- 1Department of Geographical and Sustainability Sciences, University of Iowa, Iowa City, IA, United States
- 2BIOME Lab, Department of Biological, Geological and Environmental Sciences, Alma Mater Studiorum University of Bologna, Bologna, Italy
- 3LifeWatch Italy, Lecce, Italy
- 4Research Unit of Biodiversity (CSIC/UO/PA), University of Oviedo, Mieres, Spain
Introduction: Whether the distribution and assembly of plant species are adapted to current climates or legacy effects poses a problem for their conservation during ongoing climate change. The alpine regions of southern and central Europe are compared to those of the western United States and Canada because they differ in their geographies and histories.
Methods: Individual-based simulation experiments disentangled the role of geography in species adaptations and legacy effects in four combinations: approximations of observed alpine geographies vs. regular lattices with the same number of regions (realistic and null representations), and virtual species with responses to either climatic or simple spatial gradients (adaptations or legacy effects). Additionally, dispersal distances were varied using five Gaussian kernels. Because the similarity of pairs of regional species pools indicated the processes of assembly at extensive spatiotemporal scales and is a measure of beta diversity, this output of the simulations was correlated to observed similarity for Europe and North America.
Results: In North America, correlations were highest for simulations with approximated geography and location-adapted species; those in Europe had their highest correlation with the lattice pattern and climate-adapted species. Only SACEU correlations were sensitive to dispersal limitation.
Discussion: The southern and central European alpine areas are more isolated and with more distinct climates to which species are adapted. In the western United States and Canada, less isolation and more mixing of species from refugia has caused location to mask climate adaptation. Among continents, the balance of explanatory factors for the assembly of regional species pools will vary with their unique historical biogeographies, with isolation lessening disequilibria.
1. Introduction
The degree to which patterns of species distribution and diversity are coupled with environmental drivers, versus being in geographical or historical disequilibria, will affect their response to ongoing climatic change (Pinto-Ledezma et al., 2018; Marta et al., 2019; Storch et al., 2022). Disentangling the relative importance of adaptations to the environment versus historical legacies and spatial patterns is a continuing project in ecology (Ricklefs et al., 1999; Whittaker et al., 2001; Van Meerbeek et al., 2021) that is motivated by the need to anticipate the effects of ongoing climate change (e.g., Graae et al., 2018). To explore how geographical and historical legacies or adaptations to the environment could determine patterns of beta diversity, we developed simulations of the assembly of alpine vegetation in the western United States and Canada and southern and central Europe. These regions differ in the spatial pattern created by geological forces over the past 100 million years.
Alpine habitats provide a useful case for elucidating how diversity is structured (e.g., Testolin et al., 2020, 2021) because their spatial and climatic limits are relatively distinct. If species distributions and climates are tightly coupled, current associations of species with climate would indicate major losses of diversity in alpine regions over the next century (e.g., Barredo et al., 2020; Malanson et al., 2022b); if not, their role as sentinels becomes problematic (Malanson et al., 2019). Alpine vegetation has distinct climatic limits with restricted spatial expression (Körner, 2003), and the spatial response to climate change, involving refugia that have varied in extent and connection to other habitats (e.g., Birks and Willis, 2008) has differed globally (Testolin et al., 2020, 2021). Thus, the relative importance of environmental adaptations, geographical patterns, and historical legacies may differ among alpine plant communities between regions. We compare an ecological pattern, beta diversity, between the western United States and Canada (WUSAC) and southern and central Europe (SACEU) because of their geographical and historical differences (these areas are biogeographically distinct from regions of eastern North America and northern Europe, respectively).
For alpine vegetation in WUSAC, dissimilarities in composition have been linked to spatial differentiation Taylor (1977) and Hadley (1987) but differences in climate may play an important role (Malanson et al., 2015). In Europe, Lenoir et al. (2010) identified different glacial histories as the cause of different patterns of diversities between the Alps and the Scandes, and Jiménez-Alfaro et al. (2021) reported that area and isolation accounted for most of the variation in alpha diversity for 23 mountain regions in southern and central Europe (SACEU), with a lesser role for precipitation. For those ranges, and at a larger scale including the mountain regions of oroarctic and mid-latitude North America and Europe, Malanson et al. (2020, 2022a, respectively) reported an increase in the explanatory role of distance over climate with increased separation. Elsewhere, Anthelme et al. (2014) identified isolation within the Andes as the primary determinant of patterns of endemism, diversity, and similarity across plant and animal taxa. Finally, Testolin et al. (2021) found that the extent, isolation and bedrock variability were primary determinants of plant species richness in global alpine regions. These studies leave the continental biogeography of mountain regions under-investigated.
Since the Last Glacial Maximum (LGM), alpine vegetation has spatially tracked climate from more extensive lower-elevation distributions to its current position. With such movement through time, the potential for disequilibrium (i.e., wherein following and during change in the abiotic environment the community composition and diversity have not reached, a steady state) is high. Instances of such disequilibrium has been documented in paleoecology and inferred from current patterns. We focus on this period because of its primary relevance to current assemblages (e.g., Svenning and Skov, 2007; Cubino et al., 2022).
The mountain regions of WUSAC and SACEU are spatially and temporally different. WUSAC has mountains aligned on two major, parallel axes, the Rocky Mountain and Sierra-Cascade cordilleras, with a scattering of fault-blocks and volcanoes between. The Rocky Mountains, except for the Wyoming Gap, are relatively continuous for over 4,000 km. The Sierra-Cascades include the largest individual area, the Sierra Nevada, and a series of isolated volcanoes between it and the coastal ranges of British Columbia, which merge into the Rocky Mountains farther north. In WUSAC the mountains arose in a east-to-west sequence. The Rocky Mountains were uplifted in the late Cretaceous and early Paleogene; the Great Basin ranges arose in the Oligocene and more recently for some volcanoes. The Sierra-Cascades are youngest, with some mountains dating from the Pliocene but the main Sierra Nevada and Cascade volcanoes from less than 5 mya. The alpine flora of WUSAC developed in the Eocene following uplift (Axelrod and Raven, 1985; Wolfe, 1987), and adaptations to local environments began in the Pliocene (Billings, 1974).
The mountains of SACEU are divided into more distinct regions but with fewer isolated volcanic peaks. The ranges are part of a large arc that extends from northwestern Africa into west-central Asia, but the separation by low elevations is more distinct than the pattern in WUSAC. Although the Alpide Orogeny of Europe can be lumped into a single framework, it was more complicated than the orogenies of western North America. Uplift in the Carpathians began in the late Mesozoic, in the central Alps in the late Cretaceous, from Eocene to Miocene in the Pyrenees and Cantabria, and most recently in the Apennines. Comes and Kadereit (2003) argued that the species pools of these alpine habitats developed throughout the Quaternary, not only late, and preceded the most intensive climatic fluctuations; however, evidence exists for specific responses to glacial cycles (e.g., Stehlik, 2003).
In Europe, Normand et al. (2011) reported that, for several vegetation types, climate has been the most important driver of range shifts since LGM in Europe, but dispersal limitations were also important and contributed to disequilibria. Dullinger et al. (2012) identified slow dispersal as a primary cause of disequilibrium in vegetation in the Alps but cited differences in niche breadth as a factor. Rixen and Wipf (2017) added facilitation among neighbors as another. Differences in substrate affinities have further differentiated vegetation response to climate change (Nicklas et al., 2021). Refugia in SACEU were predominantly on the southern slopes of the more northern ranges and on the Iberian, Italian, and Hellenic peninsulas and would have been less isolated at LGM than now but still distinct (Gentili et al., 2015; Fauquette et al., 2018; Jiménez-Alfaro et al., 2021).
In North America post-glacial assembly of alpine plant communities would have been influenced by the sizes and proximity of refugial alpine habitats, which were more extensive than at present (Billings, 1978; Harris, 2002, 2007; Shafer et al., 2010; DeChaine et al., 2013; Roberts and Hamann, 2015). Harris (2002) described a concept of disequilibrium conditions for the Rocky Mountains in Canada as a consequence, and Malanson et al. (2017) subsequently attributed the low association of alpine community differences with abiotic gradients to disequilibrium. The association of the nestedness component of similarity with low climatic stability and historical legacies among North American biomes reported by Marta et al. (2019) may not be relevant within the alpine habitat, which they did not differentiate, because of its high diversity.
We expect that the contrast between SACEU and WUSAC, with more spatially distinct ranges and climates in the former, will elucidate differences in the importance of climatic niches and geographical and historical legacy effects. The greater isolation in SACEU should have resulted in less biological exchange in the vascular plant taxa through the climate changes of the late Quaternary than would be expected for WUSAC. The species pools of SACEU will have developed over longer periods in their local climates. Thus, the differences in geography may underlie differences in the importance of variables that affect the beta diversity of these systems. To investigate this conjecture, we devised a simulation experiment that compares representations of adaptations as well as spatial patterns, and null alternatives, at sub-continental scale. Within the model framework and for explaining beta diversity, we hypothesize that:
• Differences in climate will be relatively more important in SACEU than in WUSAC.
• Legacy effects will be more important in WUSAC than in SACEU.
We assess the models by correlating simulated and observed regional patterns of similarity as indicative of beta diversity (Anderson et al., 2011). We evaluate climatic and legacy effects, but with only two systems we do not infer a general pattern of the drivers of community assembly on a gradient of geographical isolation.
2. Materials and methods
To investigate the connections among mountain regions we develop comparable simulations that simplified the systems. Simplified models can represent species adaptations completely determined either by the abiotic environment or by the spatial pattern on templates where these can be controlled; these are never definitive tests but can be heuristic. We constructed simulations for a simplification of the geographies of WUSAC and SACEU and a null model geography. We created virtual species with fitness representing geographic legacies or adaptation to climatic gradients, assuming that the former was useful for the purpose of comparison because a true null model was a weak strawman (substantiated preliminary analyses of a null model; cf. Malanson, 2018). We created a spatially explicit individual-based simulation with Monte Carlo reproduction, dispersal, and mortality in Netlogo (v6.1.1; Wilensky, 1999); Bauduin et al. (2019) summarized the advantages of such simulations, which have a long history in alpine ecology (e.g., Humphries et al., 1996). A particular advantage is that regional ecological patterns can emerge from population-level processes in spatially explicit landscapes and the details of both can be varied at any level of abstraction (e.g., Zeng and Malanson, 2006).
We describe model development and provide an example of the code in Supplementary material Appendix 1. Then we detail the analyses of model results.
2.1. Simulations
A comparison of different spatial configurations using the virtual species adapted to different drivers can elucidate how the pattern of species distributions responds to control by geography vs. biology. Our purpose is to assess the relative importance of adaptations to climatic gradients and the legacies of spatial response to climate change to the determination of broad patterns of beta diversity in alpine environments.
We designed four versions of the simulation model:
A. Approximate spatial pattern of mountain regions; species response functions on two climate gradients (Figure 1A). This combination represents strong climatic niche with approximated extant spatial pattern.
B. Approximate spatial pattern of mountain regions; species response functions on the x, y (longitude, latitude) coordinates (Figure 1B). This combination represents species fitness as a function of locations, not climate, to represent legacy effects.
C. Regions regularly arranged in space; species response functions on two climate gradients (Figure 1C). This combination represents niche without extant spatial pattern.
D. Regions regularly arranged in space; species response functions on the x, y coordinates (Figure 1D). This combination represents legacy effects without extant spatial pattern.
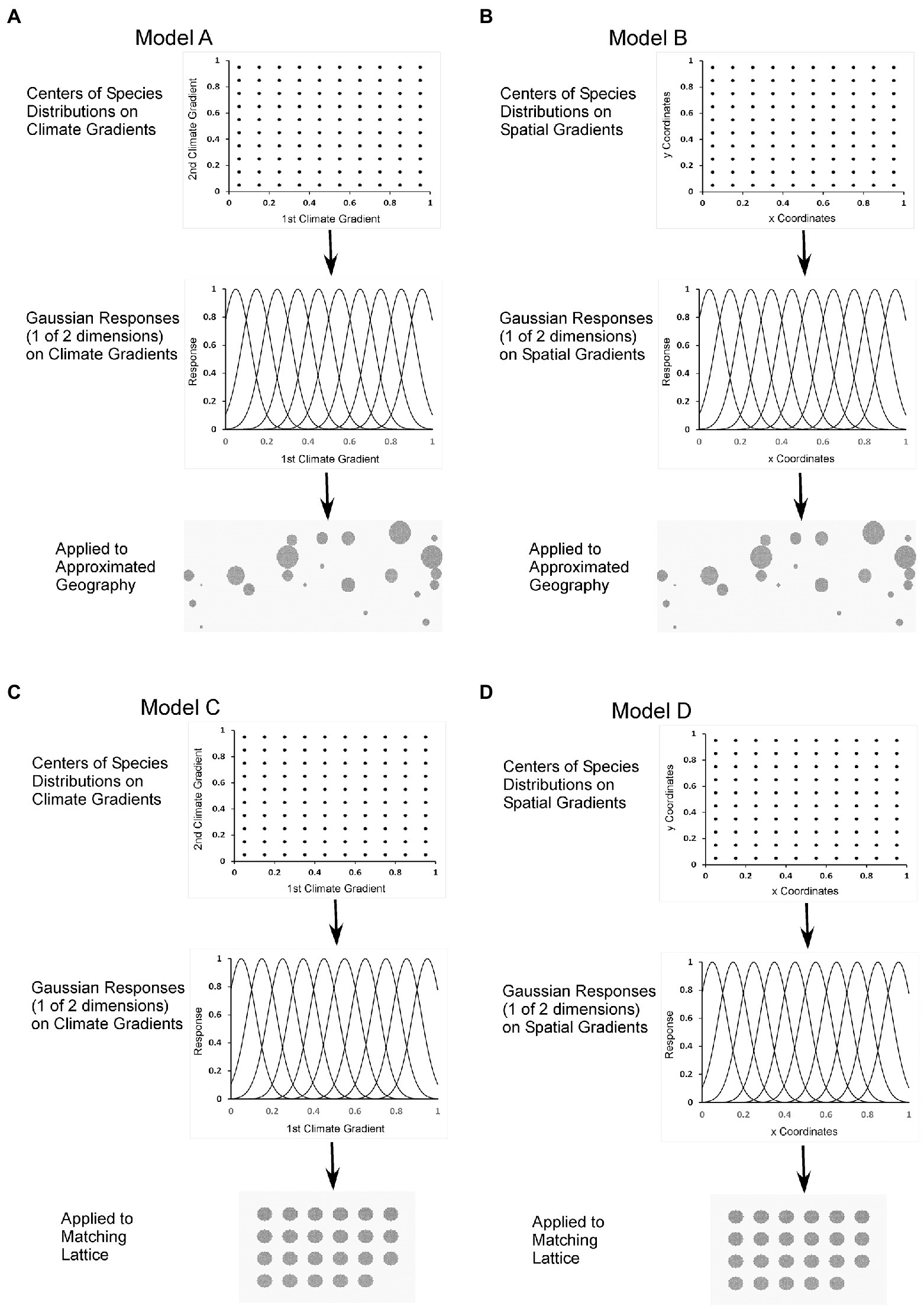
Figure 1. The four models (A–D) that were the basis of the simulations. The four models are for two parameterizations of the virtual species (adaptations to climate and to location) and two representations of geography (an approximation of the mountain ranges and a regular lattice). For either two climatic gradients or two spatial gradients (top panels) species adaptations were set as Gaussian response functions (middle panels; single climate and location gradients are shown, but two were used to define 100 virtual species). Simplified geographies and a regular lattice of the same number of ranges of SACEU are shown (bottom panels), but similar constructs for WUSAC were also used.
Thus, the combinations were: geographic pattern-climate adaptation; geographic pattern-legacy effects; lattice pattern-climate adaptation; lattice pattern- legacy effects. These four combinations were implemented with five levels of dispersal limitation. Comparisons among model designs qualitatively parse the relative importance of niche, location, and dispersal limitation.
The comparisons of designs A with B and of C with D are contrasts of representations of strong climatic niche determination versus neutral assembly (sensu Hubbell, 2001) relations of species with the environment – but having been filtered by dispersal and evolution. Contrasts of designs A with C and of B with D should differentiate the role of geographical patterns of the mountain regions (A, B) by extracting the role of spatial pattern in the regular arrangements (C, D); these contrasts are with climatic control in the former and only spatial patterns in the latter, which reflect legacies but otherwise neutral biogeographies. Comparisons are in terms of how well the pattern of similarity among regions replicates the similarity of the observed species pools for WUSAC and SACEU. In all designs we examined degrees of dispersal limitation.
The four high-level model designs were run independently, and their results are compared for both regions. Each design is run independently with five levels of dispersal limitation. Within each version, at each iteration agents reproduce, and the offspring disperse across the grid; agents are removed randomly to maintain a maximum density; and agents are removed depending on their adaptation to the grid cell climate or location. The number of agents per species of each grid cell is updated at each iteration, and the similarity of the areas of habitat is calculated at the end of all iterations (following equilibrium).
2.1.1. Initialization of species
The plant communities of these alpine habitats are represented by 101 individual species, the agents. To simplify computation, 101 species is a virtual microcosm of the system that allows for inferences on relative relationships. Preliminary sensitivity analyses with 50 and 150 virtual species produced results that were slightly different, with higher correlations for WUSAC and lower correlations for SACEU in both alternatives, so 101 was retained as a compromise. One of these 101 was added post-simulation with a ubiquitous distribution because some species are found nearly everywhere within a subcontinent (e.g., Nardus stricta is in all 23 regions SACEU regions and Trisetum spicatum is in 50 of the WUSAC regions). Then the adaptations of 100 virtual species were created with Gaussian response functions, or bells, in two dimensions of either climate or location coordinates. This approach follows earlier theoretical models of species distributions on environmental gradients (e.g., Gauch and Whittaker, 1976), but we recognize that Gaussian functions are not necessarily common (Falster et al., 2021). The peaks of the Gaussian bells were located on the surfaces (0–1 in both dimensions) at 0.10 increments from 0.05 through 0.95 (top panels of Figure 1), and for each species i and all climate or coordinate combinations x, a response value, Rix, which is the central element of all Monte Carlo processes, was assigned as:
–where E’x and E”x are the positions of the cell on the two climate or spatial gradients; the m’I and m”I are the positions of the modes of the species on the gradients; and σ2 is the standard deviation of the Gaussian function, chosen here as 0.001, which produced distributions of regional species richness spanning nearly the full possible range across all simulations. Thus, 100 such equations define 100 virtual species with the potential to fill all niches and occupy all locations. The shape of the bells and their overlap for a single row or column are illustrated in Figure 1, middle panels.
2.1.2. Initialization of regions
For designs A-B, we assigned locations of centers for the 54 or 23 regions on a grid with 300 cells in the longest dimension based on their latitude and longitude, ignoring the planet’s curvature (e.g., Figure 1, bottom panels). The centroids of the regions, based on observed geography, were located on the grid (Table A1 in Supplementary material Appendix 2). The cells within a circle of area proportional to the number of species observed (see section 2.4.1) were designated as the given region (radii are listed in Supplementary Table A1). For designs C and D, the regions were placed in a regular 6 × 9 or 6 × 4 less 1 pattern with 20-cell spacing between centers and evenly sized with radii of 7 cells.
The environment of the cells of each mountain range on the grid was set for two variables. First, we selected individual climate variables for temperature and precipitation. Individual variables are more directly interpretable in terms of their biological relevance than are derived variables, such as PCA axes, which can mix irrelevant variables or combinations if correlated. Based on preliminary analyses indicating constraining relationships, we selected mean temperature of the hottest quarter (HQT) and precipitation of the hottest quarter (HQP) for SACEU from CHELSA (Karger et al., 2017, 2018). For WUSAC we selected May 1 snow-water-equivalent (SWE) from DAYMET (Thornton et al., 2018) and HQT from CHELSA. The locations were selected within the alpine zone between treeline and the elevation of peaks or peak near the center of the mountain range. These values were assigned to all cells of the range (Supplementary Table A1). The values were then recalculated relative to the largest to create a gradient, 0–1, for both variables. For the location-based adaptations, the two values were set as the relative position of the cell on the grid in both the x and y dimensions.
2.1.3. Processes and iterations
To initialize the distributions, the species were stochastically allotted on each active cell if a uniform random number (0–1) was less than their response value for that cell. Individuals were randomly selected and removed while the number per cell exceeded 100, which limited computation size while allowing the possibility of complete coexistence.
To simulate reproduction with dispersal, in each iteration individuals produce an offspring that is dispersed in a random direction at a random distance (number of cells; >0) selected from a truncated Gaussian distributions with varying standard deviations: 1, 10, 20, 30, and 50 cells (e.g., Figure 2), which restrict interaction to regions or allows interaction across most regions. A Gaussian distribution has a relatively thin tail and so limits the effects of rare long-distance dispersal and was chosen to limit noise within replication runs (Malanson and Rodriguez, 2018).
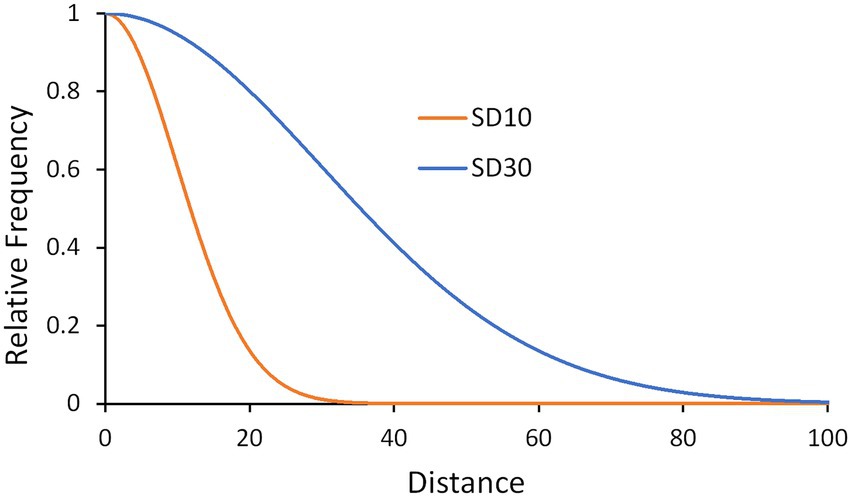
Figure 2. Example distributions of the relative frequency of dispersal distances for two of the five kernels.
To simulate mortality, individuals were removed at each iteration if a uniform random number (0–1) was greater than their response value for that cell. Additionally, individuals were randomly selected and removed while the number of individuals per cell exceeded 100.
The simulations equilibrated at <50 iterations, and the presence of individuals of each species on each region was written to an external file after 60 iterations. The simulations were replicated 10 times. In total, for 2 continents, 4 designs were run at 5 levels of dispersal limitation, and the averages of the 10 replications of the 40 runs were compared in post-simulation analyses.
2.2. Analyses
2.2.1. Observed vegetation
We recorded presence-absence of vascular plant species using a dataset of alpine grassland vegetation extracted from the European Vegetation Archive (Chytrý et al., 2016) by Jiménez-Alfaro et al. (2021). The WUSAC dataset was derived from a variety of sources, including theses and regional floras by Malanson et al. (2015) with some additions. The species lists for added regions that did not well-differentiate the alpine zone were reduced to those species that were in the alpine zone of at least three of the regions with good differentiation or were linked to the alpine by name (e.g., binomial alpinus or common name “alpine …”). This choice was a compromise between excluding regions and including species as alpine that were not. The species nomenclature was updated and reconciled using the Taxonomic Name Resolution Service1 cross-checked with the UDSA PLANTS Database.2 The 54 regions with the sources of the species pools are listed in Supplementary material Appendix 2, Table A2 and the range by species table is stored in Dryad and associated with this paper.3 The two datasets are not directly comparable, but both allow comparison to the patterns of beta diversity in the simulation results.
In the 23 ranges of SACEU Regional species richness ranged from 95 to 869 species and across all regions was 3,261 species. In the 54 ranges of WUSAC, richness ranged from 14 to 504 and totaled 1,437 species. From these presence-absence records we calculated the Sørensen (1948) similarity index of all pairs per continent, which are also archived in Dryad.
2.2.2. Correlations of simulations to observations
The distributions of >1,400 species on the 54 regions in WUSAC or >3,300 on the 23 regions in Europe and those of the 100 virtual species in the simulations could not be compared directly. Given the limitation of the virtual microcosm approach, and thus the outcomes produced by the model – presence of a species in a region – we calculated the Sørensen (1948) similarity index between all pairs of regions as observed and for each replicate simulation of each model. Similarity of species composition is commonly used to represent beta diversity (e.g., Izsak and Price, 2001) and as an indicator of the process of community assembly (e.g., Jurasinski and Kreyling, 2007). We compared the similarity outcomes in the simulations to those of the 1,431 pairs of sites in WUSAC and the 253 pairs in SACEU by calculating the Pearson correlation between the observed and means of the 10 replications of each model, for which we assessed the significance using randomization (10,000 repetitions using PC-ORD v.7, McCune and Mefford, 2018) to bypass the problem of lack of independence among the similarities.
3. Results
The average observed Sørensen indices are similar (means of 0.263 for SACEU, 0.266 for WUSAC; Supplementary Material Appendix 2 Tables A3, A4). The correlations between the simulations and the observed similarities range from non-significant to >0.5 (Figure 3). The correlations differ among the designs and on the gradient of dispersal limitation. In all cases, the correlations are for the pattern of similarity among pairs, but the simulated similarities are consistently higher than those observed, as might be expected for models with well-defined adaptations of a limited number of species when compared to nature.
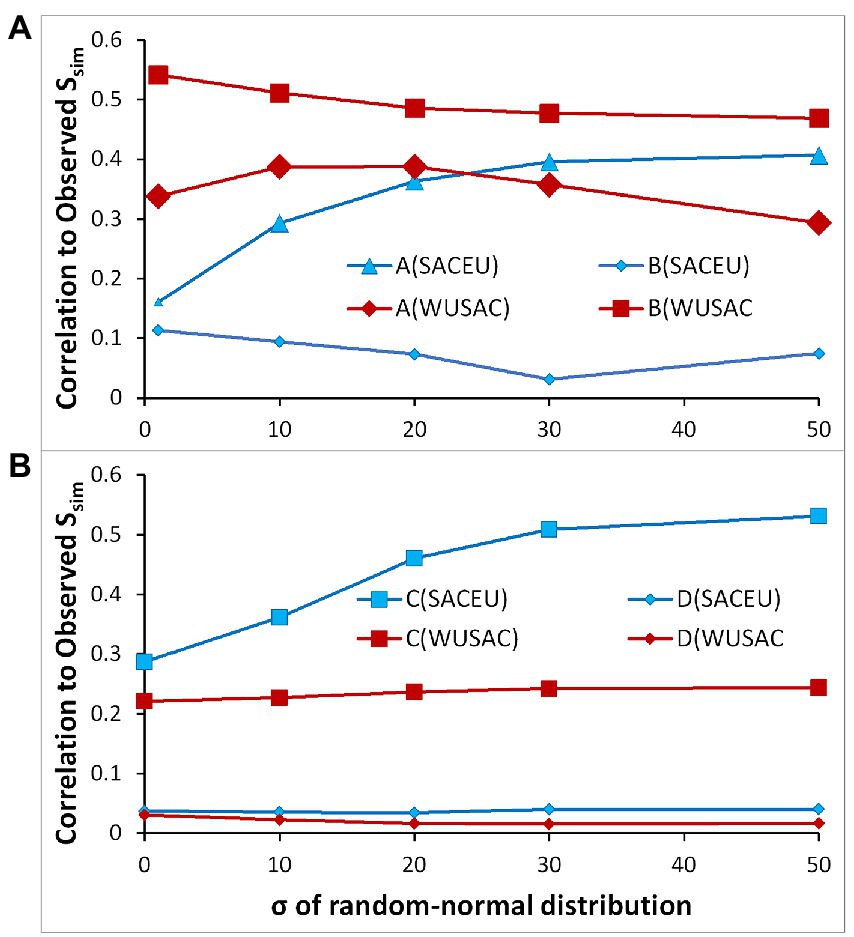
Figure 3. Correlations of simulated with observed similarities among all possible pairs of ranges in (A,B) SACEU (253) and WUSAC (1431) for designs A,B and C,D. The dispersal paramterCases with larger symbols have p < 0.01 and their 99% confidence intervals are within the size of the symbol.
The WUSAC simulations have consistent correlations with the observed pattern of similarity, and the one with species adapted to location has the highest correlation at all dispersal limitations (Figure 3, design B). This result indicates that spatial pattern of the mountain regions is a strong determinant of current similarity in WUSAC. The simulations with species adapted to climate are still well-correlated to the observed similarities, which indicates that the abiotic environment has some effect on the pattern.
For SACEU, simulations with species adapted to location have consistently low and non-significant correlations (Figure 3, designs B, D). The virtual species adapted to climate have correlations exceeding the matching case in WUSAC and approaching the highest correlations for the location-adapted species there – but only with minimal dispersal limitation (Figure 3, design A). Longer-distance dispersal re-sorts the species distributions at every iteration, and the resulting distributions have patterns of similarity more like the observed patterns.
The designs with the regular arrangement of mountain regions reveal the importance of different processes. All such simulation runs with the species adapted to x, y location had low, non-significant correlations with observed similarities (Figure 3, design D), which was a marked contrast for WUSAC when the actual spatial position of the ranges was approximated, and coordinate-adaptation resulted in higher correlations. The designs with the species adapted to climate had lower correlations for WUSAC, but those for SACEU, however, had similarities that significantly exceeded those with the approximated spatial position of the ranges (Figure 3, design C). In WUSAC, the climate plays a lesser, but significant role, and the extant spatial pattern is most important. In SACEU, climate plays a dominant role in these simulations, but only because the lattice arrangement of the ranges masks their true isolation.
4. Discussion
We hypothesized that the simulated beta diversity would more closely correlate with observed in the simulations that were designed to represent climatic adaptations and patterns in SACEU but in WUSAC the correlations would be higher in the simulations representing legacy effects. These hypotheses were generally supported. Given that the simulations are for 100 species in a simplified environment with >1,400 and >3,300 species in complex, continental-scale environments, those that are significant can be compared. Simulations suggest that the processes that primarily determine the patterns of beta diversity in WUSAC and SACEU differ because of the distinct spatiotemporal pattern of orogenies over the past 100 million years, not because of current climatic factors influencing plant reproduction, growth, or mortality. Within these regions, these differences have left stronger associations with climate in SACEU than in WUSAC. These results may help explain the differences in the climatic envelopes for the alpine grasslands in SACEU (Malanson et al., 2022b) and the weak or moderate relations with climate reported for WUSAC (Hadley, 1987; Malanson et al., 2015, 2017).
4.1. Specific results
The correlations of observed and simulated similarity support our hypotheses. Four lines of evidence support the conclusion that the pattern of beta diversity across alpine vegetation at the scale of mountain regions on the continents is determined by different factors in WUSAC and SACEU:
1. With climate-adapted species and approximated geography (Model A: Figures 1A, 3A), dispersal limitation is evident among ranges in SACEU whereas it was not in WUSAC.
2. With species responding to legacy effects and approximated geography (Model B: Figures 1B, 3A), correlations with observed patterns in SACEU were consistently low and non-significant, whereas in WUSAC the correlations were consistent and reached the highest observed in all simulations for almost every degree of dispersal limitation.
3. With the climate-adapted species and the lattice arrangement of ranges (Model C: Figures 1C, 3B), correlations with observations in SACEU again displayed sensitivity to dispersal limitation that was not evident for WUSAC. SACEU simulations on the lattice had higher correlations than with approximated geography, whereas WUSAC correlations were lower for the lattice cases (Model C vs. Model A).
4. With legacy effect species on a lattice arrangement of ranges (Model D: Figures 1D, 3B), all levels of dispersal limitation for both SACEU and WUSAC had near-zero correlations – the only combination in which they were similar.
For WUSAC, a spatial but climate-neutral determination of species reproduction and mortality came closest to replicating the observed pattern of similarity among mountain ranges. The pattern is moderately controlled by climate variables, and instead the relative importance of location is evidence that the template of mountain geography is more likely responsible for the current pattern of species distributions. This balance of factors in the simulation is similar to the relative importance of climatic and spatial variables reported by Malanson et al. (2015).
In SACEU, however, adaptations to climate gradients appear to be more important for the determination of patterns of alpine vegetation, but these simulations are sensitive to dispersal limitations and can be equaled or exceeded with abstract mountain locations. Although climate is significant, its effects in determining the pattern of similarities among ranges is overridden by their isolation and dispersal limitation. The higher correlations with the lattice arrangement relative to those with approximate geography of the regions, especially with greater dispersal limitation, indicate that the isolation of the regions has limited how well the vegetation could have conformed to changing climate in the past. These simulations better matched observed patterns of similarity among pairs of ranges than did those for WUSAC even though SACEU is a more challenging system with more than twice as many species in half as many locations. The stronger role of climate variables for beta diversity between nearby regions, as reported by Malanson et al. (2022a), is paralleled in the simulations.
4.2. Spatial and temporal constraints
The low correlations are expected with a simulation based on a purely theoretical distribution of 100 virtual species vs. >3,300 species for SACEU and >1,400 for WUSAC. That the climate-based correlations are similar for the two continents, given the difference in gamma diversity, indicates that the environmental controls are relatively simple in SACEU. WUSAC has more extremes because of a greater latitudinal extent of the Rocky Mountains, both Mediterranean and marine west coast climates of the Sierra-Cascades, and the desert climates of the Great Basin (Beck et al., 2018).
These results indicate that the climatic template is relevant, but the geography is more important. In both continental systems the pattern is adjusting to climate change, but the different geographies determine the outcomes to this point in time. WUSAC species pools are more strongly determined by historical biogeography – especially the division between the Rocky Mountain and Sierra-Cascade cordilleras – than by climatic niche (Billings, 1978; Malanson et al., 2015). The climatic gradients are confounded with latitude and longitude in WUSAC, and the use of x,y coordinates captures the simple patterns of the regions. Conversely, SACEU does not have this relationship because the geography is structured differently, with a broad east–west arc with Mediterranean-type climates in the southwest and southeast and mid-latitude climates with greater continentality in the center (Beck et al., 2018), and climate has a greater effect many species (Normand et al., 2011) and on beta diversity within broad regions than across the entire arc (Malanson et al., 2022a). Thus, the biogeographic connections of species pools in SACEU to past refugia are more specific than in WUSAC. These results depend on the degree to which our abstraction captures the spatial pattern of the observed mountain geography.
A clear response to limited dispersal is evident only in the simulations for SACEU with climate-adapted species show any response to limited dispersal. This result agrees with the report of Dullinger et al. (2012) and may further indicate that these ranges have been spatially isolated in the short term. If exchange is improbable, then the increased affinity of species to climate gradients is maintained because any initial species not well-adapted to climate are replaced only by immediate neighbors. Turnover rates will be lower, and the relative importance of climatic gradients in SACEU are not disrupted through repeated arrivals and mortality.
4.3. Environmental adaptations and legacies
Any difference between SACEU and WUSAC in the relative importance of factors contributing to the pattern of beta diversity across the ranges would be some indication of legacy effects but, given the differences in the vascular plant species pools, this is a trivial conclusion. The pattern of difference, however, suggests that the geography of the ranges affects this relative importance of causal factors, i.e., the geographical legacies affect the historical legacies. In SACEU, the more distinct isolation of ranges and consequent lesser interactions of their species pools indicates that the species pools are more limited – and adapted – to the given climates (e.g., Svenning and Skov, 2007; Normand et al., 2011; Jiménez-Alfaro et al., 2021; Cubino et al., 2022). The less distinct isolation in WUSAC, especially in the Rocky Mountains, may have contributed to greater similarities among the species pools of refugia, which were not as spatially distinct as those in SACEU, and there has been greater mixing of Arctic and southern pools in the Holocene (Billings, 1978; Harris, 2002, 2007; Shafer et al., 2010; DeChaine et al., 2013; Roberts and Hamann, 2015). The legacies that differentiate the current species pools are from the more distant past in SACEU than in WUSAC, which is also seen in the higher rate of endemism among plant species in the former (Nagy and Grabherr, 2009, p. 185). With more distinct species pools in the refugia of SACEU, the pattern of beta diversity is more determined by the adaptations to climate that have evolved since initial periods of mountain building and assembly.
5. Conclusion
An underlying pattern for how legacy effects matter would be informative (Ricklefs et al., 1999). While the lesser role for climate in WUSAC could be argued as evidence for the neutral (sensu Hubbell, 2001) assembly of the species pools, it instead is a product of greater disequilibrium. Indeed, neutral assembly is an equilibrium theory, and is not relevant to most alpine systems. It may be that legacy effects could explain some instances of seemingly neutral assembly.
These simulations apply only to two places, and inference to a general pattern of geography and legacy effects is limited. New work using larger datasets may be able to take on this challenge. For example, a global dataset would allow comparisons of multiple regions (e.g., Testolin et al., 2021), but further database developments are needed (e.g., to sPlot; Bruelheide et al., 2019) as are simulations with higher spatial and biological resolution. Better quantification of isolation, with recognition of past pathways as well as dispersal kernels, could define a gradient of isolation that could underlie differences in the processes determining plant community assembly (Saura et al., 2014), and the inclusion of more realistic response or fitness functions of species, with competition, facilitation, and intraspecific variation (e.g., Malanson, 2015, 2018), would allow more rigorous tests of outcomes other than beta diversity.
The experimental design using alternative combinations of virtual species with climate adaptation vs. legacy effects and of realistic and lattice patterns of virtual geographies was effective in elucidating the differences in processes that could explain the biogeographic patterns in Europe and North America. However, the regular parameterization of the virtual species, which evenly filled the space of the two environmental or spatial gradients, might be improved by better matching them to the actual extant climate and its spatial distribution on alpine habitats. Further, parameterization of varied dispersal limitations, competitive and facilitative interactions, and intraspecific variability among virtual species within simulation runs can increase their heuristic value (Brooker et al., 2008; Phillips et al., 2008; Smith-McKenna et al., 2014). Analysis of the outcomes of well-defined virtual species in addition to community level results can inform the intersection of theory and the contingencies of historical and geographical constraints. Nevertheless, representing species adapted to climate versus legacy effects in general, when used in simulation experimental design with realistic and abstract patterns of geography, provide a pathway for elucidating the influences of geography and history, which are often unobserved. This understanding will be necessary for preparing for the impacts of climate change in alpine vegetation.
Data availability statement
The datasets presented in this study can be found in online repositories. The names of the repository/repositories and accession number(s) can be found at: https://doi.org/10.5061/dryad.0cfxpnw1h, https://doi.org/10.5061/dryad.8w9ghx3nz and https://doi.org/10.5061/dryad.xksn02vkp.
Author contributions
GM developed the concept, wrote the simulations, did the analyses, and wrote the first draft. EP contributed to the concept, organized some of the data, and commented on early drafts. RT organized some of the data and commented on early drafts. BJ-A supervised part of the data management, contributed to interpretation, and commented on early drafts. All authors contributed to the article and approved the submitted version.
Funding
This work was supported by US National Science Foundation award 1853665 to GM, by funding from LifeWatchPLUS (CIR-01_00028) to RT, and from the Marie Curie Clarín-COFUND programme of the Principality of Asturias-EU (ACB17-26) and Spain’s Agencia Estatal de Investigación (AEI/10.13039/501100011033) to BJ-A. The funders supported independent research.
Conflict of interest
The authors declare that the research was conducted in the absence of any commercial or financial relationships that could be construed as a potential conflict of interest.
Publisher’s note
All claims expressed in this article are solely those of the authors and do not necessarily represent those of their affiliated organizations, or those of the publisher, the editors and the reviewers. Any product that may be evaluated in this article, or claim that may be made by its manufacturer, is not guaranteed or endorsed by the publisher.
Supplementary material
The Supplementary material for this article can be found online at: https://www.frontiersin.org/articles/10.3389/fevo.2023.1053017/full#supplementary-material
Footnotes
References
Anderson, M. J., Crist, T. O., Chase, J. M., Vellend, M., Inouye, B. D., Freestone, A. L., et al. (2011). Navigating the multiple meanings of β diversity: a roadmap for the practicing ecologist. Ecol. Lett. 14, 19–28. doi: 10.1111/j.1461-0248.2010.01552.x
Anthelme, F., Jacobsen, D., Macek, P., Meneses, R. I., Moret, P., Beck, S., et al. (2014). Biodiversity patterns and continental insularity in the tropical high Andes. Arct. Antarct. Alp. Res. 46, 811–828. doi: 10.1657/1938-4246-46.4.811
Axelrod, D. I., and Raven, P. H. (1985). Origins of the cordilleran flora. J. Biogeogr. 12, 21–47. doi: 10.2307/2845027
Barredo, J. L., Mauri, A., and Caudullo, G. (2020). Alpine tundra contraction under future warming scenarios in Europe. Atmos. 11:698. doi: 10.3390/atmos11070698
Bauduin, S., McIntire, E. J. B., and Chubaty, A. M. (2019). NetLogoR: a package to build and run spatially explicit agent-based models in R. Ecography 42, 1841–1849. doi: 10.1111/ecog.04516
Beck, H. E., Zimmermann, N. E., McVicar, T. R., Vergopolan, N., Berg, A., and Wood, E. F. (2018). Present and future Köppen-Geiger climate classification maps at 1-km resolution. Sci. Data 5:180214. doi: 10.1038/sdata.2018.214
Billings, W. D. (1974). Adaptations and origins of alpine plants. Arct. Alp. Res. 6, 129–142. doi: 10.2307/1550081
Billings, W. D. (1978). Alpine phytogeography across the Great Basin. Great Basin Nat. Mem. 2, 105–117.
Birks, H. J. B., and Willis, K. J. (2008). Alpines, trees, and refugia in Europe. Plant Ecol. Divers. 1, 147–160. doi: 10.1080/17550870802349146
Brooker, R. W., Maestre, F. T., Callaway, R. M., Lortie, C. L., Cavieres, L. A., Kunstler, G., et al. (2008). Facilitation in plant communities: the past, the present, and the future. J. Ecol. 96, 18–34. doi: 10.1111/j.1365-2745.2007.01295.x
Bruelheide, H., Dengler, J., Jiménez-Alfaro, B., Purschke, O., Hennekens, S. M., Chytrý, M., et al. (2019). sPlot – a new tool for global vegetation analyses. J. Veg. Sci. 30, 161–186. doi: 10.1111/jvs.12710
Chytrý, M., Hennekens, S. M., Jiménez-Alfaro, B., Knollová, I., Dengler, J., Jansen, F., et al. (2016). European vegetation archive EVA: an integrated database of European vegetation plots. Appl. Veg. Sci. 19, 173–180. doi: 10.1111/avsc.12191
Comes, H. P., and Kadereit, J. W. (2003). Spatial and temporal patterns in the evolution of the flora of the European alpine system. Taxon 52, 451–462. doi: 10.2307/3647382
Cubino, J. P., Chytrý, M., Divíšek, J., and Jiménez-Alfaro, B. (2022). Climatic filtering and temporal instability shape the phylogenetic diversity of European alpine floras. Ecography 2022:e06316. doi: 10.1111/ecog.06316
DeChaine, E. G., Forester, B. R., Schaefer, H., and Davis, C. C. (2013). Deep genetic divergence between disjunct refugia in the arctic-alpine King’s crown, Rhodiola integrifolia (Crassulaceae). PLoS One 8:e79451. doi: 10.1371/journal.pone.0079451
Dullinger, S., Willner, W., Plutzar, C., Englisch, T., Schratt-Ehrendorfer, L., Moser, D., et al. (2012). Post-glacial migration lag restricts range filling, of plants in the European Alps. Glob. Ecol. Biogeogr. 21, 829–840. doi: 10.1111/j.1466-8238.2011.00732.x
Falster, D. S., Kunstler, G., Fitzjohn, R. G., and Westoby, M. (2021). Emergent shapes of trait-based competition functions from resource-based models: a Gaussian is not normal in plant communities. Am. Nat. 198, 253–267. doi: 10.1086/714868
Fauquette, S., Suc, J. P., Medail, F., Muller, S. D., Jimenez-Moreno, G., Bertini, A., et al. (2018). “The Alps: a geological, climatic and human perspective on vegetation history and modern plant diversity” in Mountains, Climate and Biodiversity. eds. C. Hoorn, A. Perrigo, and A. Antonelli (Chichester, UK: Wiley), 413–428.
Gauch, H. G., and Whittaker, R. H. (1976). Simulation of community patterns. Vegetatio 33, 13–16. doi: 10.1007/BF00055294
Gentili, R., Badola, H. K., and Birks, H. J. B. (2015). Alpine biodiversity and refugia in a changing climate. Biodiversity 16, 193–195. doi: 10.1080/14888386.2015.1117023
Graae, B. J., Vandvik, V., Armbruster, W. S., Eiserhardt, W. L., Svenning, J. C., Hylander, K., et al. (2018). Stay or go–how topographic complexity influences alpine plant population and community responses to climate change. Perspect. Plant Ecol. Evol. Syst. 30, 41–50. doi: 10.1016/j.ppees.2017.09.008
Hadley, K. S. (1987). Vascular alpine plant distributions within the central and southern Rocky Mountains, USA. Arct. Alp. Res. 19, 242–251.
Harris, S. A. (2002). “Biodiversity of the vascular timberline flora in the Rocky Mountains of Alberta, Canada” in Mountain biodiversity: A global assessment. eds. C. Korner and E. M. Spehn (Boca Raton, FL: CRC Press), 49–57.
Harris, S. A. (2007). Biodiversity of the alpine vascular flora of the NW north American cordillera: the evidence from phyto-geography. Erdkunde 61, 344–357. doi: 10.3112/erdkunde.2007.04.05
Hubbell, S.P. (2001). A Unified Neutral Theory of Biodiversity and Biogeography. Princeton, NJ: Princeton University Press.
Humphries, H. C., Coffin, D. P., and Lauenroth, W. K. (1996). An individual-based model of alpine plant distributions. Ecol. Model. 84, 99–126. doi: 10.1016/0304-3800(94)00144-8
Izsak, C., and Price, A. R. G. (2001). Measuring b-diversity using a taxonomic similarity index, and its relation to spatial scale. Mar. Ecol. Prog. Ser. 215, 69–77. doi: 10.3354/meps215069
Jiménez-Alfaro, B., Abdulhak, S., Attorre, F., Bergamini, A., Carranza, M. L., Chiarucci, A., et al. (2021). Postglacial determinants of regional species pools in alpine grasslands. Glob. Ecol. Biogeogr. 30, 1101–1115. doi: 10.1111/geb.13274
Jurasinski, G., and Kreyling, J. (2007). Upward shift of alpine plants increases floristic similarity of mountain summits. J. Veg. Sci. 18, 711–718. doi: 10.1111/j.1654-1103.2007.tb02585.x
Karger, D. N., Conrad, O., Böhner, J., Kawohl, T., Kreft, H., Soria-Auza, R. W., et al. (2017). Climatologies at high resolution for the earth’s land surface areas. Sci. Data 4:170122. doi: 10.1038/sdata.2017.122
Karger, D. N., Conrad, O., Böhner, J., Kawohl, T., Kreft, H., Soria-Auza, R. W., et al. (2018). Data from: climatologies at high resolution for the earth’s land surface areas. Dryad Dataset. doi: 10.5061/dryad.kd1d4
Lenoir, J., Gegout, J.-C., Guisan, A., Vittoz, P., Wohlgemuth, T., Zimmermann, N. E., et al. (2010). Cross-scale analysis of the region effect on vascular plant species diversity in southern and northern European mountain ranges. PLoS One 5:e15734. doi: 10.1371/journal.pone.0015734
Malanson, G. P. (2015). Diversity differs among three variations of the stress gradients hypothesis in two representations of niche space. J. Theor. Biol. 384, 121–130. doi: 10.1016/j.jtbi.2015.08.012
Malanson, G. P. (2018). Intraspecific variability may not compensate for increasing climatic volatility. Popul. Ecol. 60, 287–295. doi: 10.1007/s10144-018-0612-y
Malanson, G. P., Cheney, A. B., and Kinney, M. (2015). Climatic and geographic relations of alpine tundra floras in western North America. Alp. Bot. 125, 21–29. doi: 10.1007/s00035-014-0144-9
Malanson, G. P., Pansing, E. R., Testolin, R., Abdulhak, S., Bergamini, A., Ćušterevska, R., et al. (2022a). Explanation of beta diversity in European alpine grasslands changes with scale. Ecosphere 13:e4066. doi: 10.1002/ecs2.4066
Malanson, G. P., Resler, L. M., Butler, D. R., and Fagre, D. B. (2019). Mountain plant communities: Uncertain sentinels? Prog. Phys. Geogr. 43, 521–543. doi: 10.1177/0309133319843873
Malanson, G. P., and Rodriguez, N. (2018). Traveling waves and spatial patterns from dispersal on homogeneous and gradient habitats. Ecol. Complex. 33, 57–65. doi: 10.1016/j.ecocom.2017.12.001
Malanson, G. P., Testolin, R., Pansing, E. R., and Jiménez-Alfaro, B. (2022b). Mesoscale refugia for European alpine grasslands based on climatic envelopes. Alp. Bot. 132, 169–180. doi: 10.1007/s00035-022-00283-0
Malanson, G. P., Virtanen, R., Britton, A. J., Jiménez-Alfaro, B., Qian, H., Petraglia, A., et al. (2020). Hemispheric and continental scale patterns of similarity in mountain tundra. Ann. Am. Assoc. Geogr. 110, 1005–1021. doi: 10.1080/24694452.2019.1677450
Malanson, G. P., Zimmerman, D. L., Kinney, M., and Fagre, D. B. (2017). Relations of alpine plant communities across environmental gradients: multilevel versus multiscale analyses. Ann. Am. Assoc. Geogr. 107, 41–53. doi: 10.1080/24694452.2016.1218267
Marta, S., Lacasella, F., Cesaroni, D., and Sbordoni, V. (2019). Effects of Holocene climate changes on alpine ecosystems: nonequilibrium dynamics drive insect species richness on alpine islands. J. Biogeogr. 46, 2248–2259. doi: 10.1111/jbi.13655
McCune, B., and Mefford, M.J., (2018). PC-ORD. Multivariate Analysis of Ecological Data v.7.08. (Corvallis, OR: Wild Blueberry Media).
Nagy, L., and Grabherr, G., (2009). The Biology of Alpine Habitats. (Oxford, UK: Oxford University Press).
Nicklas, L., Walde, J., Wipf, S., Lamprecht, A., Mallaun, M., Rixen, C., et al. (2021). Climate change affects vegetation change differently on siliceous and calcareous summits of the European Alps. Front. Ecol. Evol. 9:#642309. doi: 10.3389/fevo.2021.642309
Normand, S., Ricklefs, R. E., Skov, F., Bladt, J., Tackenberg, O., and Svenning, J. C. (2011). Postglacial migration supplements climate in determining plant species ranges in Europe. Proc. R. Soc. B 278, 3644–3653. doi: 10.1098/rspb.2010.2769
Phillips, B. L., Brown, G. P., Travis, J. M., and Shine, R. (2008). Reid’s paradox revisited: the evolution of dispersal kernels during range expansion. Am. Nat. 172, S34–S48. doi: 10.1086/588255
Pinto-Ledezma, J. N., Larkin, D. J., and Cavender-Bares, J. (2018). Patterns of beta diversity of vascular plants and their correspondence with biome boundaries across North America. Front. Ecol. Evol. 6:#194. doi: 10.3389/fevo.2018.00194
Ricklefs, R. E., Latham, R. E., and Qian, H. (1999). Global patterns of tree species richness in moist forests: distinguishing ecological influences and historical contingency. Oikos 86, 369–373. doi: 10.2307/3546454
Rixen, C., and Wipf, S. (2017). “Non-equilibrium in alpine plant assemblages: shifts in Europe’s summit floras” in High Mountain Conservation in a Changing World. eds. J. Catalan, J. M. Ninot, and M. M. Aniz (Cham, CH: Springer), 285–303.
Roberts, D. R., and Hamann, A. (2015). Glacial refugia and modern genetic diversity of 22 western north American tree species. Proc. R. Soc. B 282:20142903. doi: 10.1098/rspb.2014.2903
Saura, S., Bodin, Ö., and Fortin, M. J. (2014). Stepping stones are crucial for species’ long-distance dispersal and range expansion through habitat networks. J. Appl. Ecol. 51, 171–182. doi: 10.1111/1365-2664.12179
Shafer, A. B., Cullingham, C. I., Cote, S. D., and Coltman, D. W. (2010). Of glaciers and refugia: a decade of study sheds new light on the phylogeography of northwestern North America. Mol. Ecol. 19, 4589–4621. doi: 10.1111/j.1365-294X.2010.04828.x
Smith-McKenna, E. K., Malanson, G. P., Resler, L. M., Carstensen, L. W., Prisley, S. P., and Tomback, D. F. (2014). Cascading effects of feedbacks, disease, and climate change on alpine treeline dynamics. Environ. Model Softw. 62, 85–96. doi: 10.1016/j.envsoft.2014.08.019
Sørensen, T. (1948). A method of establishing groups of equal amplitude in plant sociology based on similarity of species and its application to analyses of the vegetation on Danish commons. Kongelige Danske Videnskabernes Selskab. 5, 1–34.
Stehlik, I. (2003). Resistance or emigration? Response of alpine plants to the ice ages. Taxon 52, 499–510. doi: 10.2307/3647448
Storch, D., Šímová, I., Smyčka, J., Bohdalková, E., Toszogyova, A., and Okie, J. G. (2022). Biodiversity dynamics in the Anthropocene: how human activities change equilibria of species richness. Ecography 2022:e05778. doi: 10.1111/ecog.05778
Svenning, J. C., and Skov, F. (2007). Ice age legacies in the geographical distribution of tree species richness in Europe. Glob. Ecol. Biogeogr. 16, 234–245. doi: 10.1111/j.1466-8238.2006.00280.x
Taylor, D. W. (1977). Floristic relationships along the Cascade–Sierran axis. Am. Midl. Nat. 97, 333–349. doi: 10.2307/2425098
Testolin, R., Attorre, F., Borchardt, P., Brand, R. F., Bruelheide, H., Chytrý, M., et al. (2021). Global patterns and drivers of alpine plant species richness. Glob. Ecol. Biogeogr. 30, 1218–1231. doi: 10.1111/geb.13297
Testolin, R., Attorre, F., and Jiménez-Alfaro, B. (2020). Global distribution and bioclimatic characterization of alpine biomes. Ecography 43, 779–788. doi: 10.1111/ecog.05012
Thornton, P.E., Thornton, M.M., Mayer, B.W., Wei, Y., Devarakonda, R., Vose, R.S., et al. (2018). Daymet: Daily Surface Weather Data on a 1-km Grid for North America, Version 3. ORNL DAAC, Oak Ridge, Tennessee, USA.
Van Meerbeek, K., Jucker, T., and Svenning, J. C. (2021). Unifying the concepts of stability and resilience in ecology. J. Ecol. 109, 3114–3132. doi: 10.1111/1365-2745.13651
Whittaker, R. J., Willis, K. J., and Field, R. (2001). Scale and species richness: towards a general, hierarchical theory of species diversity. J. Biogeogr. 28, 453–470. doi: 10.1046/j.1365-2699.2001.00563.x
Wilensky, U. (1999). NetLogo. Center for Connected Learning and Computer-Based Modeling, Northwestern University, Evanston, IL.
Wolfe, J. A. (1987). An overview of the origins of the modern vegetation and flora of the northern Rocky Mountains. Ann. Mo. Bot. Gard. 74, 785–803. doi: 10.2307/2399450
Keywords: alpine, beta diversity, biogeography, climate, Europe, individual-based model, North America, similarity
Citation: Malanson GP, Pansing ER, Testolin R and Jiménez-Alfaro B (2023) Simulations reveal climate and legacy effects underlying regional beta diversity in alpine vegetation. Front. Ecol. Evol. 11:1053017. doi: 10.3389/fevo.2023.1053017
Edited by:
Simon Kapitza, Helmholtz Association of German Research Centres (HZ), GermanyReviewed by:
Gwendolyn Peyre, University of Los Andes, Colombia, ColombiaJason Doll, Francis Marion University, United States
Copyright © 2023 Malanson, Pansing, Testolin and Jiménez-Alfaro. This is an open-access article distributed under the terms of the Creative Commons Attribution License (CC BY). The use, distribution or reproduction in other forums is permitted, provided the original author(s) and the copyright owner(s) are credited and that the original publication in this journal is cited, in accordance with accepted academic practice. No use, distribution or reproduction is permitted which does not comply with these terms.
*Correspondence: George P. Malanson, Z2VvcmdlLW1hbGFuc29uQHVpb3dhLmVkdQ==