- 1Department of Fisheries, Wildlife, and Conservation Sciences, Oregon State University, Corvallis, OR, United States
- 2Department of Biological Sciences, Florida Atlantic University, Boca Raton, FL, United States
Because the sex of sea turtles is determined by temperature during embryonic development, many populations are vulnerable to increased bias in primary sex ratios as global temperatures rise. Higher temperatures produce more females, and some populations are already showing years with all-female offspring production. But because sea turtles take decades to mature and have long adult lifespans, these primary sex ratio biases can take years to impact adult sex ratios, and the males from cohorts that are produced during cooler years may compensate for the sex ratio bias if they can breed more frequently and with multiple females. To date, little is known about male sea turtle reproductive behavior, making predictions of sex ratio skew impacts highly speculative. We used data from southern Florida loggerhead sea turtle nests to parameterize a simple population model to explore the effects of an increase in the proportion of female hatchlings over time on population trends, effective population size (Ne), and quasi-extinction probability. We also tested the effects of increasing the frequency of relatively high male production years to simulate potential mitigation strategies. While heuristic rather than predictive, our results expectedly show a rise in nest counts due to the increase in females over time, followed by population decline as males become limiting. Population collapse due to increased female bias will take many decades to occur, but sex ratio skew can have large impacts on Ne, and thus increase the potential for inbreeding. An increase in the frequency of male production years, even just one additional “good male year” per decade, can help mitigate these outcomes if the rate of feminization is not too rapid. Male breeding frequency and mating success are critical drivers of the results and must be prioritized for research.
Introduction
For many reptiles, temperature dependent sex determination (TSD), a form of environmental sex determination (ESD), directs the primary sex ratio of hatchlings upon which all subsequent life stages’ sex ratios depend. Increasing numbers of studies speculate that current and impending environmental impacts from changing climates will be amplified by sex ratio bias (Janzen, 1994; Mitchell et al., 2010; Wyneken and Lolavar, 2015; Tanner et al., 2019), but for long-lived, late-maturing species such as marine turtles, measuring the impact of increasingly skewed sex ratios likely lasts beyond the life span of most scientists and conservation managers. Approaches currently used to understand and synthesize effects range from relatively short term to multigenerational studies, where feasible. Increasingly, studies that use systems approaches can focus greater importance of taking broad views of conservation priorities in the Anthropocene (e.g., Young et al., 2016; Woodhead et al., 2019).
A fundamental challenge for assessing species with ESD is whether incubation conditions produce sex ratios so skewed that they threaten population persistence. Primary sex ratios are environmentally dependent in at least three ways. One is by the cascade of developmental signals, initiated by incubation temperatures, that direct the embryo to be male or female. Another is through the viability of embryos when incubation temperatures range into thermal maxima or minima. Finally, extreme weather events that are clustered in one part of an incubation season or another can lead to loss of parts of the cohort such that the cohort’s sex ratio may be less extremely biased or more biased (e.g., Laloë et al., 2021). The sex ratio of an individual annual cohort affected by extreme weather events is inconsequential if the highly skewed primary sex ratio is rare. This is because many cohorts contribute to the juvenile and adult life history stages, particularly in long-lived species. However, as the frequency of highly skewed primary sex ratios increases, as inferred (Hawkes et al., 2013; Monsinjon et al., 2019) and predicted (Fuentes et al., 2010; Laloë et al., 2016), the accumulation of affected cohorts could lead to one sex becoming limiting for population productivity (Janzen, 1994; Mitchell and Janzen, 2010; Boyle et al., 2014). This is most likely to occur if the reproductive physiology or behavior of a species are phylogenetically restricted or too slow to compensate for the shift in adult sex ratio, or if populations are unable to shift their distributions due to range restriction. Long term effects of sex ratio bias can be explored through simulation exercises. For example, the fate of endangered tuatara (Sphenodon punctatus) is strongly affected by that species’ response to increasing temperatures. As this species range is restricted to remote islands, TSD adaptation or active mitigation may be required for species persistence (Mitchell et al., 2010).
Marine turtles are charismatic species with a TSD system that is relatively understood and subject to climate change; hotter nests produce more females and cooler nests produce more males (Type 1A reaction norm, Warner and Shine, 2011). A changing climate will affect beach temperatures, precipitation, storm frequency, and sea level, all of which can drive primary sex ratios and early survival in sea turtles (Hawkes et al., 2009; Kobayashi et al., 2017; Monsinjon et al., 2019; Laloë et al., 2021). There is a fundamental concern that warming temperatures will result in insufficient numbers of males and that will eventually impact population productivity and persistence. While female-biased sex ratios are not uncommon in sea turtles, there is increasing documentation of both gradual and extreme feminization in some populations, including an increase in 100% female nests (e.g., Tanner et al., 2019). When extreme temperatures occur or are coupled with lower precipitation, egg and hatchling mortality can increase (Wyneken and Salmon, 2020).
The consequences of skewed primary sex ratios at the population-level can be complex and depend on reproductive behavior and physiology of adults, as well as the age at sexual maturity (ASM), reproductive longevity of males vs. females (Maurer et al., 2021) and methods of assessment (e.g., Lasala et al., 2013; Patrício et al., 2019). While breeding frequency, nest number, and clutch size are commonly measured parameters for adult female sea turtles, adult male sea turtle behavior is far more difficult to study because they do not return to shore. If we assume that survivorship to adulthood, age at maturation and lifespan are comparable for the two sexes, the point at which males become limiting to population productivity depends on breeding frequency relative to females (for example, if males breed annually but females only breed every 2–4 years) and the mean and maximum number of females that a male can mate with in a given breeding season (Wright et al., 2012a; Hays et al., 2014). Female sea turtles store sperm to fertilize multiple clutches of eggs, but little is known about that process. Paternity studies in sea turtles indicate that nests are typically fathered by multiple males (Lee et al., 2018), but relatively few studies have attempted to estimate the realized breeding adult sex ratio (fathers:mothers) for a nesting beach (e.g., Stewart and Dutton, 2011; Lasala et al., 2013; Sari et al., 2017, see review by Komoroske et al., 2017). The physiological limitations of multiple matings are also unknown, but the energetic costs of male competition and mating are thought to be quite high (Jessop et al., 2004). While females invest substantial energy in the production of lecithotrophic eggs, male energetic investment in reproduction likely comes through competition and migration; i.e., it is a behavioral cost instead of a physiological one. This behavioral cost likely comes with an increase predation-mortality risk as well.
Recently, the impacts of increased sex ratio skew in sea turtle populations have been explored with theoretical models (Hays et al., 2010; Monsinjon et al., 2019; Wei et al., 2021) and simulation approaches (Hays et al., 2017; Jensen et al., 2022). The long generation times of sea turtles (e.g., 30 + years to sexual maturation in green turtles) mean that impacts of highly skewed (90% + female) primary sex ratios may initially lead to production increases but inevitably lead to insufficient males to maintain the populations. The effects of sex ratio skew in the models strongly depends on successful matings per male and breeding frequency, and the long generation time increases the time to population collapse (see also Wright et al., 2012a,b). Even if there is little chance of adaptation because the current changes in climate are happening so quickly relative to sea turtle generation time, the observed effects of strong sex ratio skew may be many decades in the future. However, researchers have also postulated that strongly biased sex ratios in TSD reptiles are likely to increase the potential for inbreeding depression due to loss of genetic diversity and effective population size, Ne, particularly if male reproductive success is strongly skewed (Janzen, 1994; Theissinger et al., 2009; Mitchell et al., 2010; Wright et al., 2012a; Boyle et al., 2014; Blechschmidt et al., 2020).
Simulation models also can show the potential relative effects of different mitigation strategies that increase male hatchling production. A number of model results can be examined, including population trends and the probability of extinction or quasi-extinction that is often the goal of population viability analysis (PVA) (Burgman et al., 1993). The number of turtles at some future point in time in the simulated population is highly variable and strongly dependent on model assumptions and initial conditions, but relative changes in Ne may be more informative when examining the specific questions of sex ratio impacts on populations. Effective population size of the breeding adults can be much smaller than total population size when the adult sex ratio is highly skewed, indicating potential for inbreeding or other deleterious impacts on a population (Frankham, 1995).
While uncertainties persist in our understanding of the mechanisms that contribute to population productivity in response to increased feminization of sea turtles, there is increasing pressure to actively mitigate temperature increases on nesting beaches either by directly manipulating sex ratios (e.g., translocating nests) or indirectly by reducing nest temperatures with shading and watering (Fuentes et al., 2012; Jourdan and Fuentes, 2015; Blechschmidt et al., 2020; Lolavar and Wyneken, 2021; Patrício et al., 2021). Lowering the frequency of all female cohorts, increasing the frequency of strong male production years, or reducing the trend in mean sex ratio over time all have potential to delay a sex-ratio-mediated population decline. Yet, the magnitude of the effects of mitigation are uncertain. The relative benefits of such activities can be evaluated with population models that simulate the effects of variable primary sex ratios through time. Conservation strategy evaluation with population models has been used for sea turtles since the 1980s (e.g., reviewed by Heppell et al., 2003; Patrício et al., 2019, 2021; Maurer et al., 2021) and can help managers identify actions that are more or less likely to contribute to population persistence (Heppell and Crowder, 1998; Rout et al., 2013).
Within the continental United States three sea turtle species (Loggerhead, Caretta caretta; Green turtle, Chelonia mydas, and Leatherback, Dermochelys coriacea) nest on the temperate to subtropical beaches of the Northwest Atlantic and Gulf of Mexico. The loggerhead nests most abundantly and particularly so along the Florida peninsula. Genetically, the peninsular Florida loggerheads form a subpopulation that is the largest nesting aggregation of this species in the world, with an estimated ∼51,000 females nesting each year (Ceriani et al., 2019). The loggerhead is listed as globally imperiled with different management units identified as threated or worse (Casale and Tucker, 2017; Ceriani and Meylan, 2017). This subpopulation contributes 80–85% of the hatchling loggerheads entering the ocean each year (National Marine Fisheries Service and US Fish and Wildlife Service [NMFS and USFWS], 2008). Consequently, loggerhead population trends in the Northwestern Atlantic are driven by this subpopulation, which is therefore essential for evaluating population trends and recovery.
To explore the potential population-level effects of warming beach temperatures on the peninsular Florida loggerhead population under different assumptions about adult male behavior and reproductive success, we incorporated long-term primary sex ratio observations from nesting beach studies in southern Florida into a simple, age-structured simulation model with a variety of outputs, including nest and nesting female abundance, adult sex ratio, and effective population size (Ne) of adults and breeders. The last is an important response variable because highly female-skewed populations could continue to function while suffering inbreeding depression (Maurer et al., 2021). We then evaluated the benefits of increasing the frequency of strong male production years under various model assumptions to simulate mitigation strategies. This is a heuristic exercise to compare the relative benefits of different conservation strategies, not a predictive model. Nevertheless, it reveals important patterns of abundance for management consideration.
Materials and methods
Nests were sampled each year from the same beaches from Boca Raton (26.383°N, 80.1289°W), to Juno Beach (26.8798°N, 80.0534°W). Hatchling sex was identified by laparoscopic examination (see Wyneken et al., 2007).
To explore the effects of critical uncertainties and potential mitigation strategies on a loggerhead population affected by extreme sex ratio skew (female bias), we constructed a simple age-structured, two-sex, annual time step model representing loggerhead life history, using the parameters shown in Table 1. Our parameters of interest for this paper were hatchling sex ratio bias and male reproductive strategies, so we held many of the other basic life history parameters constant. ASM was fixed at 20 years to provide a time lag between hatchling birthdates and adult production, and juvenile and adult annual survival rates were fixed at 0.76 and 0.84, respectively. Our time lag of 20 years reflects the low end of estimates for this species; later ASMs could be used with a higher average juvenile survival rate, which would result in longer time lags in population response (Heppell et al., 2002, 2003). Female breeding frequency (remigration interval), nests per female, and hatchlings per nest were also held constant, with values shown in Table 1. Very few, if any, loggerhead females nest annually. Most nesting in the peninsular Florida loggerhead subpopulation varies such that a proportion of females return to nest in 2, 3, or 4-year remigration intervals with males returning with 1, 2, and 3-year remigration intervals (National Marine Fisheries Service and US Fish and Wildlife Service [NMFS and USFWS], 2008; Turtle Expert Working Group [TEWG], 2009).
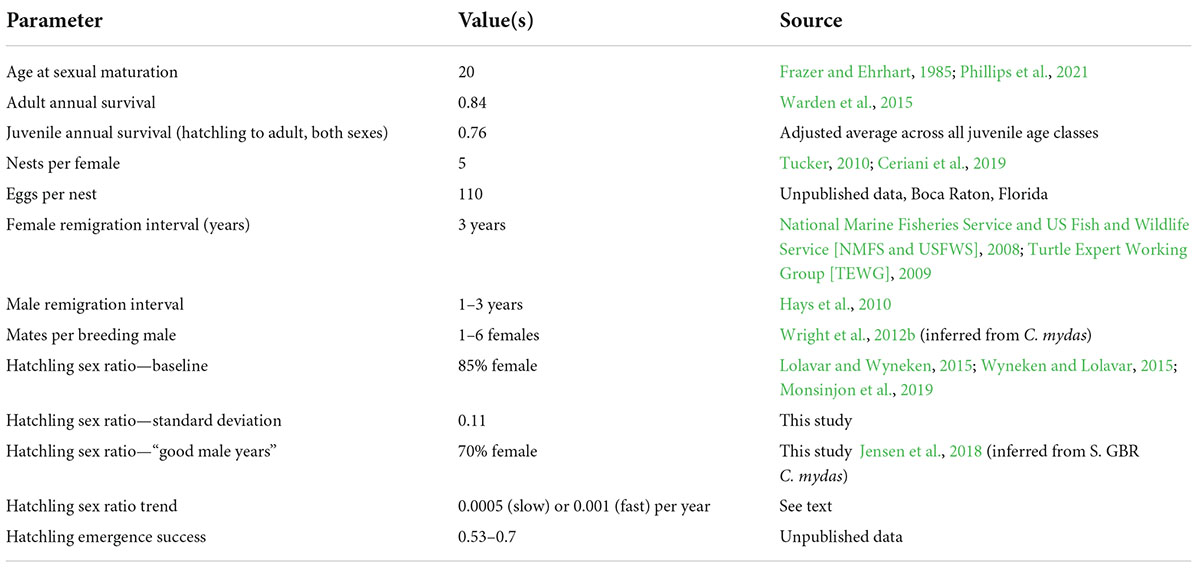
Table 1. Parameters used in the age-structured loggerhead model developed to evaluate sex ratio skew scenarios and potential mitigation.
To estimate primary sex ratio and nest survival, we evaluated 15 seasons (2002–2004, 2009–2020) of loggerhead sex ratio nest data from Boca Raton, Florida (Wyneken et al., 2007; Wyneken and Lolavar, 2015). Naturally deposited nests (n = 156) were located across the early, middle, and late parts of the loggerhead nesting season. Sampling was spread across the season such that nests from early to middle to late in the season were sampled in thirds. Observed primary sex ratios were based upon samples of 10 hatchlings/clutch from the primary hatchling emergence (if fewer hatchlings emerged, they represented the sample). In 2004, we collected 25% of the hatchlings from 9 clutches to accommodate both the long-term sex ratio study and a complementary single year sex ratio assessment. To measure how each year’s nest conditions related to incubation success and weather, nest temperatures were monitored within each clutch. Clutches were instrumented with Hobo U22-001 temperature dataloggers (Onset Computer Corporation, Bourne, Massachusetts United States) placed in the center of the clutch during oviposition or on the morning after egg deposition, within 12 h of egg deposition (per Florida Fish and Wildlife Conservation Commission [Florida FWCC], 2016) and left undisturbed to incubate and hatch. Hatchlings were collected and brought to the Florida Atlantic University Marine Lab where they were raised for several months and laparoscopically sexed. Following the last major emergence, nests were inventoried to quantify clutch size and success to provide estimates of hatchling production each season.
We examined the effects of male breeding frequency (remigration intervals) and number of matings in a breeding year on the model’s deterministic population growth rate (proportion per year change, lambda), the proportion of females unable to breed, and effective population size of the breeding adults, calculated as:
Where Mbreed is the number of males breeding in a year and Fbreed is the number of females breeding in a year, determined by their respective remigration intervals.
We incorporated the standard deviation of mean sex ratio observed into a series of simulations to explore the effects of increasing trends in the proportion of females and frequency of all female cohorts, as well as mitigation strategies that increase the frequency of years with 30% male hatchling production, which we called “good male years.” Trends in the change of primary sex ratio, proportion female (starting from the baseline of 0.85 female) were “fast” (+ 0.001 per year) or “slow” (+ 0.0005 per year). Frequency of good male years was modeled as a Monte Carlo random draw of a primary sex ratio of 0.7 females, 0.3 males with variable probability per decade of “good male years,” e.g., 1 good male year per decade (probability = 0.1), 2 good male years per decade (probability = 0.2), etc. All reported results are for 5,000 simulations of 320 years, with a starting population of 5,000 adult females and a stable age distribution from the baseline deterministic model with primary sex ratio = 0.85 female. Population trends in percent per year were calculated from mean slopes of exponential trendlines fit to nest counts from each simulation in year 100 or 300, and effective population size was based on a 20 year mean from each simulation at year 100 or 300. The quasi-extinction threshold (QET) was set as a 90% reduction in the initial number of nests, and extinction risk was reported as the probability of crossing that QET by year 300.
Results
The verified hatchling sexes for nine of the 15 years were represented by > 90% female sex ratios (Figure 1). The standard deviation for the data is 0.11 and was used in the simulation model as our measure of year-to-year variability in primary sex ratio. The trend line provides an estimate of the increase in proportion female over time that was used in the simulations: + 0.001 per year. We used this value as our “fast” increase in proportion female and halved it to + 0.0005 per year for simulations with a “slow” increase in proportion female.
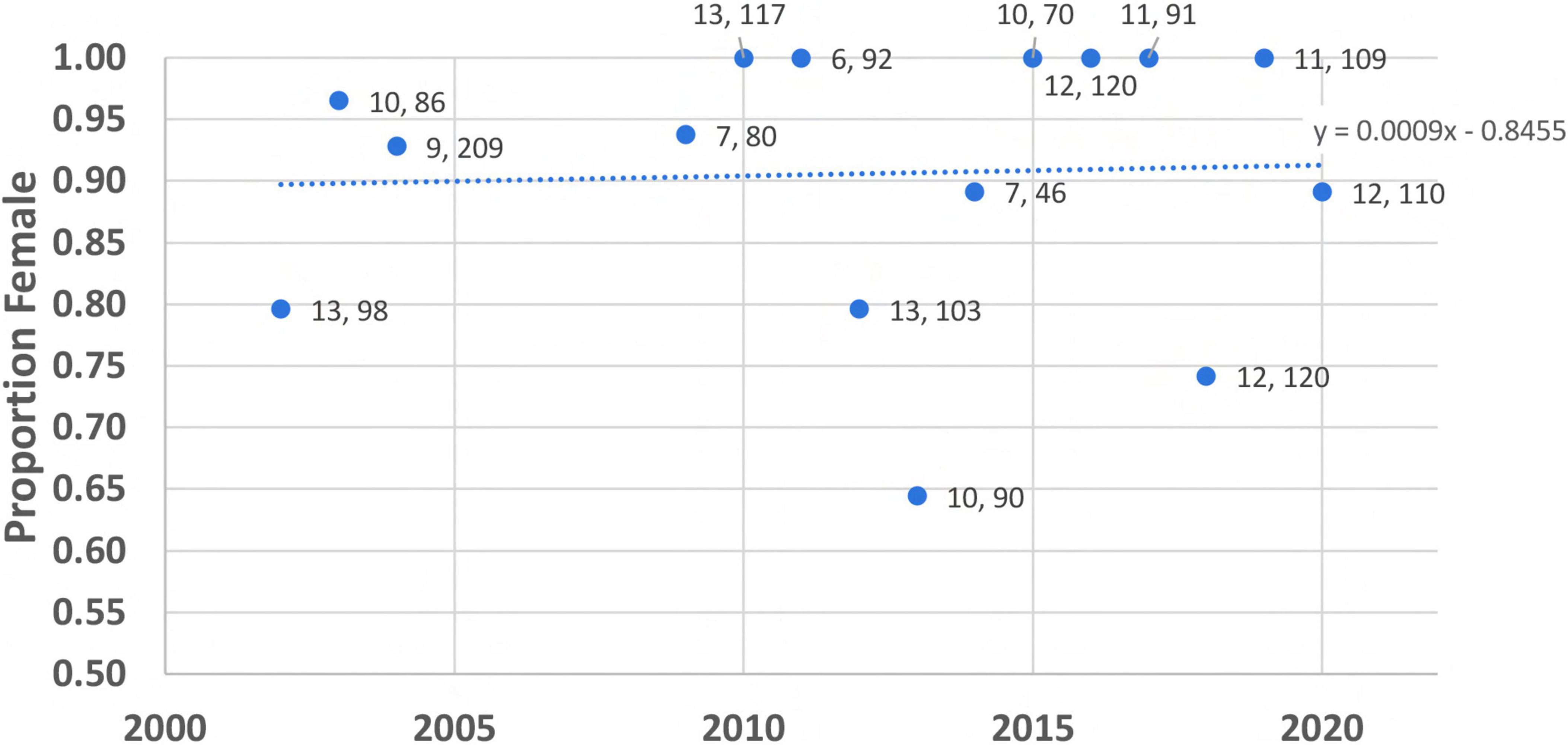
Figure 1. Observations of primary sex ratio, as proportion female, from loggerhead sea turtle nests sampled near Boca Raton, Florida. For each sex ratio observation, the number of nests and total number of hatchlings in the sample is provided (nests, hatchlings). Data were not collected in 2005 and 2006; data from 2007 to 2008 were excluded due to small sample sizes. Note that very few years approached “good male year” criteria.
While the relationship between hatchling survival and nest sex ratio given here is exploratory (Table 2), a sensitivity analysis indicated that this difference in survival had minor or undetectable effects on the model outputs, largely due to the low impact of egg survival rates on population growth rates (Heppell et al., 2002).
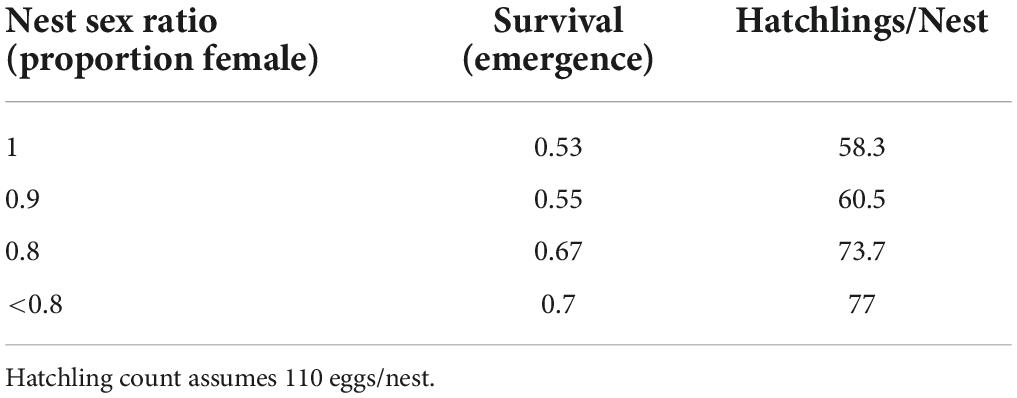
Table 2. Estimated survival and hatchling production per nest for different observed nest sex ratios for Boca Raton, Florida.
Effects of male reproductive strategy
With hatchling sex ratio set at a constant 0.85 (85% female), the deterministic model resulted in a maximum annual population rate of increase of 1.014 or 1.4% per year when males were not limiting (Figure 2A). Under conditions of reduced breeding frequency (prolonged remigration interval) or reduced matings per male, the deterministic population growth rate decreased because some proportion of females capable of breeding each year could not find a mate. Effective population size (Ne, breeding adults) declines over time if males become limiting in a simulation, so we show the Ne for simulation year 100 (Figure 2B). The largest differences in Ne at year 100 are driven by male remigration interval. If males are not limiting, as occurs in our simulations when males breed annually and can mate with at least 2 females, Ne is the same at year 100 regardless of matings per male because all females get to breed. However, when males remigrate less often, the effective population size of breeders is reduced to a fraction of that size, with a much lower maximum Ne that requires at least 4 matings per male (if males remigrate every 2 years) or 6 matings per male (if males remigrate every 3 years, the same as females in our models). Another way to look at these deterministic model outcomes is to consider the availability of males for females who have come to nest in a given year (Figure 2C). If males are less available to females because their remigration rate is longer or they can only mate with a few females, some females are unable to find mates and their reproductive potential is lost.
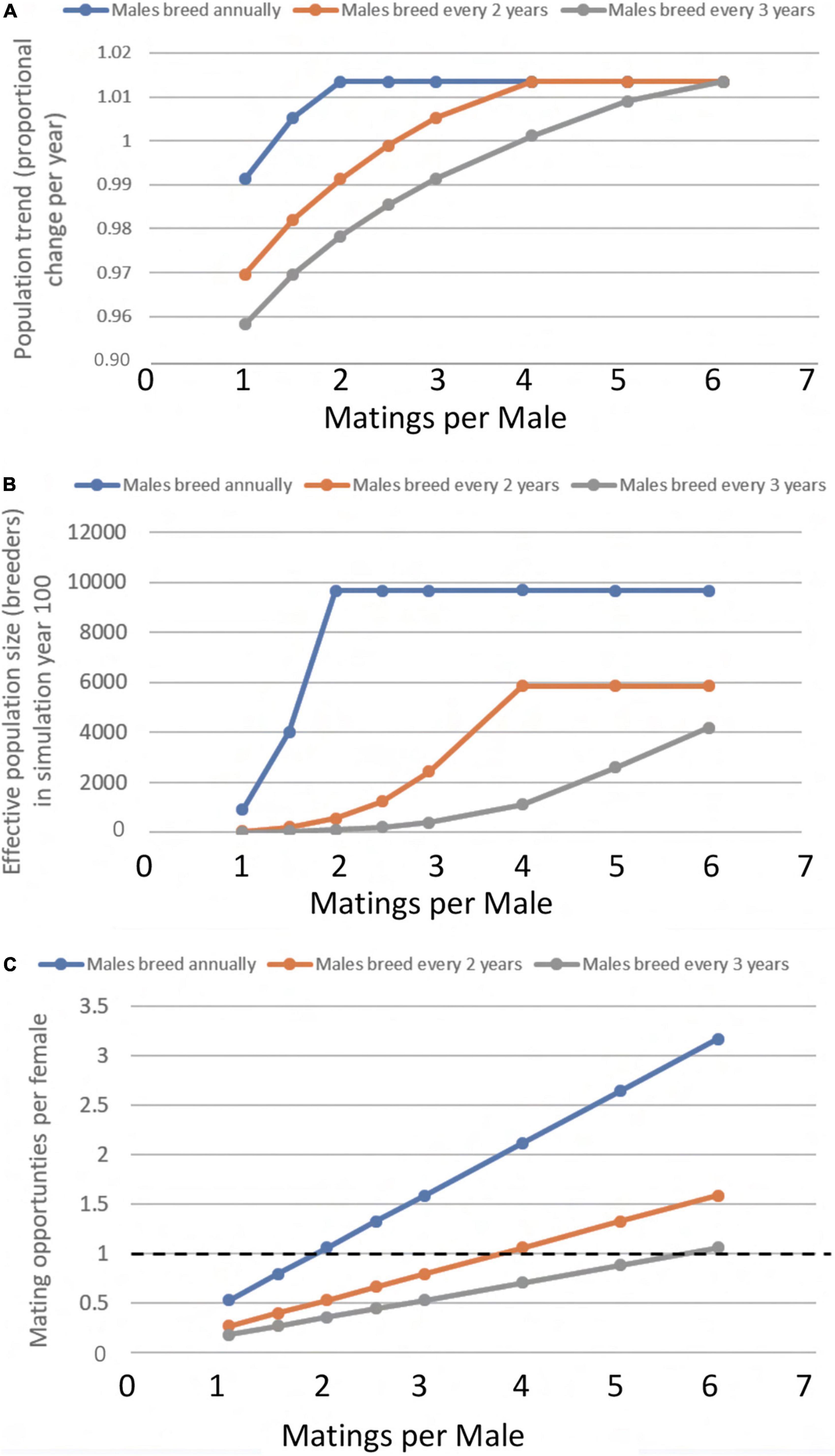
Figure 2. Deterministic age-structured model results showing effects of male remigration interval and number of matings per male on (A) Population trend at year 100 (asymptotic). (B) Effective population size Ne of the breeding adults (based on Eqn. 1 and remigration intervals of males and females). (C) Potential mates per female, based on the expected breeder sex ratio; when mating opportunities per female are less than 1.0, some females in the model do not produce nests. Primary sex ratio set at a constant 85% females, female remigration interval fixed at 3 years, other parameters shown in Table 1. Note that frequency of breeding and the number of females that a male can mate with have a large effect on predicted population stability and Ne, and provide a predictable trade-off; if breeding frequency is high, fewer matings per male are needed to achieve a positive population growth rate, and vice versa. Blue: Males breed annually; Orange: Males breed every 2 years; Gray: Males breed very 3 years.
The effects of male breeding strategy on population outputs depend on the intensity of the sex ratio skew, so another question to explore is what should be the minimum number of matings per male to achieve population stability (lambda = 1.0) for a given primary sex ratio (Figure 3). If male remigration interval is shorter than females, the simulated populations can persist with lower matings per male.
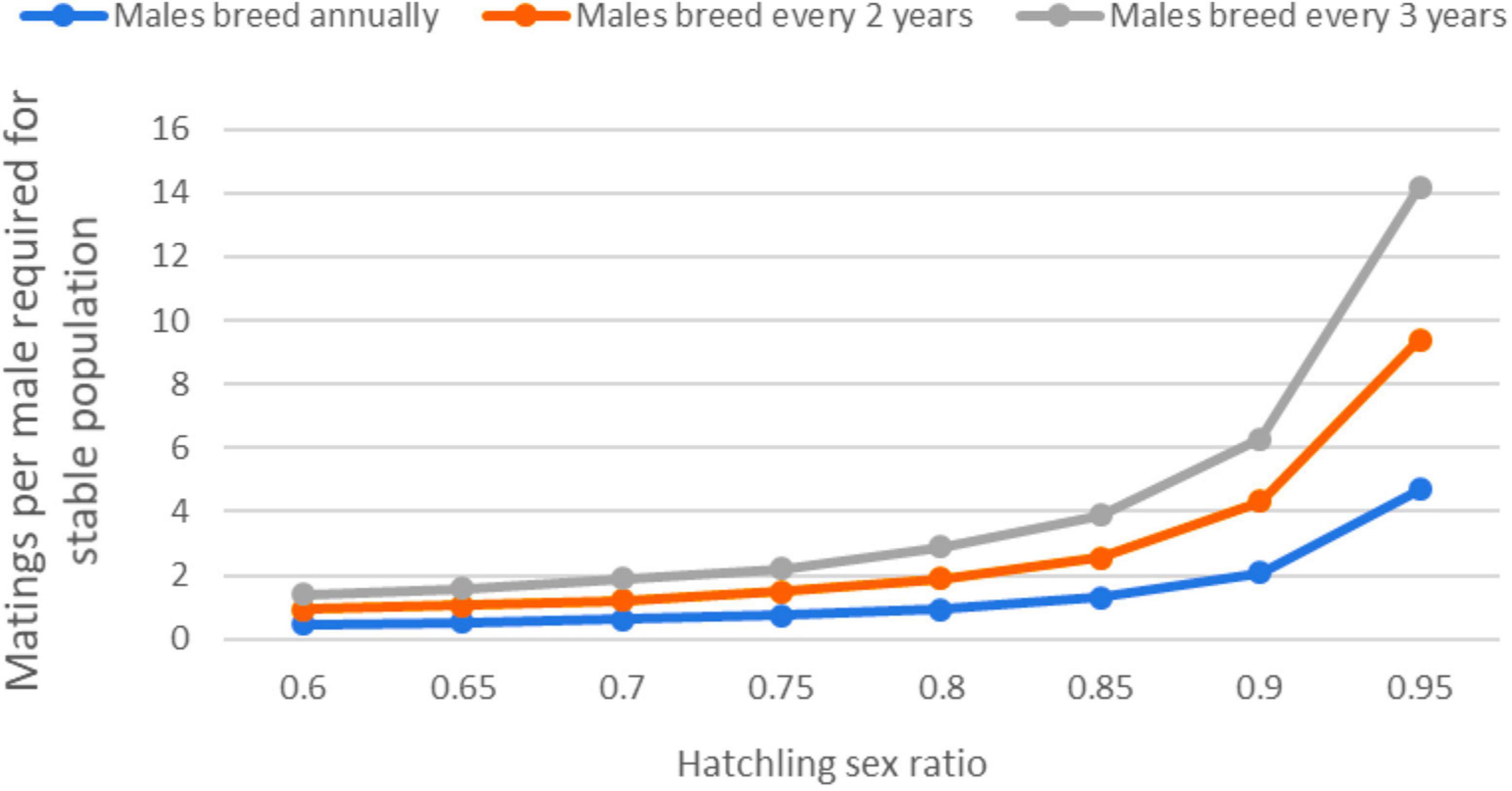
Figure 3. Matings per male required for a stable population growth rate (lambda = 1.0; 0% change per year) based on the deterministic model and three male remigration intervals (males breed every year, every 2 years, or every 3 years; females always breed every 3 years). Note that as the hatchling sex ratio becomes more female-biased that the burden of more matings per male increases. At the currently predominant primary sex ratio of 85% female, males must mate with multiple females to achieve a stable population even if they return to breed more often than females.
Stochastic population projections
When we incorporate sex ratio variability and trends in the models, we can evaluate how variance and trends in primary sex ratio affect the variance and trend of population level responses such as nest count, effective population size, and probability of extinction under different scenarios. In most simulations, the nest count initially increases, after a lag, due to an increase in females in the adult population and the underlying positive population growth rate of the base model. When there is an increasing trend in the primary sex ratio, simulated populations eventually collapse as males die out of the adult population (example shown in Figure 4), but the time lag to population collapse depends on male reproductive strategy and model parameters that we held constant, such as ASM and male lifespan (adult survival rate).
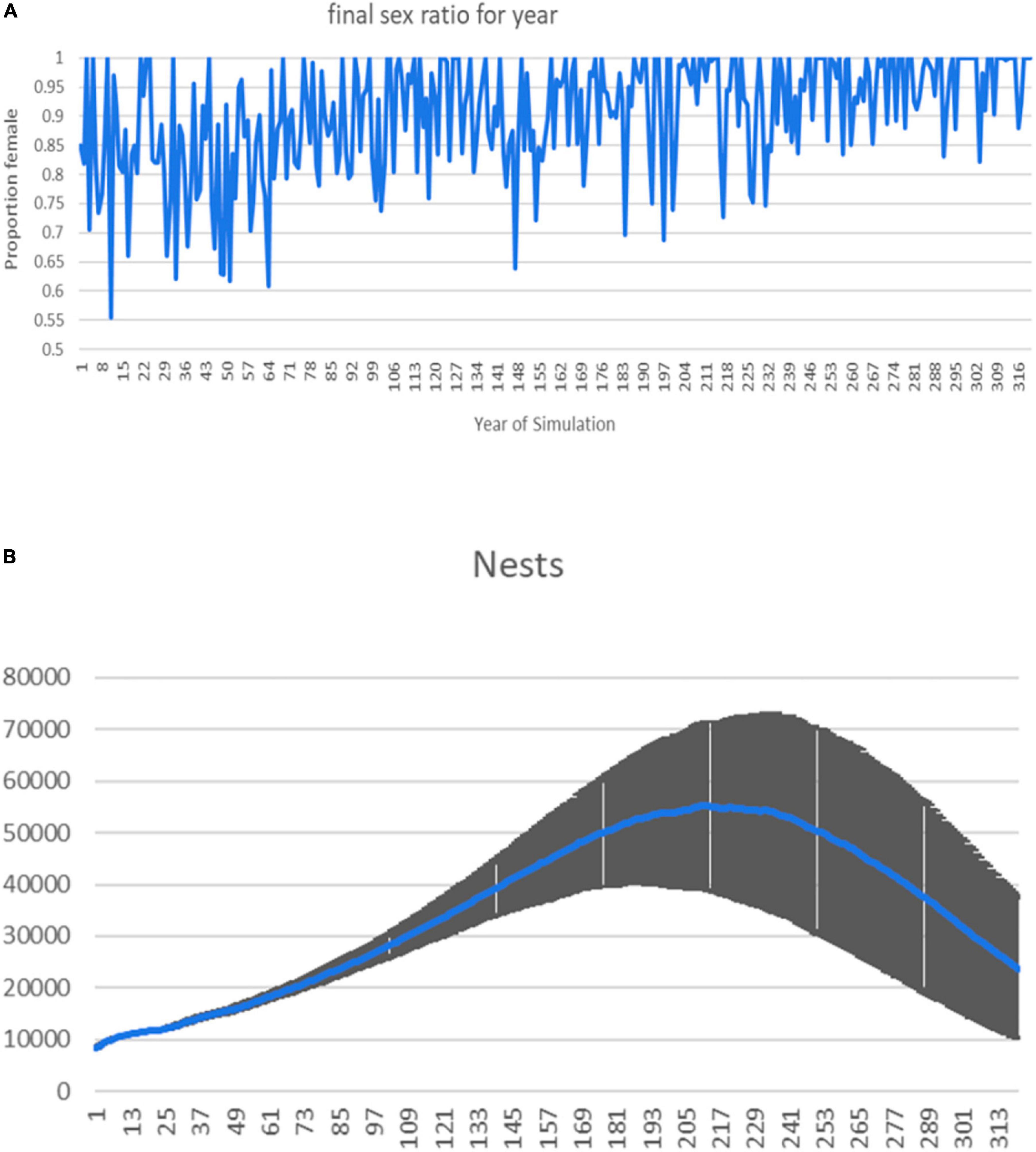
Figure 4. Example model results, showing an increasing female-biased sex ratio scenario where the mean hatching sex ratio starts at 85% female and trends upward linearly at 0.0005 (0.05%) per year (“slow increase”), reaching 99% females around year 200. (A) Example sex ratio through time from a single stochastic simulation. (B) Nest count mean and standard deviation envelope for 5,000 runs of the model. This scenario is for males breeding every 2 years, females every 3 years, and 5 matings per breeding year per male. Because the initial conditions for the simulations were based on a population with a positive annual rate of increase, this was a common pattern in the model outputs—an increase in nests due to the accumulation of highly biased female cohorts but sufficient males for reproduction, followed by a decline as males became limiting.
Mitigation strategy simulations
We used our simulation models to explore mitigation scenarios that increased the frequency of “good male years”—years with 30% male hatchling production—in loggerhead populations with different increasing female hatchling production (Table 3). We looked at population trends at year 100 and 300, probability of hitting the QET by year 300, and Ne at year 300. Under currently observed conditions in southern Florida, where hatchling sex ratio is approximately 85% female on average, adding just one good male year per decade nearly doubled the Ne of breeders and increased the predicted population growth rate by 0.2% per year (note that this simulated population was not predicted to reach its QET in the 300-year time period). Adding more good male years improved population metrics even more, but with diminishing returns. If sex ratios trend toward all female hatchling production, more good male years are needed to reduce population declines and may not be able to compensate. However, in our “slow” sex ratio trend example, adding a single good male year per decade was able to greatly decrease the probability of reaching extinction thresholds.
Discussion
Our results provide evidence for increasing feminization of loggerhead sea turtles in southeastern Florida and the potential consequences of an increasingly skewed adult sex ratio on population trend. The model simulations show that variance in hatchling sex ratios, male breeding frequency, and number of successful matings per male per breeding year have a large impact on the likelihood of population-level responses to increasing sex ratio skew. The model outputs can be viewed optimistically—it is likely to take a long time for primary sex ratio skew to have a strong negative effect at the population level, and occasional years with strong male cohorts can offset highly biased female cohorts even as they become more frequent. However, they can also be viewed pessimistically—males can and will become limiting in the future, given current trends, and this will have a significant impact on effective population size and, potentially, population viability.
Depending on the initial sex ratio, additional male production years could at least delay population collapse even if the increase in sex ratio is rapid (Figure 5). Thus, it appears that mitigation strategies that can increase the frequency of male production years could have an impact at the population level if sex ratio continues to increase in favor of females. This could be critical for Ne; when we examined the average primary sex ratio over the 300 years for all mitigation simulations, there is a predictable logistic pattern in the relationship between Ne and average proportion female hatchlings over the 300-year time period (example shown in Figure 6). Additional modeling and sensitivity analyses are needed to further evaluate this, and the feasibility of such efforts needs to be determined.
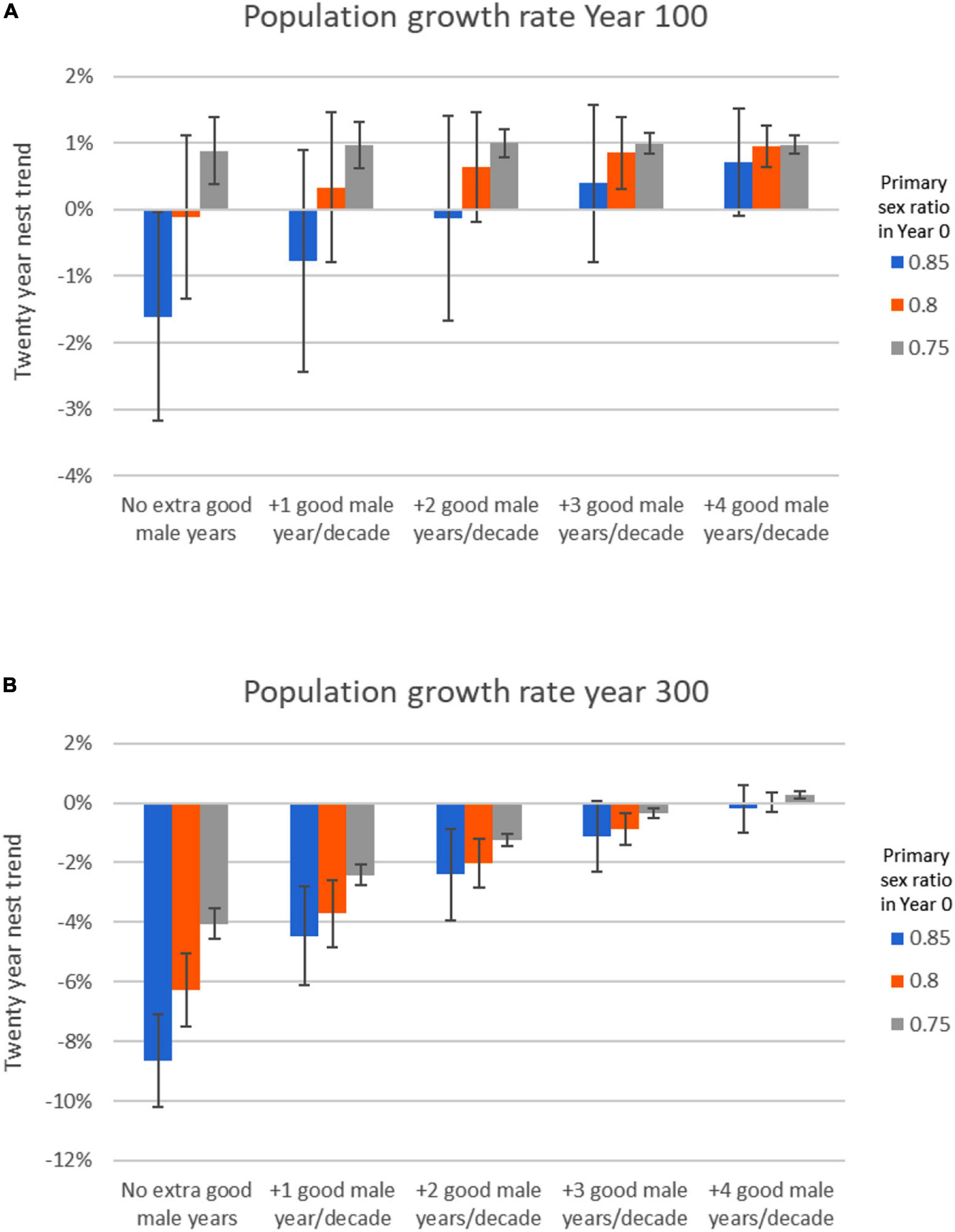
Figure 5. Exploring the potential effects of sex ratio mitigation on population growth rates for loggerhead sea turtles when hatchling sex ratios are otherwise gradually increasing to 100% female. The simulations have a primary sex ratio linear trend fixed at + 0.001 per year and start with an average of 85% female hatchling (blue bars), 80% female hatchlings (orange bars), or 75% female hatchlings (gray bars) in year 0. All simulations include a sex ratio standard deviation of 0.11, males breeding every 2 years, females breeding every 3 years, and males able to mate with 3 females per breeding season. “Good male years” are years with 30% male hatchlings, generated randomly with an average of 0, 1, 2, 3, or 4 years per decade. (A) Twenty-year trend in nests shown as % per year in simulation year 100. (B) Twenty-year trend in nests shown as % per year in simulation year 300. The population growth rate in the first 100 years is promising with just a few good male years (A). However, as the primary sex ratio becomes more skewed toward females, “good male years” must occur increasingly frequently for the population to be sustained (B).
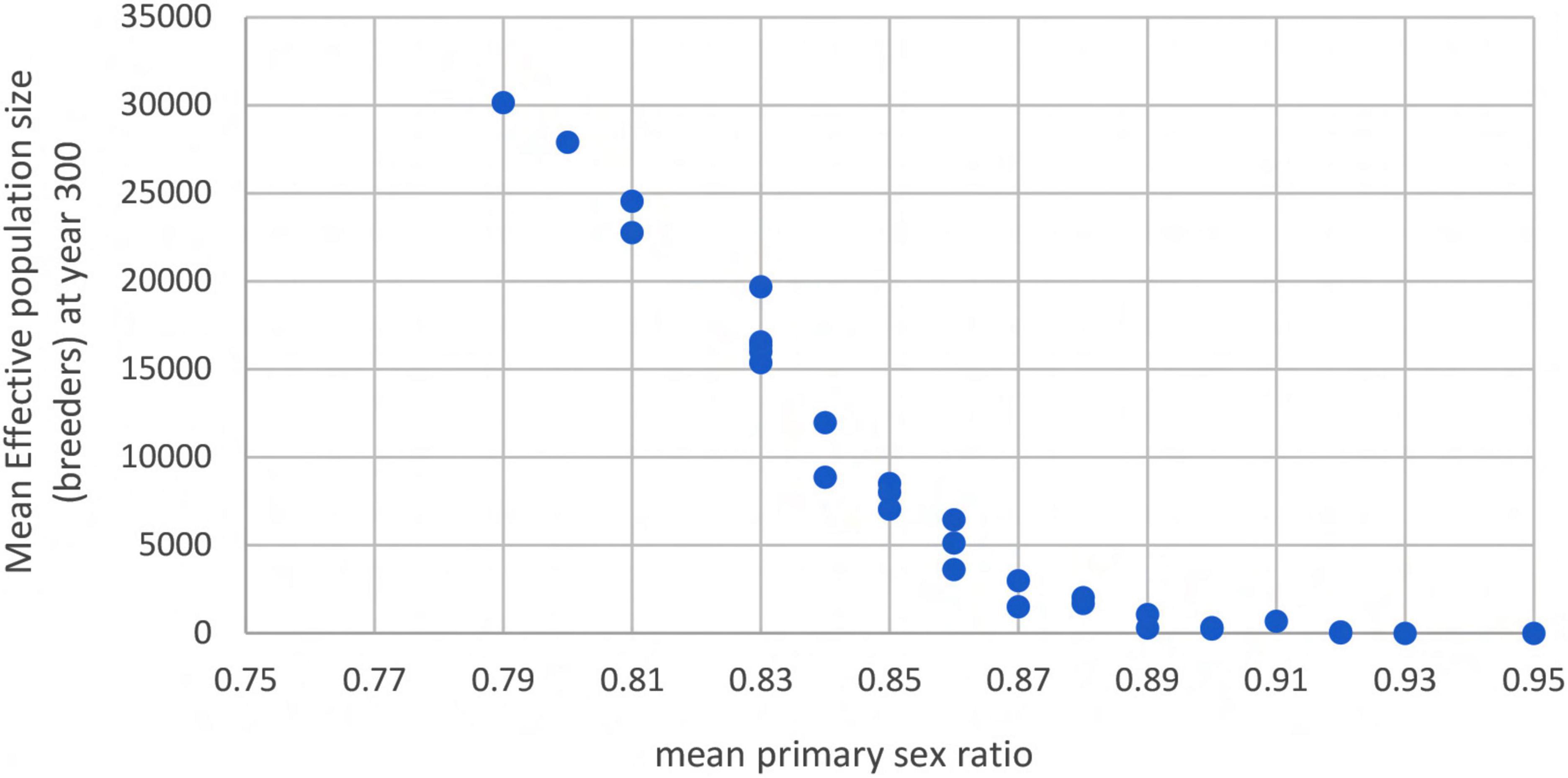
Figure 6. Example of the relationship between mean primary sex ratio (proportion females) and effective population size (Ne) when primary sex ratio is altered by increasing male hatchling production. Results shown are from simulations that included a sex ratio standard deviation of 0.11, males breeding every 2 years, females breeding every 3 years, and males able to mate with 3 females per breeding season. There is a sharp increase in Ne when mean primary sex ratio is reduced.
The sex ratio data collected across 15 seasons highlighted that there were just two seasons that approached the “good male years” primary sex ratio (70%:30% F:M). In most seasons (11 of 15), the primary sex ratios were > 85%F with most in the 93–100%F range. While strongly female-biased hatchling production appears to be typical and persistent for this species (Mrosovsky and Provancha, 1992, summary in Witt et al., 2010), our model alerts us that these sex ratios are likely to be deleterious in the long run. Maurer et al. (2021) flagged this same issue in the broader context of warmer temperatures leading to embryonic mortality and a preponderance of female hatchlings, the potential for sex-specific survival, then down-stream effects on adult sex ratios and male limitations in the breeding sex ratios. Wyneken and Salmon (2020) identified sublethal effects in the hatchlings that emerged from hot nests and hypothesized that delayed mortality is likely.
Climate change is affecting sex ratios in species with ESD. Sea turtle lineages have survived climate changes in the past (Silber et al., 2011; Wyneken, 2021), but current rates of temperature increase, changes in precipitation, sea level rise, and restricted range expansion due to human development may inhibit adaptation. Adaptation does not appear to be happening in the short term based on population-specific sampling linked to warmer vs. cooler nesting grounds (Jensen et al., 2018), and relationships of female-biasing temperatures to embryo survival (Wyneken and Lolavar, 2015; Wyneken and Salmon, 2020). Unless there are physiological shifts in thermal maxima (the temperatures above which successful embryonic development is compromised) and the sex ratio reaction norms, behavioral adaptation by older life stages may only delay extinction risks (Santidrián Tomillo et al., 2015). Others have speculated that highly female skewed sex ratios will initially increase the population as our models predict, yet the same populations later become problematic as the proportions of available males drops due to aging/death and lack of recruitment to the adult male life stage (Mrosovsky, 1994; Witt et al., 2010; Hays et al., 2014, 2017). Nevertheless, because juvenile and adult life stages are composed of many cohorts, it takes decades for the trend in primary sex ratio to appear as a strong skew. Monitoring sex ratios in nearshore populations of juveniles is important to identify impending shifts in sex ratio over time (Delgado et al., 2010; Arendt et al., 2021).
Most population assessments, especially in fisheries, assume that male abundance is not a limiting factor in reproduction, although there are some exceptions linked to sex ratio skew toward a female-dominated population (e.g., Southeast Data Assessment and Review [SEDAR], 2021). While the mating frequency range (number of female matings per male) we chose for our simulations may appear low, it is important to note a few elements that make these numbers reasonable. First, while we use the number of mating events per male on average across all males in the population, those averages are likely not normally distributed. If sea turtle reproductive behavior and female mate choice are similar to that found in many other species across the animal kingdom, there may be a few large, dominant males that garner many mating opportunities and a larger number of small males whose average reproductive success approaches zero in a given year. As the adult sex ratio becomes more biased toward females, subordinate males may be more likely to breed, but the average number of successful matings per male per year may remain very low. Second, we assign the same mortality rate to males and females for all life stages in our model. If male mortality is elevated during the breeding season due to risky behaviors and physiological demands caused by increased testosterone and mating attempts, then as a male mates with more and more females his mortality risk is increased. The energetic cost of mating will also increase with each successive breeding attempt as a male attempts copulation with females and potentially defends those copulation opportunities from other males. Clearly, we need to understand how many females a male can mate with, and the energetic and survival costs associated with increased mating attempts, to predict how behavior and potential adaptations to sex ratio change are likely to affect population productivity.
Our simulations are heuristic, and the models contain many simplifying assumptions. Thus, it is important to evaluate the relative differences in the simulation outputs rather than using the absolute results to predict specific outcomes. Extinction risk and potential loss of diversity through reductions in effective population size can be used as metrics to compare the effectiveness of alternative conservation strategies, even if uncertainty makes precise predictions of population response impossible. Mitchell et al. (2010) took a similar approach to ours in their study of extinction risk for tuatara, a highly endangered species with TSD that produces males at higher temperatures. Populations become female-limited at > 75% male in their simulations, with extinction predicted within 300 years. The authors show the relative potential for successful mitigation through nest shading and translocation, which may be feasible for this species restricted to small islands. For sea turtles, once better data are available on sex-specific vital rates, as well as male reproductive behaviors and reproductive success for these populations, more accurate assessments and mitigation planning can take place.
Research needs
Male breeding frequency and breeding capacity remain an enormous data gap for all species of sea turtles. Male hatchling production affects adult sex ratio through the accumulation of cohorts in the adult life stage, even if “good male years” are relatively rare. But the greatest uncertainties in potential population outcomes are male remigration frequency, the potential number of mates a male might have, and how the reproductive behavior of males drives effective population size. Our model highlighted how impactful male reproductive behavior is to population persistence. Further, the differences we found at year 100 and year 300 in the simulations highlight how precarious the population’s situation may be over longer time periods, yet the data to address these key parameters for southern Florida loggerheads are non-existent!
We can conduct additional physiology research to further evaluate what might happen mechanistically in a sperm-limited sea turtle population, which can guide the identification of biological indicators of climate change impacts. Because sea turtles store sperm following mating, there is some period of time during which a female might be able to “assess” her probability of producing viable offspring. Would females who fail to acquire sufficient sperm abort follicular maturation and conserve the energy and nutrients for later reproductive attempts? Abortive maturation, the interruption of maturation often attributed to unfavorable environmental factors that would lead to unsuccessful spawning, has been documented in fish (e.g., Conrath, 2017). Subsequent to the cessation of maturation, the nutrients and cellular components of the developing gametes are mobilized and resorbed through a mass atresia process (Corriero et al., 2021). While follicular atresia is documented in cheloniids (Miller and Limpus, 2003), it remains unknown whether sea turtles might undergo abortive maturation, as do fish. Evidence from other reptiles (Blackburn, 1998; Blackburn et al., 2003), indicate that they do not. It also isn’t known if sea turtles can resorb the nutrients utilized in the development of ova or if follicular atresia simple leads to waste products. Given a likely inability to utilize resorbed nutrients, might we see an increase in the proportion of unfertilized eggs in a given nest, or perhaps nests comprised entirely of unfertilized eggs? Alternatively, might female sea turtles either increase their remigration/breeding interval, reduce the number of nests laid in a year, or perhaps seek other breeding opportunities adjacent to nesting beaches further afield? Any of these potential outcomes would substantially alter population demography by decreasing the number of potential offspring produced per year for a given population.
Future work will need to be directed intentionally to try and answer these questions. At a minimum, monitoring for evidence of reduced reproductive activity and success as sex ratios continue to skew will be important. Other data needs will be more difficult to meet due to the inaccessibility of sea turtles during much of their life cycle and the protected nature of sea turtles. Fundamental data gaps need to be filled including knowing the quantity of stored sperm a female acquires as she enters the reproductive period and whether that decreases over time. In terms of behavior, enumerating the mating frequency of females, the remigration interval, and the number of fertilization attempts a male might undertake in a given breeding season are key metrics. Together these data will contribute to our understanding of how a skewed sex ratio may affect population productivity.
Finally, prognostic evaluation of the conservation benefits of manipulative mitigation strategies through simulation modeling is important (Heppell and Crowder, 1998). This starts with small scale experiments to determine if and how hatchling sex ratios can be modified in semi-natural or natural conditions. These experiments should be followed by modeling to compare projections of population response to determine how those activities must scale up to provide significant benefits at the population level. Conservation success should be evaluated with pre-defined metrics that indicate population-level responses, which will require long term monitoring.
Data availability statement
The data analyzed in this study was subject to the following licenses/restrictions: Requests to access these datasets should be directed to the corresponding author JW, jwyneken@fau.edu.
Author contributions
SSH, JW, and SAH conceived the study and contributed to the manuscript. JW collected and assembled the data. SSH designed the model, conducted the model runs and interpreted the results, and led the writing with input from all authors. SAH provided insight into the reproductive biology of the animals.
Funding
This study was supported in part by the personal funds.
Acknowledgments
We thank the M. Salmon and N. Thompson for invaluable comments early in the model design. K. Rusenko, M.D. Anderson, E. Turla, and the FAU Marine Lab students contributed to the data set. A. Pompero and G.R. Zaya provided perspectives throughout the study.
Conflict of interest
The authors declare that the research was conducted in the absence of any commercial or financial relationships that could be construed as a potential conflict of interest.
Publisher’s note
All claims expressed in this article are solely those of the authors and do not necessarily represent those of their affiliated organizations, or those of the publisher, the editors and the reviewers. Any product that may be evaluated in this article, or claim that may be made by its manufacturer, is not guaranteed or endorsed by the publisher.
References
Arendt, M. D., Schwenter, J. A., Owens, D. W., and Valverde, R. A. (2021). Theoretical modeling and neritic monitoring of loggerhead Caretta caretta [Linnaeus, 1758] sea turtle sex ratio in the southeast United States do not substantiate fears of a male-limited population. Glob. Chang. Biol. 27, 4849–4859. doi: 10.1111/gcb.15808
Blackburn, D. G. (1998). Resorption of oviductal eggs and embryos in squamate reptiles. Herpetol. J. 8, 65–71.
Blackburn, D. G., Weaber, K. K., Stewart, J. R., and Thompson, M. B. (2003). Do pregnant lizards resorb or abort inviable eggs and embryos? Morphological evidence from an Australian skink, Pseudemoia pagenstecheri. J. Morphol. 256, 219–234. doi: 10.1002/jmor.10094
Blechschmidt, J., Wittmann, M. J., and Blüml, C. (2020). Climate Change and Green Sea Turtle Sex Ratio -Preventing Possible Extinction. Genes 11:588. doi: 10.3390/genes11050588
Boyle, M., Hone, J., Schwanz, L. E., and Georges, A. (2014). Under what conditions do climate-driven sex ratios enhance versus diminish population persistence? Ecol. Evol. 4, 4522–4533. doi: 10.1002/ece3.1316
Burgman, M. A., Ferson, S., and Akçakaya, H. R. (1993). Risk Assessment In Conservation Biology. Berlin: Springer Science & Business Media.
Casale, P., and Tucker, A. D. (2017). Caretta caretta. The IUCN Red List of Threatened Species 2017: e.T3897A119333622. Available Online at: http://dx.doi.org/10.2305/IUCN.UK.2017-2.RLTS.T3897A119333622.en doi: 10.2305/IUCN.UK.2017-2.RLTS.T3897A119333622.en (accessed July 5, 2019).
Ceriani, S. A., Casale, P., Brost, M., Leone, E. H., and Witherington, B. E. (2019). Conservation implications of sea turtle nesting trends: Elusive recovery of a globally important loggerhead population. Ecosphere 10:e02936. doi: 10.1002/ecs2.2936
Ceriani, S. A., and Meylan, A. B. (2017). Caretta caretta Northwest Atlantic subpopulation (amended version of 2015 assessment). The IUCN Red List of Threatened Species 2017: e.T84131194A119339029. Available Online at: http://dx.doi.org/10.2305/IUCN.UK.2017-2.RLTS.T84131194A119339029.en (accessed December 2, 2018).
Conrath, C. L. (2017). Maturity, spawning omission, and reproductive complexity of deepwater rockfish. Trans. Am. Fish. Soc. 146, 495–507. doi: 10.1080/00028487.2017.1285352
Corriero, A., Zupa, R., Mylonas, C. C., and Passantino, L. (2021). Atresia of ovarian follicles in fishes, and implications and uses in aquaculture and fisheries. J. Fish Dis. 44, 1271–1291. doi: 10.1111/jfd.13469
Delgado, C., Canário, A. V., and Dellinger, T. (2010). Sex ratios of loggerhead sea turtles Caretta caretta during the juvenile pelagic stage. Mar. Biol. 157, 979–990. doi: 10.1007/s00227-009-1378-8
Florida Fish and Wildlife Conservation Commission [FLORIDA FWCC] (2016). Marine Turtle Conservation Handbook. Available Online at; https://myfwc.com/media/3133/fwc-mtconservationhandbook.pdf (accessed May 22, 2022).
Frankham, R. (1995). Effective population size/adult population size ratios in wildlife: A review. Genet. Res. 66, 95–107. doi: 10.1017/S0016672300034455
Frazer, N. B., and Ehrhart, L. M. (1985). Preliminary growth models for green (Chelonia mydas) and loggerhead (Caretta caretta) turtles in the wild. Copeia 1985, 73–79. doi: 10.2307/1444792
Fuentes, M. M. P. B., Fish, M. R., and Maynard, J. A. (2012). Management strategies to mitigate the impacts of climate change on sea turtle’s terrestrial reproductive phase. Mitig. Adapt. Strateg. Glob. Chang. 17, 51–63. doi: 10.1007/s11027-011-9308-8
Fuentes, M. M. P. B., Hamann, M., and Limpus, C. J. (2010). Past, current and future thermal profiles of green turtle nesting grounds: Implications from climate change. J. Exp. Mar. Biol. Ecol. 383, 56–64. doi: 10.1016/j.jembe.2009.11.003
Hawkes, L. A., Broderick, A. C., Godfrey, M. H., and Godley, B. J. (2009). Climate change and marine turtles. Endanger. Species Res. 7, 137–154. doi: 10.3354/esr00198
Hawkes, L. A., McGowan, A., Godley, B. J., Gore, S., Lange, A., Tyler, C. R., et al. (2013). Estimating sex ratios in Caribbean hawksbill turtles: Testosterone levels and climate effects. Aquat. Biol. 18, 9–19. doi: 10.3354/ab00475
Hays, G. C., Fossette, S., Katselidis, K. A., Schofield, G., and Gravenor, M. B. (2010). Breeding periodicity for male sea turtles, operational sex ratios, and implications in the face of climate change. Conserv. Biol. 24, 1636–1643. doi: 10.1111/j.1523-1739.2010.01531.x
Hays, G. C., Mazaris, A. D., and Schofield, G. (2014). Different male vs. female breeding periodicity helps mitigate offspring sex ratio skews in sea turtles. Front. Mar. Sci. 1:43. doi: 10.3389/fmars.2014.00043
Hays, G. C., Mazaris, A. D., Schofield, G., and Laloë, J. O. (2017). Population viability at extreme sex-ratio skews produced by temperature-dependent sex determination. Proc. R. Soc. B 284:20162576. doi: 10.1098/rspb.2016.2576
Heppell, S. S., Crouse, D., Crowder, L., Epperly, S., and Frazer, N. (2003). “Population models for Atlantic loggerheads: past, present and future,” in Loggerhead Sea Turtles, eds A. Bolten and B. Witherington (Washington, DC: Smithsonian Institution Press), 255–273.
Heppell, S. S., and Crowder, L. (1998). Prognostic evaluation of enhancement programs using population models and life history analysis. Bull. Mar. Sci. 62, 495–507. doi: 10.2307/1447430
Heppell, S. S., Snover, M. L., and Crowder, L. (2002). “Sea turtle population dynamics,” in Biology of Sea Turtles, Vol. 2, eds P. Lutz, J. Wyneken, and J. Musick (Boca Raton, FL: CRC Press), 275–306. doi: 10.1201/9781420040807.ch11
Janzen, F. J. (1994). Climate change and temperature-dependent sex determination in reptiles. Proc. Natl. Acad. Sci. U.S.A. 91, 7487–7490. doi: 10.1073/pnas.91.16.7487
Jensen, M. P., Allen, C. D., Eguchi, T., Bell, I. P., LaCasella, E. L., Hilton, W. A., et al. (2018). Environmental warming and feminization of one of the largest sea turtle populations in the world. Curr. Biol. 28, 154–159. doi: 10.1016/j.cub.2017.11.057
Jensen, M. P., Eguchi, T., FitzSimmons, N. N., McCarthy, M. A., Fuentes, M. M., Hamann, M., et al. (2022). Integrating climate change and management scenarios in population models to guide the conservation of marine turtles. Bull. Mar. Sci. 98, 131–154. doi: 10.5343/bms.2021.0033
Jessop, T. S., Hamann, M., and Limpus, C. J. (2004). Body condition and physiological changes in male green turtles during breeding. Mar. Ecol. Prog. Ser. 276, 281–288. doi: 10.3354/meps276281
Jourdan, J., and Fuentes, M. M. P. B. (2015). Effectiveness of strategies at reducing sand temperature to mitigate potential impacts from changes in environmental temperature on sea turtle reproductive output. Mitig. Adapt. Strateg. Glob. Chang. 20, 121–133. doi: 10.1007/s11027-013-9482-y
Kobayashi, S., Wada, M., Fujimoto, R., Kumazawa, Y., Arai, K., Watanabe, G., et al. (2017). The effects of nest incubation temperature on embryos and hatchlings of the loggerhead sea turtle: Implications of sex difference for survival rates during early life stages. J. Exp. Mar. Biol. Ecol. 486, 274–281. doi: 10.1016/j.jembe.2016.10.020
Komoroske, L. M., Jensen, M. P., Stewart, K. R., Shamblin, B. M., and Dutton, P. H. (2017). Advances in the application of genetics in marine turtle biology and conservation. Front. Mar. Sci. 4:156. doi: 10.3389/fmars.2017.00156
Laloë, J. O., Esteban, N., Berkel, J., and Hays, G. C. (2016). Sand temperatures for nesting sea turtles in the Caribbean: Implications for hatchling sex ratios in the face of climate change. J. Exp. Mar. Biol. Ecol. 474, 92–99. doi: 10.1016/j.jembe.2015.09.015
Laloë, J. O., Tedeschi, J. N., Booth, D. T., Bell, I., Dunstan, A., Reina, R. D., et al. (2021). Extreme rainfall events and cooling of sea turtle clutches: Implications in the face of climate warming. Ecol. Evol. 11, 560–565. doi: 10.1002/ece3.7076
Lasala, J. A., Harrison, J. S., Williams, K. L., and Rostal, D. C. (2013). Strong male-biased operational sex ratio in a breeding population of loggerhead turtles (Caretta caretta) inferred by paternal genotype reconstruction analysis. Ecol. Evol. 3, 4736–4747. doi: 10.1002/ece3.761
Lee, P. L., Schofield, G., Haughey, R. I., Mazaris, A. D., and Hays, G. C. (2018). A review of patterns of multiple paternity across sea turtle rookeries. Adv. Mar. Biol. 79, 1–31. doi: 10.1016/bs.amb.2017.09.004
Lolavar, A., and Wyneken, J. (2015). Effect of rainfall on loggerhead turtle nest temperatures, sand temperatures and hatchling sex. Endanger. Species Res. 28, 235–247. doi: 10.3354/esr00684
Lolavar, A., and Wyneken, J. (2021). Effects of supplemental watering on loggerhead (Caretta caretta) nests and hatchlings. J. Exp. Mar. Biol. Ecol. 534:151476. doi: 10.1016/j.jembe.2020.151476
Maurer, A. S., Seminoff, J. A., Layman, C. A., Stapleton, S. P., Godfrey, M. H., and Reiskind, M. O. B. (2021). Population viability of sea turtles in the context of global warming. Bioscience 71, 790–804. doi: 10.1093/biosci/biab028
Miller, J. D., and Limpus, C. J. (2003). “Ontogeny of marine turtle gonads,” in The Biology of Sea Turtles, eds P. L. Lutz, J. A. Musick, and J. Wyneken (Boca Raton: CRC Press, Inc), 199–224. doi: 10.1201/9781420040807.ch7
Mitchell, N. J., Allendorf, F. W., Keall, S. N., Daugherty, C. H., and Nelson, N. J. (2010). Demographic effects of temperature-dependent sex determination: Will tuatara survive global warming? Glob. Chang. Biol. 16, 60–72. doi: 10.1111/j.1365-2486.2009.01964.x
Mitchell, N. J., and Janzen, F. J. (2010). Temperature-dependent sex determination and contemporary climate change. Sex. Dev. 4, 129–140. doi: 10.1159/000282494
Monsinjon, J. R., Wyneken, J., Rusenko, K., López-Mendilaharsu, M., Lara, P., Santos, A., et al. (2019). The climatic debt of loggerhead sea turtle populations in a warming world. Ecol. Indic. 107:105657. doi: 10.1016/j.ecolind.2019.105657
Mrosovsky, N. (1994). Sex ratios of sea turtles. J. Exp. Zool. 270, 16–27. doi: 10.1002/jez.1402700104
Mrosovsky, N., and Provancha, J. (1992). Sex ratio of hatchling loggerhead sea turtles-data and estimates from a 5-year study. Can. J. Zool. 70, 530–538. doi: 10.1139/z92-080
National Marine Fisheries Service, and US Fish and Wildlife Service [NMFS AND USFWS] (2008). Recovery plan for the northwest Atlantic population of the loggerhead sea turtle (Caretta caretta). Second revision. Silver Spring: National Marine Fisheries Service.
Patrício, A. R., Hawkes, L. A., Monsinjon, J. R., Godley, B. J., and Fuentes, M. M. (2021). Climate change and marine turtles: Recent advances and future directions. Endanger. Species Res. 44, 363–395. doi: 10.3354/esr01110
Patrício, A. R., Varela, M. R., Barbosa, C., Broderick, A. C., Catry, P., Hawkes, L. A., et al. (2019). Climate change resilience of a globally important sea turtle nesting population. Glob. Chang. Biol. 25, 522–535. doi: 10.1111/gcb.14520
Phillips, K. F., Stahelin, G. D., Chabot, R. M., and Mansfield, K. L. (2021). Long-term trends in marine turtle size at maturity at an important Atlantic rookery. Ecosphere 12:e03631. doi: 10.1002/ecs2.3631
Rout, T. M., McDonald-Madden, E., Martin, T. G., Mitchell, N. J., Possingham, H. P., and Armstrong, D. P. (2013). How to decide whether to move species threatened by climate change. PLoS One 8:e75814. doi: 10.1371/journal.pone.0075814
Santidrián Tomillo, P., Genovart, M., Paladino, F. V., Spotila, J. R., and Oro, D. (2015). Climate change overruns resilience conferred by temperature-dependent sex determination in sea turtles and threatens their survival. Glob. Chang. Biol. 21, 2980–2988. doi: 10.1111/gcb.12918
Sari, F., Koseler, A., and Kaska, Y. (2017). First observation of multiple paternity in loggerhead sea turtles, Caretta caretta, nesting on Dalyan Beach, Turkey. J. Exp. Mar. Biol. Ecol. 488, 60–71. doi: 10.1016/j.jembe.2016.11.018
Silber, S., Geisler, J. H., and Bolortsetseg, M. (2011). Unexpected resilience of species with temperature-dependent sex determination at the Cretaceous–Palaeogene boundary. Biol. Lett. 7, 295–298. doi: 10.1098/rsbl.2010.0882
Southeast Data Assessment and Review [SEDAR] (2021). SEDAR 72 Gulf of Mexico Gag Grouper Final Stock Assessment Report. North Charleston SC: SEDAR, 319.
Stewart, K. R., and Dutton, P. H. (2011). Paternal genotype reconstruction reveals multiple paternity and sex ratios in a breeding population of leatherback turtles (Dermochelys coriacea). Conserv. Genet. 12, 1101–1113. doi: 10.1007/s10592-011-0212-2
Tanner, C. E., Marco, A., Martins, S., Abella-Perez, E., and Hawkes, L. A. (2019). Highly feminised sex-ratio estimations for the world’s third-largest nesting aggregation of loggerhead sea turtles. Mar. Ecol. Prog. Ser. 621, 209–219. doi: 10.3354/meps12963
Theissinger, K., FitzSimmons, N. N., Limpus, C. J., Parmenter, C. J., and Phillott, A. D. (2009). Mating system, multiple paternity and effective population size in the endemic flatback turtle (Natator depressus) in Australia. Conserv. Genet. 10, 329–346. doi: 10.1007/s10592-008-9583-4
Tucker, A. D. (2010). Nest site fidelity and clutch frequency of loggerhead turtles are better elucidated by satellite telemetry than by nocturnal tagging efforts: Implications for stock estimation. J. Exp. Mar. Biol. Ecol. 383, 48–55. doi: 10.1016/j.jembe.2009.11.009
Turtle Expert Working Group [TEWG] (2009). An assessment of the loggerhead turtle population in the western North Atlantic Ocean. NOAA Tech Memo NMFS-SEFSC-575. Washington, DC: NOAA.
Warden, M. L., Haas, H. L., Rose, K. A., and Richards, P. M. (2015). A spatially explicit population model of simulated fisheries impact on loggerhead sea turtles (Caretta caretta) in the Northwest Atlantic Ocean. Ecol. Modell. 299, 23–39. doi: 10.1016/j.ecolmodel.2014.11.025
Warner, D. A., and Shine, R. (2011). Interactions among thermal parameters determine offspring sex under temperature-dependent sex determination. Proc. R. Soc. B 278, 256–265. doi: 10.1098/rspb.2010.1040
Wei, Y., Song, B., and Yuan, S. (2021). Dynamics of a ratio-dependent population model for Green Sea Turtle with age structure. J. Theor. Biol. 516:110614. doi: 10.1016/j.jtbi.2021.110614
Witt, M. J., Hawkes, L. A., Godfrey, M. H., Godley, B. J., and Broderick, A. C. (2010). The impacts of climate change on a globally distributed species: The case of the loggerhead turtle. J. Exp. Biol. 213, 901–911. doi: 10.1242/jeb.038133
Woodhead, A. J., Hicks, C. C., Norström, A. V., Williams, G. J., Graham, N. A. J., and Fox, C. (2019). Coral reef ecosystem services in the Anthropocene. Funct. Ecol. 33, 1023–1034. doi: 10.1111/1365-2435.13331
Wright, L. I., Stokes, K. L., Fuller, W. J., Godley, B. J., McGowan, A., Snape, R., et al. (2012a). Turtle mating patterns buffer against disruptive effects of climate change. Proc. R. Soc. B 279, 2122–2127. doi: 10.1098/rspb.2011.2285
Wright, L. I., Fuller, W. J., Godley, B. J., McGowan, A., Tregenza, T., and Broderick, A. C. (2012b). Reconstruction of paternal genotypes over multiple breeding seasons reveals male green turtles do not breed annually. Mol. Ecol. 21, 3625–3635. doi: 10.1111/j.1365-294X.2012.05616.x
Wyneken, J. (2021). What does climate change mean for sea turtles? SWOT Report. State World’s Sea Turtles 16:46.
Wyneken, J., Epperly, S., Crowder, L. B., Vaughan, J., and Blair, K. (2007). Determining sex in posthatchling loggerhead sea turtles using multiple gonadal and accessory duct characteristics. Herpetologica 63, 19–30. doi: 10.1655/0018-0831(2007)63[19:DSIPLS]2.0.CO;2
Wyneken, J., and Lolavar, A. (2015). Loggerhead sea turtle environmental sex determination: Implications of moisture and temperature for climate change-based predictions for species survival. J. Exp. Zool. B Mol. Dev. Evol. 324, 295–314. doi: 10.1002/jez.b.22620
Wyneken, J., and Salmon, M. (2020). Linking ecology, morphology and behavior to conservation: lessons learned from studies of sea turtles. Integr. Comp. Biol. 60, 440–455. doi: 10.1093/icb/icaa044
Keywords: sea turtle, sex ratio, loggerhead, Caretta caretta, temperature dependent sex determination, climate change, mitigation, effective population size Ne
Citation: Heppell SS, Wyneken J and Heppell SA (2022) A morphologist, a modeler, and an endocrinologist consider sea turtle sex ratios in a changing climate. Some wine was involved. Front. Ecol. Evol. 10:952432. doi: 10.3389/fevo.2022.952432
Received: 25 May 2022; Accepted: 17 August 2022;
Published: 15 September 2022.
Edited by:
Amanda Wilson Carter, The University of Tennessee, Knoxville, United StatesReviewed by:
Fredric J. Janzen, Michigan State University, United StatesFikret Sari, Pamukkale University, Turkey
Copyright © 2022 Heppell, Wyneken and Heppell. This is an open-access article distributed under the terms of the Creative Commons Attribution License (CC BY). The use, distribution or reproduction in other forums is permitted, provided the original author(s) and the copyright owner(s) are credited and that the original publication in this journal is cited, in accordance with accepted academic practice. No use, distribution or reproduction is permitted which does not comply with these terms.
*Correspondence: Jeanette Wyneken, jwyneken@fau.edu
†These authors have contributed equally to this work and share senior authorship