- 1Centro de Investigaciones en Microbiología y Biotecnología-UR, Facultad de Ciencias Naturales, Universidad del Rosario, Bogotá, Colombia
- 2Grupo de Investigación en Ciencias Básicas (NÚCLEO), Facultad de Ciencias e Ingeniería, Universidad de Boyacá, Tunja, Colombia
- 3Centro de Tecnología en Salud, Innovaseq SAS, Bogotá, Colombia
- 4Upqua S.A.S., Bogotá, Colombia
- 5Grupo de Estudios en Salud Pública de la Amazonía, Laboratorio de Salud Pública Departamental del Amazonas, Leticia, Colombia
- 6Secretaría de Salud de Boyacá, Tunja, Colombia
- 7Secretaría Seccional de Salud de Magdalena, Santa Marta, Colombia
- 8Hospital Local de El Retén, El Retén, Colombia
- 9Secretaría Departamental de Salud del Vichada, Puerto Carreño, Colombia
- 10Department of Pathology, Molecular and Cell-Based Medicine, Icahn School of Medicine at Mount Sinai, Molecular Microbiology Laboratory, New York, NY, United States
The lack of precise and timely knowledge about the molecular epidemiology of arboviruses of public health importance, particularly in the vector, has limited the comprehensive control of arboviruses. In Colombia and the Americas, entomovirological studies are scarce. Therefore, this study aimed to describe the frequency of natural infection and/or co-infection by Dengue (DENV), Zika (ZIKV), and Chikungunya (CHIKV) in Aedes spp. circulating in different departments of Colombia (Amazonas, Boyacá, Magdalena, and Vichada) and identifying vector species by barcoding. Aedes mosquitoes were collected in departments with reported prevalence or incidence of arbovirus cases during 2020–2021, located in different biogeographic zones of the country: Amazonas, Boyacá, Magdalena, and Vichada. The insects were processed individually for RNA extraction, cDNA synthesis, and subsequent detection of DENV (serotypes DENV1-4 by multiplex PCR), CHIKV, and ZIKV (qRT-PCR). The positive mosquitoes for arboviruses were sequenced (Sanger method) using the subunit I of the cytochrome oxidase (COI) gene for species-level identification. In total, 558 Aedes mosquitoes were captured, 28.1% (n = 157) predominantly infected by DENV in all departments. The serotypes with the highest frequency of infection were DENV-1 and DENV-2 with 10.7% (n = 58) and 14.5% (n = 81), respectively. Coinfections between serotypes represented 3.9% (n = 22). CHIKV infection was detected in one individual (0.2%), and ZIKV infections were not detected. All infected samples were identified as A. aegypti (100%). From the COI dataset (593 bp), high levels of haplotype diversity (H = 0.948 ± 0.012) and moderate nucleotide diversity (π = 0.0225 ± 0.003) were identified, suggesting recent population expansions. Constructed phylogenetic analyses showed our COI sequences’ association with lineage I, which was reported widespread and related to a West African conspecific. We conclude that natural infection in A. aegypti by arbovirus might reflect the country’s epidemiological behavior, with a higher incidence of serotypes DENV-1 and DENV-2, which may be associated with high seroprevalence and asymptomatic infections in humans. This study demonstrates the high susceptibility of this species to arbovirus infection and confirms that A. aegypti is the main vector in Colombia. The importance of including entomovirological surveillance strategy within public health systems to understand transmission dynamics and the potential risk to the population is highlighted herein.
Introduction
Dengue virus (DENV), Zika virus (ZIKV), and Chikungunya virus (CHIKV) are considered to be arboviruses of major medical importance, capable of causing febrile syndromes, hemorrhagic fevers, and neurodegenerative diseases (Gould et al., 2017; Wilder-Smith et al., 2017; Paixao et al., 2018; Young, 2018). Dengue has been described as the most prevalent arboviral infection, with 40% of the human population at risk of infection, especially in tropical and subtropical countries (Wilder-Smith et al., 2010; Bhatt et al., 2013; Murray et al., 2013; Patterson et al., 2016; Sukhralia et al., 2019). Its incidence has increased 30 times in the last decades (Guzman et al., 2016; Pollett et al., 2018), with reports of more than 390 million infections annually (Bhatt et al., 2013). This virus has four serotypes, DENV-1, DENV-2, DENV-3, and DENV-4; each serotype confers specific lifelong immunity (Martina et al., 2009). However, with second infections by heterologous serotype, there is a higher risk of developing severe and/or lethal forms of the disease (Klungthong et al., 2004; Ngono and Shresta, 2018). CHIKV has caused widespread epidemics in Asian countries and East Africa; since 2004, it has spread to more than 60 countries, including the Americas, in 2013 (Leparc-Goffart et al., 2014; Gordon et al., 2018). Similarly, ZIKV has produced major outbreaks in 86 countries and territories in the Pacific, Asia, and the Americas, generating devastating congenital anomalies and neurological disorders, including microcephaly and Guillain-Barré syndrome post-2015 (Kazmi et al., 2020).
Aedes aegypti and Aedes albopictus are the main vectors of epidemic arboviruses DENV, CHIKV, and ZIKV (Kraemer et al., 2015; Leta et al., 2018; Lwande et al., 2020). These insects show broad ecological plasticity under different anthropic, climatic, and environmental conditions (Bonizzoni et al., 2013; Brady et al., 2014; Liu-Helmersson et al., 2016; Ciota et al., 2018), facilitating its spread in tropical, subtropical and some temperate zones (Leta et al., 2018; Kraemer et al., 2019; Ryan et al., 2019). In recent years, virological surveillance using molecular techniques has been proposed as a highly sensitive and efficient tool for detecting arboviral infection in vectors (Faye et al., 2013; Santiago et al., 2013; Alvarez-Diaz et al., 2021). Studies with this approach show that infection rates in A. aegypti and A. albopictus mosquitoes in endemic areas of Latin America may vary depending on the arboviral species, thus providing a promising approach to trigger control strategies. In the Aedes mosquitoes, DENV is more prevalent, with ranges between zero and 16.2% (Dos Santos et al., 2017; Kirstein et al., 2021), and CHIKV is between zero and 12.5% (Cevallos et al., 2018; Monteiro et al., 2020), while ZIKV represents the lowest rate between zero and 9.0% (Monteiro et al., 2020). Although data on arboviral co-infections in mosquitoes are limited, some studies demonstrate differences in susceptibility to mixed infections by species and serotype in mosquitoes, with frequencies ranging from 0.9 to 13.3% (Caron et al., 2012; Pena-Garcia et al., 2016; Pérez-Castro et al., 2016; Martínez et al., 2020).
The identification of Aedes species and their genetic variability has also been described using molecular markers to understand population dynamics and possible implications for disease transmission (Scarpassa et al., 2008; Paupy et al., 2012; Jaimes-Duenez et al., 2015; Pereira et al., 2017). This indicates that the infection rates and the identification of vector genetic diversity are essential from the point of view of molecular epidemiology to determine the possible routes of arboviral transmission and the progressive dispersion and/or invasion of the Aedes mosquito in endemic areas (Caron et al., 2012; Paupy et al., 2012; Jimenez-Silva et al., 2018; Joyce et al., 2018).
Colombia is a hyper-endemic country for Dengue, where the four serotypes co-circulate (Padilla et al., 2017). Moreover, it is one of the countries with the highest reported cases in the Americas and the Caribbean. Since 2016, there has been co-circulation of other arboviruses, such as CHIKV and ZIKV, a condition given by the geographical characteristics, the conditions of virus transmission, and the presence of the vector in about 90% of the national territory (Instituto Nacional De Salud, 2019b). Currently, more than 26 million people, corresponding to more than half of the national population, are exposed to arbovirus infection (Hoyos-Lopez et al., 2016; Gómez and Zapata-Úsuga, 2019; Instituto Nacional De Salud, 2019b,2020; Ministerio De Salud Y Protección Social, 2020). Despite this epidemiological situation, there is insufficient notification in some regions and a narrow active search for the circulation of different arboviral species (Gutierrez-Barbosa et al., 2020). An alarming aspect is the co-circulation of arboviral species in the same region by the same vector species (Wilder-Smith et al., 2017; Ciota, 2019; Vogels et al., 2019).
The lack of accurate and timely knowledge about the molecular epidemiology of arboviruses of public health importance, particularly in the mosquito vector, has limited the prediction of the genuine risk of emergence and spread of new arboviral strains in human populations. In Colombia and the Americas, studies on entomological characterization of Aedes mosquitoes and detection of arboviruses are scarce (Jaimes-Duenez et al., 2015; Pena-Garcia et al., 2016; Pérez-Castro et al., 2016; Peña-Garcia et al., 2017; Martínez et al., 2020; Carrasquilla et al., 2021), which has implications for effective arboviral control strategies. Therefore, this study aimed to describe the frequency of infection and/or natural co-infection by DENV, CHIKV, and ZIKV in Aedes spp. mosquitoes circulating in different Colombian departments and identify using molecular barcoding the vector species. We highlight the potential of molecular tests for the surveillance of arboviruses in mosquitoes and the identification of the vector species (entomovirologial surveillance).
Materials and methods
Location and capture of adult Aedes
Adult mosquitoes of the genus Aedes were captured between December 2020 and August 2021 in different municipalities of four departments of Colombia: the municipality of Leticia (4°12′29″S, 69°56′36″W) located in the department of Amazonas, in the south of the country in the Amazon region, shares borders with Brazil; municipalities of Puerto Boyacá (5°58′33.6″N 74°35.11″W) and Otanche (5°39′24.2″N 74°10.949′W), located in the department of Boyacá, in the central Andean region; municipality of Retén (10°36′40.9.9″ N 74°16. 094′W), in the department of Magdalena, located on the Colombian Caribbean coast; and the municipalities of Santa Rosalia (5°07′1″N 70°52′1″W) and La Primavera (5°28′59″ N 70°24′ 0″W) in the department of Vichada, located on the east in the vast plains of the Orinoco region, bordering Venezuela to the east (Figure 1). The selection of the study sites was made considering the location of the departments in different biogeographic areas of Colombia and the records of incidence of cases of arbovirus infection (Dengue, Chikungunya, and Zika), as reported by the Colombian Public Health Surveillance System (Sivigila) (Instituto Nacional De Salud, 2020, 2022; Figure 1). With the support of the local Public Health Secretaries, a non-probabilistic convenience sampling was conducted to obtain a range of sampling efforts from 100 to 150 individuals in urban and/or peri-urban areas. Adult mosquitoes were collected in a single sampling moment per department. The department of Boyacá was sampled in November 2020 (end of rainy season), while the departments of Magdalena, Amazonas and Vichada were sampled in the months of April and June 2021 (rainy season). Black light traps placed in the selected areas for 24 h and/or manually captured with mechanical vacuum cleaners during the day were used. At each site, only mosquitoes of the Aedes genus were selected, and subsequently pooled to optimize both the transport and storage of insects from the sampling sites. The entomological material was preserved in RNA later (DNA/RNA shield, Zymo. R1100-50) at 4°C in flasks marked with the coordinates and/or collection site. The samples were then sent to the Universidad del Rosario microbiology laboratory in Bogotá, Colombia, for processing and molecular analysis.
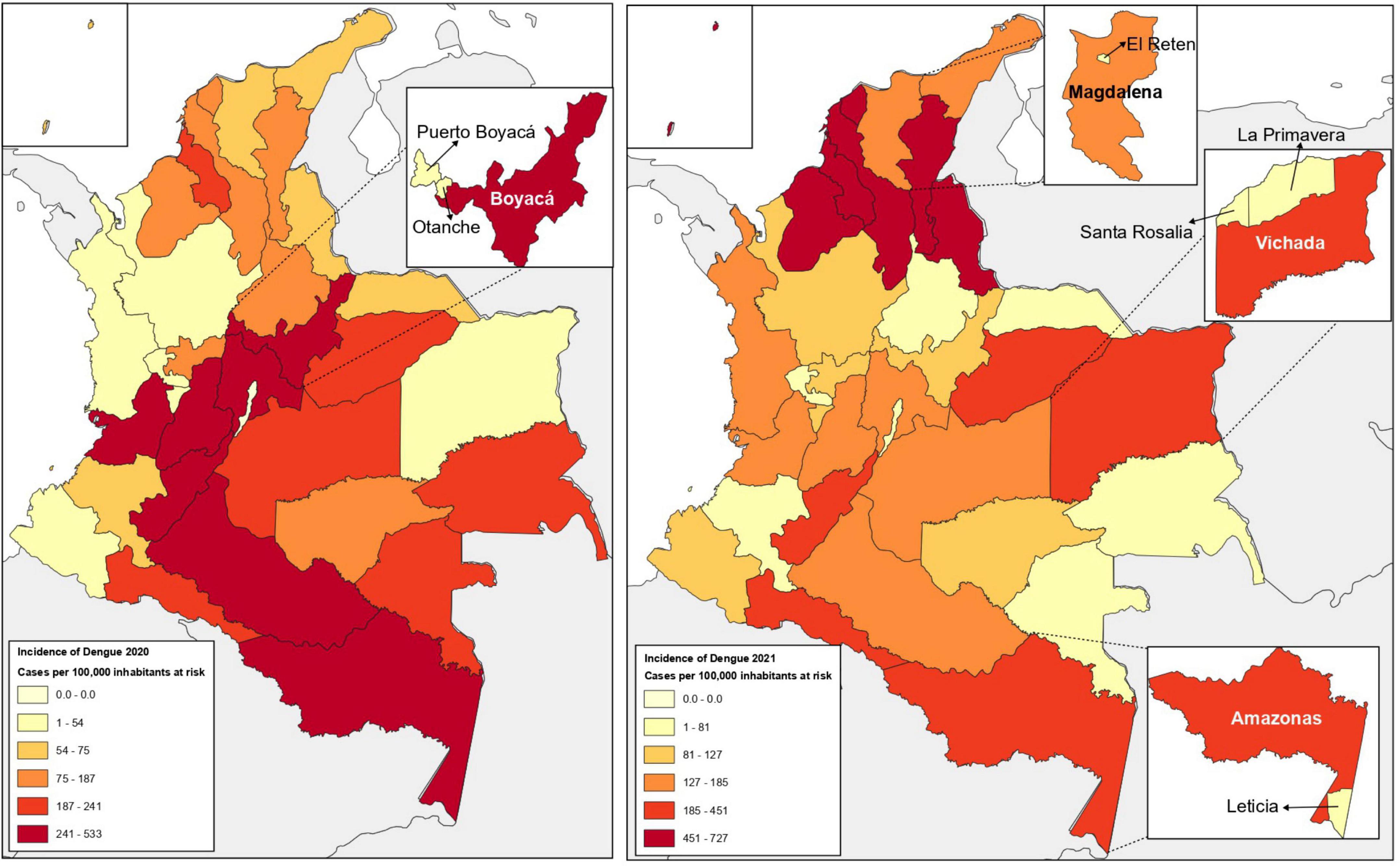
Figure 1. Dengue incidence in Colombia during 2020–2021 and study sites. Map of Colombia, showing Dengue incidence data (reported cases per 100,000 population at risk) for the years 2020 (left) and 2021 (right). The sampled departments and municipalities are highlighted. Entomological sampling of Aedes mosquitoes in 2020 in the department of Boyacá (Dengue incidence data 241-533). Entomological sampling of Aedes mosquitoes in 2021 in the departments of Magdalena (Dengue incidence data 127-185), Amazonas and Vichada (Dengue incidence data 185-451). The data used for these maps were obtained from Colombia’s Public Health Surveillance System (Sivigila) (Instituto Nacional De Salud, 2020, 2022).
RNA extraction and cDNA synthesis
In the laboratory, Aedes mosquitoes collected from each department were processed individually as a more accurate strategy for arbovirus detection, as previously reported (Martínez et al., 2020). Each mosquito (each specimen) was disrupted using ZR BashingBead™ lysis tubes (Lysis Tubes-ref. S6003-50) with 200 μL of DNA/RNA Shield buffer in TissueLyser II® tissue homogenizer (Qiagen, Hilden, Germany), followed by centrifugation at 10,000 rpm for 2 min. RNA extraction was performed with the Quick-RNA Viral–ZYMO kit (Zymo, ref 1035), following the manufacturer’s recommended instructions. Once RNA was obtained, its concentration and quality were measured using a Nanodrop-2000 spectrophotometer (Thermo Fisher Scientific, Waltham, MA, United States) and stored at −80°C in a Revco freezer (Thermo Fisher Scientific, Waltham, MA, United States). From the RNA obtained, RT-PCR was performed to generate cDNA using LunaScript RT SuperMix Reverse Transcriptase Kit (NEB #E3010). Finally, the cDNA was stored at −30°C.
Conventional polymerase chain reaction for the identification of Dengue virus serotypes
Detection of DENV serotypes (DENV 1-4) was performed by multiplex PCR, using primers for the region of the C-prM gene previously reported (Table 1; Chien et al., 2006). GoTaq®Green PCR Master Mix (2×) enzyme (Promega, # M7123) was used with 10 μmol of each primer (MD1, rTS1, rTS2, rTS3, and rTS4) and 0.8 μl of cDNA. The thermal profile consisted of the following: an initial denaturation cycle at 95°C for 5 min, 35 cycles of 95°C for 30 s, 57°C for 45 s and 72°C for 33 s, and finally, a final extension cycle of 72°C for 10 min. Visualization of the gene fragments was carried out using 2% agarose gel electrophoresis in 1× TBE buffer and as an intercalating agent 1 μL of SYBR® Safe (Invitrogen®, Carlsbad, CA, United States), then the gel was placed under UV light to observe the amplification band of each serotype, in the case of DENV-1 a band of 208 base pairs (bp) was observed, DENV-2 a band of 119 bp, DENV-3 a band of 288 bp and DENV-4 a band of 260 bp. The DENV positive controls (supernatant of culture cells individually infected with serotypes DENV1, DENV2, DENV3, DENV4) were provided by the Universities of Antioquia and Antonio Nariño in Colombia. Finally, confirmation of positive samples was performed in duplicate.
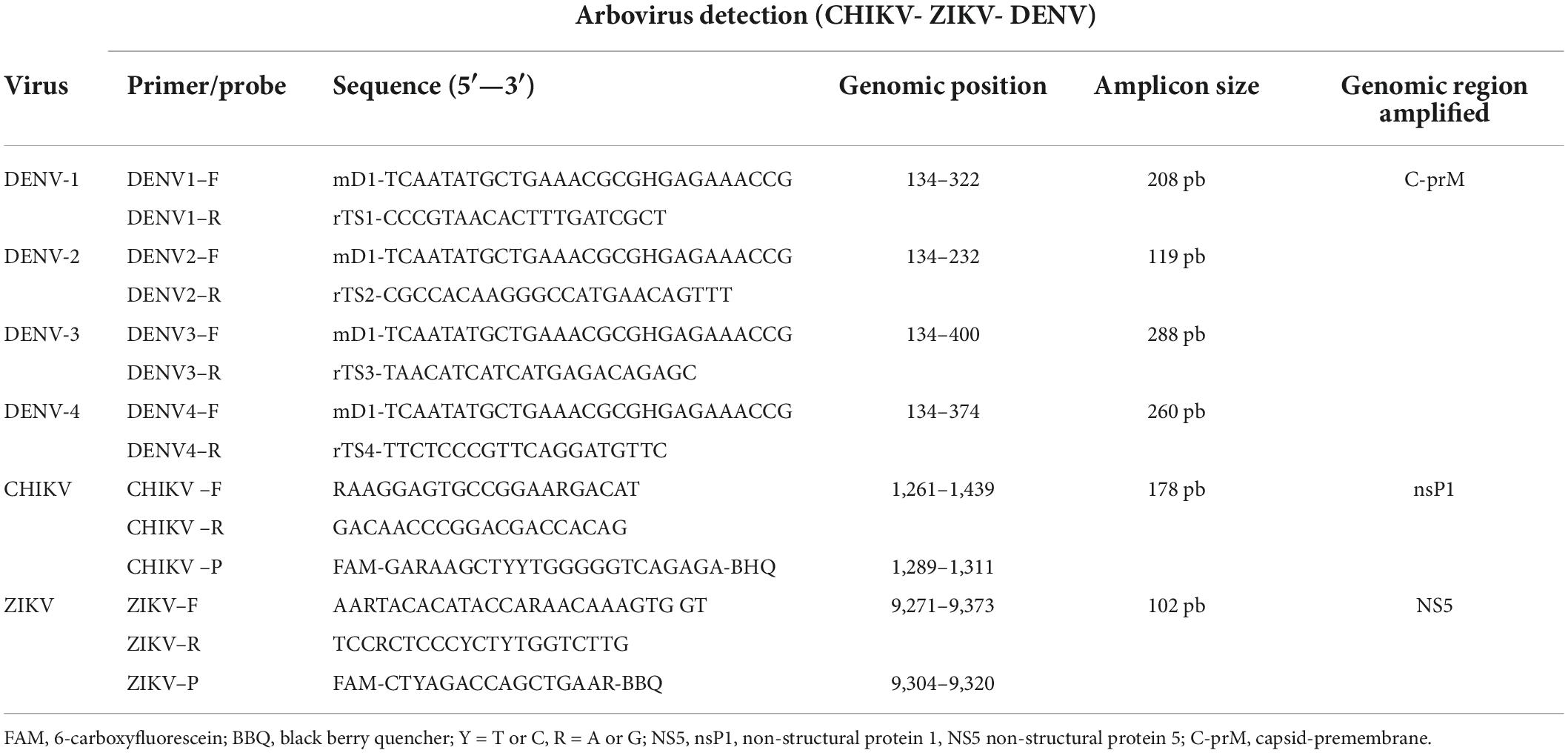
Table 1. List of primers and Taqman probes used in PCR for the detection of DENV, serotypes DENV1-4 (Chien et al., 2006), CHIKV (Alvarez-Diaz et al., 2021), and ZIKV (Faye et al., 2013).
Real-time polymerase chain reaction for the detection of Chikungunya and Zika
The identification of CHIKV and ZIKV was performed by real-time reverse transcription-polymerase chain reaction (qRT-PCR) using the corresponding primers and Taqman probes previously proposed (Faye et al., 2013; Alvarez-Diaz et al., 2021; Table 1). The qRT-PCR reaction for CHIKV and ZIKV (per sample) contained qScriptTM One-Step (2×), Low ROXTM (Quantabio, Carlsbad, CA, United States). For CHIKV, 10 μM of each primer (F-5′RAAGGAGTGAGTGCCGGAARGACAT) and (R-5′ GACAACCCCCGGACGACCACAG), 5 μM of the probe (5′FAM-GARAAGCTYYYTGGGGGGGTCAGAGA) and 5 μL of RNA were used. The thermal profile used consisted of one cycle at 50°C for reverse transcriptase activation for 10 min, followed by initial denaturation at 95°C for 2 min, 40 cycles of 95°C for 15 s, 55°C for 45 s. For ZIKV, 10 μM of each primer (F-5′ AARTACACATACCARAACACCARAACAAAGTG GT) and (R-5 TCCRCTCCCYCTYCTYTGGTCTTG), 25 μM of the probe (5′-FAM-CTYAGACCAGCTGAAR) and 5 μL of RNA were used. Amplification conditions were: one cycle at 50°C for 10 min, 95°C for 1 min, 40 cycles of 95°C for 15 s, and 56°C for 1 min. To perform the real-time PCR, the cut-off Ct value was ≤ 39 based on different criteria, among which are CDC (Centers for Disease Control and Prevention) interpretation criteria, considering a specimen positive if primer sets showed amplification with cycle threshold (Ct) values ≤ 38.5. Likewise, positive tests were confirmed in duplicate. The positive controls for CHIKV and ZIKV were donated by the Universidad de Antioquia and the Universidad Antonio Nariño. The frequency of infection for CHIKV and ZIKV was expressed as the infection rate.
Molecular identification of Aedes species
A fragment of subunit I of the mitochondrial Cytochrome Oxidase gene (mt-COI) (species-specific barcode) was amplified from the resulting positive mosquitoes for DENV, CHIKV, or ZIKV. Reactions contained 2× GoTaq®Green Master Mix, (Promega, # M7123), 10 μM of each primer LCO1490 (5′-GGTCAAATCATAATAAAGATATTGG-3′) and HCO2198 (5′-TAAACT TCAGGGTGACCAAAAAATCA-3′) (Joyce et al., 2018), and 5 μL of cDNA. Thermal profiling started with a DNA polymerase activation step at 95°C for 1 min, 45 cycles of 94°C for 10 s for denaturation, 60°C for 1 min for primer annealing, and a final extension at 72°C for 10 min. This was followed by 2% agarose gel electrophoresis and SYBR-Safe as an intercalating agent (Invitrogen®, Carlsbad, CA, United States) to observe a band of ∼650 base pairs (bp). The amplified products were sequenced using the Sanger sequencing platform, following cleanup using ExoSAP-IT™ PCR Product Cleanup Reagent (Applied Biosystems™ #78205). In addition to rule out degradation of the samples, the COI amplification was used as internal control. Therefore, a random selection of 10 negative mosquitoes per collection site were analyzed observing the amplification of the marker COI in all of them.
Cytochrome oxidase gene sequence analysis (barcoding)
Consensus sequences were obtained using Geneious Prime program.1 Each sequence was then compared with the GenBank database using the BLAST (Basic Local Alignment Search Tool) sequence alignment tool (Mount, 2007), and the Aedes species were assigned considering the percentage of identity (higher than 95%) and the best e-value result. Multiple sequence alignments were performed with the MAFFT algorithm (Katoh and Standley, 2013) in Unipro UGENE software (Okonechnikov et al., 2012) using sequences retrieved from Genbank (Supplementary Table 1).
Subsequently, the genetic variability of the COI marker for A. aegypti in the samples from the study departments was evaluated through genetic diversity indices, including the number of haplotypes (h), the number of segregation sites (s), the average number of differences (k), haplotype diversity (hd), nucleotide diversity (π), using DnaSP software (Rozas et al., 2017). Using the same software, the divergence test was performed using Tajima’s D (Rozas et al., 2017). In addition, haplotype networks were constructed using the median joint method (MJN) in PopART (Population Analysis with Reticulate Trees) software (Leigh and Bryant, 2015) in order to observe the relationships between the sequences of COI haplotypes obtained for Colombia. Additionally, to infer relationships between the COI haplotypes of A. aegypti generated in our study with COI haplotypes from outside Colombia, a Maximum Likelihood (ML) phylogenetic analysis was performed on the haplotypes from each department, together with haplotypes from Africa, Asia, America, and the Caribbean regions published in similar studies, and obtained from the GenBank database (Supplementary Table 1). Representative COI haplotype sequences between and within each department were selected for phylogenetic analysis. IQtree version 1.6.12 software was used (Nguyen et al., 2015), with the TIM3 + F + I + G4 substitution model (chosen as the best in software). Branch support was performed using 10,000 repeats by ultrafast bootstrap approximation (UFBoot), visualized iTOL.2 The COI gene sequence of Culex spinosus (KM593059.1) was used as outgroup (Martínez et al., 2020).
Statistical analyses
Initially, the results of arbovirus infection were analyzed in terms of absolute and relative frequencies for the variables considered in the study (departments and arboviral species and/or serotypes). Subsequently, the Chi-square test was used to establish possible associations between these variables. In order to perform multiple comparisons between the different departments under study with the arbovirus species, post hoc tests were implemented through the vcd package in R software using the chisq.post hoc.test function that allows pairwise comparisons using the Bonferroni adjustment method. Statistical analyses were carried out using the R software (RStudio Team, 2019). All significance tests were two-tailed, and p-values < 0.01 were considered statistically significant.
Results
Natural arbovirus infection in Aedes mosquitoes
A total of 558 Aedes specimens were captured (female and male), with 150 individuals collected in Amazonas, Boyacá, and Magdalena, respectively, and 108 in the department of Vichada (Table 2). In general, in all study departments, the arbovirus with the highest frequency was DENV, found in 28.1% (n = 157) of Aedes specimens, with a predominance of serotypes DENV-1 and DENV-2, with a frequency of 9.9% (n = 55) and 14.2% (n = 79), respectively (Supplementary Table 2). Only one individual was infected by CHIKV (0.18%), and none by ZIKV (0.0%). The DENV-1 serotype was detected with a higher frequency in Boyacá at 18% (n = 27), followed by the department of Amazonas with a frequency of 11.3% (n = 17) and Magdalena with 6.0% (n = 9), while Vichada with 1.9% (n = 2) registered the lowest frequency of infection by this serotype (Figure 2 and Supplementary Table 2). In contrast, DENV-2 was detected with a higher frequency in samples from Vichada 30.6% (n = 33), with the highest infection rate per serotype, followed by 14.7% in Amazonas (n = 22), 9.3% in Boyacá (n = 14) and 6.7% in Magdalena (n = 10). A significant relationship was found between the distribution of DENV among departments (chi-square tests, p < 0.005). Multiple comparisons showed that only DENV-2 was specifically associated with the department of Vichada (chisq.post hoc.test p < 0.000036). In addition, the total study areas found a frequency of 3.9% (n = 22) of mixed infections by DENV serotypes. Co-infections by DENV-1/DENV-2 were found in all departments, with a higher frequency in Amazonas at 5.3% (n = 8). Only in Magdalena were two individuals coinfected with DENV-1/DENV-3 (1.3%) and three individuals with DENV-1/DENV-2/DENV-3 (2.0%), while in Vichada, one (1) individual was coinfected with DENV-2/DENV-3 (0.9%) (Figure 2). No relationship was found between the distribution of DENV co-infections between departments (chi-square tests, p < 0.1549).
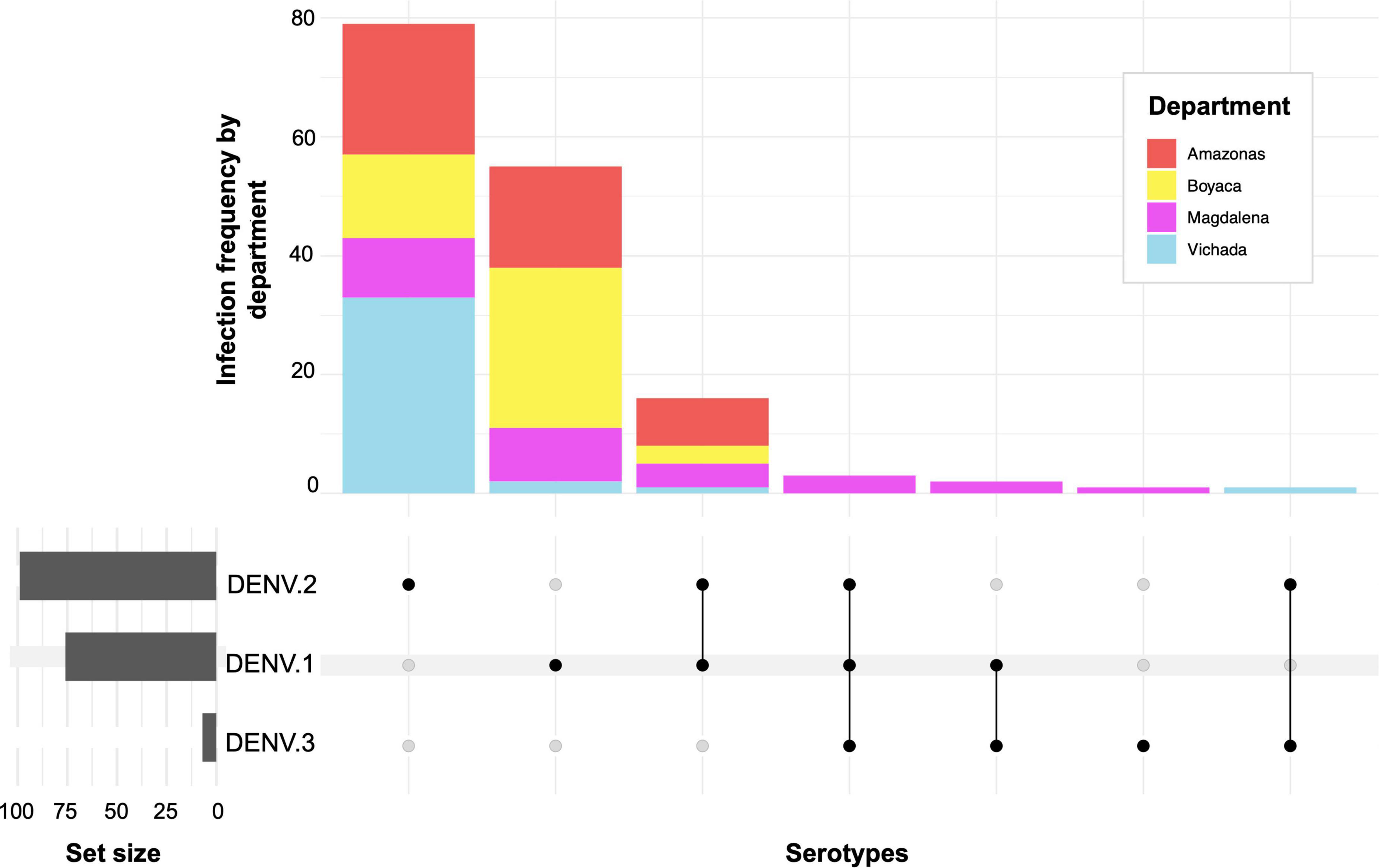
Figure 2. Frequency of infection and co-infection by Dengue serotypes in Aedes aegypti mosquitoes in Colombia.
Cytochrome oxidase gene sequence analysis (haplotypes)
Based on the analysis of the COI sequences of the arbovirus-positive mosquito samples infected with DENV and CHIKV (157 sequences), they were all classified as A. aegypti using the BLASTn algorithm in the NCBI database. Subsequently, a total of 145 sequences were used to study genetic variations in the COI gene: 44 from the department of Amazonas, 42 from the department of Boyacá, 28 from the department of Magdalena, and 31 from the department of Vichada (Table 3).
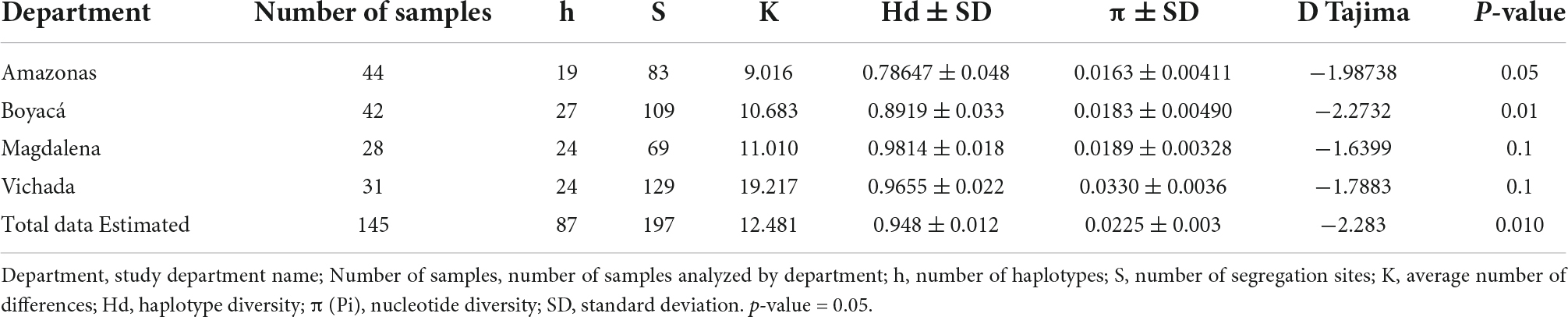
Table 3. Genetic diversity analysis in Aedes aegypti mosquitoes based on molecular analysis of the COI gene.
Eighty-seven haplotypes were identified from the COI dataset, with 197 variable sites. High levels of haplotypic diversity (H = 0.948 ± 0.012) and moderate nucleotide diversity were observed (π = 0.0225 ± 0.003) (Table 3). The number of haplotypes per department was similar in Boyacá (h = 28), Magdalena, and Vichada (h = 24), while it varied in Amazonas (h = 19). Haplotypic diversity was comparatively higher in the departments of Magdalena (H = 0.9814 ± 0.018), Vichada (H = 0.9655 ± 0.022), and Boyacá (H = 0.8919 ± 0.033) compared to the departments of Amazonas (H = 0.78647 ± 0.048), while nucleotide diversity was similar in all departments (∼π = 0.017) and marginally higher in the department of Vichada (π = 0.0330 ± 0.0036). The mean number of haplotypic differences (K) for the populations of the departments of Amazonas, Boyacá, Magdalena, and Vichada were, respectively, 9,016, 10,683, 11,010, and 19,217.
The mean value of Tajima’s D in the analyzed sequences from the four study departments was negative (-2.283) (Table 3). In addition, the Tajima D values for Amazonas and Boyacá were negative and statistically significant (p < 0.01), suggesting recent population expansions. In Magdalena and Vichada, however, Tajima’s D was negative but not significant (p > 0.1). A significant difference in π values was observed between the municipalities.
Figure 3A shows the COI haplotype network of A. aegypti from the study departments produced by the median junction network (MJN). Of the 145 mitochondrial sequences analyzed, we obtained 87 haplotypes distributed among the four departments, with many low-frequency haplotypes. A dispersed haplotype network was observed; 51.72% of the haplotypes were shared among the departments. The network shows two main haplogroups of higher frequency and geographic distribution. The first (haplotype color network) predominantly consists of Amazon haplotypes, composed of ancestral haplotypes (H1). The second haplogroup (haplotype color red, pink, yellow, and blue) consists of haplotypes from all locations (H6).
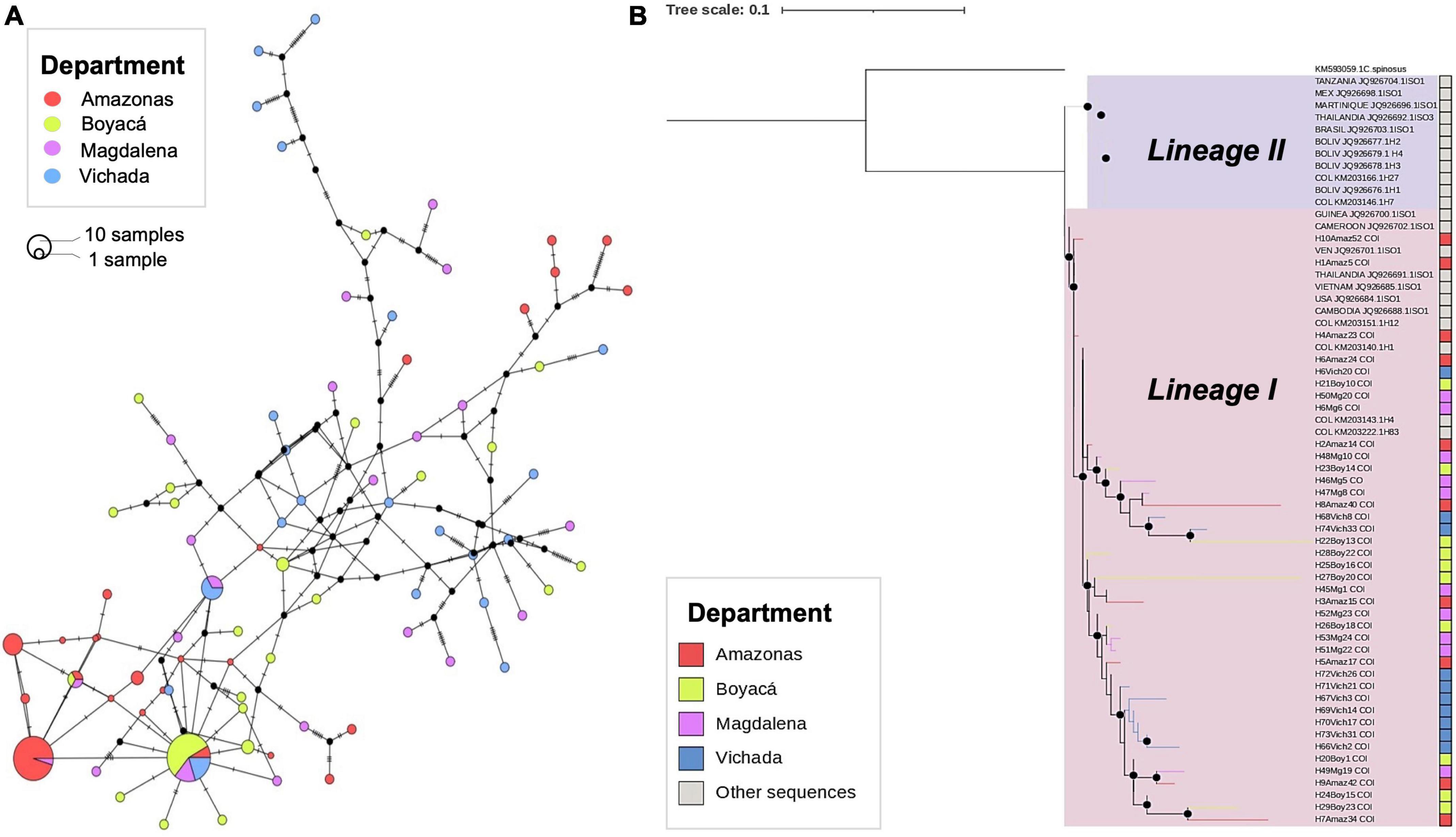
Figure 3. Median-joining network (MJN) and phylogenetic analysis of mitochondrial COI haplotypes between Aedes aegypti populations in different departments of Colombia. (A) The haplotype network from A. aegypti sequences was collected in the departments of Amazonas, Boyacá, Magdalena, and Vichada. The lines on each branch indicate the number of mutational steps between haplotypes, and the size of the circle is proportional to the number of individuals. (B) A maximum likelihood (ML) tree based on the TIM3 + F + I + G4 nucleotide substitution model of A. aegypti individuals from the study departments. Black dots indicate bootstrap support > 80. The red, yellow, purple, and turquoise indicate haplotypes found in Colombia’s Amazonas, Boyacá, Magdalena, and Vichada departments. Shown in gray are haplotype sequences from Africa, Asia, the Americas, and the Caribbean, obtained from the GenBank database.
A multiple sequence alignment of A. aegypti haplotype sequences was constructed with the COI sequences generated in this study and with the 22 COI haplotype sequences retrieved from NCBI genebank worldwide (Supplementary Table 1). Phylogenetic relationships between COI gene haplotype sequences from a representative group of our samples (30%) were examined along with sequences from around the world using maximum likelihood methods. The phylogenetic tree showed two main clusters, with Bootstrap support > 80% (Figure 3B). One group was associated with COI sequences of haplotypes from East Africa (Tanzania), the Caribbean region (H2 Martinique), and the Americas (Mexico, H1 Brazil, Colombia, and H1, H2, H3, and H4 Bolivia) reported as Lineage II (Bracco et al., 2007; Paupy et al., 2012). The second cluster was associated with haplotypes from West Africa (H1 Cameroon, H1 Guinea, and H1 Cambodia) and the Americas (H1 Venezuela, H1 United States, H1 Colombia, H4, H58.) reported as Lineage I. (Bracco et al., 2007; Paupy et al., 2012). All A. aegypti sequences in the present study were associated with Lineage I (Figure 3B).
Discussion
Currently, vector control efforts and active surveillance for the early detection of epidemic events caused by arboviruses are fundamental for the integrated management of arboviral diseases (Kilpatrick and Randolph, 2012; Golding et al., 2015; Kauffman and Kramer, 2017). In the present study, we identified natural infection by DENV and CHIKV in specimens of Aedes spp., collected from different departments of Colombia. However, ZIKV was not detected in this study. DENV was the arbovirus with the highest frequency of infected mosquitoes (28.1%), while CHIKV and ZIKV had low (0.2%) or none (0.0%) infection rates, respectively (Table 2). These results are consistent with the behavior of arboviruses in Colombia, where in recent years, Dengue notifications have represented 99.5% of the total number of arbovirus notifications in the country (Instituto Nacional De Salud, 2020, 2022). They are also related to the high seroprevalence of asymptomatic cases of DENV infections, more than 85% in children and 90% in adults identified in the national territory (Velandia-Romero et al., 2020), which may play an important role in the silent maintenance and progression of the transmission cycle, between humans and mosquitoes (Pollett et al., 2018). In addition, the detection of CHIKV in only one individual and the non-detection of ZIKV in Aedes mosquitoes may reflect the decrease in transmission of these viruses following the outbreaks that occurred in 2015 and 2016. In recent years, a gradual decrease in the notification of Chikungunya and Zika cases has been observed (Instituto Nacional De Salud, 2020, 2022), with a low incidence in 2020 and 2021 (0.3–0.5 cases per 100.000 inhab) for both arboviruses. The reduced circulation of these arboviruses may be associated with low rates of susceptible population turnover and reintroduction (Carrasquilla et al., 2021), which limits the circulation of these viruses in humans and Aedes.
Our results on the frequency of infection by arboviruses are within the ranges found in other studies of infection in A. aegypti in Latin America. The most prevalent viral infection in A. aegypti is DENV, with frequencies in Brazil of 0.81% from 2012 to 2013 (Dos Santos et al., 2017) and 0.2% in 2017 (Monteiro et al., 2020), while in Mexico, 7.7% frequency was found for 2016 and 2017 (Kirstein et al., 2021). In Colombia, studies on DENV infection frequencies in this mosquito-vector show infection rates between 12% in the north and center of the country in 2012–2013 (Pena-Garcia et al., 2016) of 62% in the central region by 2015 (Pérez-Castro et al., 2016) and 29.6% for eastern Colombia in 2018–2019 (Martínez et al., 2020). These results may be closely related to the epidemic and inter-epidemic periods for Dengue in Colombia and the Americas (Ramos-Castaneda et al., 2017), as well as sampling effort, local abundance of A. aegypti and intensity of arbovirus circulation (Kirstein et al., 2021). Several studies reveal the vast and permanent circulation of DENV in the continent, which is related to the extensive distribution of A. aegypti in the region, the co-circulation of the four serotypes (DENV 1-4) (Ramos-Castaneda et al., 2017; Gutierrez-Barbosa et al., 2020; Instituto Nacional De Salud, 2020) and the susceptibility profiles of the local populations (Jimenez-Silva et al., 2018; Velandia-Romero et al., 2020). In addition, some authors indicate that the successful transmission of arboviruses such as DENV by A. aegypti may also be due to transovarial or vertical transmission (Pena-Garcia et al., 2016; Pérez-Castro et al., 2016; Ferreira-De-Lima and Lima-Camara, 2018), which can become an efficient strategy to maintain the constant circulation of arboviruses in a territory.
We reported the circulation of DENV-1-3 serotypes in A. aegypti mosquitoes. The highest frequency of infection by serotypes DENV-1 and DENV-2 was identified in all the departments studied, while DENV-3 was detected only in the department of Magdalena in one individual, and no infection by DENV-4 was detected (Figure 2). This finding is consistent with epidemiological trends for Colombia, which indicate that DENV-1 and DENV-2 are the predominant serotypes in the national territory (Gutierrez-Barbosa et al., 2020; Instituto Nacional De Salud, 2022), while DENV-3, followed by DENV-4 are the serotypes with the lowest incidence (Gutierrez-Barbosa et al., 2020). According to the historical dynamics of Dengue transmission in Colombia, DENV-1 and DENV-2 are responsible for the last epidemic outbreaks from 2010 to 2019. National registries report changes in the dominant serotype over time, evidencing the shift from the most prevalent serotype of DENV-1 toward DENV-2 between 2014 and 2016; and subsequently reported DENV-1 as the predominant serotype, followed by DENV-2 in 2019 (Instituto Nacional De Salud, 2019a,b, 2020; Gutierrez-Barbosa et al., 2020). Also, studies of natural infection in Aedes mosquitoes in central departments of the country in 2016 reported high rates of DENV-2 infection in A. aegypti, possibly associated with the epidemic that occurred during that year (Pérez-Castro et al., 2016; Ruiz-López et al., 2016; Gómez-Palacio et al., 2017; Pérez-Pérez et al., 2017). Similarly, reports of infection in Aedes during 2018 and 2019 showed DENV-1 infections related to the latest outbreak in Colombia (Martínez et al., 2020; Carrasquilla et al., 2021). Given this scenario, our findings on the frequency of infection in A. aegypti could be associated with this national trend and reveal the predominant serotype transition from serotype DENV-1 to DENV-2 in all departments, suggesting that the latter serotype could be the cause of the next outbreak in the country. In addition, some authors suggest that changing serotype prevalence has been associated with lineage extinction and replacement (Allicock et al., 2012; Salvo et al., 2019). However, epidemiological and entomovirological surveillance should be continued to verify these assertions coupled with genomic surveillance.
Interestingly, we demonstrate that in departments located in different biogeographic regions, there is an active circulation of DENV in A. aegypti mosquitoes. The department of Vichada presented the lowest frequency of DENV-1 infection (1.9%) and the highest frequency of DENV-2 infection (30.6%) in A. aegypti mosquitoes with significant differences compared to the other departments studied. These findings may be explained by the proportion of dependent serotypes per department and the local viral population dynamics, as previously reported for other Colombian departments (Jimenez-Silva et al., 2018; Gutierrez-Barbosa et al., 2020; Velandia-Romero et al., 2020). In addition, it may imply a regional evolutionary process of the virus probably associated with vector populations, immunological characteristics, and geographical barriers that prevent the homogenization of circulating serotypes (Gutierrez-Barbosa et al., 2020). Furthermore, the geographic proximity between Vichada and Venezuela probably reflects one of the most significant viral diffusion routes between geographic locations and shows a migratory pathway of Dengue viral lineages (Jimenez-Silva et al., 2018). Therefore, this information highlights the importance of comprehensive monitoring DENV transmission in transboundary mosquito and human regions, such as Vichada and even Amazonas (an area bordering Brazil), which can contribute to controlling the diversification, transmission, and persistence of strains or gene variants of this or other arboviruses.
The data on co-infections between species, serotypes, or viral genotypes in Aedes mosquitos are limited. However, laboratory evidence confirms that A. aegypti mosquitoes can be simultaneously or sequentially infected with different arbovirus species in all combinations and that they are capable of co-transmitting simultaneously without affecting vectorial competence (Nuckols et al., 2015; Goertz et al., 2017; Ruckert et al., 2017; Magalhaes et al., 2018; Mourya et al., 2018; Vogels et al., 2019). Here, we found natural co-infection in A. aegypti by different Dengue serotypes, with the highest frequency between DENV-1 and DENV-2 (2.9%, n = 16). Recent investigations in Colombia also found mixed infections in Aedes with serotypes DENV-1 and DENV-2, DENV-2 and DENV-4 (Pérez-Castro et al., 2016), DENV-4 and DENV-1 (Pena-Garcia et al., 2016), and by arboviral species DENV-CHIKV (Martínez et al., 2020), which could be related to the differences in the endemic and entomological patterns. Some reports indicate that Aedes mosquitoes have a high probability of infection by two or more DENV serotypes (Guzman et al., 2016; Vazeille et al., 2016), and may be related to increased susceptibility to infection by one of these serotypes. Currently, the implications of co-infection and co-transmission on the epidemiology, pathogenesis, and evolution of these viral agents remain unclear, mainly due to limited clinical information, underdiagnosis, and poor detection in both humans and mosquitoes (Carrillo-Hernandez et al., 2018; Mercado-Reyes et al., 2019; Vogels et al., 2019). Nevertheless, in regions where there is active co-circulation of arboviral agents, especially in areas with high endemicity for Dengue, it is essential to clarify the dynamics of infection in mosquitoes and possible implications for arboviral transmission and evolution. The implementation of accurate diagnostic tests for single or multiple arboviral detections deserves greater attention as part of epidemiological surveillance.
The present study shows that A. aegypti populations sampled in different departments of Colombia exhibited high haplotype diversity 0.948 ± 0.012, but medium nucleotide diversity (0.0225 ± 0.003) (Table 3). Similar results were found in studies on the genetic structure of A. aegypti in Latin American countries such as Brazil (Bracco et al., 2007), Mexico (Gorrochotegui-Escalante et al., 2002), Venezuela (Herrera et al., 2006), and Colombia (Jaimes-Duenez et al., 2015). The high diversity of haplotypes in different populations of A. aegypti in the continent indicates a rapid population demographic expansion in a relatively recent period (Joyce et al., 2018) and a gene flow between countries in the region (Jaimes-Duenez et al., 2015). In contrast, other countries such as Bolivia (Paupy et al., 2012) show low genetic diversity, probably related to geographical isolation and limited terrestrial access, which prevents reinvasion processes by A. aegypti in this country. Analysis of Tajima’s D test showed negative values in all study departments and significant values for A. aegypti samples in Amazonas and Boyacá (P < 0.05), suggesting an excess of rare alleles, which may reflect demographic instability and confirm the population expansion of this vector species (Paupy et al., 2012). These analyses are related to the haplotype network (Figure 3A), which shows the genetic diversity of A. aegypti in the study populations, with greater nucleotide diversity for samples from the department of Vichada. It also reveals a haplogroup among most Amazon sequences, which presented the lowest haplotype diversity, while departments with the highest haplotype diversity (Boyacá, Magdalena, and Vichada) are in the second-highest haplogroup. This may suggest that the Amazonas populations present some degree of isolation from the other A. aegypti populations in the study areas, while the populations of the other departments may present a greater genetic flow between them. As the Colombian Aedes populations from Amazonas seem to be different from the rest of the country, future studies should study the complex ecological, evolutionary, and epidemiological context of Amazonas, as this department limits with the Brazilian, Venezuelan, and Peruvian amazon comprising a fascinating hotspot for understanding Aedes population structure.
In addition, phylogenetic analyses of haplotype sequences from our study and haplotype sequences from other countries suggest the predominance of a Lineage I of A. aegypti in Colombia, where all sequences from Amazonas, Boyacá, Magdalena, and Vichada were related (Figure 3B). This lineage has been described as the most widespread in countries of the Americas (Herrera et al., 2006; Bracco et al., 2007; Paupy et al., 2012) and is related to West African ancestors (Moore et al., 2013). Recent studies on the genetic characteristics and population structure of A. aegypti in Colombia, based on COI and NAD4 (dehydrogenase subunit 4) molecular markers, show the existence of a second lineage (Lineage II), recently introduced to the country and related to East African analogs (Jaimes-Duenez et al., 2015). According to the authors, Lineage I is found in areas with a high incidence of Dengue and chemical insecticides, while Lineage II is present in cities with a low incidence of Dengue where chemical insecticides are not constant (Jaimes-Duenez et al., 2015). This suggests that the epidemiology of Dengue may be influenced by the population dynamics of A. aegypti in the territory. However, it is necessary to continue molecular epidemiology studies in this type of vector populations, including more molecular markers or even comparative genomics, which would help to clarify the associations between vector competence and the origin of the populations of Aedes species.
Finally, our study shows that entomovirological surveillance using molecular tools is a powerful tool that can support decision-making for preventing and controlling vector-borne diseases such as Dengue. Thus, individual detection of infection and/or co-infection by arboviruses (DENV, CHIKV, ZIKV) in field-collected Aedes mosquitoes, and molecular discrimination of vector species using molecular markers, can become a strategy that generates highly accurate entomological information. It could be used as an early warning system to support the adoption of vector control and prevention measures in emergency or re-emergence of arboviral outbreaks, especially in predicting infections up to six weeks before the onset of cases (Pena-Garcia et al., 2016). On the other hand, considering the installed capacity due to the Coronavirus disease (COVID-19) sanitary contingency (Alvarez-Diaz et al., 2021), health entities could take advantage of the Next Generation Sequencing (NGS) technology initiative of viral strains to detect new DENV lineages, in both humans and mosquitoes, and learn about regional dissemination patterns. These sequencing strategies, routinely implemented for pathogen detection and monitoring by public health agencies in Europe (Public Health England) and North America (Centers for Disease Control and Prevention, Public Health Agency of Canada), have enabled the successful surveillance of arboviruses such as West Nile virus (WNV) (Wollants et al., 2018) and Zika (Quick et al., 2017), which provided crucial information for early decision making to prevent the spread of these diseases, as well as assisting in the development of new schemes for the comprehensive management of these outbreaks.
It is essential to recognize some limitations in our study, such as the sample size of Aedes mosquitoes in the study areas, as well as the use of a single molecular marker to perform the population structure analysis, that would allow us to carry out a more robust analysis. We consider that our findings on entomovirological surveillance in different areas of Colombia provide valuable information that supports comprehensive surveillance and control systems for A. aegypti (Lineage I) as the primary vector of arboviruses in Colombia. Likewise, our findings highlight the importance of protecting the population at risk of serotype transmission, especially DENV-1 and DENV-2, and focusing on the potential serotype change in the next epidemic outbreak.
Conclusion
This study reports on the natural infection by DENV-1, DENV-2, DENV-3, and CHIKV of A. aegypti from departments in different biogeographical regions of Colombia. Furthermore, it confirms that co-infection with DENV serotypes in different combinations is possible. This demonstrates the high susceptibility of this species to arbovirus infection and confirms that A. aegypti is the primary vector in Colombia. The high rates of DENV infection may be associated with the high seroprevalence and asymptomatic infections in the human population, which may influence the constant maintenance of the arboviral infection cycle. In contrast, the low and null detection of CHIKV and ZIKV, respectively, may reflect the decrease in transmission after the outbreaks in 2015 and 2016. Finally, from the analysis of genetic diversity, we demonstrate the predominance of lineage I of A. aegypti in Colombia, widely spread in the Americas, and previously related to areas with a high incidence of Dengue. Our findings highlight the importance of including entomovirological surveillance to better understand its epidemiological dynamics and take preventive measures against future outbreaks. We suggest, especially to health and government entities, to take advantage of the installed capacity in the face of sanitary containment by COVID-19 to monitor the circulation of DENV serotypes and genotypes as a strategy for comprehensive disease management of arboviruses. We also recommend that future studies include and identify other disease-carrying vectors circulating in the area (e.g., Culex spp.). This would be useful to inform the public system of new viruses and vectors. Finally, we believe that our results on the molecular epidemiology of arboviruses in different areas of Colombia provide valuable information that supports institutional capacities for the prevention and control of arboviral agents.
Data availability statement
The original contributions presented in this study are included in the article/Supplementary material, further inquiries can be directed to the corresponding author.
Author contributions
JDR and MG conceived the study. RBM, LAS, MP-C, LMM, LGP, LEB, MAM, KAC, HDP, AZF, and JLD collected the samples. DM, LHP, and MG performed the processing of the samples in the laboratory and analysis. MG drafted the manuscript. DM, CH, NL, MM, and JDR contributed to interpretation and critical review. All authors read and approved the final manuscript.
Funding
This work was supported by Dirección de Investigación e Innovación from Universidad del Rosario. We thank the Ministerio de Ciencia y Tecnologia e Innovacion from Colombia in the framework of the call 891 “Convocatoria fortalecimiento de vocaciones y formacion en CTeI para la reactivacion economica en el marco de la postpandemia 2020” for funding LHP.
Acknowledgments
We thank the local health authorities from Amazonas, Boyacá, Magdalena, and Vichada departments for their support and assistance with the fieldwork. Equally, thanks to the Universidad del Rosario for covering the publication fees. We thank the Universidad de Boyacá for their support in financing MG’s doctoral studies. Also, we thank Sergio Castañeda for his expertise in statistical analysis.
Conflict of interest
CH was employed by Innovaseq S.A.S. LHP was employed by Upqua S.A.S.
The remaining authors declare that the research was conducted in the absence of any commercial or financial relationships that could be construed as a potential conflict of interest.
The handling editor JO declared a past co-authorship with the author JDR.
Publisher’s note
All claims expressed in this article are solely those of the authors and do not necessarily represent those of their affiliated organizations, or those of the publisher, the editors and the reviewers. Any product that may be evaluated in this article, or claim that may be made by its manufacturer, is not guaranteed or endorsed by the publisher.
Supplementary material
The Supplementary Material for this article can be found online at: https://www.frontiersin.org/articles/10.3389/fevo.2022.999169/full#supplementary-material
Footnotes
References
Allicock, O. M., Lemey, P., Tatem, A. J., Pybus, O. G., Bennett, S. N., Mueller, B. A., et al. (2012). Phylogeography and population dynamics of dengue viruses in the Americas. Mol. Biol. Evol. 29, 1533–1543. doi: 10.1093/molbev/msr320
Alvarez-Diaz, D. A., Valencia-Alvarez, E., Rivera, J. A., Rengifo, A. C., Usme-Ciro, J. A., Pelaez-Carvajal, D., et al. (2021). An updated RT-qPCR assay for the simultaneous detection and quantification of chikungunya, dengue and zika viruses. Infect. Genet. Evol. 93:104967. doi: 10.1016/j.meegid.2021.104967
Bhatt, S., Gething, P. W., Brady, O. J., Messina, J. P., Farlow, A. W., Moyes, C. L., et al. (2013). The global distribution and burden of Dengue. Nature 496, 504–507. doi: 10.1038/nature12060
Bonizzoni, M., Gasperi, G., Chen, X., and James, A. A. (2013). The invasive mosquito species Aedes albopictus: Current knowledge and future perspectives. Trends Parasitol. 29, 460–468. doi: 10.1016/j.pt.2013.07.003
Bracco, J. E., Capurro, M. L., Lourenco-De-Oliveira, R., and Sallum, M. A. (2007). Genetic variability of Aedes aegypti in the Americas using a mitochondrial gene: Evidence of multiple introductions. Mem. Inst. Oswaldo Cruz 102, 573–580. doi: 10.1590/S0074-02762007005000062
Brady, O. J., Golding, N., Pigott, D. M., Kraemer, M. U., Messina, J. P., and Reiner, R. C. Jr., et al. (2014). Global temperature constraints on Aedes aegypti and Ae. albopictus persistence and competence for dengue virus transmission. Parasit. Vectors 7:338. doi: 10.1186/1756-3305-7-338
Caron, M., Paupy, C., Grard, G., Becquart, P., Mombo, I., Nso, B. B., et al. (2012). Recent introduction and rapid dissemination of Chikungunya virus and Dengue virus serotype 2 associated with human and mosquito co-infections in Gabon, central Africa. Clin. Infect. Dis. 55, e45–e53. doi: 10.1093/cid/cis530
Carrasquilla, M. C., Ortiz, M. I., Leon, C., Rondon, S., Kulkarni, M. A., Talbot, B., et al. (2021). Entomological characterization of Aedes mosquitoes and arbovirus detection in Ibague, a Colombian city with co-circulation of Zika, dengue and chikungunya viruses. Parasit. Vectors 14:446. doi: 10.1186/s13071-021-04908-x
Carrillo-Hernandez, M. Y., Ruiz-Saenz, J., Villamizar, L. J., Gomez-Rangel, S. Y., and Martinez-Gutierrez, M. (2018). Co-circulation and simultaneous co-infection of Dengue, chikungunya, and zika viruses in patients with febrile syndrome at the Colombian-Venezuelan border. BMC Infect. Dis. 18:61. doi: 10.1186/s12879-018-2976-1
Cevallos, V., Ponce, P., Waggoner, J. J., Pinsky, B. A., Coloma, J., Quiroga, C., et al. (2018). Zika and Chikungunya virus detection in naturally infected Aedes aegypti in Ecuador. Acta Trop. 177, 74–80. doi: 10.1016/j.actatropica.2017.09.029
Chien, L. J., Liao, T. L., Shu, P. Y., Huang, J. H., Gubler, D. J., and Chang, G. J. (2006). Development of real-time reverse transcriptase PCR assays to detect and serotype dengue viruses. J. Clin. Microbiol. 44, 1295–1304. doi: 10.1128/JCM.44.4.1295-1304.2006
Ciota, A. T. (2019). The role of co-infection and swarm dynamics in arbovirus transmission. Virus Res. 265, 88–93. doi: 10.1016/j.virusres.2019.03.010
Ciota, A. T., Chin, P. A., Ehrbar, D. J., Micieli, M. V., Fonseca, D. M., and Kramer, L. D. (2018). Differential effects of temperature and mosquito genetics determine transmissibility of arboviruses by Aedes aegypti in Argentina. Am. J. Trop. Med. Hyg. 99, 417–424. doi: 10.4269/ajtmh.18-0097
Dos Santos, T. P., Cruz, O. G., Da Silva, K. A. B., De Castro, M. G., De Brito, A. F., Maspero, R. C., et al. (2017). Dengue serotype circulation in natural populations of Aedes aegypti. Acta Trop. 176, 140–143. doi: 10.1016/j.actatropica.2017.07.014
Faye, O., Faye, O., Diallo, D., Diallo, M., Weidmann, M., and Sall, A. A. (2013). Quantitative real-time PCR detection of Zika virus and evaluation with field-caught mosquitoes. Virol J. 10:311. doi: 10.1186/1743-422X-10-311
Ferreira-De-Lima, V. H., and Lima-Camara, T. N. (2018). Natural vertical transmission of dengue virus in Aedes aegypti and Aedes albopictus: A systematic review. Parasit. Vectors 11:77. doi: 10.1186/s13071-018-2643-9
Goertz, G. P., Vogels, C. B. F., Geertsema, C., Koenraadt, C. J. M., and Pijlman, G. P. (2017). Mosquito co-infection with Zika and chikungunya virus allows simultaneous transmission without affecting vector competence of Aedes aegypti. PLoS Negl. Trop. Dis. 11:e0005654. doi: 10.1371/journal.pntd.0005654
Golding, N., Wilson, A. L., Moyes, C. L., Cano, J., Pigott, D. M., Velayudhan, R., et al. (2015). Integrating vector control across diseases. BMC Med. 13:249. doi: 10.1186/s12916-015-0491-4
Gómez, W., and Zapata-Úsuga, G. (2019). Presencia de Aedes aegypti y Aedes albopictus (Diptera: Culicidae) en área rural del departamento de Santander, Colombia. Biosalud 18, 7–21.
Gómez-Palacio, A., Suaza-Vasco, J., Castaño, S., Triana, O., and Uribe, S. (2017). Infección de Aedes albopictus (Skuse, 1894) con el genotipo asiático-americano del virus del dengue serotipo 2 en Medellín y su posible papel como vector del dengue en Colombia. Biomédica 37, 135–142. doi: 10.7705/biomedica.v37i0.3474
Gordon, A., Gresh, L., Ojeda, S., Chowell, G., Gonzalez, K., Sanchez, N., et al. (2018). Differences in transmission and disease severity between 2 successive waves of Chikungunya. Clin. Infect. Dis. 67, 1760–1767. doi: 10.1093/cid/ciy356
Gorrochotegui-Escalante, N., Gomez-Machorro, C., Lozano-Fuentes, S., Fernandez-Salas, L., De Lourdes Munoz, M., Farfan-Ale, J. A., et al. (2002). Breeding structure of Aedes aegypti populations in Mexico varies by region. Am. J. Trop. Med. Hyg. 66, 213–222. doi: 10.4269/ajtmh.2002.66.213
Gould, E., Pettersson, J., Higgs, S., Charrel, R., and De Lamballerie, X. (2017). Emerging arboviruses: Why today? One Health 4, 1–13. doi: 10.1016/j.onehlt.2017.06.001
Gutierrez-Barbosa, H., Medina-Moreno, S., Zapata, J. C., and Chua, J. V. (2020). Dengue infections in Colombia: Epidemiological trends of a hyperendemic country. Trop. Med. Infect. Dis. 5:156. doi: 10.3390/tropicalmed5040156
Guzman, M. G., Gubler, D. J., Izquierdo, A., Martinez, E., and Halstead, S. B. (2016). Dengue infection. Nat. Rev. Dis. Primers 2:16055. doi: 10.1038/nrdp.2016.55
Herrera, F., Urdaneta, L., Rivero, J., Zoghbi, N., Ruiz, J., Carrasquel, G., et al. (2006). Population genetic structure of the dengue mosquito Aedes aegypti in Venezuela. Mem. Inst. Oswaldo Cruz 101, 625–633. doi: 10.1590/S0074-02762006000600008
Hoyos-Lopez, R., Suaza-Vasco, J., Rua-Uribe, G., Uribe, S., and Gallego-Gomez, J. C. (2016). Molecular detection of flaviviruses and alphaviruses in mosquitoes (Diptera: Culicidae) from coastal ecosystems in the Colombian Caribbean. Mem. Inst. Oswaldo Cruz 111, 625–634. doi: 10.1590/0074-02760160096
Instituto Nacional De Salud (2019b). Informe técnico entomológico de arbovirus–Colombia, 2018. Bogota, CO: Ministerio de Salud y Protección Social.
Instituto Nacional De Salud (2019a). Boletín epidemiológico semana 30. Dirección de vigilancia y análisis del riesgo en salud pública publicación en línea. Bogota, CO: Ministerio de Salud y Protección Social. doi: 10.33610/23576189.2019.30
Instituto Nacional De Salud (2020). Instituto nacional de salud. Boletín epidemiológico semana 41. Dirección de vigilancia y análisis del riesgo en salud pública publicación en línea: ISSN 2357-6189 2018. Bogota, CO: Ministerio de Salud y Protección Social. doi: 10.33610/23576189.2020.41
Instituto Nacional De Salud (2022). Instituto nacional de salud. Boletín epidemiológico semana 33. Dirección de vigilancia y análisis del riesgo en salud pública publicación en línea: ISSN 2357-6189 2018. Bogota, CO: Ministerio de Salud y Protección Social. doi: 10.33610/23576189.2019.20
Jaimes-Duenez, J., Arboleda, S., Triana-Chavez, O., and Gomez-Palacio, A. (2015). Spatio-temporal distribution of Aedes aegypti (Diptera: Culicidae) mitochondrial lineages in cities with distinct dengue incidence rates suggests complex population dynamics of the dengue vector in Colombia. PLoS Negl. Trop. Dis. 9:e0003553. doi: 10.1371/journal.pntd.0003553
Jimenez-Silva, C. L., Carreno, M. F., Ortiz-Baez, A. S., Rey, L. A., Villabona-Arenas, C. J., and Ocazionez, R. E. (2018). Evolutionary history and spatio-temporal dynamics of dengue virus serotypes in an endemic region of Colombia. PLoS One 13:e0203090. doi: 10.1371/journal.pone.0203090
Joyce, A. L., Torres, M. M., Torres, R., and Moreno, M. (2018). Genetic variability of the Aedes aegypti (Diptera: Culicidae) mosquito in El Salvador, vector of Dengue, yellow fever, chikungunya and Zika. Parasit. Vectors 11:637. doi: 10.1186/s13071-018-3226-5
Katoh, K., and Standley, D. M. (2013). MAFFT multiple sequence alignment software version 7: Improvements in performance and usability. Mol. Biol. Evol. 30, 772–780. doi: 10.1093/molbev/mst010
Kauffman, E. B., and Kramer, L. D. (2017). Zika virus mosquito vectors: Competence, biology, and vector control. J. Infect. Dis. 216, S976–S990. doi: 10.1093/infdis/jix405
Kazmi, S. S., Ali, W., Bibi, N., and Nouroz, F. (2020). A review on Zika virus outbreak, epidemiology, transmission and infection dynamics. J. Biol. Res. (Thessalon) 27:5. doi: 10.1186/s40709-020-00115-4
Kilpatrick, A. M., and Randolph, S. E. (2012). Drivers, dynamics, and control of emerging vector-borne zoonotic diseases. Lancet 380, 1946–1955. doi: 10.1016/S0140-6736(12)61151-9
Kirstein, O. D., Ayora-Talavera, G., Koyoc-Cardena, E., Chan Espinoza, D., Che-Mendoza, A., Cohuo-Rodriguez, A., et al. (2021). Natural arbovirus infection rate and detectability of indoor female Aedes aegypti from Merida. Yucatan, Mexico. PLoS Negl. Trop. Dis. 15:e0008972. doi: 10.1371/journal.pntd.0008972
Klungthong, C., Zhang, C., Mammen, M. P. Jr., Ubol, S., and Holmes, E. C. (2004). The molecular epidemiology of dengue virus serotype 4 in Bangkok, Thailand. Virology 329, 168–179. doi: 10.1016/j.virol.2004.08.003
Kraemer, M. U. G., Reiner, R. C. Jr., Brady, O. J., Messina, J. P., Gilbert, M., Pigott, D. M., et al. (2019). Past and future spread of the arbovirus vectors Aedes aegypti and Aedes albopictus. Nat. Microbiol. 4, 854–863. doi: 10.1038/s41564-019-0376-y
Kraemer, M. U., Sinka, M. E., Duda, K. A., Mylne, A. Q., Shearer, F. M., Barker, C. M., et al. (2015). The global distribution of the arbovirus vectors Aedes aegypti and Ae. albopictus. Elife 4:e08347. doi: 10.7554/eLife.08347
Leigh, J., and Bryant, D. (2015). POPART: Full-feature software for haplotype network construction. Methods Ecol. Evol. 6, 1110–1116. doi: 10.1111/2041-210X.12410
Leparc-Goffart, I., Nougairede, A., Cassadou, S., Prat, C., and De Lamballerie, X. (2014). Chikungunya in the Americas. Lancet 383:514. doi: 10.1016/S0140-6736(14)60185-9
Leta, S., Beyene, T. J., De Clercq, E. M., Amenu, K., Kraemer, M. U. G., and Revie, C. W. (2018). Global risk mapping for major diseases transmitted by Aedes aegypti and Aedes albopictus. Int. J. Infect. Dis. 67, 25–35. doi: 10.1016/j.ijid.2017.11.026
Liu-Helmersson, J., Quam, M., Wilder-Smith, A., Stenlund, H., Ebi, K., Massad, E., et al. (2016). Climate change and Aedes vectors: 21st century projections for dengue transmission in Europe. EBioMedicine 7, 267–277. doi: 10.1016/j.ebiom.2016.03.046
Lwande, O. W., Obanda, V., Lindstrom, A., Ahlm, C., Evander, M., Naslund, J., et al. (2020). Globe-trotting Aedes aegypti and Aedes albopictus: Risk factors for arbovirus pandemics. Vector Borne Zoonotic Dis. 20, 71–81. doi: 10.1089/vbz.2019.2486
Magalhaes, T., Robison, A., Young, M. C., Black, W. C. T., Foy, B. D., Ebel, G. D., et al. (2018). Sequential infection of Aedes aegypti Mosquitoes with Chikungunya virus and Zika virus enhances early Zika virus transmission. Insects 9:177. doi: 10.3390/insects9040177
Martina, B. E., Koraka, P., and Osterhaus, A. D. (2009). Dengue virus pathogenesis: An integrated view. Clin. Microbiol. Rev. 22, 564–581. doi: 10.1128/CMR.00035-09
Martínez, D., Hernández, C., Muñoz, M., Armesto, Y. A. C., and Jd, R. (2020). Identification of Aedes (Diptera: Culicidae) species and arboviruses circulating in Arauca, Eastern Colombia. Front. Ecol. Evol. 8:602190. doi: 10.3389/fevo.2020.602190
Mercado-Reyes, M., Acosta-Reyes, J., Navarro-Lechuga, E., Corchuelo, S., Rico, A., Parra, E., et al. (2019). Dengue, chikungunya and zika virus coinfection: Results of the national surveillance during the zika epidemic in Colombia. Epidemiol. Infect. 147:e77. doi: 10.1017/S095026881800359X
Ministerio De Salud Y Protección Social (2020). Lineamiento para la gestion y operación del programa de enfermedades transmitidas por vectores y zoonosis y otras consideraciones para la ejecución de sus transferencias nacionales de funcionamiento. Dirección de Promoción y Prevención, ed. S. D. E. T. G. Endemoepidémicas (Combia: Ministerio de Salud y Protección Social. República de Colmbia).
Monteiro, F. J. C., Mourao, F. R. P., Ribeiro, E. S. D., Rego, M., Frances, P., Souto, R. N. P., et al. (2020). Prevalence of dengue, Zika and chikungunya viruses in Aedes (Stegomyia) aegypti (Diptera: Culicidae) in a medium-sized city, Amazon, Brazil. Rev. Inst. Med. Trop. Sao Paulo 62:e10. doi: 10.1590/s1678-9946202062010
Moore, M., Sylla, M., Goss, L., Burugu, M. W., Sang, R., Kamau, L. W., et al. (2013). Dual African origins of global Aedes aegypti s.l. Populations revealed by mitochondrial DNA. PLoS Negl. Trop. Dis. 7:e2175. doi: 10.1371/journal.pntd.0002175
Mount, D. W. (2007). Using the basic local alignment search tool (BLAST). CSH Protoc. 2007:pdb top17. doi: 10.1101/pdb.top17
Mourya, D. T., Gokhale, M. D., Majumdar, T. D., Yadav, P. D., Kumar, V., and Mavale, M. S. (2018). Experimental Zika virus infection in Aedes aegypti: Susceptibility, transmission & co-infection with dengue & chikungunya viruses. Indian J. Med. Res. 147, 88–96. doi: 10.4103/ijmr.IJMR_1142_17
Murray, N. E., Quam, M. B., and Wilder-Smith, A. (2013). Epidemiology of Dengue: Past, present and future prospects. Clin. Epidemiol. 5, 299–309. doi: 10.2147/CLEP.S34440
Ngono, A. E., and Shresta, S. (2018). Immune response to dengue and zika. Annu. Rev. Immunol. 36, 279–308. doi: 10.1146/annurev-immunol-042617-053142
Nguyen, L. T., Schmidt, H. A., Von Haeseler, A., and Minh, B. Q. (2015). IQ-TREE: A fast and effective stochastic algorithm for estimating maximum-likelihood phylogenies. Mol. Biol. Evol. 32, 268–274. doi: 10.1093/molbev/msu300
Nuckols, J. T., Huang, Y. J., Higgs, S., Miller, A. L., Pyles, R. B., Spratt, H. M., et al. (2015). Evaluation of simultaneous transmission of chikungunya virus and dengue virus type 2 in infected Aedes aegypti and Aedes albopictus (Diptera: Culicidae). J. Med. Entomol. 52, 447–451. doi: 10.1093/jme/tjv017
Okonechnikov, K., Golosova, O., Fursov, M., and Team, U. (2012). Unipro UGENE: A unified bioinformatics toolkit. Bioinformatics 28, 1166–1167. doi: 10.1093/bioinformatics/bts091
Padilla, J., Lizarazo, F., Murillo, O., Mendigaña, F., Pachón, E., and Vera, M. (2017). Epidemiología de las principales enfermedades transmitidas por vectores en Colombia, 1990–2016. Biomedica 37, 27–40. doi: 10.7705/biomedica.v37i0.3769
Paixao, E. S., Teixeira, M. G., and Rodrigues, L. C. (2018). Zika, chikungunya and Dengue: The causes and threats of new and re-emerging arboviral diseases. BMJ Glob. Health 3:e000530. doi: 10.1136/bmjgh-2017-000530
Patterson, J., Sammon, M., and Garg, M. (2016). Dengue, zika and chikungunya: Emerging arboviruses in the new world. West. J. Emerg. Med. 17, 671–679. doi: 10.5811/westjem.2016.9.30904
Paupy, C., Le Goff, G., Brengues, C., Guerra, M., Revollo, J., Barja Simon, Z., et al. (2012). Genetic structure and phylogeography of Aedes aegypti, the Dengue and yellow-fever mosquito vector in Bolivia. Infect. Genet. Evol. 12, 1260–1269. doi: 10.1016/j.meegid.2012.04.012
Peña-Garcia, V. H., Triana-Chavez, O., and Arboleda-Sanchez, S. (2017). Estimating Effects of temperature on dengue transmission in Colombian Cities. Ann. Glob. Health 83, 509–518. doi: 10.1016/j.aogh.2017.10.011
Pena-Garcia, V. H., Triana-Chavez, O., Mejia-Jaramillo, A. M., Diaz, F. J., Gomez-Palacio, A., and Arboleda-Sanchez, S. (2016). Infection rates by dengue virus in mosquitoes and the influence of temperature may be related to different endemicity patterns in three Colombian Cities. Int. J. Environ. Res. Public Health 13:734.
Pereira, L., Brito, M. C. A., Araruna, F. B., De Andrade, M. S., Moraes, D. F. C., Borges, A. C. R., et al. (2017). Molecular studies with Aedes (Stegomyia) aegypti (Linnaeus, 1762), mosquito transmitting the dengue virus. Parasitol. Res. 116, 2057–2063. doi: 10.3390/ijerph13070734
Pérez-Castro, R., Castellanos, J. E., Olano, V. A., Matiz, M., Jaramillo, J., Vargas, S., et al. (2016). Detection of all four dengue serotypes in Aedes aegypti female mosquitoes collected in a rural area in Colombia. Mem. Inst. Oswaldo Cruz 111, 233–240. doi: 10.1007/s00436-017-5484-0
Pérez-Pérez, J., Sanabria, W., Restrepo, C., Rojo, R., Henao, E., Triana, O., et al. (2017). Vigilancia virológica de Aedes (Stegomyia) aegypti yAedes (Stegomyia) albopictus como apoyo para la adopción de decisiones en el control del dengue en Medellín. Biomédica 37, 155–166. doi: 10.1590/0074-02760150363
Pollett, S., Melendrez, M. C., Maljkovic Berry, I., Duchene, S., Salje, H., Cummings, D. A. T., et al. (2018). Understanding dengue virus evolution to support epidemic surveillance and counter-measure development. Infect. Genet. Evol. 62, 279–295. doi: 10.7705/biomedica.v37i0.3467
Quick, J., Grubaugh, N. D., Pullan, S. T., Claro, I. M., Smith, A. D., Gangavarapu, K., et al. (2017). Multiplex PCR method for MinION and Illumina sequencing of Zika and other virus genomes directly from clinical samples. Nat. Protoc. 12, 1261–1276. doi: 10.1016/j.meegid.2018.04.032
Ramos-Castaneda, J., Barreto Dos, Santos, F., Martinez-Vega, R., Galvao, De Araujo, J. M., et al. (2017). Dengue in latin America: Systematic review of molecular epidemiological trends. PLoS Negl. Trop. Dis. 11:e0005224. doi: 10.1038/nprot.2017.066
Rozas, J., Ferrer-Mata, A., Sanchez-Delbarrio, J. C., Guirao-Rico, S., Librado, P., Ramos-Onsins, S. E., et al. (2017). DnaSP 6: DNA sequence polymorphism analysis of large data sets. Mol. Biol. Evol. 34, 3299–3302. doi: 10.1371/journal.pntd.0005224
Ruckert, C., Weger-Lucarelli, J., Garcia-Luna, S. M., Young, M. C., Byas, A. D., Murrieta, R. A., et al. (2017). Impact of simultaneous exposure to arboviruses on infection and transmission by Aedes aegypti mosquitoes. Nat. Commun. 8:15412. doi: 10.1093/molbev/msx248
Ruiz-López, R., González-Mazo, A., Vélez-Mira, A., Gómez, G., Zuleta, L., and Vélez-Bernal, I. (2016). Presencia de Aedes (Stegomyia) aegypti (Linnaeus, 1762) y su infección natural con el virus del dengue en alturas no registradas para Colombia. Biomédica 36, 303–308. doi: 10.1038/ncomms15412
Ryan, S. J., Carlson, C. J., Mordecai, E. A., and Johnson, L. R. (2019). Global expansion and redistribution of Aedes-borne virus transmission risk with climate change. PLoS Negl. Trop. Dis. 13:e0007213. doi: 10.7705/biomedica.v36i2.3301
Salvo, M. A., Aliota, M. T., Moncla, L. H., Velez, I. D., Trujillo, A. I., Friedrich, T. C., et al. (2019). Tracking dengue virus type 1 genetic diversity during lineage replacement in an hyperendemic area in Colombia. PLoS One 14:e0212947. doi: 10.1371/journal.pntd.0007213
Santiago, G. A., Vergne, E., Quiles, Y., Cosme, J., Vazquez, J., Medina, J. F., et al. (2013). Analytical and clinical performance of the CDC real time RT-PCR assay for detection and typing of dengue virus. PLoS Negl. Trop. Dis. 7:e2311. doi: 10.1371/journal.pone.0212947
Scarpassa, V. M., Cardoza, T. B., and Cardoso Junior, R. P. (2008). Population genetics and phylogeography of Aedes aegypti (Diptera: Culicidae) from Brazil. Am. J. Trop. Med. Hyg. 78, 895–903. doi: 10.1371/journal.pntd.0002311
Sukhralia, S., Verma, M., Gopirajan, S., Dhanaraj, P. S., Lal, R., Mehla, N., et al. (2019). From dengue to zika: The wide spread of mosquito-borne arboviruses. Eur. J. Clin. Microbiol. Infect. Dis. 38, 3–14. doi: 10.4269/ajtmh.2008.78.895
Vazeille, M., Gaborit, P., Mousson, L., Girod, R., and Failloux, A. B. (2016). Competitive advantage of a dengue 4 virus when co-infecting the mosquito Aedes aegypti with a dengue 1 virus. BMC Infect. Dis. 16:318. doi: 10.1007/s10096-018-3375-7
Velandia-Romero, M. L., Coronel-Ruiz, C., Castro-Bonilla, L., Camacho-Ortega, S., Calderon-Pelaez, M. A., Castellanos, A., et al. (2020). Prevalence of dengue antibodies in healthy children and adults in different Colombian endemic areas. Int. J. Infect. Dis. 91, 9–16. doi: 10.1186/s12879-016-1666-0
Vogels, C. B. F., Ruckert, C., Cavany, S. M., Perkins, T. A., Ebel, G. D., and Grubaugh, N. D. (2019). Arbovirus co-infection and co-transmission: A neglected public health concern? PLoS Biol. 17:e3000130. doi: 10.1016/j.ijid.2019.10.045
Wilder-Smith, A., Gubler, D. J., Weaver, S. C., Monath, T. P., Heymann, D. L., and Scott, T. W. (2017). Epidemic arboviral diseases: Priorities for research and public health. Lancet Infect. Dis. 17, e101–e106. doi: 10.1371/journal.pbio.3000130
Wilder-Smith, A., Ooi, E. E., Vasudevan, S. G., and Gubler, D. J. (2010). Update on Dengue: Epidemiology, virus evolution, antiviral drugs, and vaccine development. Curr. Infect. Dis. Rep. 12, 157–164. doi: 10.1016/S1473-3099(16)30518-7
Wollants, E., Smolders, D., Naesens, R., Bruynseels, P., Lagrou, K., Matthijnssens, J., et al. (2018). Use of next-generation sequencing for diagnosis of west nile virus infection in patient returning to Belgium from Hungary. Emerg. Infect. Dis. 24, 2380–2382. doi: 10.1007/s11908-010-0102-7
Keywords: arbovirus, Dengue, Zika, Chikungunya, infection, Aedes
Citation: Gómez M, Martínez D, Hernández C, Luna N, Patiño LH, Bohórquez Melo R, Suarez LA, Palma-Cuero M, Murcia LM, González Páez L, Estrada Bustos L, Medina MA, Ariza Campo K, Padilla HD, Zamora Flórez A, De las Salas JL, Muñoz M and Ramírez JD (2022) Arbovirus infection in Aedes aegypti from different departments of Colombia. Front. Ecol. Evol. 10:999169. doi: 10.3389/fevo.2022.999169
Received: 20 July 2022; Accepted: 24 August 2022;
Published: 16 September 2022.
Edited by:
Jader Oliveira, University of São Paulo, BrazilReviewed by:
Fabiana Feitosa-Suntheimer, Boston University, United StatesPedro Augusto Alves, René Rachou Institute, Brazil
Copyright © 2022 Gómez, Martínez, Hernández, Luna, Patiño, Bohórquez Melo, Suarez, Palma-Cuero, Murcia, González Páez, Estrada Bustos, Medina, Ariza Campo, Padilla, Zamora Flórez, De las Salas, Muñoz and Ramírez. This is an open-access article distributed under the terms of the Creative Commons Attribution License (CC BY). The use, distribution or reproduction in other forums is permitted, provided the original author(s) and the copyright owner(s) are credited and that the original publication in this journal is cited, in accordance with accepted academic practice. No use, distribution or reproduction is permitted which does not comply with these terms.
*Correspondence: Juan David Ramírez, anVhbmQucmFtaXJlekB1cm9zYXJpby5lZHUuY28=; anVhbi5yYW1pcmV6Z29uemFsZXpAbW91bnRzaW5haS5vcmc=