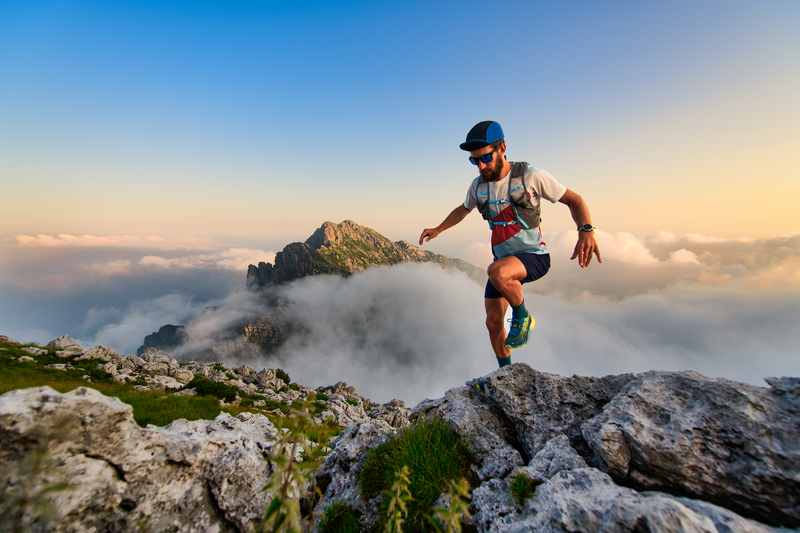
95% of researchers rate our articles as excellent or good
Learn more about the work of our research integrity team to safeguard the quality of each article we publish.
Find out more
ORIGINAL RESEARCH article
Front. Ecol. Evol. , 22 August 2022
Sec. Ecophysiology
Volume 10 - 2022 | https://doi.org/10.3389/fevo.2022.974449
This article is part of the Research Topic Forest Adaptation to Extreme Environments and Climate Changes View all 5 articles
Ectomycorrhizal fungi (EMF) are widespread in northern conifer forests. By competing with the free-living saprotrophic fungal and bacterial communities for limited soil nitrogen, EMF are expected to suppress litter decomposition and thus drive soil carbon accumulation. The EMF may also stimulate rhizosphere microbial growth through inputs of labile plant carbon, and subsequently contribute to the soil carbon pool via microbial necromass. Here we examined the relative strength of these two potential EMF effects in a northern conifer plantation of the Saihanba Forest, the largest plantation in China. The soil fungal and bacterial biomass, as well as their respiration, were quantified within the two types of soil cores that either allow or exclude the ingrowth of EMF. We also set up a nitrogen fertilization gradient (0, 5, 10, 15 g N m–2 y–1) in this plantation to quantify the influence of external inorganic nitrogen on the EMF effects. We found evidence that EMF inhibit the overall fungal and bacteria biomass, confirming the suppression of saprotrophs by EMF. In addition, high levels of external nitrogen fertilization (15 g N m–2 y–1) might further enhance the suppression by EMF. In contrast, the presence of EMF consistently increased soil microbial respiration across all nitrogen fertilization levels, indicating that the carbon allocated to EMF could have been largely consumed by microbial respiration and contributed minimally to the accumulation of microbial biomass. Our results also indicated that the suppression of saprotrophs by EMF may play a critical role in driving continuous soil carbon accumulation in this northern pine plantation under atmospheric nitrogen deposition.
Ectomycorrhizal fungi (EMF) are widespread in northern conifer forests that function as a major terrestrial carbon (C) sink (Tagesson et al., 2020). EMF play a critical role in tree survival and vegetative C sequestration in these nitrogen (N)-limited forests (Smith and Read, 2008). Increasing evidence is also showing that EMF can drive soil C accumulation in some ecosystems, partly via the suppression of soil organic matter decomposition, a phenomenon known as the “Gadgil effect” (Gadgil and Gadgil, 1971; Fernandez and Kennedy, 2016). One of the possible underlying mechanisms responsible for the “Gadgil effect” is that soil saprotrophs (SAP, saprotrophic fungi and bacteria) are often considered as stronger decomposers of soil organic matter than EMF, while EMF are stronger competitors for soil N than SAP (Fernandez and Kennedy, 2016). The growth of SAP may be hindered by EMF as soil N becomes increasingly limited, resulting in slowed decomposition of the soil organic matter (Figure 1).
Figure 1. Diagram showing the influence of ectomycorrhizal fungi (EMF) on soil carbon (C) accumulation through two potential pathways. The first pathway is nitrogen (N)-centered (light-gray arrows), in which saprotrophs (SAP) were suppressed by EMF via N competition. We asked if external N fertilization can relieve the N suppression and stimulate SAP. The second pathway is C-centered (dark-gray arrows), in which EMF transfer plant-derived C to the soil C through microbial necromass. We asked whether the plant C transported to EMF can significantly contribute to microbial biomass, or would have mainly been consumed through microbial respiration.
As the ectomycorrhizal suppression of SAP is mainly driven by N limitation, external inorganic N fertilization may relieve the Gadgil effect. In addition, inputs of inorganic N often trigger plants to reduce C allocation to EMF (Hasselquist et al., 2012; Kjøller et al., 2012), which may limit the EMF activity and relieve the suppression of SAP. Therefore, the widespread atmospheric N deposition across the northern conifer forests may reduce soil carbon accumulation via the Gadgil effect. Indeed, a recent continental-scale meta-analysis showed that the impacts of ectomycorrhizas on soil C accumulation have been weakened under atmospheric N deposition (Averill et al., 2018). Examining the responses of EMF-SAP interactions to elevated N is thus critical for a better prediction of soil C dynamics in northern conifer forests.
To examine the EMF-SAP interactions under N fertilization, it is necessary to isolate the effects of EMF from their host roots. The host roots of EMF also compete for inorganic N with soil microorganisms (Kaye and Hart, 1997), and previous studies have often examined the impacts of the ectomycorrhizal symbioses (EMF + roots) on the decomposition of soil organic matter (Gadgil and Gadgil, 1971; Koide and Wu, 2003; Averill and Hawkes, 2016). A recent study suggests that roots can more strongly influence the rhizosphere carbon and nutrient dynamics than the associated EMF since the root-derived condensed tannins can stabilize the soil organic N (Adamczyk et al., 2019), and EMF alone may not suppress its decomposition (Carteron et al., 2021). Yet, there is evidence that it is the type of mycorrhizal fungi associated with the roots (i.e., EMF vs. arbuscular mycorrhizal fungi), rather than the roots per se, that predicts the outcome of the N competition with SAP (Averill et al., 2014, 2018), suggesting the critical role of EMF alone in suppression of SAP. Moreover, compared to saprotrophic fungi, bacteria were often not considered in the EMF-SAP interactions in previous studies (Fernandez and Kennedy, 2016; Sterkenburg et al., 2018; Fernandez et al., 2020; but see Maaroufi et al., 2019), even though bacteria are also N acquirers and many bacterial taxa are decomposers. It is important to distinguish the responses of saprotrophic fungi and bacteria to the presence of EMF and N fertilization given their differences in stoichiometry and physiology (Cleveland and Liptzin, 2007).
In addition to the Gadgil effect, EMF could potentially drive soil C accumulation by transporting photosynthate to the pool of microbial biomass and necromass (Figure 1; Hobbie, 2006; Ekblad et al., 2013; Zak et al., 2019). A recent meta-analysis suggests that more than half of the global soil organic matter is transferred from plant C to the necromass of EMF and other soil microorganisms (Liang et al., 2019). The accumulation of soil C in northern ecosystems could largely originate from mycorrhizas rather than from aboveground plant litter in northern forests (Clemmensen et al., 2013). In theory, when SAP were suppressed by EMF, the reduction of SAP biomass may be compensated for by increased biomass of EMF (i.e., niche filling), which might result in relatively unchanged overall microbial biomass. On the other hand, the EMF exudation of labile C compounds and turnover of EMF necromass may feed the free-living microbes (i.e., priming effect, Kuzyakov, 2002), which potentially increases the overall microbial biomass. However, this EMF-derived C allocation to the soil microbial pool could be minimal if the allocated C is primarily consumed by the respiration of soil microorganisms (Högberg et al., 2001), including EMF (Figure 1). Previous studies showed that EMF could respire more carbon than their host roots (Heinemeyer et al., 2007). Thus, quantifying the response of microbial biomass and microbial respiration to the presence of EMF is thus important for a holistic understanding of EMF-driven soil C accumulation.
In this study, we test the influence of EMF on saprotrophs through two potential pathways (Figure 1). One is a nitrogen-centered pathway, in which SAP was suppressed by EMF through N competition; and the other is a carbon-centered pathway, in which EMF transport plant C to the soil C through microbial biomass and necromass. Since the Gadgil effect is expected to be mainly driven by N competition between EMF and SAP, we hypothesized that external inorganic N inputs would reduce the suppression of SAP from N limitation caused by EMF. Also, because northern conifers are strongly dependent on EMF activities in nutrient acquisition, we hypothesized that the presence of EMF could increase the overall soil microbial respiration rather than microbial biomass. We created two types of in-situ soil cores in a temperate conifer plantation in northern China. One type of core included SAP (bacteria and saprotrophic fungi) and EMF hyphae, and the other type of core included SAP only. We applied a N fertilization gradient to both cores to examine the potential response of EMF-SAP interactions to increased soil N availability.
Our study site is located in a northern temperate conifer plantation (42.413°N, 117.241°E, 1466 masl, Figure 2) of the Saihanba National Forestry Park in Hebei Province, which is the largest area of plantations in China (94,700 ha). In this area, conifer species were widely planted since 1960s after the primary forests were clear-cut. In the study site, tree density was on average 3,460 stems ha–1. Evergreen Scots pine (Pinus sylvestris var. mongolica) represented ∼85% of the number of stems and the remaining trees were deciduous Prince Rupprecht’s larch (Larix principis-rupprechtii, ∼15%). Fire suppression had been practiced for several decades in this area, resulting in a thick forest litter layer. Understory vegetation was mostly herbaceous and sparse. In 2009, the conifer trees in the study site were ∼30 years old, with an average tree height of 10.1 m and diameter at breast height of 12.1 cm. In the belowground, absorptive root biomass (first three root orders, McCormack et al., 2015) was 608 g m–2 within the top 30 cm soil depth. Annual temperature in this area was −1.2°C (−12.8°C in January and 14.7°C in July), and annual precipitation was 450.1 mm. Soils were classified as Aeolian sandy soils, with an atmospheric wet N deposition of around 1 g N m–2 y–1.
Figure 2. Nitrogen fertilization plots and installation of the mesh collar tubes. (A) The 20 m × 20 m plots were established with four levels of N fertilization. Each level has three replicate plots. Fertilization began in 2009 and conducted three times a year since then. (B) In each plot, there were four pairs of T tubes and M tubes for soil respiration measurement and two pairs for PLFA assay. The M tube is a 30 cm long PVC tube with eight circular windows (2.5 cm diameter) drilled in the upper 10 cm of the tube. Windows were covered with nylon frame in a 40-μm mesh size (insert image). To install an M tube, a T tube was first installed. After 2 months, the T tube and its inside soil core were lifted. The M tube was inserted into the resulting hole, and the intact soil core was refilled. Respiration was measured 2 years after tube installation.
Beginning in August of 2009, 12 plots were set up for N fertilization experiment across the plantation. Each plot was 20 m × 20 m in area and was separated by 10 m buffers. Plots were established with four levels of N fertilization: (1) control, no fertilization; (2) low, N added to soil at a level of 5 g N m–2 y–1, (3) medium, at a level of 10 g N m–2 y–1, and (4) high, at a level of 15 g N m–2 y–1. Each level has three replicate plots and the overall 12 plots were deployed using a Latin-square design. We used urea as N fertilizer, which was dissolved in 20 L water and applied as a spray right after rain, which could promote the infiltration of the fertilizer to the soil. In control plots, 20 L of water was sprayed. Fertilization was conducted three times a year (May, July, and September) since 2009.
We created in-situ soil cores with SAP only and cores with SAP + EMF in our control and N fertilization plots. First, we used 30-cm length, 10-cm diameter polyvinyl chloride (PVC) tubes as trenching tubes (T tubes, SAP only). Second, we used the same size PVC tubes, but drilled eight windows (2.5-cm-diameter circles) in the upper 10 cm of each tube (Figure 2). The windows were covered with 40-μm nylon mesh (Normesh Ltd., Oldham, United Kingdom) to make the mesh-tubes (M tubes, SAP + EMF). We used hammers to install the T tubes into the soil in June 2009. Because the M tubes were too fragile to be hammered, we first hammered a T tube into the soil right next to its paired tube. Two months later (August 2009), we lifted the whole T tube (PVC wall and soil core) and inserted an M tube into the resultant hole. The intact soil core including surface litter layer was retrieved from the lifted T tube and refilled into the M tube, retaining the soil structure and horizons to minimize disturbance (Figure 2). We installed four pairs of M and T tubes in each plot for respiration measurements. The location of each paired M tube and T tube was randomly assigned within the plot prior to installation. All M and T tubes remained undisturbed after installation.
Two years after tube installation, microbial respiration from M and T tubes was measured from May to September 2011. Respiration rate (CO2 efflux rate) was measured using a Li-8100 soil CO2 flux system (LI-COR Inc., Lincon, NE, United States) every 10 days (if weather conditions were acceptable). Right after each respiration measurement, the soil temperature and soil moisture was recorded inside each tube at 5-cm soil depth. Background soil temperature and moisture were also measured near each pair of tubes. Soil temperature was recorded using the Li-8100 temperature probe, and soil moisture (volumetric water content) was measured using time domain reflectometry (TDR, Soil Moisture Equipment Corp., Santa Barbara, CA, United States). In total, there were 864 sets of measurements of respiration, temperature, and moisture across all tubes. All measurements were conducted from 10:00 to 16:00.
We examined the influence of N fertilization on soil mineral N dynamics in 2011. After the fertilization on May 11, the forest litter layer and 0–10 cm soils were sequentially sampled on May 13, 16, 19, 22, and 24 in each plot (three replicates per plot). Soil extracts were analyzed by continuous flow analyzer (TRAACS 2000; Bran and Luebbe, Norderstedt, Germany) to determine soil mineral N (NH4+-N and NO3–-N) concentrations.
In the summer of 2011, two new pairs of M and T tubes were installed at random locations in each plot for microbial biomass assessment. Tubes were deployed using the same procedures described above, with T tubes installed in June and M tubes in August. In early October 2011 (before snow), 0–10 cm soils inside the tubes were cored (5-cm-diameter) and kept frozen for later analysis in the lab. We employed phospholipid fatty acid analysis (PLFA) to assess the microbial biomass in these soil samples as described by Bossio and Scow (1998). Briefly, polar lipids in initial soil extracts were separated from neutral and glycolipids by elution with 5 ml chloroform and 10 ml acetone followed by 5 ml methanol. Mild alkaline methanolysis was done on the polar lipid fraction. The peak area of each resulting fatty acid methyl ester was recorded on the chromatogram for each sample before identification. Peaks were identified by chromatographic retention time and a standard qualitative mix that ranged from C9 to C30 using a microbial identification system (Microbial ID Inc., Newark, DE, United States). The sum of the i14:0, i15:0, a15:0, 15:0, i16:0, 10Me16:0, i17:0, a17:0, cy17:0, 17:0, br18, 10Me17:0, 18:1ω7, 10Me18:0, and cy19:0 markers was used a measure of the bacterial biomass. The sum of 18:1ω6 and 18:2ω9 was used as a measure of fungal biomass.
Soil microenvironment (e.g., temperature and moisture) may differ between the paired T tube and M tube, which consequently influences the measured microbial respiration rate. Thus, we adjusted the respiration rate measured in each pair of M and T tube to the same temperature and moisture. We first fitted an exponential regression between the raw respiration rate (R) and soil temperature at each N level of each tube type. The R was then converted to respiration rate at 15°C (R15) using the fitted exponential regression function. Second, we calculated the difference of R15 between the paired M tube and T tube (R15_diff) in each measurement and explored its potential linear relationships with the corresponding difference in soil moisture across the measurement of each N fertilization level.
where SWM and SWT were the soil moisture at the time of respiration measurements for M tubes and T tubes; a was the fitted intercept and b was the slope of the linear regression. With the fitted slope b obtained from Eq. 1, we removed the impacts of difference of soil moisture on R15_diff using Eq. 2.
where Adj_R15_diff was the adjusted difference of respiration rate between the paired M tube and T tube with the same soil temperature (15°C) and soil moisture (moisture in T tube of each pair).
Finally, the fungal and bacterial PLFAs of M tubes and T tubes, as well as their differences were regressed to the N fertilization gradients. All statistics were performed using R (R Core Team, 2021; version 4.1.1; R Foundation for Statistical Computing). Data are expressed as mean ± standard error.
In general, there were significant (or marginally significant) positive correlations between mineral N concentration and N fertilization levels in the litter layer, as well as in the 0–10 cm soil layer except those on 5/22 and 5/24. Thus, the positive influence of fertilization persisted for at least 2 weeks following fertilization on the forest litter floor, and lasted for nearly 10 days in the 0–10 cm soil layer (Figure 3). Fungal and bacterial PLFA were negatively and significantly (or marginally significantly) influenced by N fertilization levels in the M Tubes (r2 = 0.15, P = 0.05 for bacteria, and r2 = 0.12, P = 0.10 for fungi) but not in the T tubes (P = 0.31 for bacteria and P = 0.42 for fungi, Figures 4A–D). The EMF impacts on microbial PLFAs (i.e., differences of PLFA between M tubes and T tubes) varied largely within and among N fertilization levels (Figures 4E,F), and negatively correlated with the N fertilization levels for both bacteria (r2 = 0.17, P = 0.04) and fungi (r2 = 0.13, P = 0.08) (Figures 4E,F). In control plots, the EMF induced PLFA changes were 6.1% in bacteria (P = 0.74) and 7.9% in fungi (P = 0.70). At the 5 g N m–2 y–1 fertilization level, the changes were still positive, with 8.5% in bacteria (P = 0.54) and 7.0% in fungi (P = 0.67). At the 10 g N m–2 y–1 fertilization level, the EMF-induced PLFA changes shifted from small increase to moderate reduction, with −31.5% in bacteria (P = 0.23) and −30.8% in fungi (P = 0.21). At the highest fertilization level, the PLFA changes induced by EMF significantly (or marginally significantly) differ from 0, with −30.3% in bacteria (P = 0.03) and −25.8% in fungi (P = 0.09).
Figure 3. The relationship between mineral nitrogen concentration (NO3–-N+ NH4+-N) and N fertilization levels in both forest litter layer (left) and 0–10 cm soil layer. Fertilization was applied three times a year since 2009, and the last N application was on 5/11/2011. Mineral N concentration assay was performed on 5/13, 5/16, 5/19, 5/22, and 5/24/2011. Significant (or marginally significant) positive correlations were found between mineral N concentration and N fertilization levels in the litter layer (r2 = 0.32, P < 0.001 for 5/13, r2 = 0.29, P < 0.001 for 5/16, r2 = 0.38, P < 0.001 for 5/19, r2 = 0.26, P = 0.001 for 5/22, and r2 = 0.17, P = 0.013 for 5/24), as well as in the 0–10 cm soil layer except those on 5/22 and 5/24 (r2 = 0.41, P < 0.001 for 5/13, r2 = 0.10, P = 0.06 for 5/16, r2 = 0.14, P = 0.025 for 5/19, P = 0.84 for 5/22, and P = 0.51 for 5/24).
Figure 4. Response of fungal and bacterial PLFAs, as well as ectomycorrhizal fungi (EMF) induced PLFA changes to N fertilization levels. The PLFAs were extracted from soil at 0–10 cm. The bacterial and fungal PLFA negatively and significantly (or marginally significantly) responded to increased N fertilization only in the M tubes (r2 = 0.15, P = 0.05 for bacteria and r2 = 0.12, P = 0.10 for fungi) (A,B), but not in the T tubes (P = 0.31 for bacteria and P = 0.42 for fungi) (C,D). The EMF induced PLFA changes (M tube—T tube) also negatively and significantly (or marginally significantly) responded to increased N fertilization for both bacteria (r2 = 0.17, P = 0.04) and fungi (r2 = 0.13, P = 0.08) (E,F).
We found significantly greater soil moisture, but unchanged soil temperature in the M tubes compared to the T tubes across the 864 measurements throughout the growing season in 2011 (Table 1 and Supplementary Figure 1). On average, soil moisture was 19.5% higher in M tubes than in T tubes. The raw respiration rates were also higher in M tubes than in T tubes (except those in the 10 g N m–2 y–1 plots) (Table 1), and exhibited exponential relationships with soil temperature for both tube treatments (Figures 5A,B). The difference in respiration rate at 15°C between the paired M and T tubes (R15_diff) was negatively and significantly correlated with their differences in soil moisture at each fertilization level (Figure 5C). The Adj_R15_diff, which quantified the difference of respiration between the paired M tube and T tube with the same soil temperature (15°C) and soil moisture (moisture in T tube of each pair), was on average greater than 0 throughout the growing season, except in the 10 g N m–2 y–1 plots (Figure 5D). Even within the 10 g N m–2 y–1 plots, the Adj_R15_diff was on average positive on 8 out of 9 measurement days (Figure 5D). Thus, adjusted microbial respiration was greater in M tubes than in T tubes, despite that the overall microbial biomass was not increased with the presence of EMF (Figures 4, 5).
Table 1. Soil temperature and moisture at 5 cm depth and soil respiration of both M tubes and T tubes for each fertilization level.
Figure 5. Raw and adjusted respiration (CO2 efflux) rates of different fertilization levels in 2011. Exponential relationship between raw respiration rate and soil temperature (5 cm depth) in both M tubes (A) and T tubes (B). Linear regression of the differences in respiration rate at 15°C between the paired M tubes and T tubes (R15_diff) against the corresponding differences in soil moisture (C). The seasonal dynamics (May to September) of the Adj_R15_diff, which was the adjusted difference of respiration between the paired M tubes and T tubes with the same soil temperature (15°C) and soil moisture (moisture in T tube of each pair) (D). Both M tubes and T tubes were installed in 2009.
In this study, we focused on the interactions between EMF and SAP that might influence the soil C dynamics. It has been shown in some ecosystems that, competition between SAP and ectomycorrhizas (roots + EMF) for limited N plays a critical role in driving global soil C accumulation (Fernandez and Kennedy, 2016; Figure 1). We showed evidence that the presence of EMF alone, without the symbiotic roots, could result in suppression of SAP in our northern pine plantation, the largest plantation in China. Although we did not directly measure the biomass of saprotrophic and mycorrhizal fungi, we found that the overall fungal biomass in the tubes with both EMF and SAP was either unchanged or decreased from that in the tubes with SAP only (Figure 4F). Since EMF often constitute a significant portion of the soil microbial biomass (Högberg and Högberg, 2002), the saprotrophic fungal biomass has to be decreased with the decreased total fungal biomass. In addition, the averaged bacterial biomass in the tubes that had EMF was not increased compared to that in the tubes with SAP only (Figure 4E). We thus concluded that the overall SAP biomass (saprotrophic fungi + bacteria) was suppressed by EMF. Nonetheless, future studies using modern molecular techniques (e.g., real-time quantitative polymerase chain reaction) are needed to directly quantify the degree of reduction in SAP with the presence of EMF.
As EMF and SAP are both common microorganisms in the soil, our results seem contrary to the accumulating evidence of increased productivity (“overyielding”) in species mixtures relative to monocultures, given that diversity promotes community productivity through niche partitioning among species (Tilman et al., 2001; Huang et al., 2018). Yet, the niche partitioning might be limited in our EMF-SAP interaction since the EMF niches could overlap intensively with those of SAP as they strongly compete for the same N resources. In addition to N competition, the ectomycorrhizal symbioses (roots + EMF) and SAP could also compete for water (Koide and Wu, 2003). In previous studies, excluding roots and EMF had yielded greater soil moisture since water uptake was eliminated (Moyano et al., 2007; Averill and Hawkes, 2016). In our study, soil moisture was higher in M tubes (excluding roots only) than in the surrounding undisturbed soils (Supplementary Figure 1), suggesting that the roots alone can drive potential suppression of SAP via water competition. Yet, we found higher soil moisture in M tubes (EMF + SAP) than in T tubes (SAP only, Table 1 and Supplementary Figure 1), suggesting that mycorrhizal hyphae alone may increase soil moisture (Moyano et al., 2007). The reason for higher soil moisture in M tubes than in T tubes is not fully clear, probably because of the hydraulic redistribution from plant to fungus under drought (Querejeta et al., 2003), which could be frequent in soil with low water retention (e.g., sandy soil in our system), or it is simply a side effect of tube design and installation. Since elevation of soil moisture can likely benefit SAP growth (Xu et al., 2020), the difference in SAP between paired M and T tubes might be an underestimation of the N-suppression by EMF.
We also hypothesized an alternative pathway that EMF could contribute to soil C accumulation via transporting photosynthetic C to microbial biomass (Figure 1). However, in contrast to our hypothesis, we found that fungal and bacterial biomass did not increase in the presence of EMF in our pine plantation (Figure 4). Therefore, although EMF provided plant-derived C to the soil, the incorporation of this labile C into fungal and bacteria biomass was limited. Instead, the presence of EMF hyphae increased the overall soil microbial respiration (Figures 4, 5), indicating that the plant-derived C allocated to EMF could have been largely consumed through respiration in our pine plantation. This result is in line with a recent finding in a mature Eucalyptus forest where the majority of increased photosynthetic carbon under elevated CO2 was consumed via microbial respiration rather than contributing to soil C accumulation (Jiang et al., 2020). A number of previous studies have also reported greater respiration rate in tubes with SAP + EMF (M tubes) than in tubes with SAP only (T tubes) (Heinemeyer et al., 2007; Nottingham et al., 2010; Hasselquist et al., 2012; Vallack et al., 2012; Zeng et al., 2018; Yan et al., 2019). Theoretically, the increased respiration could come from the ingrowth of EMF hyphae, and from the potential stimulation of SAP given that exudation and necromass of EMF could be used as a metabolic substrate for saprotrophs (i.e., EMF priming effect). However, the potential EMF-priming effect on SAP should be relatively small since we found suppression, rather than stimulation, of SAP biomass by EMF. Therefore, the increased respiration should be mainly from the ingrowth of EMF hyphae. We suggest future studies use advanced techniques such as DNA-SIP to better trace the carbon flow from hosts to EMF and other rhizosphere microorganisms (Hannula et al., 2020).
The impacts of EMF on microbial respiration were mostly positive along the N fertilization gradient (Figure 5D), while the impacts of EMF on microbial biomass were more negative with greater level of N fertilization (Figures 4E,F). Higher levels of N fertilization have resulted in greater concentration of mineral N in both forest litter layer and surface soil (Figure 3), but this higher N availability did not stimulate the growth of SAP. In addition, EMF-bacteria interaction responded to N fertilization in a similar manner as did the interaction between the EMF and saprotrophic fungi (Figures 4E,F), confirming that the role of bacteria should not be ignored in understanding and predicting impacts of N fertilization on EMF-SAP interactions. Previous studies also suggested that total microbial biomass continue to decrease with increasing N fertilization (Demoling et al., 2008; Treseder, 2008), and our results further suggested that the negative response of microbial biomass to inorganic N fertilization could be partly driven by EMF.
As EMF often became less dominant with increasing soil N (Morrison et al., 2016), it has been expected that suppression of SAP by EMF would be relieved under N deposition (Fernandez and Kennedy, 2016). We found the exact opposite pattern in our plantation. One of the possible explanations is that rather than the relative abundances of EMF and SAP, the abundances of key fungal taxa are the main drivers of EMF-SAP interactions (Fernandez et al., 2020; Lindahl et al., 2021). For instance, some EMF taxa often more strongly decrease in response to N fertilization, but these taxa are not necessarily strong N acquirers and competitors (Morrison et al., 2016). Thus, reduction in the relative abundance of EMF may not always translate into relief of the Gadgil effect. In addition, some key EMF taxa may even increase its relative abundance under N fertilization (e.g., Russula vinacea, Morrison et al., 2016), potentially enhancing its competition with SAP. Therefore, the overall response of EMF-SAP interactions to N fertilization may vary from site to site and depends on the local community structure (Morrison et al., 2016; Maaroufi et al., 2019; Fernandez et al., 2020; Moore et al., 2021). Also, the dose of N fertilization that caused significant reduction in SAP in our site (15 g N m–2 y–1) was relatively high compared to the dose found significant in other sites (e.g., 5 g N m–2 y–1 in Maaroufi et al., 2019). Yet, the tree density in our plantation (3,460 stem ha–1) was much higher than the global average in temperate coniferous (∼400 stem ha–1) and boreal (∼800 stem ha–1) forests (Crowther et al., 2015), probably leading to a substantial amount of the N inputs being sequestrated by the densely planted trees.
Since the suppression of SAP by EMF was stronger under higher level of N fertilization in our study, the soil C accumulation driven by the Gadgil effect would likely be amplified with accumulation of anthropogenic N deposition in this area, which is the largest plantation in China. Previous studies also showed that soil C accumulation was enhanced by N deposition in N-limited boreal and temperate forests (Frey et al., 2014; Zak et al., 2017; Maaroufi et al., 2019). A meta-analysis suggests that nitrogen deposition impedes organic matter decomposition, and thus stimulates carbon sequestration in forest soils (Janssens et al., 2010). We did not measure decomposition directly, but the reduced SAP biomass, or some keystone SAP taxa (Jin et al., 2022), could very likely lead to slowed decomposition and enhanced soil C accumulation. More specifically, our results suggested that N fertilization could enhance soil C accumulation in N-limited temperate conifer forests only where EMF were present.
By studying the EMF-SAP interactions in a northern conifer plantation, we found that the plant-derived C allocated to EMF could have been largely respired by soil microbes (mainly EMF) and contributed little to soil microbial biomass accumulation. We also found direct evidence of the Gadgil effect that EMF tend to reduce SAP biomass. This reduction of SAP by EMF was unlikely to be relieved when soil N availability was increased. Instead, suppression of SAP seemed to be even stronger under higher levels of N fertilization, suggesting that future C accumulation in China’s largest plantation would likely be amplified with accumulation of anthropogenic N deposition.
The original contributions presented in this study are included in the article/Supplementary material, further inquiries can be directed to the corresponding authors.
SL and WC conceived the idea and performed the research. SL, WW, and WC designed the study. SL, XZ, and RW analyzed the data. SL wrote the manuscript, with inputs from WW, XZ, RW, and WC. All authors contributed to the article and approved the submitted version.
This study was supported by the Projects of National Natural Science Foundation of China (Nos. 31972939, 32001163, and 32101293), the Zhejiang University K. P. Chao’s High Technology Development Foundation (No. 2020QN018), and the Zhejiang Provincial Funds for Distinguished Young Scientists (No. LR21C030002).
We thank Xinyue Zhang and Na Tao for their help in phospholipid fatty acid analysis.
The authors declare that the research was conducted in the absence of any commercial or financial relationships that could be construed as a potential conflict of interest.
All claims expressed in this article are solely those of the authors and do not necessarily represent those of their affiliated organizations, or those of the publisher, the editors and the reviewers. Any product that may be evaluated in this article, or claim that may be made by its manufacturer, is not guaranteed or endorsed by the publisher.
The Supplementary Material for this article can be found online at: https://www.frontiersin.org/articles/10.3389/fevo.2022.974449/full#supplementary-material
Adamczyk, B., Sietiö, O. M., Straková, P., Prommer, J., Wild, B., Hagner, M., et al. (2019). Plant roots increase both decomposition and stable organic matter formation in boreal forest soil. Nat. Commun. 10:3982. doi: 10.1038/s41467-019-11993-1
Averill, C., Dietze, M. C., and Bhatnagar, J. M. (2018). Continental-scale nitrogen pollution is shifting forest mycorrhizal associations and soil carbon stocks. Glob. Change Biol. 24, 4544–4553. doi: 10.1111/gcb.14368
Averill, C., and Hawkes, C. V. (2016). Ectomycorrhizal fungi slow soil carbon cycling. Ecol. Lett. 19, 937–947. doi: 10.1111/ele.12631
Averill, C., Turner, B. L., and Finzi, A. C. (2014). Mycorrhiza-mediated competition between plants and decomposers drives soil carbon storage. Nature 505, 543–545. doi: 10.1038/nature12901
Bossio, D. A., and Scow, K. M. (1998). Impacts of carbon and flooding on soil microbial communities: phospholipid fatty acid profiles and substrate utilization patterns. Microb. Ecol. 35, 265–278. doi: 10.1007/s002489900082
Carteron, A., Cichonski, F., and Laliberté, E. (2021). Ectomycorrhizal stands accelerate decomposition to a greater extent than arbuscular mycorrhizal stands in a northern deciduous forest. Ecosystems 1–15. doi: 10.1007/s10021-021-00712-x
Clemmensen, K. E., Bahr, A., Ovaskainen, O., Dahlberg, A., Ekblad, A., Wallander, H., et al. (2013). Roots and associated fungi drive long-term carbon sequestration in boreal forest. Science 339, 1615–1618. doi: 10.1126/science.1231923
Cleveland, C. C., and Liptzin, D. (2007). C: N: P stoichiometry in soil: is there a “Redfield ratio” for the microbial biomass? Biogeochemistry 85, 235–252. doi: 10.1007/s10533-007-9132-0
Crowther, T. W., Glick, H. B., Covey, K. R., Bettigole, C., Maynard, D. S., Thomas, S. M., et al. (2015). Mapping tree density at a global scale. Nature 525, 201–205. doi: 10.1038/nature14967
Demoling, F., Nilsson, L. O., and Bååth, E. (2008). Bacterial and fungal response to nitrogen fertilization in three coniferous forest soils. Soil Biol. Biochem. 40, 370–379. doi: 10.1016/j.soilbio.2007.08.019
Ekblad, A., Wallander, H., Godbold, D. L., Cruz, C., Johnson, D., Baldrian, P., et al. (2013). The production and turnover of extramatrical mycelium of ectomycorrhizal fungi in forest soils: role in carbon cycling. Plant Soil 366, 1–27. doi: 10.1007/s11104-013-1630-3
Fernandez, C. W., and Kennedy, P. G. (2016). Revisiting the ‘Gadgil effect’: do interguild fungal interactions control carbon cycling in forest soils? New Phytol. 209, 1382–1394. doi: 10.1111/nph.13648
Fernandez, C. W., See, C. R., and Kennedy, P. G. (2020). Decelerated carbon cycling by ectomycorrhizal fungi is controlled by substrate quality and community composition. New Phytol. 226, 569–582. doi: 10.1111/nph.16269
Frey, S. D., Ollinger, S., Nadelhoffer, K. E., Bowden, R., Brzostek, E., Burton, A., et al. (2014). Chronic nitrogen additions suppress decomposition and sequester soil carbon in temperate forests. Biogeochemistry 121, 305–316. doi: 10.1007/s10533-014-0004-0
Gadgil, R. L., and Gadgil, P. D. (1971). Mycorrhiza and litter decomposition. Nature 233, 133–133. doi: 10.1038/233133a0
Hannula, S. E., Morrien, E., van der Putten, W. H., and de Boer, W. (2020). Rhizosphere fungi actively assimilating plant-derived carbon in a grassland soil. Fung. Ecol. 48:100988. doi: 10.1016/j.funeco.2020.100988
Hasselquist, N. J., Metcalfe, D. B., and Högberg, P. (2012). Contrasting effects of low and high nitrogen additions on soil CO2 flux components and ectomycorrhizal fungal sporocarp production in a boreal forest. Glob. Change Biol. 18, 3596–3605. doi: 10.1111/gcb.12001
Heinemeyer, A., Hartley, I. P., Evans, S. P., Carreira, de La Fuente, J. A., and Ineson, P. (2007). Forest soil CO2 flux: uncovering the contribution and environmental responses of ectomycorrhizas. Glob. Change Biol. 13, 1786–1797. doi: 10.1111/j.1365-2486.2007.01383.x
Hobbie, E. A. (2006). Carbon allocation to ectomycorrhizal fungi correlates with belowground allocation in culture studies. Ecology 87, 563–569. doi: 10.1890/05-0755
Högberg, M. N., and Högberg, P. (2002). Extramatrical ectomycorrhizal mycelium contributes one-third of microbial biomass and produces, together with associated roots, half the dissolved organic carbon in a forest soil. New Phytol. 154, 791–795. doi: 10.1046/j.1469-8137.2002.00417.x
Högberg, P., Nordgren, A., Buchmann, N., Taylor, A. F. S., Ekblad, A., Högberg, M. N., et al. (2001). Large-scale forest girdling shows that current photosynthesis drives soil respiration. Nature 411, 789–792. doi: 10.1038/35081058
Huang, Y., Chen, Y., Castro-Izaguirre, N., Baruffol, M., Brezzi, M., Lang, A., et al. (2018). Impacts of species richness on productivity in a large-scale subtropical forest experiment. Science 362, 80–83. doi: 10.1126/science.aat6405
Janssens, I. A., Dieleman, W., Luyssaert, S., Subke, J. A., Reichstein, M., Ceulemans, R., et al. (2010). Reduction of forest soil respiration in response to nitrogen deposition. Nat. Geosci. 3, 315–322. doi: 10.1038/ngeo844
Jiang, M., Medlyn, B. E., Drake, J. E., Duursma, R. A., Anderson, I. C., Barton, C. V., et al. (2020). The fate of carbon in a mature forest under carbon dioxide enrichment. Nature 580, 227–231. doi: 10.1038/s41586-020-2128-9
Jin, X., Wang, Z., Wu, F., Li, X., and Zhou, X. (2022). Litter mixing alters microbial decomposer community to accelerate tomato root litter decomposition. Microbiol. Spect. 8, 186–122.e. doi: 10.1128/spectrum.00186-22
Kaye, J. P., and Hart, S. C. (1997). Competition for nitrogen between plants and soil microorganisms. Trends Ecol. Evolut. 12, 139–143. doi: 10.1016/S0169-5347(97)01001-X
Kjøller, R., Nilsson, L. O., Hansen, K., Schmidt, I. K., Vesterdal, L., and Gundersen, P. (2012). Dramatic changes in ectomycorrhizal community composition, root tip abundance and mycelial production along a stand-scale nitrogen deposition gradient. New Phytol. 194, 278–286. doi: 10.1111/j.1469-8137.2011.04041.x
Koide, R. T., and Wu, T. (2003). Ectomycorrhizas and retarded decomposition in a Pinus resinosa plantation. New Phytol. 158, 401–407. doi: 10.1046/j.1469-8137.2003.00732.x
Kuzyakov, Y. (2002). Factors affecting rhizosphere priming effects. J. Plant Nutr. Soil Sci. 165, 382–396. doi: 10.1002/1522-2624(200208)165:4<382::AID-JPLN382>3.0.CO;2-#
Liang, C., Amelung, W., Lehmann, J., and Kästner, M. (2019). Quantitative assessment of microbial necromass contribution to soil organic matter. Glob. Change Biol. 25, 3578–3590. doi: 10.1111/gcb.14781
Lindahl, B. D., Kyaschenko, J., Varenius, K., Clemmensen, K. E., Dahlberg, A., Karltun, E., et al. (2021). A group of ectomycorrhizal fungi restricts organic matter accumulation in boreal forest. Ecol. Lett. 24, 1341–1351. doi: 10.1111/ele.13746
Maaroufi, N. I., Nordin, A., Palmqvist, K., Hasselquist, N. J., Forsmark, B., Rosenstock, N. P., et al. (2019). Anthropogenic nitrogen enrichment enhances soil carbon accumulation by impacting saprotrophs rather than ectomycorrhizal fungal activity. Glob. Change Biol. 25, 2900–2914. doi: 10.1111/gcb.14722
McCormack, M. L., Dickie, I. A., Eissenstat, D. M., Fahey, T. J., Fernandez, C. W., Guo, D., et al. (2015). Redefining fine roots improves understanding of below-ground contributions to terrestrial biosphere processes. New Phytol. 207, 505–518. doi: 10.1111/nph.13363
Moore, J. A., Anthony, M. A., Pec, G. J., Trocha, L. K., Trzebny, A., Geyer, K. M., et al. (2021). Fungal community structure and function shifts with atmospheric nitrogen deposition. Glob. Change Biol. 27, 1349–1364. doi: 10.1111/gcb.15444
Morrison, E. W., Frey, S. D., Sadowsky, J. J., van Diepen, L. T., Thomas, W. K., and Pringle, A. (2016). Chronic nitrogen additions fundamentally restructure the soil fungal community in a temperate forest. Fung. Ecol. 23, 48–57. doi: 10.1016/j.funeco.2016.05.011
Moyano, F. E., Kutsch, W. L., and Schulze. (2007). Response of mycorrhizal, rhizosphere and soil basal respiration to temperature and photosynthesis in a barley field. Soil Biol. Biochem. 39, 843–853. doi: 10.1016/j.soilbio.2006.10.001
Nottingham, A. T., Turner, B. L., Winter, K., van der Heijden, M. G., and Tanner, E. V. (2010). Arbuscular mycorrhizal mycelial respiration in a moist tropical forest. New Phytol. 186, 957–967. doi: 10.1111/j.1469-8137.2010.03226.x
Querejeta, J., Egerton-Warburton, L. M., and Allen, M. F. (2003). Direct nocturnal water transfer from oaks to their mycorrhizal symbionts during severe soil drying. Oecologia 134, 55–64. doi: 10.1007/s00442-002-1078-2
R Core Team (2021). R: A Language and Environment for Statistical Computing. Vienna: R Foundation for Statistical Computing.
Sterkenburg, E., Clemmensen, K. E., Ekblad, A., Finlay, R. D., and Lindahl, B. D. (2018). Contrasting effects of ectomycorrhizal fungi on early and late stage decomposition in a boreal forest. ISME J. 12, 2187–2197. doi: 10.1038/s41396-018-0181-2
Tagesson, T., Schurgers, G., Horion, S., Ciais, P., Tian, F., and Brandt, M. (2020). Recent divergence in the contributions of tropical and boreal forests to the terrestrial carbon sink. Nat. Ecol. Evolut. 4, 202–209. doi: 10.1038/s41559-019-1090-0
Tilman, D., Reich, P. B., Knops, J., Wedin, D., Mielke, T., and Lehman, C. (2001). Diversity and productivity in a long-term grassland experiment. Science 294, 843–845. doi: 10.1126/science.1060391
Treseder, K. K. (2008). Nitrogen additions and microbial biomass: a meta-analysis of ecosystem studies. Ecol. Lett. 11, 1111–1120. doi: 10.1111/j.1461-0248.2008.01230.x
Vallack, H. W., Leronni, V., Metcalfe, D. B., Högberg, P., Ineson, P., and Subke, J. A. (2012). Application of nitrogen fertilizer to a boreal pine forest has a negative impact on the respiration of ectomycorrhizal hyphae. Plant Soil 352, 405–417. doi: 10.1007/s11104-011-1005-6
Xu, S., Geng, W., Sayer, E. J., Zhou, G., Zhou, P., and Liu, C. (2020). Soil microbial biomass and community responses to experimental precipitation change: A meta-analysis. Soil Ecol. Lett. 2, 93–103. doi: 10.1007/s42832-020-0033-7
Yan, T., Qu, T., Song, H., Sun, Z., Zeng, H., and Peng, S. (2019). Ectomycorrhizal fungi respiration quantification and drivers in three differently-aged larch plantations. Agricult. Forest Meteorol. 265, 245–251. doi: 10.1016/j.agrformet.2018.11.024
Zak, D. R., Freedman, Z. B., Upchurch, R. A., Steffens, M., and Kögel-Knabner, I. (2017). Anthropogenic N deposition increases soil organic matter accumulation without altering its biochemical composition. Glob. Change Biol. 23, 933–944. doi: 10.1111/gcb.13480
Zak, D. R., Pellitier, P. T., Argiroff, W., Castillo, B., James, T. Y., Nave, L. E., et al. (2019). Exploring the role of ectomycorrhizal fungi in soil carbon dynamics. New Phytol. 223, 33–39. doi: 10.1111/nph.15679
Keywords: Gadgil effect, microbial respiration, nitrogen competition, nitrogen deposition, phospholipid fatty acid analysis, Scots pine
Citation: Liang S, Wang W, Zeng X, Wu R and Chen W (2022) Enhanced suppression of saprotrophs by ectomycorrhizal fungi under high level of nitrogen fertilization. Front. Ecol. Evol. 10:974449. doi: 10.3389/fevo.2022.974449
Received: 21 June 2022; Accepted: 05 August 2022;
Published: 22 August 2022.
Edited by:
Giovanna Battipaglia, University of Campania Luigi Vanvitelli, ItalyReviewed by:
César Marín, Santo Tomás University, ChileCopyright © 2022 Liang, Wang, Zeng, Wu and Chen. This is an open-access article distributed under the terms of the Creative Commons Attribution License (CC BY). The use, distribution or reproduction in other forums is permitted, provided the original author(s) and the copyright owner(s) are credited and that the original publication in this journal is cited, in accordance with accepted academic practice. No use, distribution or reproduction is permitted which does not comply with these terms.
*Correspondence: Wei Wang, d2FuZ3dAdXJiYW4ucGt1LmVkdS5jbg==; Weile Chen, Y2hlbndlaWxlQHpqdS5lZHUuY24=
Disclaimer: All claims expressed in this article are solely those of the authors and do not necessarily represent those of their affiliated organizations, or those of the publisher, the editors and the reviewers. Any product that may be evaluated in this article or claim that may be made by its manufacturer is not guaranteed or endorsed by the publisher.
Research integrity at Frontiers
Learn more about the work of our research integrity team to safeguard the quality of each article we publish.