- 1School of Agricultural, Earth and Environmental Sciences, University of KwaZulu-Natal, Durban, South Africa
- 2Discipline of Occupational and Environmental Health, University of KwaZulu-Natal, Durban, South Africa
- 3Centre for Coastal Palaeoscience, Botany Department, Nelson Mandela University, Gqeberha, South Africa
Our understanding of global diversity patterns relies overwhelmingly on ecological and evolutionary correlates of latitude, and largely ignores longitude. However, the two major explanations of biodiversity patterns – energy and stability – are confounded across latitudes, and longitude offers potential solutions. Recent literature shows that the global biogeography of the Cenozoic world is structured by longitudinal barriers. In a few well-studied regions, such as South Africa’s Cape, the Himalayas and the Amazon-Andes continuum, there are strong longitudinal gradients in biodiversity. Often, such gradients occur where high and low past climatic velocities are juxtaposed, and there is clear evidence of higher biodiversity at the climatically-stable end. Understanding longitudinal biodiversity variations more widely can offer new insights towards biodiversity conservation in the face of anthropogenic climatic change.
Biodiversity across latitude and longitude
Global patterns in biodiversity are described primarily in terms of latitude (Hillebrand, 2004; Worm and Tittensor, 2018). The comparative richness of tropical biotic assemblages was noticed early during European explorations in the tropics (Forster, 1778). Species numbers tend to decrease from the equator towards the poles in most groups of organisms (Fischer, 1960; Worm and Tittensor, 2018), as do some other biodiversity measures (Gratton et al., 2017; Daru et al., 2019). This pattern has been explained using present-day abiotic variables such as water-energy dynamics, historical climatic stability, biotic interactions, and various combinations and interactions thereof (Currie, 1991; Mittelbach et al., 2007; Schluter, 2016; Cannon et al., 2021). That this pattern is not a smooth one, but presents major anomalies, has also been long recognized (Hawkins and Diniz-Filho, 2004; Hillebrand, 2004). These anomalies have been studied in detail at the regional scale, and generally ascribed to peculiarities in precipitation, often linked to geomorphic features (e.g., Kidane et al., 2019; McDonald et al., 2021), although the specific ways in which these influence biodiversity often are region-specific, and thus hard to generalize. On a global scale, the most common approach has been to treat deviations from the general latitudinal patterns as outliers, and work towards minimising their contribution in global models which are still primarily focused on latitudinally-varying climatic measures (Huang et al., 2021). Such modeling exercises have indeed been able to reproduce global biodiversity patterns with great accuracy (Brummitt et al., 2021), although a handful of regions have remained notably recalcitrant in this respect.
Reducing global biodiversity patterns to latitude is no doubt a major oversimplification, and has created a number of problems (Hawkins and Diniz-Filho, 2004). In particular, the assumption that greater biodiversity in tropical regions is due to a combination of higher speciation and lower extinction has come under scrutiny. This assumption appears to be at least partly based on the coincidence of patterns derived from energy, and those derived from stability, across latitudes. While there is little doubt that Pleistocene glaciation cycles took their toll on polar and some temperate biotas, resulting in greater extinction risk at higher latitudes, speciation levels are not necessarily higher in the tropics, and in some groups are in fact lower (Dowle et al., 2013; Schluter, 2016; Igea and Tanentzap, 2020). This is reflected in patterns of phylogenetic diversity and ancient lineage diversity, which are often not well described as latitudinal gradients, and when they are, they can be the opposite of the regular species-based patterns, with values lower in the tropics compared to some temperate and subtropical regions (Procheş et al., 2015; Willig and Presley, 2018; Massante et al., 2019; McFadden et al., 2019). It also appears that, historically, a strong poleward reduction in species diversity was only recorded in the icehouse stages of Earth’s history (Mannion et al., 2014). Another factor not to be ignored is the role of land area distribution in latitudinal biodiversity patterns. The large extent of land in the tropics compared to southern temperate, and benign-climate northern temperate regions, is suggestive of a strong area effect in the tropical diversity peak (Jetz and Fine, 2012), and this pattern is compounded by the overrepresentation of mountainous areas – and hence higher ecological heterogeneity – in tropical regions (Körner, 2000). All these shortcomings call into question the frequently held assumption that the influence of climate on biodiversity is best described in terms of latitude. In particular, there is a need to decouple energy from stability as explanations of patterns of regional diversity, and the most obvious, albeit neglected, proxy variable to this effect is longitude.
A recent article (Cowling et al., 2017) has drawn attention to longitudinal patterns in species diversity as relevant, in that case, to South Africa’s Cape Floristic Region. This is not the very first mention of longitudinal biodiversity patterns, but it is one of the few to make this the central point of a study in an endemic-rich region – and one particularly hard to model (Colville et al., 2020). Climatic processes that are essentially longitudinal have long been mentioned in biogeography textbooks. Macarthur (1984) went to great lengths to explain the Coriolis effect, a fundamentally longitudinal process, to biogeography readers, but stopped short of translating this into a description of longitudinal biodiversity patterns. A reading of the few available works describing longitudinal gradients in biodiversity leads one to suspect that longitudinal patterns, far from being simply a small missing puzzle piece, are in fact essential drivers of major global patterns.
Here we undertake to briefly review this literature, seek common explanations of the patterns observed, and discuss possible implications for future theoretical research, but also climate-minded conservation. Our focus is on terrestrial systems, with examples from both plants and animals. While we are primarily looking for patterns with explanatory power at the regional scale, we will first characterize the longitudinal distribution of biodiversity worldwide in relation to the current (Cenozoic) tectonic and climatic patterns. We hypothesize that, as with latitudinal patterns, explanations for longitudinal patterns in biodiversity are to be found in both past and present climatic factors.
Global patterns, long-term processes
Unlike biodiversity measurements, which are likely to first reveal latitudinal differences, comparisons of biotic assemblages are a fascinating combination of longitudinal and latitudinal patterns, with longitudinal often clearer. In terms of primary regional units, a longitudinal separation is the main pattern for northern temperate biotas (Palearctic vs. Nearctic). Secondary divisions show substantial differences between the western and eastern sections in both Palearctic and Nearctic floras (Donoghue and Smith, 2004). Further south, equatorial rainforest assemblages are divided into three major floras and faunas (Neotropical, Congolean, Indo-Malay; Parmentier et al., 2007), with a minor fourth one in Madagascar. At intermediate latitudes, mediterranean-type biotas comprise two in the Northern and three in the Southern Hemisphere (Cowling et al., 2015), and warm deserts are found in both hemispheres on all continents (Goudie, 2002). These broad patterns in continental-level biome patterns are summarized in Figure 1.
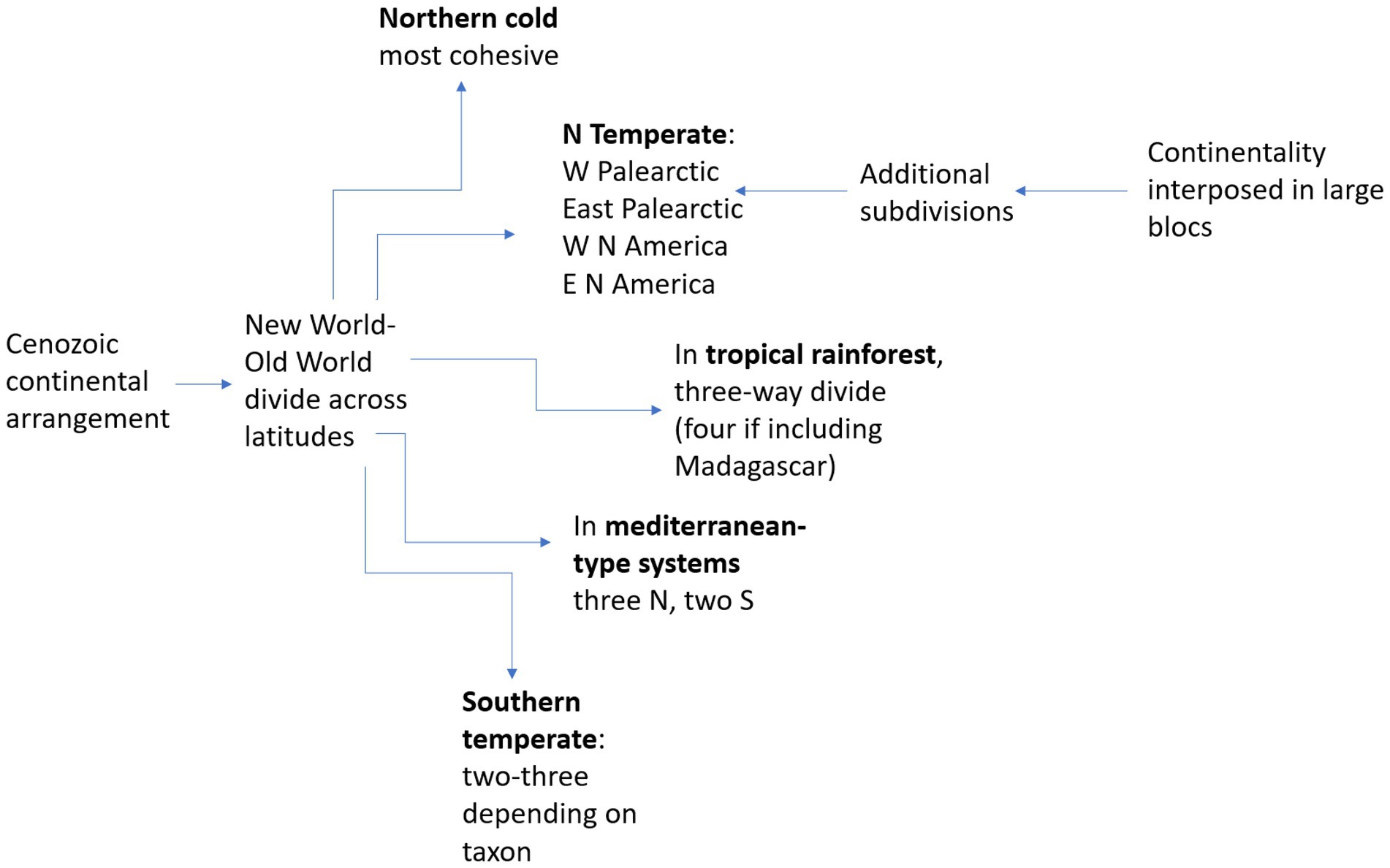
Figure 1. Regional-level longitudinal subdivisions (with numbers) in biotic assemblages derived from present-day continental arrangement and climatic patterns.
While most of these patterns can be predicted by simply analyzing continental shapes across latitudes in a mid-domain perspective (Jetz and Rahbek, 2001), biodiversity differences between regions requires a deeper understanding of present and past stability in terms of both climate and geomorphology. For example, in tropical rainforest biodiversity, the Neotropics stand out in terms of species diversity (Barthlott et al., 1996; Hawkins et al., 2007; Hagen et al., 2021), but Southeast Asia reigns in terms of higher-level phylogenetic diversity (Procheş et al., 2015) in both plants and vertebrates, while Africa is comparatively impoverished for both measures (Parmentier et al., 2007; Silva de Miranda et al., 2022). In contrast, for Mediterranean-climate systems, South Africa’s Cape is exceptionally diverse, which has been explained by a combination of relative climatic stability and topographic heterogeneity (Cowling et al., 2015). These differences and the associated variables suggest a way forward towards elucidating longitudinal variation at finer scales, within regions.
To grasp how these longitudinal differences came into being, one needs to summarise the geological events affecting climate, environments and biodiversity in a longitude-centred way. From the time that the Earth’s magnetosphere first protected this planet from the harmful solar wind (Tarduno et al., 2010), life on this planet has had to adapt to changes of a physico-chemical nature, but also some of a biological nature.
The physical changes have been due to extra-terrestrial factors, such as variations in solar radiation and orbital solar forcing of the Earth’s climate (Meyers and Malinverno, 2018; Lear et al., 2020), and to internal factors such as the cooling of the Earth by convection cells in the mantle, the rate of which may be faster than previously calculated (Murakami et al., 2022). Despite suggestions to the contrary, the Coriolis effect plays no significant role in mantle convection and orientation of continents (Ogawa, 2008). It is this convection that is the ultimate driving force of plate tectonics which has opened and closed ocean basins through time while molding the growth, shape, movement and geomorphology of the continents (Coltice et al., 2019).
Although slow in biological terms, the breakup, drifting and colliding of continents have not just changed the location of terrestrial environments but have also affected both oceanic and atmospheric circulation. This has played a fundamental role in the continuous change of physical environments and climate through time.
After the Permo-Triassic extinction 252 million years ago (Benton, 2018), Mesozoic terrestrial life was distributed across the supercontinent Pangea which comprised two halves: Laurasia in the north and Gondwana in the south. Separating these was the wedge-shaped ocean Tethys, tapering towards the west and opening up in the east into a global ocean (Scotese, 2014a). Laurasia began to break-up about 200 million years ago into North America and Eurasia (Marzoli et al., 1999) while slightly later Gondwana started to separate into Africa, South America, Madagascar and India-Australia-Antarctica (Watkeys, 2006). Eventually this process resulted in present distribution of the land masses: an east–west alignment in the Northern Hemisphere (Eurasia-North America) and three north–south alignments in the Southern Hemisphere (South America, Africa, Australasia) with Antarctica isolated at the South Pole (see Figure 2, maps).
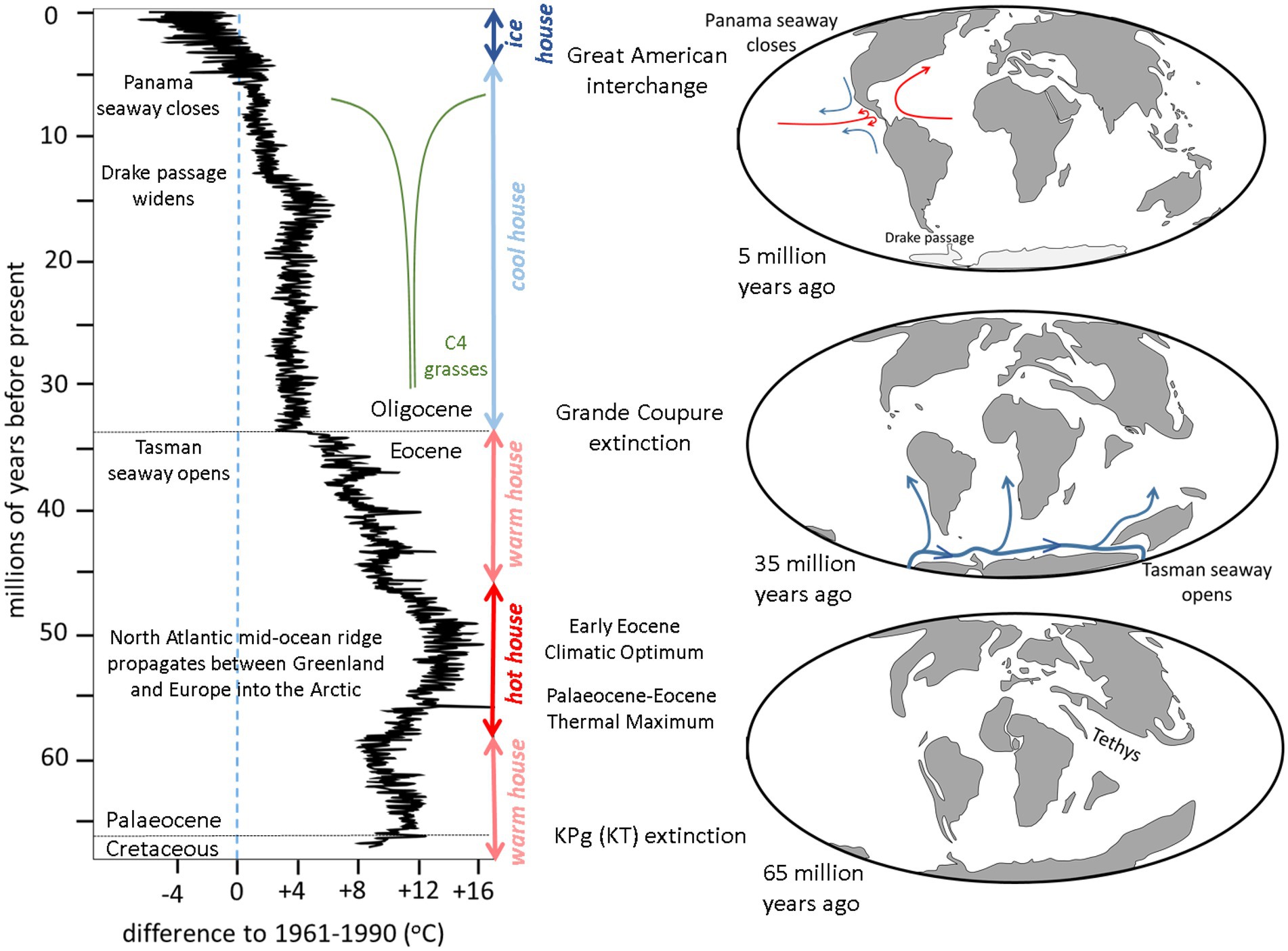
Figure 2. Summary of the changes in global temperatures (after Westerhold et al., 2020) and distribution of exposed land masses during the past 66 million years (after Scotese, 2022) relevant to latitudinal and longitudinal biodiversity patterns.
When Mesozoic life was devastated by the Cretaceous-Paleogene (K-Pg, formerly K-T) extinction 66 million years ago (Hildebrand et al., 1991), all the continents were isolated within oceans (Figure 2, bottom right map). Furthermore, all the low-lying continental areas had been extensively flooded by marine waters due to high sea levels caused by fast spreading mid-oceanic ridges (Seton et al., 2009). In the early Cenozoic the remnants of the Mesozoic terrestrial ecosystems that survived the K-Pg extinction evolved independently on each isolated continent. An exception was Africa, which maintained a tenuous connection with Eurasia via Iberia (Scotese, 2014b).
Since the K-Pg extinction, the Earth’s climate has gone from a warm house to a hot house, back to a warm house, then a cool house and into the present ice house (Figure 2, graph on the left after Westerhold et al., 2020). Each of these changes relate to geological processes, one of which was a critical control on longitudinal biogeographic distribution.
After the K-Pg extinction the Earth’s temperature progressively increased from a warm house to a hot house. The broad overall increase was a consequence of appearance of the Iceland hot spot that caused the mid-Atlantic Ridge to propagate northwards between Greenland and Europe into the Arctic (Srivastava and Tapscott, 1986). This resulted in the release of frozen methane hydrates on the Arctic continental shelf causing Early Eocene Climatic Optimum (Storey et al., 2007). During this warming there was a spike in temperature, the Palaeocene-Eocene Thermal Maximum, caused by orbital forcing of the Earth’s climate (Li et al., 2022).
There then followed was a progressive return to a warm house during the Eocene and then a sharp drop in global temperature at the Eocene–Oligocene boundary to cool house conditons. This was due the collision of India with Asia changing plate motion (Torsvik et al., 2017), causing the separation of Australia from Antarctica to produce the Tasman seaway (van den Ende et al., 2017; Figure 2, right middle map). This allowed a cold circum-polar current to encircle Antarctica triggering the onset of the glaciation that has lasted to the present day. It also cooled the northerly flowing currents on the west side of the southern hemisphere continents which caused desiccation of the adjacent terrestrial environments, loss of soil and the development of deserts (Van Zinderen Bakker, 1975). It was this event that produced the marked longitudinal contrast of environments across continents at the same latitude.
It was also responsible for the rapid global cooling that caused the Grande Coupure extinction in Europe (Ivany et al., 2000) and its equivalents in Asia (Meng and McKenna, 1998) and Africa (de Vries et al., 2021). This is the time of the first appearance of C4 grasses that were to have a profound effect on terrestrial ecosystems, particularly from about 7 million years ago (Bouchenak Khelladi et al., 2009).
The cool house of the Oligocene started to warm slightly in the Miocene (O’Brien et al., 2020) before cooling again at around 15 million years ago due to the increase in in volume of circum-Antarctic current because of widening of Drake Passage (Livermore et al., 2007). This cooling continued when the Panama seaway was closed around 5 million years ago when the volcanic island arc between North and South America developed into an isthmus (Coates et al., 2004; Figure 2, top right map). This shut down the flow between the central Pacific and central Atlantic, possibly initiating the El Niño-Southern Oscillation (ENSO), and diverted North Atlantic Deep Water around the southern margin of Africa (Ovechkina et al., 2021). The Central America isthmus enabled the Great American Interchange to take place (Woodburne, 2010). Meanwhile, on the other side of the globe, the continuing movement of India northwards into Asia caused uplift of the Himalayas that precipitated the monsoonal rains that flushed increased amounts of CO2 out of the atmosphere causing the planet to cool further into an ice house (Tada et al., 2016).
Thus, unlike the Mesozoic world, where the main biogeographic division was along latitudinal lines (Laurasia in the north vs. Gondwana in the south), today we see primarily longitudinal biogeographic differences (Procheş, 2006).
Continentality in temperate systems
The early works examining latitudinal variation in biodiversity in Northern Hemisphere systems were primarily in endemic-poor regions, and found it to be linked most often to area / mid-domain / peninsula effects in butterflies and vertebrates (Järvinen, 1978; Martin and Gurrea, 1990; Danell et al., 1996). Based on the low endemism, these areas exhibited low if any speciation, but presumably substantial extinction throughout the study regions. Even so, some parts of these regions would have experienced higher extinction than others, resulting in extinction patterns arranged along longitudinal gradients.
More interesting from an endemism perspective, among the temperate Northern Hemisphere studies, is the work on the floras of eastern North America and eastern Asia (e.g., Qian et al., 2005, 2017). Overall, East Asia has a vastly more complex topography, and more diverse climates – and accordingly higher plant diversity (species and phylogenetic) and endemism. The very separation of eastern and western assemblages in both Eurasia and North America is due to the interposition of low diversity central regions, derived from a continentality effect (Donoghue and Smith, 2004). The onset of this interposition has been mapped across geological time, for example in the case of Tibet (He et al., 2020). Nevertheless, the species-poorer continental assemblages of these northern continents have their fair share of endemics (Copeland and Harrison, 2015).
Mediterranean and tropical systems
Perhaps the most detailed illustration of a longitudinal biodiversity gradient across multiple plant lineages comes from mediterranean-climate regions. This is best seen in the Cape Floristic Region, and the pattern has been named Levyns’ Law, after Margaret Levyns, who first documented it (Levyns, 1938; Cowling et al., 2017). This pattern of decreasing plant diversity and endemism from west to east is best explained by higher stability in terms of climate and biome distribution during the Pleistocene, but is also well matched by present-day patterns in rainfall seasonality (winter rainfall) and reliability. This is the case both in typical mediterranean-climate areas, with substantial winter rainfall (Cowling et al., 2017; Colville et al., 2020) and in arid vegetation further north, where rainfall is a reliable winter occurrence too, albeit much reduced (Cowling et al., 1999). A similar pattern, specifically explained by lower extinction rates at the Oligocene–Eocene boundary in the west compared to the east, is illustrated for the mediterranean-type flora of Australia (Nge et al., 2020). However, these west-to-east Cape and Australian gradients of decreasing diversity are based on the diversity of the dominant typical mediterranean-climate plants, which are most diverse in these regions – shrubby, fire-adapted, and more often than not re-seeders, that are killed by fire and regenerate from seed. Conversely, plants characteristic of forests, such as trees, are more diverse in the eastern parts of southern Africa, where summer rainfall is dominant (O’Brien and Peters, 1998; Keil and Chase, 2019). Further north, this pattern is matched by higher tree genetic diversity in the eastern parts of the Mediterranean Basin (Fady and Conord, 2010).
Elsewhere, and in a different climatic setting, a well-documented longitudinal gradient is that in species richness and nestedness in Himalayan vertebrates (Srinivasan et al., 2014), with most endemics found in the east, and the impoverished western assemblages nested within richer, eastern ones. Again, this is best explained by past climate, as the western Himalayas were more extensively glaciated at the last maximum (Yan et al., 2020).
Both of these examples thus make a case for climatic stability as a driver of longitudinal patterns in species richness. Apparently contradicting this is Amazonia (Hoorn et al., 2010), where higher diversity and endemism in the west have been linked to the Andean uplift via a number of processes involving topographic heterogeneity and climate, but perhaps even more so nutrient enrichment. This needs not contradict the South African and Himalayan examples, but more likely builds a case for maximum diversification being associated with orogenesis and intermediate levels of stability in the long term, which can nevertheless be viewed as highly stable over the intermediate time scales most relevant to speciation and extinction. This has in fact been advocated in mediterranean climates (Cowling et al., 2015) as well as the Himalayas (Favre et al., 2014; Xu et al., 2021).
Other patterns on global maps
The Amazonian and Himalayan examples, with diversity/endemism increasing in the west and east, respectively, can also be documented with patterns from global and broad regional studies on diverse taxa such as plants (Barthlott et al., 1996; McFadden et al., 2019), vertebrates (Hawkins et al., 2007; Parmentier et al., 2007) and fungi (Tedersoo et al., 2014). The Cape gradient, with species diversity peaking in the west, is only noted in plants, as dominated by herbaceous and shrubby species (Barthlott et al., 1996); fungi and most large-bodied animal groups show the opposite pattern here, in line with trees (Hawkins et al., 2007; Tedersoo et al., 2014; Keil and Chase, 2019). The aforementioned global maps also illustrate continentality effects, with lower diversity values in the central parts of North America and Eurasia, compared to the east and west coasts. In these cases, endemism is proportionally higher close to the coastlines, and thus, all-in-all, nestedness outweighs turnover. By comparison, in the Mediterranean-climate and Andean cases, endemism is typically high (not just in absolute terms, but also proportionally, in relation to total species richness) even towards the species-poor end of the gradient, meaning that an important turnover component is present (Colville et al., 2020). It has to be noted though that, at least for plants, there is no comprehensive global mapping exercise with fine-enough scale data to back up this statement across all the regions discussed here.
Sharp decreases in diversity from the coast inland are also seen in many other regions besides those detailed above, as described in dedicated studies. Particularly abrupt changes characterise west coasts in the Mediterranean climates of California, Chile, but also further polewards, where Mediterranean vegetation is replaced by temperate rainforest. Inland, both Mediterranean vegetation and temperate rainforest are replaced by grasslands or arid systems, in most places the transition happening across high mountain chains. Strong decreases inland also are found on east coasts, such as in Madagascar and Queensland, where coastal tropical rainforest is replaced inland by savanna or thickets (Barthlott et al., 1996; Hawkins et al., 2007; Tedersoo et al., 2014).
Explanations
Based on the examples listed above, the factors potentially involved in explaining longitudinal gradients have to do with climate, tectonics and geomorphology – present and past. As mentioned, past orogenic events, such as the Andean uplift (Hoorn et al., 2010), have been essential in promoting the accumulation of biological diversity. Past continental movements have certainly also played a role in shaping present-day diversity patterns. One such example is Australia’s rapid movement across latitudes during the Cenozoic, meaning that the southwestern part of that continent has enjoyed a proper Mediterranean climate for much shorter the South Africa’s Cape, which partly explains the differences in plant diversity between the two regions, but also the shaping of the longitudinal gradients with each region (Cowling et al., 2015; Nge et al., 2020). Despite these important roles of orogeny and tectonics, climate remains probably the single most important factor in the context of our discussion, and we will discuss how climatic patterns correlate with the occurrence of strong gradients, while also pointing out its interactions with topography. We argue that the two key factors which commonly vary longitudinally and have the most profound impacts on biodiversity, are present climate (best represented by inter-annual climatic variability) and past climate (best described in terms of long-term climatic stability).
Present climate
In a present climate context, decreases in diversity and endemism, both associated with biome transitions and within a single biome, are most often found in coastal areas where air circulation from the sea brings in more abundant and/or more reliable rainfall close to the coast, but precipitation, or the reliability thereof, decreases inland (Barthlott et al., 1996; Hawkins et al., 2007; McFadden et al., 2019). This is most pronounced where precipitation-bearing air masses meet perpendicular mountain ranges, e.g., where westerly tropical air circulation or easterly temperate circulation meet north–south trending mountain ranges (Hoorn et al., 2010; Baldwin et al., 2017; Colville et al., 2020). Often, coastal mountain ranges stop precipitation from reaching inland, but in the case of the tropical Andes much of the circulation is easterly, most of the continent at that latitude is humid, and only the western coastal and mountainous section are arid (Hoorn et al., 2010). The most predictable patterns remain those in Mediterranean climates, always associated with westerlies (Donoghue and Smith, 2004; Cowling et al., 2017). Even in the tropics, the presence of gradients (in either direction!) seems to be more often associated with the predominance of westerlies (Figure 3), although often these are cases characterized by either very low velocities, or with substantial longitudinal components (Oliver, 1987; Hu et al., 2020).
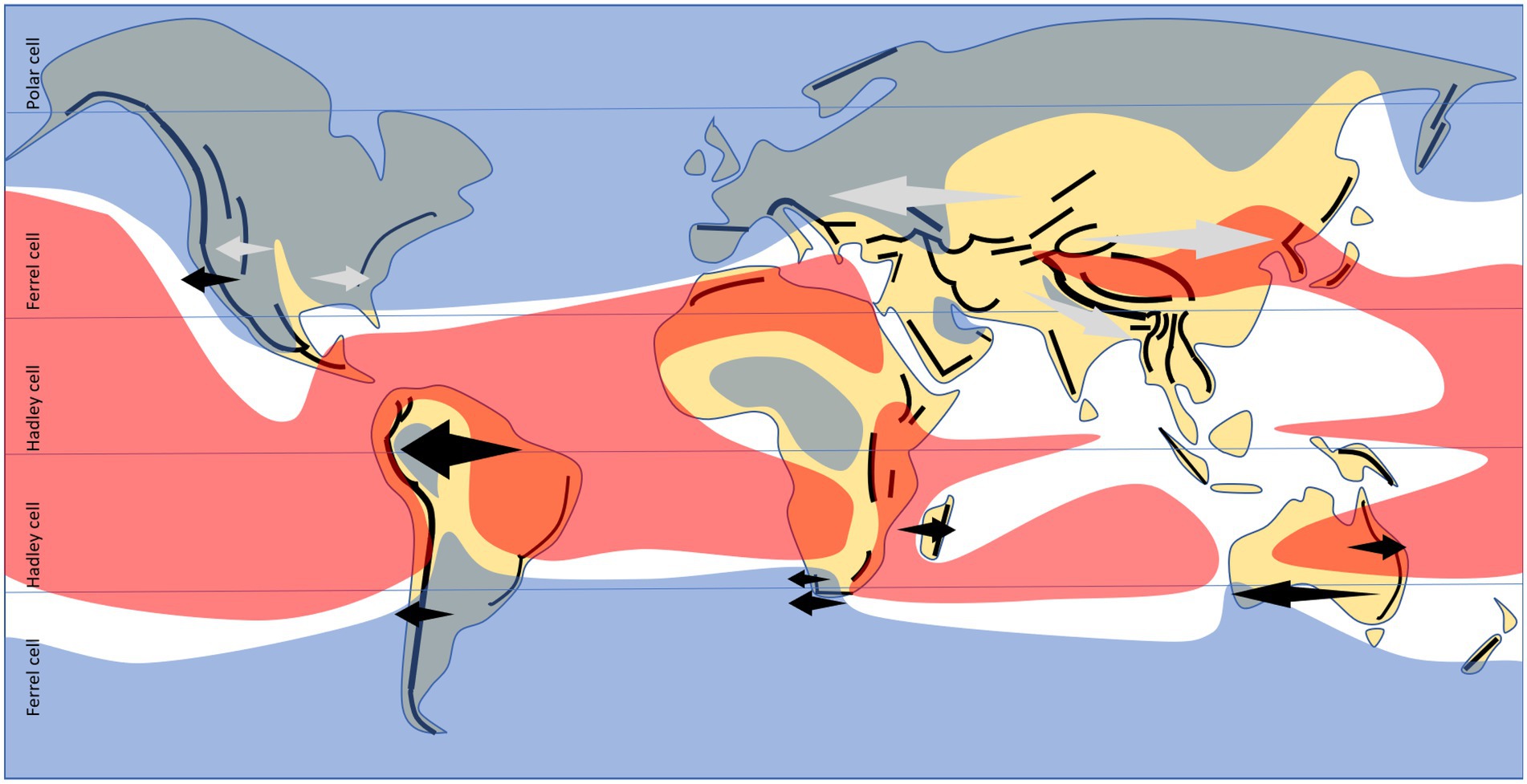
Figure 3. Documented longitudinal gradients in species diversity and endemism (arrows), as mentioned in the text, in relation to present-day continental arrangement, major mountain chains, and predominant direction of tropospheric circulation (westerlies in blue, easterlies in red, otherwise seasonally-varying (Oliver, 1987; Hu et al., 2020); horizontal lines indicate limits between tropical Hadley atmospheric cells – mostly easterlies, and temperate Ferrel cells – mostly westerlies). Black arrows indicate gradients with a strong endemism component, involving both nestedness and turnover; grey (continentality) patterns are mostly nestedness-driven.
Besides the zonal circulation detailed above, which seems to be the leading atmospheric component in terms of longitudinal gradient effects, one should not discard other components such as meridional and vertical circulation. In particular, multi-year climatic cycles such as ENSO have unevenly distributed effects across and within regions. Apart from affecting Pacific coasts more intensely than other continents, this recurring climatic pattern also amplifies the effects derived from the average zonal circulation, as seen in southern Africa, with variations in ocean-land interactions that have different effects on the eastern vs. western coastlines (Meuser et al., 2013).
It appears that the failure of global models to comprehensively explain biodiversity in all regions has to do with region-specific climatic variables. For example, a recent study (Antonelli et al., 2018) found that seasonality has no predictive value for the world as a whole, although it does have a clear value in some regions (while keeping in mind the use of analytically-defined regions (Coops et al., 2018) could have improved prediction power).
Based on these aspects of present climate, we predict that biodiversity gradients will be stronger in places where air circulation meets mountain ranges with high ranges, which are not only barriers to dispersal, but are more so in the tropics, where biodiversity is higher and the study of gradients based on endemism is stronger (Ghalambor et al., 2006). ENSO could theoretically impact biodiversity both negatively and positively, via an intermediate disturbance effect (Connell, 1978).
Past climate
Climatic fluctuations over evolutionary time scales have profoundly influenced global patterns of biodiversity (Rosenzweig, 1995; Jansson, 2003), stimulating diversification where changes are sufficiently muted to prevent the extinction of resident lineages (Hewitt, 2004), and reducing it where large climatic changes result in the mass extinction of lineages, as has occurred in high-latitude regions of the Northern Hemisphere during Pleistocene glacials (Jansson and Dynesius, 2002). Indeed, areas of the globe that have experienced mild Pleistocene climate fluctuations are invariably associated with taxonomically and phylogenetically diverse and endemic-rich biotas, irrespective of latitude (McGlone, 1996; Sandel et al., 2011; Harrison and Noss, 2017; Colville et al., 2020).
Perhaps the most important opportunity provided by longitudinal gradients is to decouple variables reflecting climate stability from those associated with water-energy or productivity (Cowling et al., 2017). The increase of both productivity and Pleistocene climate stability with latitude – a pattern especially pronounced in the Northern Hemisphere - confounds attempts to independently test hypotheses related to historical and contemporary climate processes for driving biodiversity patterns (Jetz and Fine, 2012). While the vast tropical regions of the world are home to much of its biodiversity, there are significant nodes of high diversity in temperate regions, especially in the Southern Hemisphere (Dubey and Shine, 2011; Sandel et al., 2011). These cases refute the generality of high water-energy dynamics as a determinant of biodiversity (Currie, 1991; O’Brien, 2006); thus, a general theory of global biodiversity patterns must accommodate such cases, thus far treated as exceptions (Fine, 2015). The study of longitudinal gradients is key to developing a more general theory of biodiversity than those currently invoked, ones that considers both historical and contemporary drivers independently (Cowling et al., 2017). Patterns reviewed here suggest that relative Pleistocene climate stability combined with high habitat heterogeneity can explain a large proportion of the global variation in phylogenetic diversity and turnover (Srinivasan et al., 2014; Cowling et al., 2015; Colville et al., 2020). On a shorter time scale, variations in climatic cycles can also impact biodiversity. For example, in southern Africa there is a connection between the strength of El Niño events over geological history and shifts in the boundary between the summer and winter rainfall regions, with the areas that shift from one regime to the other having lower long-term stability (Meuser et al., 2013).
Long-term climatic stability is commonly described in terms of its inverse measure, climatic velocity. In terms of past climates, sharp longitudinal biodiversity gradients are often located in regions where low and high past climatic velocities are juxtaposed (Sandel et al., 2011; Harrison and Noss, 2017). Long-term climatic stability can be achieved via geographic stability of the tectonic plates, thus avoiding major latitudinal movements, in combination with a more or less constant pattern of atmospheric circulation, as was the case in the Cape (Jansson and Dynesius, 2002), or buffering through, e.g., multiple monsoon systems, as in the eastern Himalayas (Spicer, 2017). Recent studies modeling upcoming climatic change tend to suggest such buffering will continue to function in the future (Tangang et al., 2020), although this will require confirmation for other regions and scenarios.
Concluding remarks
In conclusion, there are good theoretical reasons to expect that longitude can be a powerful variable for understanding regional and global biodiversity patterns. Globally, longitude is critical in defining the regional subdivisions of the Cenozoic world. However, within regions, an appraisal of the importance of longitude has only been achieved partly and then only for regions where substantial work on present and past climate and geomorphology has been conducted. Illustrating changing longitudinal biodiversity over geological time has the potential to clarify some of the complexities associated with the biotic impacts of contemporary climate change.
The mechanisms linking longitude and biodiversity may be more complex than those relevant to latitude effects – but this does not make them any less important. While longitude does help solve the energy vs. stability puzzle persistent in latitudinal patterns – and stability is the winner, longitude comes with its own puzzle. Understanding longitudinal gradients is to a great extent about disentangling the effects of (a) current and (b) past environmental factors, which can both be invoked to explain biodiversity variation along various unidimensional gradients (Fitzpatrick et al., 2013) and are often correlated. The relative importance of the two may vary from taxon to taxon depending on dispersal abilities (Graham et al., 2006).
Our results have clear implications relating to climate change preparedness. While areas with high biodiversity due to past stability are more often than not likely to be more climatically-stable in the future too, their conservation status needs to be reassessed having in mind the potential shifting of biodiversity gradients (Chase et al., 2019), in conjunction with habitat fragmentation. Longitudinal, together with elevational, gradients, are more likely than latitudinal gradients to be relevant to medium- and fine-scale climatic variability, which, in conjunction with temporal climatic variability, in turn showing specific longitudinal patterns, is key to predicting species’ responses to climate change (Nadeau et al., 2017; Freeman et al., 2018; Kling and Ackerly, 2020). It is of particular concern that regions with strong longitudinal gradients, such as Mediterranean-climate regions and tropical mountains, are overall likely to be more severely impacted by land-use and climatic changes (Newbold et al., 2020), and are likely to be hit by abrupt ecological disruption sooner than other regions (Trisos et al., 2020). Key types of information on biodiversity responses to climate change, which are well studied in temperate regions, are poorly understood in the tropics (Sheldon, 2019).
We recommend that the use of established (Chevalier et al., 2020) and innovative (Chase et al., 2019) methods for understanding past climates be employed along longitudinal gradients, towards an improved theoretical framework aimed at conservation in the face of contemporary anthropogenic climate change. Based on our summary, biodiversity modelling can use new indices combining distance from key regional features such as coastlines, and topography, as proxies for longitudinal biodiversity gradients. Presently, planning for conservation in the face of climate change is happening at local, regional, and global scales (Harrison and Noss, 2017; Olazabal et al., 2019; McDonald et al., 2021). Our summary of results to date highlights the importance of the regional scale, although more cross-scale studies are needed to confirm this. If confirmed, it could prove vital to delimit natural regions analytically on climatic and ecological criteria (Coops et al., 2018) rather than intuitive biotic regions or political boundaries, although the latter remain of course essential in an implementation perspective.
Author contributions
RC and ŞP conceived the ideas for this study. All authors contributed to the article and approved the submitted version.
Conflict of interest
The authors declare that the research was conducted in the absence of any commercial or financial relationships that could be construed as a potential conflict of interest.
Publisher’s note
All claims expressed in this article are solely those of the authors and do not necessarily represent those of their affiliated organizations, or those of the publisher, the editors and the reviewers. Any product that may be evaluated in this article, or claim that may be made by its manufacturer, is not guaranteed or endorsed by the publisher.
References
Antonelli, A., Kissling, W. D., Flantua, S. G. A., Bermúdez, M. A., Mulch, A., Muellner-Riehl, A. N., et al. (2018). Geological and climatic influences on mountain biodiversity. Nat. Geosci. 11, 718–725. doi: 10.1038/s41561-018-0236-z
Baldwin, B. G., Thornhill, A. H., Freyman, W. A., Ackerly, D. D., Kling, M. M., Morueta-Holme, M., et al. (2017). Species richness and endemism in the native flora of California. Am. J. Bot. 104, 487–501. doi: 10.3732/ajb.1600326
Barthlott, W., Lauer, W., and Placke, A. (1996). Global distribution of species diversity in vascular plants: towards a world map of phytodiversity. Erdkunde 50, 317–327. doi: 10.3112/erdkunde.1996.04.03
Benton, M. J. (2018). Hyperthermal-driven mass extinctions: killing models during the Permian-Triassic mass extinction. Phil. Trans. R. Soc. A 376:20170076. doi: 10.1098/rsta.2017.0076
Bouchenak Khelladi, Y., Verboom, G. A., and Hodkinson, T. R. (2009). The origins and diversification of C4 grasses and savanna-adapted ungulates. Glob. Chang. Biol. 15, 2397–2417. doi: 10.1111/j.1365-2486.2009.01860.x
Brummitt, N., Araújo, A. C., and Harris, T. (2021). Areas of plant diversity—what do we know? Plants People Planet 3, 33–44. doi: 10.1002/ppp3.10110
Cannon, P. G., Edwards, D. P., and Freckleton, R. P. (2021). Asking the wrong question in explaining tropical diversity. Trends Ecol. Evol. 36, 482–484. doi: 10.1016/j.tree.2021.02.011
Chase, B. M., Niedermeyer, E. M., Boom, A., Carr, A. S., Chevalier, M., He, F., et al. (2019). Orbital controls on Namib Desert hydroclimate over the past 50,000 years. Geology 47, 867–871. doi: 10.1130/G46334.1
Chevalier, M., Davis, B. A. S., Heiri, O., Seppä, H., Chase, B. M., Gajewski, K., et al. (2020). Pollen-based climate reconstruction techniques for late quaternary studies. Earth Sci. Rev. 210:103384. doi: 10.1016/j.earscirev.2020.103384
Coates, A. G., Colling, L. S., Aubry, M.-P., and Berggren, W. A. (2004). The geology of the Darien, Panama, and the Miocene–Pliocene collision of the Panama arc with northwestern South America. Geol. Soc. Am. Bull. 116, 1327–1344. doi: 10.1130/B25275.1
Coltice, N., Husson, L., Faccenna, C., and Arnould, M. (2019). What drives tectonic plates? Sci. Adv. 5:eaax4295. doi: 10.1126/sciadv.aax4295
Colville, J. F., Beale, C. M., Forest, F., and Cowling, R. M. (2020). Plant richness, turnover and evolutionary diversity track gradients of stability and ecological opportunity in a megadiversity Centre. Proc. Natl. Acad. Sci. U. S. A. 117, 20027–20037. doi: 10.1073/pnas.1915646117
Connell, J. H. (1978). Diversity in tropical rain forests and coral reefs. Science 199, 1302–1310. doi: 10.1126/science.199.4335.1302
Coops, N. C., Kearney, S. P., Bolton, D. K., and Radeloff, V. C. (2018). Remotely-sensed productivity clusters capture global biodiversity patterns. Sci. Rep. 8:16261. doi: 10.1038/s41598-018-34162-8
Copeland, S. M., and Harrison, S. P. (2015). Identifying plant traits associated with topographic contrasts in a rugged and diverse region (Klamath-Siskiyou Mts, OR, USA). Ecography 38, 569–577. doi: 10.1111/ecog.00802
Cowling, R. M., Bradshaw, P. L., Colville, J. F., and Forest, F. (2017). Levyns’ law: explaining the evolution of a remarkable longitudinal gradient in cape plant diversity. Trans. R. Soc. S. Afr. 72, 184–201. doi: 10.1080/0035919X.2016.1274277
Cowling, R. M., Esler, K. J., and Rundel, P. W. (1999). Namaqualand, South Africa—an overview of a unique winter-rainfall desert ecosystem. Plant Ecol. 142, 3–21. doi: 10.1023/A:1009831308074
Cowling, R. M., Potts, A. J., Bradshaw, P. L., Colville, J. F., Arianoutsou, M., Ferrier, S., et al. (2015). Variation in plant diversity in mediterranean-climate ecosystems: the role of climatic and topographical stability. J. Biogeogr. 42, 552–564. doi: 10.1111/jbi.12429
Currie, D. J. (1991). Energy and large-scale patterns of animal– and plant–species richness. Am. Nat. 137, 27–49. doi: 10.1086/285144
Danell, K., Lundberg, P., and Niemela, P. (1996). Species richness in mammalian herbivores: patterns in the boreal zone. Ecography 19, 404–409. doi: 10.1111/j.1600-0587.1996.tb00251.x
Daru, B. H., le Roux, P. C., Gopalraj, J., Park, D. S., Holt, B. G., and Greve, M. (2019). Spatial overlaps between the global protected areas network and terrestrial hotspots of evolutionary diversity. Glob. Ecol. Biogeogr. 28, 757–766. doi: 10.1111/geb.12888
de Vries, D., Heritage, S., Borths, M. R., Sallam, H. M., and Seiffert, E. R. (2021). Widespread loss of mammalian lineage and dietary diversity in the early Oligocene of afro-Arabia. Commun. Biol. 4:1172. doi: 10.1038/s42003-021-02707-9
Donoghue, M. J., and Smith, S. A. (2004). Patterns in the assembly of temperate forests around the northern hemisphere. Philos. Trans. R. Soc. B 359, 1633–1644. doi: 10.1098/rstb.2004.1538
Dowle, E. J., Morgan-Richards, M., and Trewick, S. A. (2013). Molecular evolution and the latitudinal biodiversity gradient. Heredity 110, 501–510. doi: 10.1038/hdy.2013.4
Dubey, S., and Shine, R. (2011). Geographic variation in the age of temperate–zone reptile and amphibian species: southern hemisphere species are older. Biol. Lett. 7, 96–97. doi: 10.1098/rsbl.2010.0557
Fady, B., and Conord, C. (2010). Macroecological patterns of species and genetic diversity in vascular plants of the Mediterranean basin. Divers. Distrib. 16, 53–64. doi: 10.1111/j.1472-4642.2009.00621.x
Favre, A., Päckert, M., Pauls, S. U., Jähnig, S. C., Uhl, D., Michalak, I., et al. (2014). The role of the uplift of the Qinghai–Tibetan plateau for the evolution of Tibetan biotas. Biol. Rev. 90, 236–253. doi: 10.1111/brv.12107
Fine, P. V. A. (2015). Ecological and evolutionary drivers of geographic variation in species diversity. Ann. Rev. Ecol. Evol. Syst. 46, 369–392. doi: 10.1146/annurev-ecolsys-112414-054102
Fischer, A. G. (1960). Latitudinal variation in organic diversity. Evolution 14, 64–81. doi: 10.2307/2405923
Fitzpatrick, M. C., Sanders, N. J., Normand, S., Svenning, J.-C., Ferrier, S., Gove, A. D., et al. (2013). Environmental and historical imprints on beta diversity: insights from variation in rates of species turnover along gradients. Proc. R. Soc. B 280:20131201. doi: 10.1098/rspb.2013.1201
Forster, J. R. (1778). Observations made during a voyage round the world, on physical geography, natural history, and ethic philosophy. In: Foundations of Biogeography (Eds.) by M. V. Lomolino, D. F. Sax, and J. H. Brown)., pp. 19–27. University of Chicago Press, Chicago, IL.
Freeman, B. G., Scholer, M. N., Ruiz-Gutierrez, V., and Fitzpatrick, J. W. (2018). Climate change causes upslope shifts and mountaintop extirpations in a tropical bird community. Proce. Natl. Acad. Sci. U. S. A 115, 11982–11987. doi: 10.1073/pnas.1804224115
Ghalambor, C. K., Huey, R. B., Martin, P. R., Tewksbury, J. J., and Wang, G. (2006). Are mountain passes higher in the tropics? Janzen’s hypothesis revisited. Integr. Comp. Biol. 46, 5–17. doi: 10.1093/icb/icj003
Goudie, A. (2002). Great Warm Deserts of the World: Landscapes and Evolution, Oxford University Press, Oxford, UK.
Graham, C. H., Moritz, C., and Williams, S. E. (2006). Habitat history improves prediction of biodiversity in rainforest fauna. Proce. Natl. Acad. Sci. U. S. A. 103, 632–636. doi: 10.1073/pnas.0505754103
Gratton, P., Marta, S., Bocksberger, G., Winter, M., Keil, P., Trucchi, E., et al. (2017). Which latitudinal gradients for genetic diversity? Trends Ecol. Evol. 32, 724–726. doi: 10.1016/j.tree.2017.07.007
Hagen, O., Skeels, A., Onstein, R. E., Jetz, W., and Pellissier, L. (2021). Earth history events shaped the evolution of uneven biodiversity across tropical moist forests. Proce. Natl. Acad. Sci. U. S. A. 118:e2026347118. doi: 10.1073/pnas.2026347118
Harrison, S., and Noss, R. (2017). Endemism hotspots are linked to stable climatic refugia. Ann. Bot. 119, 207–214. doi: 10.1093/aob/mcw248
Hawkins, B. A., and Diniz-Filho, J. A. (2004). 'Latitude' and geographic patterns in species richness. Ecography 27, 268–272. doi: 10.1111/j.0906-7590.2004.03883.x
Hawkins, B. A., Diniz-Filho, J. A. F., Jaramillo, C. A., and Soeller, S. A. (2007). Climate, niche conservatism, and the global bird diversity gradient. Am. Nat. 170, S16–S27. doi: 10.1086/519009
He, J.-K., Lin, S.-L., Li, J.-T., Yu, J.-H., and Jiang, H.-S. (2020). Evolutionary history of zoogeographical regions surrounding the Tibetan plateau. Commun. Biol. 3:415. doi: 10.1038/s42003-020-01154-2
Hewitt, G. (2004). Genetic consequences of climatic oscillations in the quaternary. Philos. Trans. R. Soc. B 359, 183–195. doi: 10.1098/rstb.2003.1388
Hildebrand, A. R., Penfield, G. T., Kring, D. A., Pilkington, M., Zanoguera, A. C., Jacobsen, S. B., et al. (1991). Chicxulub crater; a possible cretaceous/tertiary boundary impact crater on the Yucatan peninsula, Mexico. Geology 19, 867–871. doi: 10.1130/0091-7613(1991)019<0867:CCAPCT>2.3.CO;2
Hillebrand, H. (2004). On the generality of the latitudinal diversity gradient. Am. Nat. 163, 192–211. doi: 10.1086/381004
Hoorn, C., Wesselingh, F. P., Ter Steege, H., Bermudez, M. A., Mora, A., Sevink, J., et al. (2010). Amazonia through time: Andean uplift, climate change, landscape evolution, and biodiversity. Science 330, 927–931. doi: 10.1126/science.1194585
Hu, S.-J., Zhou, B.-Q., Gao, C.-B., Xu, Z.-H., Wang, Q.-W., and Chou, J.-F. (2020). Theory of three–pattern decomposition of global atmospheric circulation. Sci. China Earth Sci. 63, 1248–1267. doi: 10.1007/s11430-019-9614-y
Huang, E.-H., Chen, Y.-X., Fang, M., Zheng, Y., and Yu, S.-X. (2021). Environmental drivers of plant distributions at global and regional scales. Glob. Ecol. Biogeogr. 30, 697–709. doi: 10.1111/geb.13251
Igea, J., and Tanentzap, A. J. (2020). Angiosperm speciation cools down in the tropics. Ecol. Lett. 23, 692–700. doi: 10.1111/ele.13476
Ivany, L. C., Patterson, W. P., and Lohmann, K. C. (2000). Cooler winters as a possible cause of mass extinctions at the Eocene/Oligocene boundary. Nature 407, 887–890. doi: 10.1038/35038044
Jansson, R. (2003). Global patterns in endemism explained by past climatic change. Proc. R. Soc. B 270, 583–590. doi: 10.1098/rspb.2002.2283
Jansson, R., and Dynesius, M. (2002). The fate of clades in a world of recurrent climate change: Milankovitch oscillations and evolution. Ann. Rev. Ecol. Evol. Syst. 33, 741–777. doi: 10.1146/annurev.ecolsys.33.010802.150520
Järvinen, O. (1978). Species richness of small mammals in Finland. Oikos 31, 253–256. doi: 10.2307/3543570
Jetz, W., and Fine, P. V. A. (2012). Global gradients in vertebrate diversity predicted by historical area-productivity dynamics and contemporary environment. PLoS Biol. 10:e1001292. doi: 10.1371/journal.pbio.1001292
Jetz, W., and Rahbek, C. (2001). Geometric constraints explain much of the species richness pattern in African birds. Proce. Natl. Acad. Sci. U. S. A. 98, 5661–5666. doi: 10.1073/pnas.091100998
Keil, P., and Chase, J. M. (2019). Global patterns and drivers of tree diversity integrated across a continuum of spatial grains. Nat. Ecol. Evol. 3, 390–399. doi: 10.1038/s41559-019-0799-0
Kidane, Y. O., Steinbauer, M. J., and Beierkuhnlein, C. (2019). Dead end for endemic plant species? A biodiversity hotspot under pressure. Glob. Ecol. Conserv. 19:e00670. doi: 10.1016/j.gecco.2019.e00670
Kling, M. D., and Ackerly, D. D. (2020). Global wind patterns and the vulnerability of wind-dispersed species to climate change. Nat. Clim. Chang. 10, 868–875. doi: 10.1038/s41558-020-0848-3
Körner, C. (2000). Why are there global gradients in species richness? Mountains might hold the answer. Trends Ecol. Evol. 15, 513–514. doi: 10.1016/S0169-5347(00)02004-8
Lear, C. H., Anand, P., Blenkinsop, T., Foster, G. L., Gagen, M., Hoogakker, B., et al. (2020). Geological Society of London scientific statement: what the geological record tells us about our present and future climate. J. Geol. Soc. 178:jgs2020-239. doi: 10.1144/jgs2020-239
Levyns, M. R. (1938). Some evidence bearing on the past history of the cape Flora. Trans. R. Soc. S. Afr. 26, 401–424. doi: 10.1080/00359193809519782
Li, M., Bralower, T. J., Kump, L. R., Self-Trail, J. M., Zachos, J. C., Rush, W. D., et al. (2022). Astrochronology of the Paleocene-Eocene thermal maximum on the Atlantic coastal plain. Nat. Commun. 13:5618. doi: 10.1038/s41467-022-33390-x
Livermore, R., Hillenbrand, C.-D., Meredith, M., and Eagles, G. (2007). Drake Passage and Cenozoic climate: an open and shut case? Geochem. Geophys. Geosyst. 8:Q01005. doi: 10.1029/2005GC001224
MacArthur, R. H. (1984). Geographical Ecology: Patterns in the Distribution of Species, Princeton University Press, Princeton, NJ.
Mannion, P. D., Upchurch, P., Benson, R. B. J., and Goswami, A. (2014). The latitudinal biodiversity gradient through deep time. Trends Ecol. Evol. 29, 42–50. doi: 10.1016/j.tree.2013.09.012
Martin, J., and Gurrea, P. (1990). The peninsular effect in Iberian butterflies (Lepidoptera: Papilionoidea and Hesperioidea). J. Biogeogr. 17, 85–96. doi: 10.2307/2845190
Marzoli, A., Renne, P. R., Piccirillo, E. M., Ernesto, M., Bellieni, G., Min, D., et al. (1999). Extensive 200-million-year-old continental flood basalts of the Central Atlantic Magmatic Province. Science 284, 616–618. doi: 10.1126/science.284.5414.616
Massante, J. C., Götzenberger, L., Takkis, K., Hallikma, T., Kaasik, A., Laanisto, L., et al. (2019). Contrasting latitudinal patterns in phylogenetic diversity between woody and herbaceous communities. Sci. Rep. 9:6443. doi: 10.1038/s41598-019-42827-1
McDonald, P. J., Jobson, P., Köhler, F., Nano, C. E. M., and Oliver, P. M. (2021). The living heart: climate gradients predict desert mountain endemism. Ecol. Evol. 11, 4366–4378. doi: 10.1002/ece3.7333
McFadden, I. R., Sandel, B., Tsirogiannis, C., Morueta-Holme, N., Svenning, J.-C., Enquist, B. J., et al. (2019). Temperature shapes opposing latitudinal gradients of plant taxonomic and phylogenetic β diversity. Ecol. Lett. 22, 1126–1135. doi: 10.1111/ele.13269
McGlone, M. S. (1996). When history matters: scale, time, climate and tree diversity. Glob. Ecol. Biogeogr. 5, 309–314. doi: 10.2307/2997586
Meng, J., and McKenna, M. C. (1998). Faunal turnovers of Palaeogene mammals from the Mongolian plateau. Nature 394, 364–367. doi: 10.1038/28603
Meuser, E., Mooers, A. Ø., and Cleary Daniel, F. R. (2013). El Niño and biodiversity. In: Encyclopedia of Biodiversity, 2nd, 3 (Ed.) by S. A. Levin), pp. 155–163. Academic Press, Waltham, MA.
Meyers, S. R., and Malinverno, A. (2018). Proterozoic Milankovitch cycles and the history of the solar system. Proce. Natl. Acad. Sci. U. S. A. 115, 6363–6368. doi: 10.1073/pnas.1717689115
Mittelbach, G. G., Schemske, D. W., Cornell, H. V., Allen, A. P., Brown, J. M., Bush, M. B., et al. (2007). Evolution and the latitudinal diversity gradient: speciation, extinction and biogeography. Ecol. Lett. 10, 315–331. doi: 10.1111/j.1461-0248.2007.01020.x
Murakami, M., Goncharov, A. F., Miyajima, N., Yamazaki, D., and Holtgrewe, N. (2022). Radiative thermal conductivity of single-crystal bridgmanite at the core-mantle boundary with implications for thermal evolution of the earth. Earth Planet. Sci. Lett. 578:117329. doi: 10.1016/j.epsl.2021.117329
Nadeau, C. P., Urban, M. C., and Bridle, J. R. (2017). Climates past, present, and yet-to-come shape climate change vulnerabilities. Trends Ecol. Evol. 32, 786–800. doi: 10.1016/j.tree.2017.07.012
Newbold, T., Oppenheimer, P., Etard, A., and Williams, J. J. (2020). Tropical and Mediterranean biodiversity is disproportionately sensitive to land-use and climate change. Nat. Ecol. Evol. 4, 1630–1638. doi: 10.1038/s41559-020-01303-0
Nge, F. J., Biffin, E., Thiele, K. R., and Waycott, M. (2020). Extinction pulse at Eocene–Oligocene boundary drives diversification dynamics of two Australian temperate floras. Proc. R. Soc. B 287:20192546. doi: 10.1098/rspb.2019.2546
O’Brien, E. M. (2006). Biological relativity to water–energy dynamics. J. Biogeogr. 33, 1868–1888. doi: 10.1111/j.1365-2699.2006.01534.x
O’Brien, C. L., Huber, M., Thomas, E., Pagani, M., Super, J. R., Elder, L. E., et al. (2020). The enigma of Oligocene climate and global surface temperature evolution. Proce. Natl. Acad. Sci. U. S. A. 117, 25302–25309. doi: 10.1073/pnas.2003914117
O’Brien, E. M., and Peters, C. R. (1998). Wild fruit trees and shrubs of southern Africa: geographic distribution of species richness. Econ. Bot. 52, 267–278. doi: 10.1007/BF02862145
Ogawa, M. (2008). Mantle convection: A review. Fluid Dyn. Res. 40, 379–398. doi: 10.1016/j.fluiddyn.2007.09.001
Olazabal, M. R., de Gopegui, M., Tompkins, E. L., Venner, K., and Smith, R. (2019). A cross-scale worldwide analysis of coastal adaptation planning. Environ. Res. Lett. 14:124056. doi: 10.1088/1748-9326/ab5532
Oliver, J. E. (1987). Zonal circulation and index. In: The Encyclopedia of Climatology (Eds.) by J. E. Oliver and R. W. Fairbridge), pp. 942–946. Van Nostrand Reinhold, New York, NY.
Ovechkina, M. N., Watkeys, M. K., Mostovski, M. B., Kretzinger, W., and Perritt, S. M. (2021). Calcareous nannofossils identify the age and precipitation rates of manganese deposits of the Mozambique ridge and Mozambique Basin, SW Indian Ocean. Geo Marine Lett. 41:36. doi: 10.1007/s00367-021-00707-6
Parmentier, I., Malhi, Y., Senterre, B., Whittaker, R. J., Alonso, D., Nusbaumer, L. P., et al. (2007). “The odd man out”? Might climate explain the lower tree α-diversity of African rain forests relative to Amazonian rain forests? J. Ecol. 95, 1058–1071. doi: 10.1111/j.1365-2745.2007.01273.x
Procheş, Ş. (2006). Latitudinal and longitudinal barriers in global biogeography. Biol. Lett. 2, 69–72. doi: 10.1098/rsbl.2005.0396
Procheş, Ş., Ramdhani, S., Perera, S. J., Ali, J. R., and Gairola, S. (2015). Global hotspots in the present-day distribution of ancient animal and plant lineages. Sci. Rep. 5:15457. doi: 10.1038/srep15457
Qian, H., Jin, Y., and Ricklefs, R. E. (2017). Phylogenetic diversity anomaly in angiosperms between eastern Asia and eastern North America. Proce. Natl. Acad. Sci. U. S. A. 114, 11452–11457. doi: 10.1073/pnas.1703985114
Qian, H., Ricklefs, R. E., and White, P. S. (2005). Beta diversity of angiosperms in temperate floras of eastern Asia and eastern North America. Ecol. Lett. 8, 15–22. doi: 10.1111/j.1461-0248.2004.00682.x
Rosenzweig, M. L. (1995). Species Diversity in Space and Time. Cambridge University Press, Cambridge, UK.
Sandel, B., Arge, L., Dalsgaard, B., Davies, R. G., Gaston, K. J., Sutherland, W. J., et al. (2011). The influence of late quaternary climate-change velocity on species endemism. Science 334, 660–664. doi: 10.1126/science.1210173
Schluter, D. (2016). Speciation, ecological opportunity, and latitude. Am. Nat. 187, 1–18. doi: 10.1086/684193
Scotese, C. R. (2014a). Atlas of Permo-Triassic Paleogeographic Maps (Mollweide Projection), Maps 43–52, Volumes 3 and 4 of the PALEOMAP Atlas for ArcGIS, PALEOMAP Project, Evanston, IL.
Scotese, C. R., (2014b). Atlas of Neogene Paleogeographic Maps (Mollweide Projection), Maps 1–7, Volume 1, The Cenozoic, PALEOMAP Atlas for ArcGIS, PALEOMAP Project, Evanston, IL.
Scotese, C.R. (2022). PALEOMAP. Available at: https://www.scostese.com (Accessed December 13, 2022).
Seton, M., Gaina, C., Müller, R. D., and Heine, C. (2009). Mid-cretaceous seafloor spreading pulse: fact or fiction? Geology 37, 687–690. doi: 10.1130/G25624A.1
Sheldon, K. S. (2019). Climate change in the tropics: ecological and evolutionary responses at low latitudes. Ann. Rev. Ecol. Evol. Syst. 50, 303–333. doi: 10.1146/annurev-ecolsys-110218-025005
Silva de Miranda, P. L., Dexter, K. G., Swaine, M. D., Teixeira de Oliveira-Filho, A., Hardy, O. J., and Fayolle, A. (2022). Dissecting the difference in tree species richness between Africa and South America. Proce. Natl. Acad. Sci. U.S.A. 119:e2112336119. doi: 10.1073/pnas.2112336119
Spicer, R. A. (2017). Tibet, the Himalaya, Asian monsoons and biodiversity? In what ways are they related? Plant Divers. 39, 233–244. doi: 10.1016/j.pld.2017.09.001
Srinivasan, U., Tamma, K., and Ramakrishnan, U. (2014). Past climate and species ecology drive nested species richness patterns along an east–west axis in the Himalaya. Glob. Ecol. Biogeogr. 23, 52–60. doi: 10.1111/geb.12082
Srivastava, S. P., and Tapscott, C. R. (1986). “Plate kinematics of the North Atlantic,” in The Western North Atlantic region. The Geology of North America. eds. P. R. Vogt and B. E. Tucholke (Boulder, CO: Geological Society of America), 379–405.
Storey, M., Duncan, R. A., and Swisher, C. C. (2007). Paleocene-Eocene thermal maximum and the opening of the Northeast Atlantic. Science 316, 587–589. doi: 10.1126/science.1135274
Tada, R., Zheng, H., and Clift, P. D. (2016). Evolution and variability of the Asian monsoon and its potential linkage with uplift of the Himalaya and Tibetan plateau. Prog Earth Planet Sci 3:4. doi: 10.1186/s40645-016-0080-y
Tangang, F., Chung, J. X., Juneng, L., Supari Salimun, E., Ngai, S. T., Jamaluddin, A. F., et al. (2020). Projected future changes in rainfall in Southeast Asia based on CORDEX-SEA multi-model simulations. Clim. Dyn. 55, 1247–1267. doi: 10.1007/s00382-020-05322-2
Tarduno, J. A., Cottrell, R. D., Watkeys, M. K., Hofmann, A., Doubrovine, P. V., Mamajek, E. E., et al. (2010). Geodynamo, solar wind and magnetopause 3. 4 to 3. 45 billion years ago. Science 327, 1238–1240. doi: 10.1126/science.1183445
Tedersoo, L., Bahram, M., Põlme, S., Kõljalg, U., Yorou, N. S., Wijesundera, R., et al. (2014). Global diversity and geography of soil fungi. Science 346, 1078–1088. doi: 10.1126/science.1256688
Torsvik, T. H., Doubrovine, P. V., Steinberger, B., Gaina, C., Spakman, W., and Domeier, M. (2017). Pacific plate motion change caused the Hawaiian‐Emperor Bend. Nat. Commun. 8:15660. doi: 10.1038/ncomms15660
Trisos, C. H., Merow, C., and Pigot, A. L. (2020). The projected timing of abrupt ecological disruption from climate change. Nature 580, 496–501. doi: 10.1038/s41586-020-2189-9
Van Zinderen Bakker, E. M. (1975). The origin and palaeoenvironment of the Namib Desert biome. J. Biogeogr. 2, 65–73. doi: 10.2307/3038074
van den Ende, C., White, L. T., and van Welzen, P. C. (2017). The existence and break–up of the Antarctic land bridge as indicated by both amphi-Pacific distributions and tectonics. Gondwana Res. 44, 219–227. doi: 10.1016/j.gr.2016.12.006
Watkeys, M. K. (2006). “The break-up of Gondwana: a South African perspective,” in The Geology of South Africa. eds. M. R. Johnson, C. R. Anhaeusser, and R. Thomas (Pretoria: Geological Society of South Africa and Council for Geoscience), 531–539.
Westerhold, T., Marwan, N., Drury, A. J., Liebrand, D., Agnini, C., Anagnostou, E., et al. (2020). An astronomically dated record of Earth’s climate and its predictability over the last 66 million years. Science 369, 1383–1387. doi: 10.1126/science.aba6853
Willig, M. R., and Presley, S. J. (2018). Latitudinal gradients of biodiversity: theory and empirical patterns. In: Encyclopedia of the Anthropocene (Ed.) by D. DellaSala and M. Goldstein), pp. 13–19. Elsevier, Oxford, UK.
Woodburne, M. O. (2010). The Great American Biotic Interchange: dispersals, tectonics, climate, sea level and holding pens. J. Mamm. Evol. 17, 245–264. doi: 10.1007/s10914-010-9144-8
Worm, B., and Tittensor, D. P. (2018). A Theory of Global Biodiversity, Princeton University Press, Princeton, NJ.
Xu, W., Dong, W. J., Fu, T.-T., Gao, W., Lu, C.-Q., Yan, F., et al. (2021). Herpetological phylogeographic analyses support a Miocene focal point of Himalayan uplift and biological diversification. Nat. Sci. Rev. 8:nwaa263. doi: 10.1093/nsr/nwaa263
Keywords: biodiversity, climatic change, extinction, longitude, speciation, zonal circulation
Citation: Procheş Ş, Watkeys MK, Ramsay LF and Cowling RM (2023) Why we should be looking for longitudinal patterns in biodiversity. Front. Ecol. Evol. 11:1032827. doi: 10.3389/fevo.2023.1032827
Edited by:
Peter Convey, British Antarctic Survey, United KingdomReviewed by:
Jian-Li Zhao, Yunnan University, ChinaMaría Leonor Sandoval Salinas, Instituto de Investigación en Luz, Ambiente y Visión (CONICET), Argentina
Copyright © 2023 Procheş, Watkeys, Ramsay and Cowling. This is an open-access article distributed under the terms of the Creative Commons Attribution License (CC BY). The use, distribution or reproduction in other forums is permitted, provided the original author(s) and the copyright owner(s) are credited and that the original publication in this journal is cited, in accordance with accepted academic practice. No use, distribution or reproduction is permitted which does not comply with these terms.
*Correspondence: Şerban Procheş, ✉ c2V0YXBpb25AZ21haWwuY29t