species coexistence (Bever, 2003), and community assembly (Kardol et al., 2006). Therefore, PSFs may act as a critical constraint of tree migration within and beyond range limits (Cardinaux et al., 2018). Overlooking PSFs may lead to mixed or low establishment success, as tree fitness often declines outside its native range (Benning and Moeller, 2021).
Past studies in temperate forests have shown a range of PSF effects on tree fitness, including negative (Packer and Clay, 2000; Reinhart et al., 2005; Jevon et al., 2020; Lance et al., 2020), positive (Carteron et al., 2020; Lance et al., 2020), and neutral (Yang et al., 2013; McCarthy-Neumann and Kobe, 2019). The effect of PSFs on tree fitness depends on the net effect of several interdependent mechanisms. PSFs are considered negative when natural enemies (e.g., pathogens, herbivores), autotoxic compounds, or declines in litter quality and soil nutrients reduce soil suitability for conspecific plant species and thus decrease host plant fitness (Bever et al., 2015). In contrast, PSFs are considered positive when increased access to resources, allelopathic chemicals, or accumulation of mutualists make the soil more suitable for conspecifics and therefore increase the chances of that species dominating (Kulmatiski et al., 2017; Smith-Ramesh and Reynolds, 2017; Bennett and Klironomos, 2019).
Given the large variation in PSF effects on tree fitness, there is a strong need to understand the factors that govern the strength and direction of PSFs in forest communities. A plant trait-based approach is a promising framework to help understand differential tree species responses to PSF, especially in diverse forest communities (Baxendale et al., 2014). Plant functional traits reflect a fundamental trade-off between resource acquisition and conservation, also known as a growth-defense trade-off (Connell, 1971; Coley et al., 1985) and leaf economics spectrum (Wright et al., 2004; Reich, 2014). Fast-growing plant species are characterized by resource acquisitive traits (e.g., high tissue nitrogen [N], specific leaf area [SLA], and specific root length [SRL]), poor investment in defense, and high-quality soil inputs shown to promote rapid nutrient cycling. In contrast, slow-growing plant species are characterized by resource conservative traits (e.g., low tissue N, low SLA, and high fine root diameter [FRD]), high investment in defense, and low-quality soil inputs shown to promote slow nutrient cycling (Orwin et al., 2010; de Vries et al., 2012). Past studies of predominately grassland communities have shown that early successional and fast-growing species tend to suffer from more negative PSFs than late-successional and slow-growing species (Kardol et al., 2006; Kulmatiski et al., 2008, 2017; Bauer et al., 2015; Lemmermeyer et al., 2015; Cortois et al., 2016; Semchenko et al., 2018; Xi et al., 2021), a pattern presumably caused by fast-growing plant species having fewer defenses against soil pathogens (Semchenko et al., 2018).
Additionally, recent research in shrubland and forest communities has shown that the nutrient acquisition strategy of plants can affect PSFs by determining the associated soil biota (i.e., mutualists, pathogens) (Bennett et al., 2017; Teste et al., 2017). Specifically, the benefits plants derive from mycorrhizal fungi may depend on the type of association formed. Plants that form symbioses with ectomycorrhizal (EM) fungi may benefit from greater access to and transfer of nitrogen by EM (Corrales et al., 2016). Additionally, the protective sheaths EM fungi create around plant roots may offer protection from antagonists (Laliberté et al., 2015). As a result, EM plants may experience more positive PSFs than plants that form symbioses with arbuscular mycorrhizal (AM) fungi (Duchesne et al., 1989; Bennett et al., 2017). Despite the growing body of evidence on the importance of plant traits for mediating the effects of PSFs in herbaceous plant communities, knowledge of how PSFs and traits interact in forest communities is critically lacking (Thakur et al., 2021). Synthesizing our state of knowledge can help advance our understanding of the role of PSFs in constraining/facilitating tree establishment (Xi et al., 2021) and inform forest management, including restoration and climate-adaptive forestry (Suding et al., 2013; Wubs et al., 2016).
We performed a meta-analysis of greenhouse and field experiments to summarize the effects of plant-soil feedbacks (PSF) on the growth of trees native to temperate forests of eastern North America. Our goal was to synthesize our understanding on the effects of PSFs on tree establishment and evaluate how plant functional traits mediate PSFs. Additionally, we sought to identify key knowledge gaps concerning PSFs as a critical constraint to tree establishment, especially in the context of assisted migration and reforestation. Our study sought to address the following three research questions: (1) How do manipulations of the soil biota alter the direction/strength of PSFs on tree growth? (2) How well do above- and below-ground plant functional traits explain PSF variation in tree species? and (3) How well does mycorrhizal guild explain variation in PSFs? Our synthesis will help explain variation of PSFs among tree species, facilitate prediction of PSF effects for understudied species, and advance understanding of the factors that may constrain species range expansion under climate change.
2. Methods
2.1. Study selection
We conducted an extensive literature search for PSF studies on temperate tree species of eastern North America by searching Web of Science through 10 December 2021 using the following terms: (“temperate forest*” OR “boreal forest*” OR “North America*” OR Canada OR “United States”) AND (experiment* OR “common garden*” OR microcosm* OR greenhouse OR glasshouse OR feedback) AND (germinat* OR growth OR height OR biomass OR surviv* OR emerg* OR establish* OR recruit* OR feedback) AND [(“plant-soil” OR “plant-soil feedback*” OR “plant soil feedback*” OR mycorrhiza*) OR (soil, feedback AND experiment) OR (plant, soil AND transplant) OR (conspecific* AND “density-dependen*”) OR (“Janzen-Connell”) OR (“host-specific” AND “soil” AND “pathogen*”)]. Our search yielded 1,700+ publications.
From this pool, we screened titles and abstracts to ensure that papers were in the relevant subject area. After this preliminary screening, the eligibility of 343 full-text articles was assessed using four criteria: First, the study had to be empirical with at least one focal tree species native to boreal and/or temperate forests of eastern North America. Second, the experimental design had to include at least one PSF treatment: plants grown in (i) conspecific and heterospecific soils (i.e., soils conditioned by the same or different tree species, respectively), (ii) live and sterile soils, or (iii) with and without fungicide. Observation studies that were instead focused on distance- or density-dependent effects on tree fitness were excluded. Soils conditioned by heterospecific species were limited to native and invasive non-native tree and shrub species. We did not exclude studies where soils were conditioned in the field, as long as the authors stated that soils were collected near specific species. Third, the study was not solely focused on soil inoculum sourced from sites highly impacted by human activities (e.g., agricultural or mining activities). Fourth, the studies had to report measures of mean, error and sample size for the performance of each focal tree species in the PSF testing phase. We considered one type of plant performance: growth, defined as the change in biomass, height or basal diameter. After applying these selection criteria, we retained a total of 26 publications (Supplementary material S1). A PRISMA flow diagram of the studies screened and included in the meta-analysis can be found in the supplement (Supplementary Figure S1).
2.2. Data extraction and moderators
Means, measures of error and sample sizes were extracted from text, tables, figures or correspondence with authors. Where data were not available in tabular form, we used the metaDigitise package in R (Pick et al., 2019) to extract data from figures. When replication varied among treatments in a study, we used the smallest reported value. Note that rather than attempt to delineate “biological” vs. “technical” replication ourselves, we instead relied on the sample size reported by the original authors (see “Potential caveats” section in Discussion). When studies reported multiple experiments (e.g., multiple PSF treatments or focal species), we considered these different “experiments” within the same study and collected data for each treatment. Similar to previous meta-analyses (e.g., Levine et al., 2004; Hoeksema et al., 2010; Lekberg et al., 2018), we considered experiments independent unless they used the same plant species, soil inoculum, and PSF treatments. When studies reported multiple plant growth responses, we preferentially extracted data on total biomass, followed by shoot biomass, height, and basal diameter.
For each article, we collected information on thirteen moderators we hypothesized could influence the strength and direction of PSF (Table 1). We recorded PSF treatment, focal tree species, conditioning tree species, inoculant type (whole soil, roots only, microbial wash), inoculant mycorrhizal guild (AM fungi, EM fungi, or both), latitude and longitude of soil collection locations, conditioning environment (field, lab, or both in cases where soil was conditioned in the field and further conditioned in the greenhouse), testing environment (field or lab), testing community (individual, population, or community), and the duration of the experiment. Hereafter, we refer to the three ways soil biota were manipulated in our retained set of publications as follows: (1) soil was previously inoculated by the focal plant species or a competitor (PSFhome/away), (2) live or sterilized soil (PSFlive/sterile), or (3) soil was untreated or treated with fungicide (PSFdiverse/fungicide).
Where available, we recorded the fraction of inoculum to sterile, background soil to identify those experiments that controlled for differences in abiotic soil properties. In time series experiments, we retained only the last measurement to ensure independence of data. If more than one publication presented results from the same experiment, data from only the most recent paper were extracted. If experiments manipulated additional factors, such as soil origin or resource availability (e.g., light or nutrients), we included data as dependent “trials” within experiments. We incorporated multiple trials within experiments in our analysis as random permutations (sensu Lekberg et al., 2018).
2.3. Plant functional traits
To explore the relationship between PSFs and plant functional traits, we chose seven traits closely associated with plant life-history strategies: mycorrhizal guild (AM, EM or ericoid [ERM]), specific leaf area (SLA, mm2 mg−1), leaf N concentration (LNC, mg g−1), specific root length (SRL, cm g−1), fine root diameter (FRD, mm), maximum plant height (m), and shade tolerance score (Niinemets and Valladares, 2006). SLA, LNC, SRL, and FRD are related to above- and below-ground carbon and nutrient acquisition and reflect the trade-off between growth and defense (Wright et al., 2004; Reich, 2014). Maximum plant height correlates with tree size and lifespan. Shade tolerance is a fundamental life history tradeoff in light-limited forest communities and is often associated with late successional species that invest in defense over growth (Coley and Barone, 1996; Reich et al., 1998). Given their strong phylogenetic signal, we focused on quantitative leaf traits rather than clade information (i.e., gymnosperm and angiosperm) in. our analysis. Trait data were obtained from the TRY Database (Kattge et al., 2020), the Fine-Root Ecology Database (FRED; Iversen et al., 2021), and additional sources (Tobner et al., 2013; Comas et al., 2014; Lihui et al., 2021; Ward, 2021; Weemstra et al., 2021). We used average trait values rather than site- or population-specific trait values because intraspecific trait variability was beyond the scope of our study. Although our compiled set of studies included some dual-mycorrhizal tree species, we considered only the dominant type of mycorrhizal association for a given focal and conditioning tree species.
2.4. Effect size calculations
Overall PSF effects were calculated across all experiments (overall effect) and by treatment type: (i) in soil conditioned by conspecific or heterospecific tree species (PSFhome/away), (ii) in live or sterile soil (PSFlive/sterile), or (iii) with or without fungicides (PSFdiverse/fungicide). We focused on these treatments because they address different aspects of PSF. Specifically, home/away tests for tree species-specific effects of soil biota, live/sterile assesses the net effect of all soil biota, and diverse/fungicide examines the net effect of soil fungi affected by fungicide. Although they do not occur naturally, sterilization and fungicide treatments may more adequately create conditions where key soil biota (e.g., pathogens and mutualists) are present, ineffective, or absent than home/away comparisons (e.g., Augspurger and Wilkinson, 2007; Hersh et al., 2012; Cardillo et al., 2018; see references in Lekberg et al., 2018).
The effect size of individual PSFs were calculated using the natural log of the response ratio (RR):
where and are the mean biomass or height in treatment and control soil, respectively. The natural log of the RR was used for all analyses because it has a more normal distribution and less bias than untransformed RR (Hedges et al., 1999). Prior to reporting, estimates of effect size were back-transformed. We report the RR as the effect size because its interpretation is straightforward (e.g., RR = 1.5 indicates 50% increase in plant growth; 0.5 indicates 50% decrease). Variance of each effect size was estimated as:
where SDt and SDc are the standard deviations of biomass or height and Nt and Nc are the sample sizes in treatment and control soils, respectively. We considered effect sizes significant when estimates of 95% confidence intervals excluded the value of no effect (RR = 1). Individual effect sizes and associated variances were calculated using the “escalc” function and the “ROM” measure (the log-transformed ratio of means) within the “metafor” package (Viechtbauer, 2010) in R (R Core Team, 2022).
2.5. Meta-analysis statistics
A multilevel random-effects meta-analysis model was used to estimate the mean effect and confidence intervals for overall PSF effect on tree performance, with study and focal species specified as random effects. We also used multilevel mixed-effects models to determine if PSF treatments (home/away, live/sterile, diverse/fungicide) explained a significant portion of variation in overall effect size. The multilevel structure allowed us to estimate variance among studies (, sigma2.1 in Supplementary Table S1) separate from variance attributable to focal species identity (, sigma2.2 in Supplementary Table S1). The model creates a weight matrix that depends on sigma2.1, sigma2.2, and the variance-covariance of the sampling errors. As a result, more precise estimates were given more weight in our analysis than less precise estimates (Konstantopoulos, 2011). Models were fit using the restricted maximum-likelihood estimation and run using the “rma.mv” function in the “metafor” package (Viechtbauer, 2010). We report mean values from 1,000 permutations of the dataset where each permutation included a randomly selected trial of each experiment. Associated P-values correspond to the mean test statistics across permutations. All statistical analyses were conducted using R (R Core Team, 2022).
2.6. Evaluation of models
Evidence for heterogeneity (models without moderators) and residual heterogeneity (models with moderators) was evaluated using the generalized least-squares extension of Cochran's Q and QU tests, respectively (Cochran, 1954). We found significant study heterogeneity in our models, indicating that unexplained variance occurred outside the expected sampling error, suggesting that additional explanatory factors may be required to understand patterns. This is a commonly reported issue in meta-analyses (e.g., Treseder, 2004; Barto and Rillig, 2010; Lekberg et al., 2018) that may be improved with better reporting of critical covariates (e.g., resource availability) in PSF experiments. There was no evidence of systematic publication bias in models from visual analysis of funnel plots (Light and Pillemer, 1984).
2.7. Correlation and residual analyses
We used a series of univariate meta-regressions with random effects to test our hypothesis regarding linkages between traits and PSFs. For each trait model, articleID and focal tree species were used as random intercepts. The total heterogeneity of effect sizes (QT) was partitioned into residual heterogeneity (QE) and explained heterogeneity (QM; Borenstein et al., 2009). The QM statistic follows a chi-squared distribution under the null hypothesis that the effect size is the same for all subgroups.
To assess the correlation between the observed strength of PSF and other moderator variables (i.e., study duration, conditioning environment, testing environment, testing community; Table 1), we calculated Pearson's correlation coefficient between individual effect sizes of PSF and each moderator. We also used multiple linear regression to assess whether study metadata could account for variation among residuals in the correlation. We proceeded with a stepwise model selection approach, where the null model included all explanatory metadata that did not have missing values. An additive model was used because metadata were not sufficiently balanced to allow for interactions to be calculated.
3. Results
Our full database included 64 trials from 104 experiments collected from 26 publications conducted over a 20-year period from 2001 to 2021 (Supplementary Figure S2). The database included 61 focal tree species and was composed primarily of short-term (4 to 16 months) greenhouse studies with field and common garden studies representing only 13 trials from 25 experiments reported in seven publications. Eighteen of the 61 temperate tree species had at least two studies that met our search criteria (Supplementary Figure S3). The most studied species were Quercus rubra (n = 9 publications), Acer saccharum (n = 8), Prunus serotina (n = 8) and A. rubrum (n = 7), followed by Q. alba, Robinia pseudoacacia, and Tsuga canadensis (n = 4). Most studies examined tree growth (n = 19), followed by survival (n = 12) and mycorrhizal colonization (n = 8) (Supplementary Figure S4A). Across all response types, most studies examined home-away PSF (n = 24) followed by inactive-active (n = 15) and diverse-fungicide treatments (n = 4) (Supplementary Figure S4B).
The overall mean PSF effect on tree growth across all studies was neutral (mean RR: 1.04; 95% CI: 0.88 to 1.22; d.f. = 143; Z = 0.46, P = 0.64), with significant variation in feedback magnitude (range: 0.30 to 3.64; QE = 1,781.7; P < 0.001). The magnitude and direction of PSF varied among treatments (Figure 1). The effect of PSFhome/away and PSFlive/sterile on tree growth were positive and negative, respectively; trees grown in away and live soils were smaller than plants grown in home or sterile soils. The PSFhome/away effect was significant (mean RR: 1.11; 95% CI: 0.96 to 1.29; Z = 12.33, P < 0.001), whereas the PSFlive/sterile effect was marginally significant (mean RR: 0.86; 95% CI: 0.74 to 1.01; Z = −1.87, P = 0.06). Of the only n = 2 studies on fungicide application, PSFdiverse/fungicide did not affect tree growth.
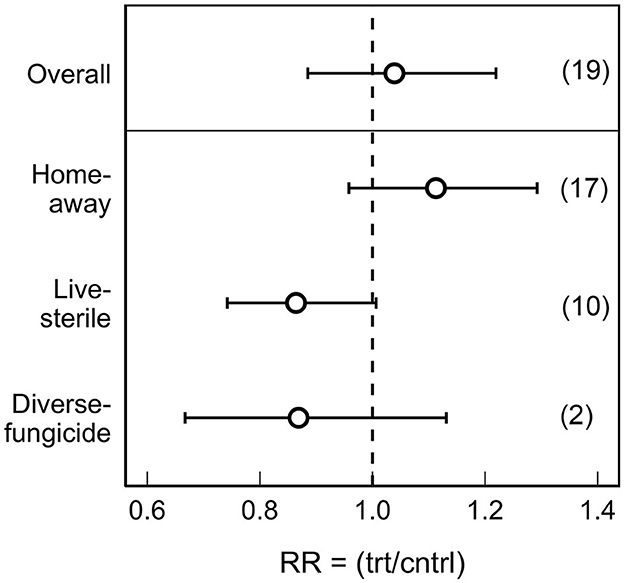
Figure 1. Mean (± 95% confidence intervals) effect sizes of plant-soil feedbacks (PSF) on tree growth across all experiments (Overall) and by treatment type. Our measure of effect size was the response ratio (RR), i.e., the mean plant biomass or height in the treatment (trt) soil divided by the control (cntrl) soil. Overall effect sizes were determined from a model including only random effects, whereas treatment effects were derived from models including treatment type and random effects. PSF treatments included plants cultured by conspecific or heterospecific (Home-away), grown in live or sterile soil (Live-sterile), and without or with fungicides (Diverse-fungicide). The number of publications included in the effect size calculations are shown in parentheses.
The direction and magnitude of PSF varied significantly with mycorrhizal guild (QM = 220.6; P < 0.001; Supplementary Table S1); the effect of PSF was generally negative for AM tree species and positive for EM tree species (Supplementary Table S2). When examined by treatment type, both PSFhome/away and PSFlive/sterile had a negative effect on AM tree growth (PSFhome/away mean RR: 0.86; 95% CI: 0.74 to 1.00; PSFlive/sterile mean RR: 0.67; 95% CI: 0.57 to 0.78); AM trees grown in home and live soils were smaller than those grown in away or sterile soils (Figure 2). In contrast, EM experienced neutral or positive PSF effects on growth under both PSF treatments, but especially in home-away soils (PSFlive/sterile mean RR: 1.12; 95% CI: 0.96 to 1.31; PSFhome/away mean RR: 1.44; 95% CI: 1.24 to 1.68); EM trees grown in home or live soils were larger than those grown in away or sterile soils.
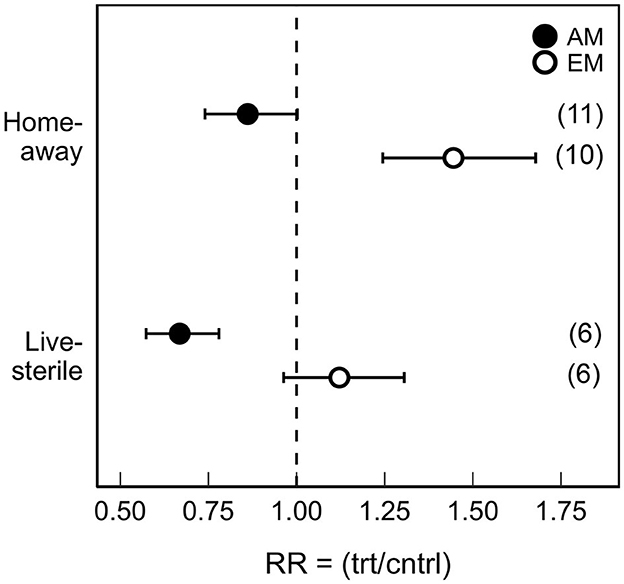
Figure 2. Mean (±95% confidence intervals) effect sizes of plant-soil feedbacks (PSF) on arbuscular (AM) and ectomycorrhizal (EM) tree growth from models including treatment type, mycorrhizal guild, and experiment and species random effects. Our measure of effect size was the response ratio (RR), i.e., the mean plant biomass or height in the treatment (trt) soil divided by the control (cntrl) soil. PSF treatments included plants cultured by conspecific or heterospecific (Home-away) and grown in live or sterile soil (Live-sterile). The number of publications included in the effect size calculations are shown in parentheses.
We found mixed evidence of trait-based relationships with PSFs. In home-away soils, tree species with greater max height (slope = 0.0089, QM = 6.84, P = 0.008, N = 93, Figure 3C) and higher ratio of fine root length to dry mass (i.e., SRL; slope = 0.2243, QM = 7.78, P = 0.005, N = 78, Figure 3D) experienced less negative or more positive PSFs, whereas tree species with greater fine root diameter (FRD; slope = −0.0786, QM = 4.33, P = 0.037, N = 72, Figure 3E) experienced more negative PSFs. No patterns were found for the other aboveground fast-slow traits or shade tolerance (Figure 3). In live-sterile soils, PSF values decreased with increased SLA (slope = −0.0123, QM = 8.51, P = 0.003, N = 47, Figure 4A), LNC (slope = −0.0144, QM = 3.41, P = 0.064, N = 45, Figure 4B), and FRD (slope = −0.602, QM = 3.44, P = 0.064, N = 42, Figure 4E), whereas PSF values increased with increased SRL (slope = 0.1922, QM = 15.85, P = 0.002, N = 42, Figure 4D). Other traits did not show patterns with PSFs in live-sterile soils (Figure 4).
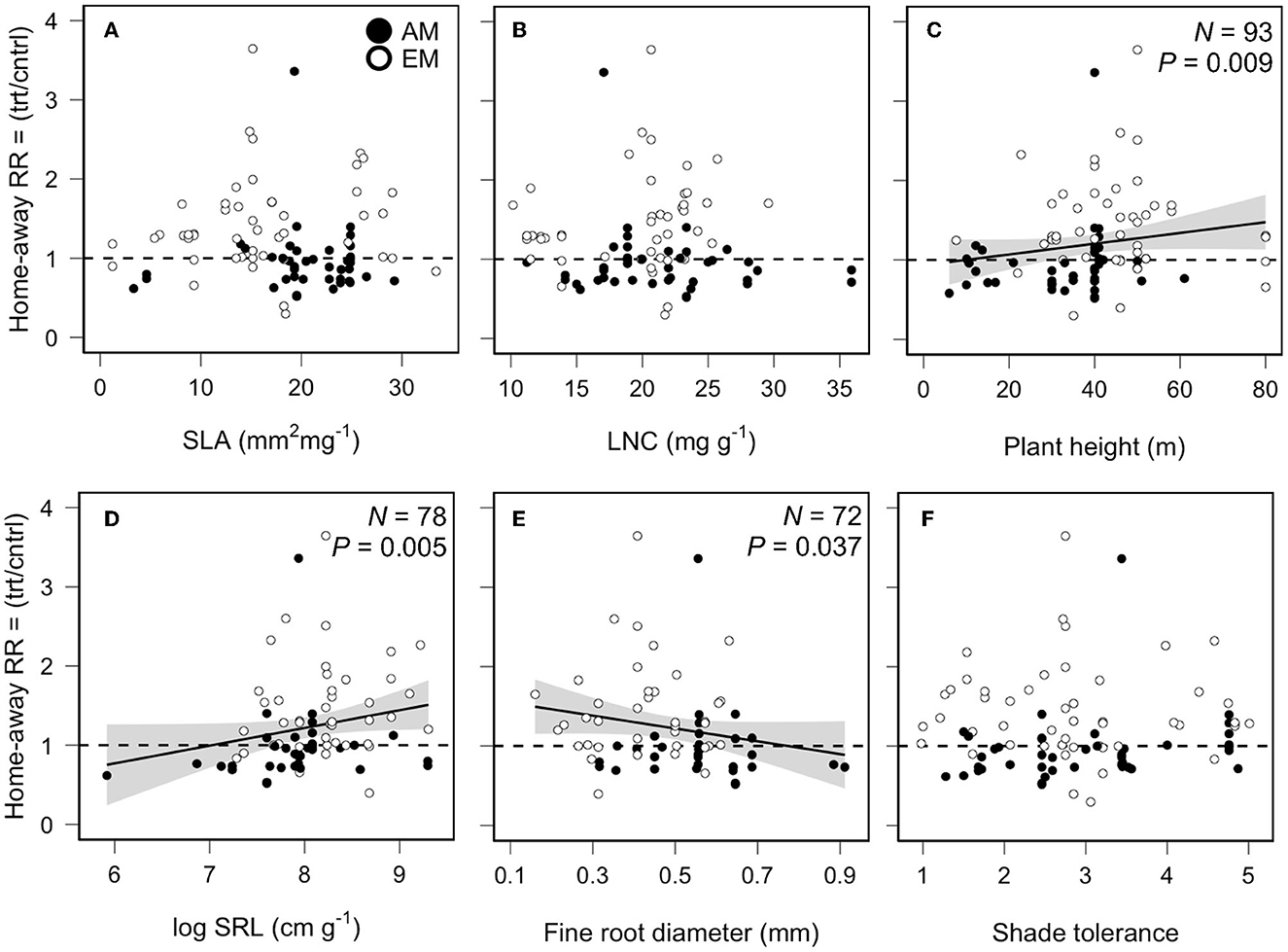
Figure 3. Relationship between plant-soil feedback effects on arbuscular (AM) and ectomycorrhizal (EM) tree growth in home vs. away soil and (A) specific leaf area (SLA), (B) leaf N concentration (LNC), (C) max plant height, (D) specific root length (SRL), (E) fine root diameter (FRD), and (F) species shade tolerance. Our measure of effect size was the response ratio (RR), i.e., the mean plant biomass or height in the treatment (trt) soil divided by the control (cntrl) soil. Solid lines indicate fitted relationships, and the gray shading indicates the 95% confidence intervals based on mixed-effects regression models.
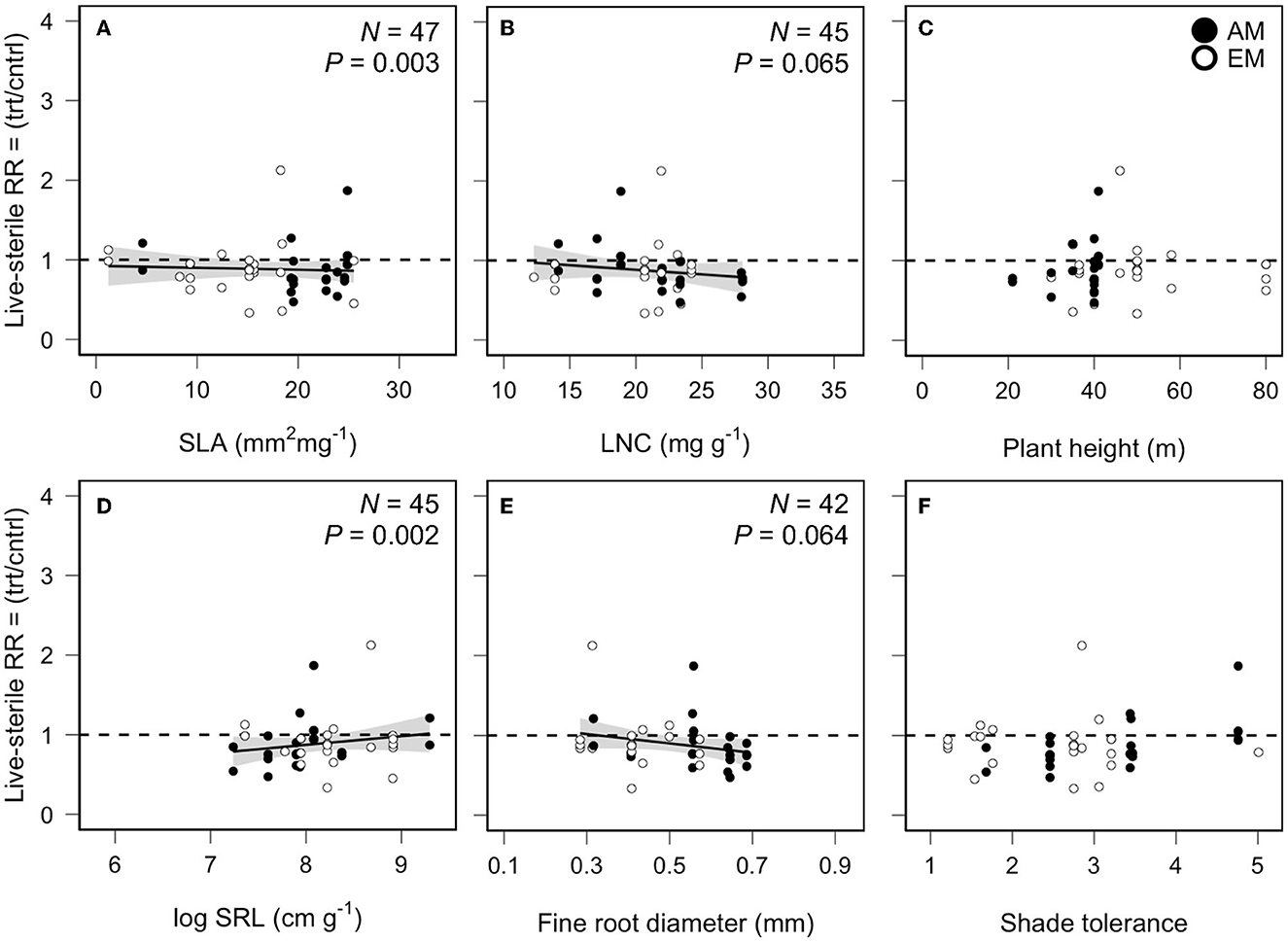
Figure 4. Relationship between plant-soil feedbacks effects on arbuscular (AM) and ectomycorrhizal (EM) tree growth in live vs. sterile soil and (A) specific leaf area (SLA), (B) leaf N concentration (LNC), (C) max plant height, (D) specific root length (SRL), (E) fine root diameter (FRD), and (F) species shade tolerance. Our measure of effect size was the response ratio (RR), i.e., the mean plant biomass or height in the treatment (trt) soil divided by the control (cntrl) soil. Solid lines indicate fitted relationships, and gray shading indicates the 95% confidence intervals based on mixed-effects regression models.
4. Discussion
Despite widespread recognition of the importance of PSFs in determining plant fitness, abundance, and community assembly, the factors that drive PSF variation in forest communities remain largely unknown (Smith-Ramesh and Reynolds, 2017; Beals et al., 2020). We synthesized PSF effects on the growth of 61 tree species native to temperate forests of eastern North America. Our analyses indicate high variability in overall magnitude and direction of PSF effects on tree growth, with the direction and magnitude of PSF effects being highly dependent on the type of PSF treatment and mycorrhizal guild. Both guilds experienced less negative or more positive PSFs in home-away relative to live-sterile soil contrasts, suggesting manipulations of edaphic conditions and/or species-specific soil mutualists and pathogens (rather than all soil biota) have an important effect in governing PSF effects. Consistent with previous research findings on herbaceous plant communities (Kulmatiski et al., 2008; Crawford et al., 2019), AM tree species commonly experienced negative PSF effects on growth. Across mycorrhizal guilds, we found support of linkages between PSFs and a subset of the above- and below-ground traits considered, with species characterized by conservative resource use (i.e., tall, low tissue N, high SRL) experiencing less negative or more positive PSFs. The unexplained variance in our models underscored that unexplored factors likely contributed to PSF effects on tree growth. Below, we describe what unexplored factors may have played a role, summarize key knowledge gaps to address in future research, and provide suggestions for resource managers on incorporating PSFs into assisted migration trials and future reforestation practices. Such research may help improve our understanding of the abiotic and biotic constraints of tree species establishment and aid in the success of climate-adaptive forest management.
4.1. Soil biota are stronger drivers of PSF relative to edaphic factors
PSFs can result from changes to soil biota as well as species-specific changes in abiotic soil properties, including physical soil properties (e.g., soil aggregation; Ehrenfeld et al., 2005) and nutrient availability (i.e., resource partitioning). We found that, across mycorrhizal types, trees experienced less negative or more positive PSFs in home-away than live-sterile soil contrasts (Figure 1). We found this pattern was driven predominately by EM tree species (Figure 2), suggesting that the presence of species-specific soil biota or edaphic conditions in home soils have a beneficial effect on EM tree growth. AM trees experienced more negative PSFs than EM trees in all PSF treatment types (i.e., home-away, live-sterile home, live-sterile away; Figure 2). Specifically, AM trees experienced slower growth in the presence of species-specific soil biota (and edaphic conditions) in home than away soils, as well as in the presence of all soil biota in live (compared to sterile) home and away soils. In contrast, EM trees experienced faster growth in the presence of species-specific soil biota (and edaphic conditions) in home than away soils, as well as in the presence of all soil biota in live (compared to sterile) home and away soils. These patterns suggest that PSF effects were not due to differences in edaphic conditions among home and away soils, but rather driven by species-specific and/or generalist soil biota.
Our findings are consistent with studies that controlled for abiotic soil differences and found support for soil microbes driving plant responses to PSFs in grasslands (Bever, 1994), temperate forests (Bennett et al., 2017), and tropical forests (Mangan et al., 2010). Furthermore, in a meta-analysis of predominately herbaceous plant communities, Crawford et al. (2019) concluded that abiotic factors weakly contributed to PSF effects after accounting for the fraction of soil inoculum used among studies.
4.2. PSFs more consistently linked with below- than above-ground traits
Traits can provide a framework for predicting variation in PSF effects among tree species, especially in diverse forest communities, where co-occurring species may differ strongly in nutrient acquisition strategies and functional traits. We found evidence in support of the relationship between PSF and below-ground traits in the direction consistent with growth-defense trade-off (i.e., fast-growing species tend to suffer from more negative PSFs), but mixed evidence for above-ground traits, depending on PSF treatment (Figures 3, 4). Our results suggest that linkages between plant growth-defense traits and PSFs may occur primarily belowground, where interactions among plant roots and soil biota occur most directly. This pattern is similar to that previously found for herbaceous (Kulmatiski et al., 2017; Semchenko et al., 2018) and woody plant communities (Xi et al., 2021).
4.3. Evidence lacking for linkage between PSFs and tree shade tolerance
The recruitment niche of trees establishing outside their natural range limits will be determined by PSFs as well as key life history characteristics. Among the most important in light-limited forests is the tree species' shade tolerance and similarly, successional stage (Pacala et al., 1996). Shade-intolerant and early-successional tree species tend to invest in traits that maximize growth during their relatively short lifespan (Reich et al., 1998; Grime, 2001), whereas shade-tolerant and later-successional species tend to invest more in defense against natural enemies (Coley and Barone, 1996). Based on linkages between PSF and fast-slow traits, we would expect shade-intolerant and pioneer tree species to be more susceptible to negative PSFs (Kobe and Vriesendorp, 2011). However, we did not find a relationship between PSF strength and species' shade tolerance (Figures 3, 4). This is in contrast with past work from herbaceous plant communities that showed pioneer species experienced more negative or less positive PSFs than late-successional species (Kardol et al., 2006; Bauer et al., 2015; Koziol and Bever, 2015), presumably due to temporal changes in the soil biota (e.g., fungal community) rather than abiotic conditions (e.g., nutrient depletion) (Kardol et al., 2006). Similarly, researchers have documented that seedlings of shade-intolerant tree species may be more susceptible to disease than shade-tolerant species (Augspurger and Kelly, 1984; McCarthy-Neumann and Kobe, 2008). Variation in results among studies on herbaceous and woody plant communities highlights the need for future research on the linkages, if any, between traits that confer survival under low light and those that protect against natural enemies, such as soil pathogens (Carson et al., 2008).
4.4. Potential caveats
Our results may have been influenced by differences among studies in soil sampling methodologies and sample size calculations. Although we were not able to determine the soil sampling practices of every study, our dataset contained studies that used both pooled and unpooled sampling practices. The practice of pooling soil samples collected from multiple conditioning plants, sites, or treatments may alter both the strength and magnitude of PSFs relative to unpooled soil samples by cross-contaminating soil biota among samples. Evidence of the bias introduced by pooling, however, is mixed. Allen et al. (2021) found inconsistent and species-specific effects of sample pooling in herbaceous plant communities. The authors concluded that pooling may be appropriate when broad patterns in PSF effects across multiple species are of interest, as was the case in the present study. However, if species-specific PSFs are of interest (e.g., intraspecific variation in PSFs, Foster et al., 2022), pooling may introduce bias and therefore the practice should be avoided.
A related concern is pseudoreplication, which can occur in PSF studies when researchers pool all conditioned soil within a treatment together, and then draw non-independent sub-samples (i.e., technical replicates) from the pool to inoculate test phase plants (Smith-Ramesh and Reynolds, 2017). Ideally, studies that employ such a practice would be identified and excluded from any meta-analysis. However, in practice it is often difficult to discern true “biological” from “technical” replication, given that such a distinction is predicated on the authors' intended study scope, research questions, and methodology. We recommend that future PSF studies clearly state the intended experimental unit in their methods (Rinella and Reinhart, 2018) and if possible, provide the raw data necessary to repeat their analysis in the Supplemental material.
4.5. Implications for management
Our synthesis shows that PSFs will be important when considering translocation of tree populations and species, especially in timber management where stand productivity is crucial. However, PSFs are currently not considered in standard practices for artificial regeneration (Park and Talbot, 2018). Conventional reforestation approaches to selecting species include matching the species ecological requirements to the site, including soil moisture, nutrients, and dominant vegetation conditions. Presently, assisted migration of tree populations and species follow similar conventional reforestation approaches but forego traditional seed zones such that seed source climate is matched to the future (rather than current) climate of the outplanting site. Below, we identify and suggest two areas where resource managers can better incorporate PSFs into the practices of assisted migration and if appropriate, reforestation more generally.
First, when determining appropriate seed sources for outplanting sites, consider the (dis)similarity of dominant mycorrhizal guilds among the forest communities. Our results suggest that the mycorrhizal guild of the translocated species as well as the community at the outplanting site are important determinants of PSFs. At minimum, assisted migration trials should explicitly test tree species based on the dominant mycorrhizal guild in the seed source and outplanting communities. Better yet, select sites based on the degree of shared fungal diversity among the seed source and outplanting communities. Collaborations with geneticists and molecular biologists to collect and analyze soil DNA can help make this information increasingly available to resource managers (e.g., Argüelles-Moyao and Garibay-Orijel, 2018). This level of specificity may be warranted given that ectomycorrhizal fungal communities can be a mixture of generalist and specialist species. Without identifying mismatches in fungal diversity, there will be a greater risk of losing fungal specialists with assisted migration, and as a result, less effective translocation of tree species (Kranabetter et al., 2012).
Second, during the nursery propagation and outplanting stages, practitioners should carefully consider the benefits and risks of using fungal inoculation for assisted migration. Past research on the effects of soil and root inoculations of mycorrhizal fungi on tree fitness have found patterns to be highly context-dependent, with positive effects on tree growth and survival in P-limited soils, at highly degraded sites, and with inoculum from reference ecosystems rather than commercial sources (Hoeksema et al., 2010; Maltz and Treseder, 2015; Neuenkamp et al., 2019). However, despite the evidence on the importance of PSFs and context-dependent benefits of inoculum, we caution against soil introductions (Hart et al., 2017; Jack et al., 2021). The basis for this caution depends on whether assisted population or species migration is being considered. In the case of assisted population migration on non-degraded soils, inoculation is likely unnecessary. When migration distances are relatively short, beneficial microbes are likely already present, especially many ubiquitous species of arbuscular mycorrhizal fungi. Furthermore, tree species that are broad range climate generalists may be good candidates for assisted migration by seedlings only, using the fungi present at the planting site. For severely altered soils (e.g., mine reclamation) or those that are highly N or P limited, we suggest using locally adapted inoculants, preferably from congeneric plant species (e.g., Dulmer et al., 2014) and avoid commercial inoculants or introduced soil, as they may disrupt coevolved microbe-mediated plant benefits (Jack et al., 2021). For assisted species migration, the careful introduction of inoculum from the seed source may be warranted, especially for specialist tree species with host-specific fungal partners (Keel et al., 2011). However, the movement of soil would likely require permits (e.g., Animal and Plant Health Inspection Service) depending on jurisdiction and risks moving soil pathogens. Future research is needed to evaluate the risks and benefits of such an approach, including the sequencing of the microbial community to identify compositional differences among sites (Jack et al., 2021).
4.6. Future directions
To help define frontiers in knowledge to inform future assisted migration research and trials, we outline several research questions to address in future research on PSFs in forest ecosystems (Table 2). As reflected by the body of research compiled in the present study, PSFs are often examined in isolation of external abiotic and biotic drivers (but see Beals et al., 2020), limiting our ability to predict PSF effects on tree fitness under “real world” conditions. The strength and direction of PSFs, however, will likely depend on global change drivers (e.g., climate change, biodiversity loss) and disturbance regimes, as well as local variation in abiotic and biotic factors (Figure 5). As a result, there are increasing calls for research that examines how abiotic and biotic conditions influence PSFs (van der Putten et al., 2013; Smith-Ramesh and Reynolds, 2017; Whitaker et al., 2017; De Long et al., 2019; Gundale and Kardol, 2021). By identifying the role of biotic and abiotic drivers of PSFs, we will be able to better predict PSF impacts in forest ecosystems and the assisted migration of tree species.
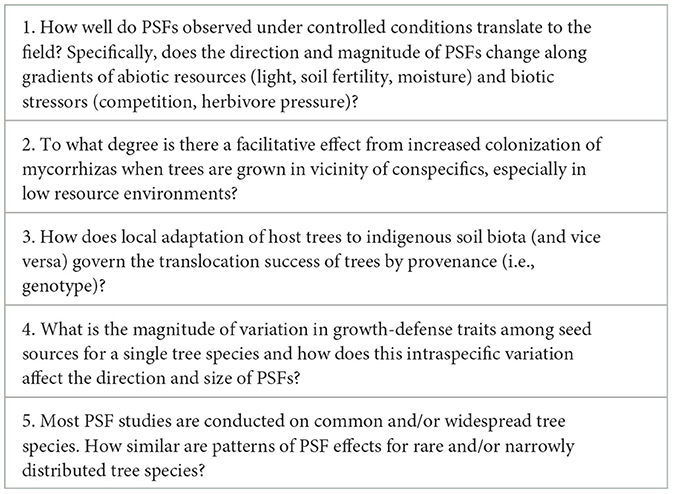
Table 2. List of questions to guide future research on plant-soil feedbacks (PSFs) in forest ecosystems.
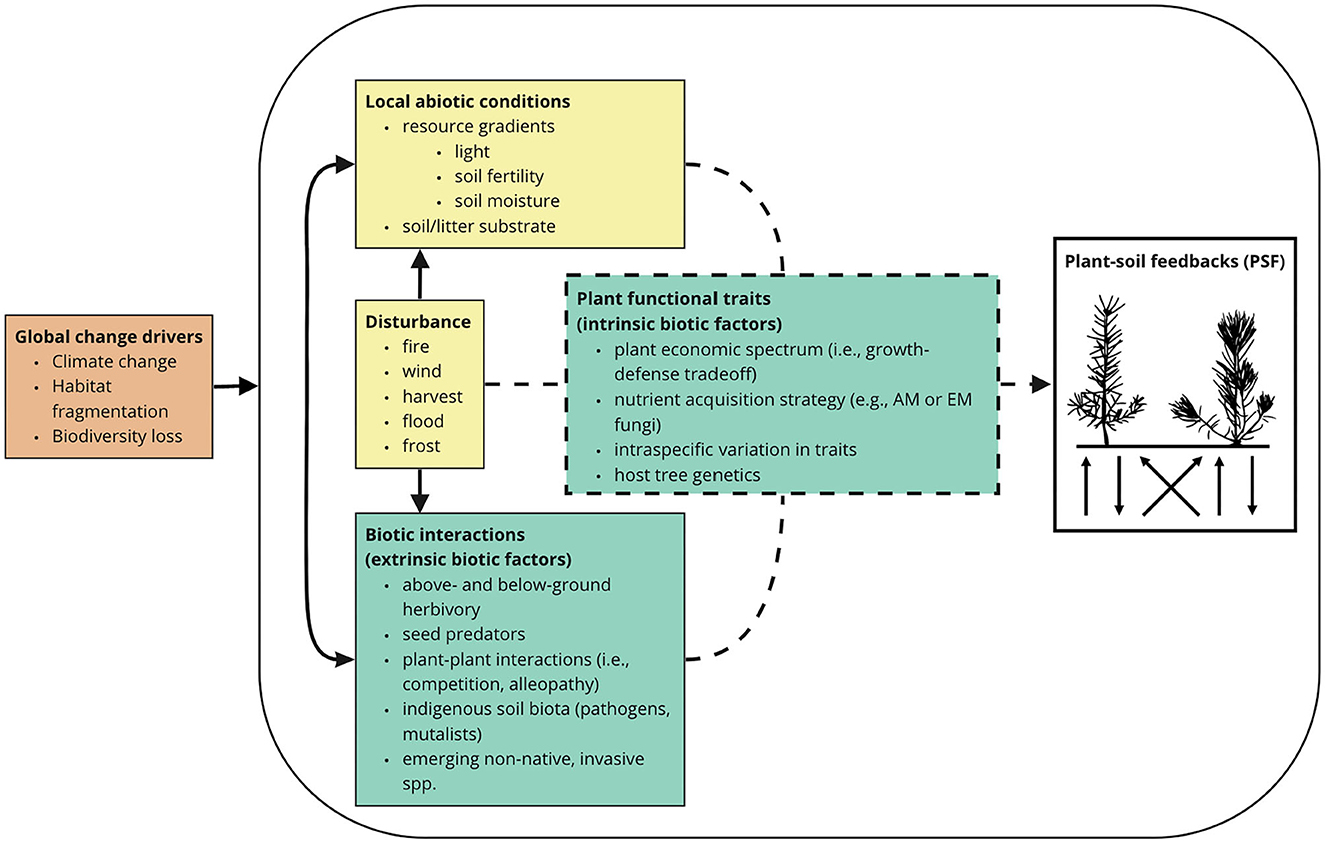
Figure 5. Conceptualized linkages between global change (orange), abiotic (yellow), and biotic (green) drivers of plant-soil feedbacks in tree populations, including the role of plant functional traits in mediating the magnitude and direction of effects (dashed lines).
Two specific knowledge gaps emerged as particularly relevant for temperate forest ecosystems. First, it is unclear how the effects of PSFs on tree fitness vary along resource gradients, including soil fertility and light availability. Given soil fertility can be key driver of AM (e.g., Werner and Kiers, 2015) and EM (Liang et al., 2023) community composition, ecotypes of plant species from nutrient limited soils will be adapted to their local soil and indigenous fungal communities (Johnson et al., 2010). Thus, mutualisms may play a larger role under nutrient-poor than nutrient-rich conditions, and consequently, positive PSFs are likely to dominate. Alternatively, by minimizing abiotic stress experienced by tree seedlings, nutrient-rich conditions may also promote neutral or positive PSFs (Jiang et al., 2020). In terms of light availability, negative PSFs may dominate for shade intolerant tree species under light limited conditions (Hood et al., 2004; Comita et al., 2009; McCarthy-Neumann and Ibáñez, 2013; Jiang et al., 2020). Without adequate carbon resources to offset tissue damage or invest into defense chemicals, trees under shaded conditions may be susceptible to negative PSFs (Shure and Wilson, 1993; Hood et al., 2004; Norghauer et al., 2008). When implementing assisted migration trials, we recommend establishing sites along resource gradients to help reveal important abiotic constraints on tree establishment.
Second, we lack an understanding of how PSFs are influenced by plant-plant interactions, especially as they concern emerging, non-native invasive plant species. Specifically, the identity of neighboring plants may directly determine PSFs through resource competition (Reuter et al., 2021) and also indirectly through effects on the soil biota (Tedersoo et al., 2020; Heklau et al., 2021). Additionally, plant-plant interactions may have a facilitative effect on the establishment of translocated trees by increasing the colonization rate of beneficial mycorrhizal fungi species (Casper and Castelli, 2007) and affecting the presence of pathogenic fungal species (Griffin et al., 2019). These plant-plant interactions can occur both in the overstory and understory plant community, highlighting the potential importance of exotic invasive plant species (e.g., Alliaria petiolata, Microstegium vimineum) in affecting PSFs in tree populations (Van Grunsven et al., 2010). We suggest that assisted migration trials consider planting tree populations in plant communities that differ in the intensity of understory and overstory competition, as well as in communities that vary in the presence or abundance of invasive species.
Finally, the effects of PSFs on tree fitness may also depend on chance, novel associations that are formed between the newly translocated plant and indigenous soil biota. Such novel associations may have temporary or long-lasting effects on tree fitness that contrast sharply with what may be expected based on the species” historical range (e.g., Fruleux et al., 2022).
5. Conclusion
Our meta-analysis showed that the direction and magnitude of PSFs in 61 tree species native to eastern North American vary strongly with plant mycorrhizal guild and growth-defense functional traits. We demonstrate that PSF effects on growth were more negative for tree species that associate with arbuscular vs. ectomycorrhizal fungi, as well as for tree species characterized by acquisitive than conservative resource use traits. Together, these results suggest that plant functional traits have utility in predicting the direction and magnitude of PSFs on tree fitness. Our work also highlighted gaps in our knowledge, such as how patterns in PSFs vary along gradients of abiotic resources and biotic stressors. By better understanding the context-dependent effects of PSFs on tree fitness, we expect improvements in our ability to identify and adopt successful climate-adaptive forestry practices.
Data availability statement
The original contributions presented in the study are included in the article/Supplementary material, further inquiries can be directed to the corresponding author.
Author contributions
TR and JM designed the research. TR conducted the literature search, screened potential papers, performed the meta-analysis, and led the writing of the manuscript and all co-authors contributed substantially to data interpretation and revisions. All co-authors participated in generating the ideas in the paper, actively partook in the writing of the manuscript, and gave final approval for publication. All authors contributed to the article and approved the submitted version.
Funding
This work was supported by the United States Department of Agriculture Forest Service, Northern Research Station, under USDA-FS Agreement 20-JV-11242307-099, and by McIntire-Stennis Project MICL06033.
Acknowledgments
This work is a contribution to the Desired REgeneration through Assisted Migration (DREAM) network. We thank our colleagues in Quebec (USDA-FS Agreement #18-IJ-11242302) for advancing the project internationally. We would also like to thank our collaborator(s) Carrie Pike and Anne Ola for comments on this manuscript. We are grateful to Camryn Brent, Kya Sparks, and Crystal Smith for their assistance with the literature search and data extraction.
Conflict of interest
The authors declare that the research was conducted in the absence of any commercial or financial relationships that could be construed as a potential conflict of interest.
Publisher's note
All claims expressed in this article are solely those of the authors and do not necessarily represent those of their affiliated organizations, or those of the publisher, the editors and the reviewers. Any product that may be evaluated in this article, or claim that may be made by its manufacturer, is not guaranteed or endorsed by the publisher.
Supplementary material
The Supplementary Material for this article can be found online at: https://www.frontiersin.org/articles/10.3389/fevo.2023.1073724/full#supplementary-material
References
Aitken, S. N., and Whitlock, M. C. (2013). Assisted gene flow to facilitate local adaptation to climate change. Annu. Rev. Ecol. Evol. Syst. 44, 367–388. doi: 10.1146/annurev-ecolsys-110512-135747
Allen, W. J., Sapsford, S. J., and Dickie, I. A. (2021). Soil sample pooling generates no consistent inference bias: a meta-analysis of 71 plant-soil feedback experiments. New Phytol. 231, 1308–1315. doi: 10.1111/nph.17455
Argüelles-Moyao, A., and Garibay-Orijel, R. (2018). Ectomycorrhizal fungal communities in high mountain conifer forests in central Mexico and their potential use in the assisted migration of Abies religiosa. Mycorrhiza 28, 509–521. doi: 10.1007/s00572-018-0841-0
Augspurger, C. K., and Kelly, C. K. (1984). Pathogen mortality of tropical tree seedlings: experimental studies of the effects of dispersal distance, seedling density, and light conditions. Oecologia 61, 211–217. doi: 10.1007/BF00396763
Augspurger, C. K., and Wilkinson, H. T. (2007). Host specificity of pathogenic Pythium species: implications for tree species diversity. Biotropica 39, 702–708. doi: 10.1111/j.1744-7429.2007.00326.x
Barto, E. K., and Rillig, M. C. (2010). Does herbivory really suppress mycorrhiza? A meta-analysis: Does herbivory really suppress mycorrhiza? J. Ecol. 98, 745–753. doi: 10.1111/j.1365-201001658.x
Bastin, J-. F., Finegold, Y., Garcia, C., Mollicone, D., Rezende, M., Routh, D., et al. (2019). The global tree restoration potential. Science 365, 76–79. doi: 10.1126/science.aax0848
Bauer, J. T., Mack, K. M. L., and Bever, J. D. (2015). Plant-soil feedbacks as drivers of succession: evidence from remnant and restored tallgrass prairies. Ecosphere 6, 158. doi: 10.1890/ES14-00480.1
Baxendale, C., Orwin, K. H., Poly, F., Pommier, T., and Bardgett, R. D. (2014). Are plant-soil feedback responses explained by plant traits? New Phytol. 204, 408–423. doi: 10.1111/nph.12915
Beals, K. K., Moore, J. A. M., Kivlin, S. N., Bayliss, S. L. J., Lumibao, C. Y., Moorhead, L. C., et al. (2020). Predicting plant-soil feedback in the field: meta-analysis reveals that competition and environmental stress differentially influence PSF. Front. Ecol. E 8, 191. doi: 10.3389/fevo.2020.00191
Bennett, J. A., and Klironomos, J. (2019). Mechanisms of plant–soil feedback: interactions among biotic and abiotic drivers. New Phytol. 222, 91–96. doi: 10.1111/nph.15603
Bennett, J. A., Maherali, H., Reinhart, K. O., Lekberg, Y., Hart, M. M., Klironomos, J., et al. (2017). Plant-soil feedbacks and mycorrhizal type influence temperate forest population dynamics. Science 355, 181–184. doi: 10.1126/science.aai8212
Benning, J. W., and Moeller, D. A. (2021). Plant–soil interactions limit lifetime fitness outside a native plant's geographic range margin. Ecology 102, 3254. doi: 10.1002./ecy.3254
Bever, J. D. (1994). Feedback between plants and their soil communities in an old field community. Ecology 75, 1965–1977. doi: 10.2307/1941601
Bever, J. D. (2003). Soil community feedback and the coexistence of competitors: conceptual frameworks and empirical tests. New Phytol. 157, 465–473. doi: 10.1046/j.1469-8137.2003.00714.x
Bever, J. D., Mangan, S. A., and Alexander, H. M. (2015). Maintenance of plant species diversity by pathogens. Annu. Rev. Ecol. Evol. Syst. 46, 305–325. doi: 10.1146/annurev-ecolsys-112414-054306
Bever, J. D., Westover, K. M., and Antonovics, J. (1997). Incorporating the soil community into plant population dynamics: the utility of the feedback approach. J. Ecol. 85, 561. doi: 10.2307/2960528
Borenstein, M., Hedges, L. V., Higgins, J. P. T., and Rothstein, H. R. (2009). “Meta-regression,” in Introduction to Meta-Analysis (Chichester, UK: John Wiley and Sons, Ltd), 187–203
Callaway, R. M., Thelen, G. C., Rodriguez, A., and Holben, W. E. (2004). Soil biota and exotic plant invasion. Nature 427, 731–733. doi: 10.1038/nature02322
Cardillo, E., Acedo, A., and Abad, E. (2018). Topographic effects on dispersal patterns of Phytophthora cinnamomi at a stand scale in a Spanish heathland. PLOS ONE 13, e0195060. doi: 10.1371/journal.pone.0195060
Cardinaux, A., Hart, S. P., and Alexander, J. M. (2018). Do soil biota influence the outcome of novel interactions between plant competitors? J. Ecol. 106, 1853–1863. doi: 10.1111/1365-2745.13029
Carson, W. P., Anderson, J. T., Leigh, E. G., and Schnitzer, S. A. (2008). “Challenges associated with testing and falsifying the Janzen–Connell Hypothesis: a review and critique,” in Tropical Forest Community Ecology, eds. W. P. Carson and S. A. Schnitzer (Chichester, UK: Wiley-Blackwell), 210–242.
Carteron, A., Parasquive, V., Blanchard, F., Guilbeault-Mayers, X., Turner, B. L., Vellend, M., et al. (2020). Soil abiotic and biotic properties constrain the establishment of a dominant temperate tree into boreal forests. J. Ecol. 108, 931–944. doi: 10.1111/1365-2745.13326
Casper, B. B., and Castelli, J. P. (2007). Evaluating plant–soil feedback together with competition in a serpentine grassland. Ecol. Lett. 10, 394–400. doi: 10.1111/j.1461-0248.2007.01030.x
Charles, K. M., and Stehlik, I. (2020). Assisted species migration and hybridization to conserve cold-adapted plants under climate change. Conserv. Biol., cobi.13583. doi: 10.1111./cobi.13583
Cochran, W. G. (1954). The combination of estimates from different experiments. Biometrics 10, 101. doi: 10.2307/3001666
Coley, P. D., and Barone, J. A. (1996). Herbivory and plant defenses in tropical forests. Annu. Rev. Ecol. Syst. 27, 305–335. doi: 10.1146/annurev.ecolsys.27.1.305
Coley, P. D., Bryant, J. P., and Chapin, F. S. (1985). Resource availability and plant antiherbivore defense. Science 230, 895–899. doi: 10.1126/science.230.4728.895
Comas, L. H., Callahan, H. S., and Midford, P. E. (2014). Patterns in root traits of woody species hosting arbuscular and ectomycorrhizas: implications for the evolution of belowground strategies. Ecol. E4, 2979–2990. doi: 10.1002/ece3.1147
Comita, L. S., Uriarte, M., Thompson, J., Jonckheere, I., Canham, C. D., Zimmerman, J. K., et al. (2009). Abiotic and biotic drivers of seedling survival in a hurricane-impacted tropical forest. J. Ecol. 97, 1346–1359. doi: 10.1111/j.1365-200901551.x
Connell, J. H. (1971). “On the role of natural enemies in preventing competitive exclusion in some marine mammals and in rain forest trees,” in Dynamics of Populations, eds. P. J. Boer and G. R. Gradwell (Wageningen, Netherlands: Centre for Agricultural Publishing and Documentation), 298–310.
Corrales, A., Mangan, S. A., Turner, B. L., and Dalling, J. W. (2016). An ectomycorrhizal nitrogen economy facilitates monodominance in a neotropical forest. Ecol. Lett. 19, 383–392. doi: 10.1111/ele.12570
Cortois, R., Schröder-Georgi, T., Weigelt, A., Putten, W. H., and De Deyn, G. B. (2016). Plant–soil feedbacks: role of plant functional group and plant traits. J. Ecol. 104, 1608–1617. doi: 10.1111/1365-2745.12643
Crawford, K. M., Bauer, J. T., Comita, L. S., Eppinga, M. B., Johnson, D. J., Mangan, S. A., et al. (2019). When and where plant-soil feedback may promote plant coexistence: a meta-analysis. Ecol. Lett. 22, 1274–1284. doi: 10.1111/ele.13278
Davis, M. B., and Shaw, R. G. (2001). Range shifts and adaptive responses to Quaternary climate change. Science 292, 673–679. doi: 10.1126/science.292.5517.673
De Long Fry, J. R., Veen, E. L., and Kardol, P. (2019). Why are plant–soil feedbacks so unpredictable, and what to do about it? Funct. Ecol. 33, 118–128. doi: 10.1111/1365-2435.13232
de Vries Manning, F. T., Tallowin, P., Mortimer, J. R. B., Pilgrim, S. R., Harrison, E. S. K. A., et al. (2012). Abiotic drivers and plant traits explain landscape-scale patterns in soil microbial communities. Ecol. Lett. 15, 1230–1239. doi: 10.1111/j.1461-0248.2012.01844.x
Duchesne, L. C., Peterson, R. L., and Ellis, B. E. (1989). The time-course of disease suppression and antibiosis by the ectomycorrhizal fungus Paxillus Involutus. New Phytol. 111, 693–698. doi: 10.1111/j.1469-8137.1989.tb02364.x
Dulmer, K. M., LeDuc, S. D., and Horton, T. R. (2014). Ectomycorrhizal inoculum potential of northeastern US forest soils for American chestnut restoration: results from field and laboratory bioassays. Mycorrhiza 24, 65–74. doi: 10.1007/s00572-013-0514-y
Ehrenfeld, J. G., Ravit, B., and Elgersma, K. (2005). Feedback in the plant-soil system. Annu. Rev. Environ. Resour. 30, 75–115. doi: 10.1146/annurev.energy.30.050504.144212
Etterson, J. R., Cornett, M. W., White, M. A., and Kavajecz, L. C. (2020). Assisted migration across fixed seed zones detects adaptation lags in two major North American tree species. Ecol. Appl. 30, e02092. doi: 10.1002/eap.2092
FAO and UNEP (2020). The State of the World's Forests 2020: Forests, Biodiversity and People. Rome, Italy: FAO and UNEPdoi: 10.4060./ca8642en
Fei, S., Desprez, J. M., Potter, K. M., Jo, I., Knott, J. A., Oswalt, C. M., et al. (2017). Divergence of species responses to climate change. Sci. Adv. 3, e1603055. doi: 10.1126/sciadv.1603055
Foster, B. S., Haile, B. B., Campnell, J. T., Canam, T., Gallagher, M. J., and Meiners, S. J. (2022). Plant performance responds to intraspecific variation in soil inocula from individual Solidago clones. Plant Ecol. 223, 201–212. doi: 10.1007/s11258-021-01198-2
Fruleux, A., Duclercq, J., Dubois, F., and Decocq, G. (2022). First report of ectomycorrhizae in Prunus serotina in the exotic range. Plant Soil 484, 171–181. doi: 10.1007/s11104-022-05780-z
Griffin, E. A., Harrison, J. G., McCormick, M. K., Burghardt, K. T., and Parker, J. D. (2019). Tree diversity reduces fungal endophyte richness and diversity in a large-scale temperate forest experiment. Diversity 11, 234. doi: 10.3390/d11120234
Grime, J. P. (2001). Plant Strategies, Vegetation Processes, and Ecosystem Properties. 2nd ed. Chichester, UK: John Wiley and Sons, Ltd.
Gundale, M., and Kardol, P. (2021). Multi-dimensionality as a path forward in plant-soil feedback research. J. Ecol., 1365-2745, 13679. doi: 10.1111/1365-2745.13679
Hart, M. M., Antunes, P. M., Chaudhary, V. B., and Abbott, L. K. (2017). Fungal inoculants in the field: Is the reward greater than the risk? Funct. Ecol. 32, 126–135. doi: 10.1111/1365-2435.12976
Hedges, L. V., Gurevitch, J., and Curtis, P. S. (1999). The meta-analysis of response ratios in experimental ecology. Ecology 80, 1150–1156. doi: 10.1890/0012-9658(1999)080(1150:TMAORR)2.0.CO;2
Heklau, H., Schindler, N., Buscot, F., Eisenhauer, N., Ferlian, O., Prada Salcedo, L. D., et al. (2021). Mixing tree species associated with arbuscular or ectotrophic mycorrhizae reveals dual mycorrhization and interactive effects on the fungal partners. Ecol. E. 11, 5424–5440. doi: 10.1002/ece3.7437
Hersh, M. H., Vilgalys, R., and Clark, J. S. (2012). Evaluating the impacts of multiple generalist fungal pathogens on temperate tree seedling survival. Ecology 93, 511–520. doi: 10.1890/11-0598.1
Hoegh-Guldberg, O., Hughes, L., McIntyre, S., Lindenmayer, D. B., Parmesan, C., Possingham, H. P., et al. (2008). Assisted colonization and rapid climate change. Science 321, 345–346. doi: 10.1126/science.1157897
Hoeksema, J. D., Chaudhary, V. B., Gehring, C. A., Johnson, N. C., Karst, J., Koide, R. T., et al. (2010). A meta-analysis of context-dependency in plant response to inoculation with mycorrhizal fungi. Ecol. Lett. 13, 394–407. doi: 10.1111/j.1461-0248.2009.01430.x
Hood, L. A., Swaine, M. D., and Mason, P. A. (2004). The influence of spatial patterns of damping-off disease and arbuscular mycorrhizal colonization on tree seedling establishment in Ghanaian tropical forest soil. J. Ecol. 92, 816–823. doi: 10.1111/j.0022-0477.2004.00917.x
Iversen, C., McCormack, M., Baer, J., Powell, A., Chen, W., Collins, C., et al. (2021). Fine-Root Ecology Database (FRED): A Global Collection of Root Trait Data with Coincident Site, Vegetation, Edaphic, and Climatic Data, Version 3. Oak Ridge National Laboratory, TES SFA, U.S. Department of Energy, Oak Ridge, Tennessee, U.S.A. doi: 10.25581/ORNLSFA.014/1459186
Jack, C. N., Petipas, R. H., Cheeke, T. E., Rowland, J. L., and Friesen, M. L. (2021). Microbial inoculants: silver bullet or microbial Jurassic Park? Trends Microbiol. 29, 299–308. doi: 10.1016/j.tim.11006
Jevon, F. V., Record, S., Grady, J., Lang, A. K., Orwig, D. A., Ayres, M. P., et al. (2020). Seedling survival declines with increasing conspecific density in a common temperate tree. Ecosphere 11, e03292. doi: 10.1002/ecs2.3292
Jiang, F., Zhu, K., Cadotte, M. W., and Jin, G. (2020). Tree mycorrhizal type mediates the strength of negative density dependence in temperate forests. J. Ecol. 108, 2601–2610. doi: 10.1111/1365-2745.13413
Johnson, N. C., Wilson, G. W. T., Bowker, M. A., Wilson, J. A., and Miller, R. M. (2010). Resource limitation is a driver of local adaptation in mycorrhizal symbioses. Proc. Natl. Acad. Sci. 107, 2093–2098. doi: 10.1073/pnas.0906710107
Kardol, P., Martijn Bezemer, T., and van der Putten, W. H. (2006). Temporal variation in plant-soil feedback controls succession: plant-soil feedback controls succession. Ecol. Lett. 9, 1080–1088. doi: 10.1111/j.1461-0248.2006.00953.x
Kattge, J., Bönisch, G., Díaz, S., Lavorel, S., Prentice, I. C., Leadley, P., et al. (2020). TRY plant trait database—Eenhanced coverage and open access. Glob. Change Biol. 26, 119–188. doi: 10.1111/gcb.14904
Keel, B. G., Zettler, L. W., and Kaplin, B. A. (2011). Seed germination of Habenaria repens (Orchidaceae) in situ beyond its range, and its potential for assisted migration imposed by climate change. Castanea 76, 43–54. doi: 10.2179/09-054.1
Klironomos, J. N. (2002). Feedback with soil biota contributes to plant rarity and invasiveness in communities. Nature 417, 67–70. doi: 10.1038/417067a
Kobe, R. K., and Vriesendorp, C. F. (2011). Conspecific density dependence in seedlings varies with species shade tolerance in a wet tropical forest: shade and density-dependent mortality. Ecol. Lett. 14, 503–510. doi: 10.1111/j.1461-0248.2011.01612.x
Konstantopoulos, S. (2011). Fixed effects and variance components estimation in three-level meta-analysis. Res. Synth. Methods 2, 61–76. doi: 10.1002/jrsm.35
Koziol, L., and Bever, J. D. (2015). Mycorrhizal response trades off with plant growth rate and increases with plant successional status. Ecology 96, 1768–1774. doi: 10.1890/14-2208.1
Kranabetter, J. M., Stoehr, M. U., and O'Neill, G. A. (2012). Divergence in ectomycorrhizal communities with foreign Douglas-fir populations and implications for assisted migration. Ecol. Appl. 22, 550–560. doi: 10.1890/11-1514.1
Kulmatiski, A., Beard, K. H., Norton, J. M., Heavilin, J. E., Forero, L. E., Grenzer, J., et al. (2017). Live long and prosper: plant–soil feedback, lifespan, and landscape abundance covary. Ecology 98, 3063–3073. doi: 10.1002/ecy.2011
Kulmatiski, A., Beard, K. H., Stevens, J. R., and Cobbold, S. M. (2008). Plant–soil feedbacks: a meta-analytical review. Ecol. Lett. 11, 980–992. doi: 10.1111/j.1461-0248.2008.01209.x
Laliberté, E., Lambers, H., Burgess, T. I., and Wright, S. J. (2015). Phosphorus limitation, soil-borne pathogens and the coexistence of plant species in hyperdiverse forests and shrublands. New Phytol. 206, 507–521. doi: 10.1111/nph.13203
Lance, A. C., Carrino-Kyker, S. R., Burke, D. J., and Burns, J. H. (2020). Individual plant-soil feedback effects influence tree growth and rhizosphere fungal communities in a temperate forest restoration experiment. Front. Ecol. E 7, 500. doi: 10.3389/fevo.2019.00500
Lekberg, Y., Bever, J. D., Bunn, R. A., Callaway, R. M., Hart, M. M., Kivlin, S. N., et al. (2018). Relative importance of competition and plant–soil feedback, their synergy, context dependency and implications for coexistence. Ecol. Lett. 21, 1268–1281. doi: 10.1111/ele.13093
Lemmermeyer, S., Lörcher, L., van Kleunen, M., and Dawson, W. (2015). Testing the plant growth-defense hypothesis belowground: do faster-growing herbaceous plant species suffer more negative effects from soil biota than slower-growing ones? Am. Nat. 186, 264–271. doi: 10.1086/682005
Levine, J. M., Adler, P. B., and Yelenik, S. G. (2004). A meta-analysis of biotic resistance to exotic plant invasions: biotic resistance to plant invasion. Ecol. Lett. 7, 975–989. doi: 10.1111/j.1461-0248.2004.00657.x
Liang, S., Arraiano-Castilho, R., Neuenkamp, L., Li, H., Bai, Z., Zhang, M., et al. (2023). Rhizosphere fungal community assembly varied across functional guilds in a temperate forest. Ecol. Process. 12, 6. doi: 10.1186/s13717-023-00417-0
Light, R. J., and Pillemer, D. B. (1984). Summing Up: The Science of Reviewing Research. Cambridge, MA, USA: Harvard University Press.
Lihui, M., Xiaoli, L., Jie, C., Youke, W., and Jingui, Y. (2021). Effects of slope aspect and rainfall on belowground deep fine root traits and aboveground tree height. Front. Plant Sci. 12, 684468. doi: 10.3389/fpls.2021.684468
Loarie, S. R., Duffy, P. B., Hamilton, H., Asner, G. P., Field, C. B., Ackerly, D. D., et al. (2009). The velocity of climate change. Nature 462, 1052–1055. doi: 10.1038/nature08649
Maltz, M. R., and Treseder, K. K. (2015). Sources of inocula influence mycorrhizal colonization of plants in restoration projects: a meta-analysis: mycorrhizal inoculation in restoration. Restor. Ecol. 23, 625–634. doi: 10.1111/rec.12231
Mangan, S. A., Schnitzer, S. A., Herre, E. A., Mack, K. M. L., Valencia, M. C., Sanchez, E. I., et al. (2010). Negative plant–soil feedback predicts tree-species relative abundance in a tropical forest. Nature 466, 752–755. doi: 10.1038/nature09273
Maron, J. L., Marler, M., Klironomos, J. N., and Cleveland, C. C. (2011). Soil fungal pathogens and the relationship between plant diversity and productivity: Soil pathogens, productivity and invasibility. Ecol. Lett. 14, 36–41. doi: 10.1111/j.1461-0248.2010.01547.x
McCarthy-Neumann, S., and Ibáñez, I. (2013). Plant-soil feedback links negative distance dependence and light gradient partitioning during seedling establishment. Ecology 94, 780–786. doi: 10.1890/12-1338.1
McCarthy-Neumann, S., and Kobe, R. K. (2008). Tolerance of soil pathogens co-varies with shade tolerance across species of tropical tree seedlings. Ecology 89, 1883–1892. doi: 10.1890/07-0211.1
McCarthy-Neumann, S., and Kobe, R. K. (2019). Site soil-fertility and light availability influence plant-soil feedback. Front. Ecol. E7, 1–10. doi: 10.3389/fevo.2019.00383
Neuenkamp, L., Prober, S. M., Price, J. N., Zobel, M., and Standish, R. J. (2019). Benefits of mycorrhizal inoculation to ecological restoration depend on plant functional type, restoration context and time. Fungal Ecol. 40, 140–149. doi: 10.1016/j.funeco.05004
Niinemets, Ü., and Valladares, F. (2006). Tolerance to shade, drought, and waterlogging of temperate northern hemisphere trees and shrubs. Ecol. Monogr. 76, 521–547. doi: 10.1890/0012-9615(2006)076(0521:TTSDAW)2.0.CO;2
Norghauer, J. M., Malcolm, J. R., and Zimmerman, B. L. (2008). Canopy cover mediates interactions between a specialist caterpillar and seedlings of a neotropical tree. J. Ecol. 96, 103–113. doi: 10.1111/j.1365-200701325.x
Orwin, K. H., Buckland, S. M., Johnson, D., Turner, B. L., Smart, S., Oakley, S., et al. (2010). Linkages of plant traits to soil properties and the functioning of temperate grassland. J. Ecol. 98, 1074–1083. doi: 10.1111/j.1365-2010.01679.x
Pacala, S. W., Canham, C. D., Saponara, J., Silander, J. A., Kobe, R. K., Ribbens, E., et al. (1996). Forest models defined by field measurements: estimation, error analysis and dynamics. Ecol. Monogr. 66, 1–43. doi: 10.2307/2963479
Packer, A., and Clay, K. (2000). Soil pathogens and spatial patterns of seedling mortality in a temperate tree. Nature 404, 278–281. doi: 10.1038/35005072
Park, A., and Talbot, C. (2018). Information underload: ecological complexity, incomplete knowledge, and data deficits create challenges for the assisted migration of forest trees. BioScience 68, 251–263. doi: 10.1093/biosci/biy001
Parmesan, C., and Yohe, G. (2003). A globally coherent fingerprint of climate change impacts across natural systems. Nature 421, 37–42. doi: 10.1038/nature01286.
Pick, J. L., Nakagawa, S., and Noble, D. W. A. (2019). Reproducible, flexible and high-throughput data extraction from primary literature: The metaDigitise R package. Methods Ecol. E. 10, 426–431. doi: 10.1111/2041-210X.13118
Prasad, A., Pedlar, J., Peters, M., McKenney, D., Iverson, L., Matthews, S., et al. (2020). Combining US and Canadian forest inventories to assess habitat suitability and migration potential of 25 tree species under climate change. Divers. Distrib. 26, 1142–1159. doi: 10.1111/ddi.13078
R Core Team (2022). R: A language and environment for statistical computing. Available online at: https://www.R-project.org/ (accessed March 20, 2023).
Reich, P. B. (2014). The world-wide “fast–slow” plant economics spectrum: a traits manifesto. J. Ecol. 102, 275–301. doi: 10.1111/1365-2745.12211
Reich, P. B., Tjoelker, M. G., Walters, M. B., Vanderklein, D. W., and Buschena, C. (1998). Close association of RGR, leaf and root morphology, seed mass and shade tolerance in seedlings of nine boreal tree species grown in high and low light: RGR and tissue morphology in boreal trees. Funct. Ecol. 12, 327–338. doi: 10.1046/j.1365-199800208.x
Reinhart, K. O., Bauer, J. T. McCarthy-Neumann, S., MacDougall, A. S., Hierro, J. L., Chiuffo, M. C., et al. (2021). Globally, plant-soil feedbacks are weak predictors of plant abundance. Ecol. E. 11, 1756–1768. doi: 10.1002/ece3.7167
Reinhart, K. O., Royo, A. A., Van der Putten, W. H., and Clay, K. (2005). Soil feedback and pathogen activity in Prunus serotina throughout its native range. J. Ecol. 93, 890–898. doi: 10.1111/j.1365-200501028.x
Reuter, R., Ferlian, O., Tarkka, M., Eisenhauer, N., Pritsch, K., Simon, J., et al. (2021). Tree species rather than type of mycorrhizal association drive inorganic and organic nitrogen acquisition in tree–tree interactions. Tree Physiol., tpab059. doi: 10.1093./treephys/tpab059
Rinella, M. J., and Reinhart, K. O. (2018). Toward more robust plant-soil feedback research. Ecology 99, 550–556. doi: 10.1002/ecy.2146
Rogers, B. M., Jantz, P., and Goetz, S. J. (2017). Vulnerability of eastern US tree species to climate change. Glob. Change Biol. 23, 3302–3320. doi: 10.1111/gcb.13585
Semchenko, M., Leff, J. W., Lozano, Y. M., Saar, S., Davison, J., Wilkinson, A., et al. (2018). Fungal diversity regulates plant-soil feedbacks in temperate grassland. Sci. Adv. 4, eaau4578. doi: 10.1126./sciadv.aau4578
Shure, D. J., and Wilson, L. A. (1993). Patch-Size Effects on Plant Phenolics in Successional Openings of the Southern Appalachians. Ecology 74, 55–67. doi: 10.2307/1939501
Smith-Ramesh, L. M., and Reynolds, H. L. (2017). The next frontier of plant–soil feedback research: unraveling context dependence across biotic and abiotic gradients. J. Veg. Sci. 28, 484–494. doi: 10.1111/jvs.12519
Suding, K. N., Stanley Harpole, W., Fukami, T., Kulmatiski, A., MacDougall, A. S., Stein, C., et al. (2013). Consequences of plant-soil feedbacks in invasion. J. Ecol. 101, 298–308. doi: 10.1111/1365-2745.12057
Tedersoo, L., Bahram, M., and Zobel, M. (2020). How mycorrhizal associations drive plant population and community biology. Science 367, eaba1223. doi: 10.1126./science.aba1223
Teste, F. P., Kardol, P., Turner, B. L., Wardle, D. A., Zemunik, G., Renton, M., et al. (2017). Plant-soil feedback and the maintenance of diversity in Mediterranean-climate shrublands. Science 355, 173–176. doi: 10.1126/science.aai8291
Thakur, M. P., van der Putten, W. H., Wilschut, R. A., Veen, G. F., Kardol, P., van Ruijven, J., et al. (2021). Plant–soil feedbacks and temporal dynamics of plant diversity–productivity relationships. Trends Ecol. Evol. 36, 651–661. doi: 10.1016/j.tree.03011
Tobner, C. M., Paquette, A., and Messier, C. (2013). Interspecific coordination and intraspecific plasticity of fine root traits in North American temperate tree species. Front. Plant Sci. 4, 242. doi: 10.3389./fpls.2013.00242
Treseder, K. K. (2004). A meta-analysis of mycorrhizal responses to nitrogen, phosphorus, and atmospheric CO2 in field studies. New Phytol. 164, 347–355. doi: 10.1111/j.1469-8137.2004.01159.x
van der Putten, W. H. (2012). Climate change, aboveground-belowground interactions, and species' range shifts. Annu. Rev. Ecol. Evol. Syst. 43, 365–383. doi: 10.1146/annurev-ecolsys-110411-160423
van der Putten, W. H., Bardgett, R. D., Bever, J. D., Bezemer, T. M., Casper, B. B., Fukami, T., et al. (2013). Plant–soil feedbacks: the past, the present and future challenges. J. Ecol. 101, 265–276. doi: 10.1111/1365-2745.12054
Van Grunsven, R. H. A., Van Der Putten, W. H., Martijn Bezemer, T., Berendse, F., and Veenendaal, E. M. (2010). Plant–soil interactions in the expansion and native range of a poleward shifting plant species. Glob. Change Biol. 16, 380–385. doi: 10.1111/j.1365-200901996.x
Viechtbauer, W. (2010). Conducting meta-analyses in R with the metafor package. J. Stat. Softw. 36, 1–48. doi: 10.18637/jss.v036.i03
Ward, D. (2021). Shade affects fine-root morphology in range-encroaching eastern redcedars (Juniperus virginiana) more than competition, soil fertility and pH. Pedobiologia 84. doi: 10.1016./j.pedobi.2021.150708
Wardle, D. A. (2002). Communities and Ecosystems: Linking the Aboveground and Belowground Components. Princeton and Oxford: Princeton University Press.
Weemstra, M., Zambrano, J., Allen, D., and Umaña, M. N. (2021). Tree growth increases through opposing above-ground and below-ground resource strategies. J. Ecol. 109, 3502–3512. doi: 10.1111/1365-2745.13729
Werner, G. D. A., and Kiers, E. T. (2015). Partner selection in the mycorrhizal mutualism. New Phytol. 205, 1437–1442. doi: 10.1111/nph.13113
Whitaker, B. K., Bauer, J. T., Bever, J. D., and Clay, K. (2017). Negative plant-phyllosphere feedbacks in native Asteraceae hosts—A novel extension of the plant-soil feedback framework. Ecol. Lett. 20, 1064–1073. doi: 10.1111/ele.12805
Wright, I. J., Reich, P. B., Westoby, M., Ackerly, D. D., Baruch, Z., Bongers, F., et al. (2004). The worldwide leaf economics spectrum. Nature 428, 821–827. doi: 10.1038/nature02403
Wubs, E. R. J., van der Putten, W. H., Bosch, M., and Bezemer, T. M. (2016). Soil inoculation steers restoration of terrestrial ecosystems. Nat. Plants 2, 16107. doi: 10.1038/nplants.2016.107
Xi, N., Adler, P. B., Chen, D., Wu, H., Catford, J. A., Bodegom, P. M., et al. (2021). Relationships between plant–soil feedbacks and functional traits. J. Ecol. 109, 3411–3423. doi: 10.1111/1365-2745.13731
Keywords: assisted migration, belowground interactions, meta-analysis, mycorrhizal fungi, plant functional traits (PFTs), range expansion, soil microbial community, tree growth
Citation: Refsland TK, Adams B, Bronson D, Kern CC, Marquardt P, McGraw AM, Royo AA and Miesel JR (2023) Synthesis of plant-soil feedback effects on eastern North American tree species: implications for climate-adaptive forestry. Front. Ecol. Evol. 11:1073724. doi: 10.3389/fevo.2023.1073724
Received: 18 October 2022; Accepted: 05 May 2023;
Published: 19 May 2023.
Edited by:
Mauricio Lima, Pontificia Universidad Católica de Chile, ChileReviewed by:
Simone Mereu, National Research Council (CNR), ItalyKurt Reinhart, Livestock and Range Research Laboratory, Agricultural Research Service (USDA), United States
Copyright © 2023 Refsland, Adams, Bronson, Kern, Marquardt, McGraw, Royo and Miesel. This is an open-access article distributed under the terms of the Creative Commons Attribution License (CC BY). The use, distribution or reproduction in other forums is permitted, provided the original author(s) and the copyright owner(s) are credited and that the original publication in this journal is cited, in accordance with accepted academic practice. No use, distribution or reproduction is permitted which does not comply with these terms.
*Correspondence: Tyler K. Refsland, cmVmc2xhbmRAbXN1LmVkdQ==; dHlsZXIucmVmc2xhbmRAZ21haWwuY29t