- 1Department of Biology, Limnological Institute, University of Konstanz, Konstanz, Germany
- 2WasserCluster Lunz—Inter-University Centre for Aquatic Ecosystem Research, University of Vienna, Lunz am See, Austria
- 3Université de Brest, CNRS, IRD, Ifremer, LEMAR, Plouzané, France
- 4UMR DECOD (Ecosystem Dynamics and Sustainability), Ifremer, INRAE, Institut Agro, Plouzané, France
- 5Max Planck Institute of Animal Behavior, Radolfzell, Germany
- 6Department of Fish Ecology and Evolution, Eawag, Swiss Federal Institute of Aquatic Science and Technology, Kastanienbaum, Switzerland
- 7Department of Biomedical Research, Danube University Krems, Krems an der Donau, Austria
- 8Department of Aquatic Ecology, Research Station Bad Saarow, Brandenburg University of Technology Cottbus–Senftenberg, Bad Saarow, Germany
Emergent insects represent a key vector through which aquatic nutrients are transferred to adjacent terrestrial food webs. Aquatic fluxes of polyunsaturated fatty acids (PUFA) from emergent insects are particularly important subsidies for terrestrial ecosystems due to high PUFA contents in several aquatic insect taxa and their physiological importance for riparian predators. While recent meta-analyses have shown the general dichotomy in fatty acid profiles between aquatic and terrestrial ecosystems, differences in fatty acid profiles between aquatic and terrestrial insects have been insufficiently explored. We examined the differences in fatty acid profiles between aquatic and terrestrial insects at a single aquatic-terrestrial interface over an entire growing season to assess the strength and temporal consistency of the dichotomy in fatty acid profiles. Non-metric multidimensional scaling clearly separated aquatic and terrestrial insects based on their fatty acid profiles regardless of season. Aquatic insects were characterized by high proportions of long-chain PUFA, such as eicosapentaenoic acid (20:5n-3), arachidonic acid (20:4n-6), and α-linolenic acid (18:3n-3); whereas terrestrial insects were characterized by high proportions of linoleic acid (18:2n-6). Our results provide detailed information on fatty acid profiles of a diversity of aquatic and terrestrial insect taxa and demonstrate that the fundamental differences in fatty acid content between aquatic and terrestrial insects persist throughout the growing season. However, the higher fatty acid dissimilarity between aquatic and terrestrial insects in spring and early summer emphasizes the importance of aquatic emergence as essential subsidies for riparian predators especially during the breading season.
Introduction
Dietary energy and nutrients are continuously exchanged at the aquatic-terrestrial interface and can support food webs in adjacent ecosystems (Polis and Hurd, 1996; Nakano and Murakami, 2001). Terrestrial ecosystems can support aquatic food webs through the discharge of terrestrial organic matter from surrounding catchment areas or the deposition of leaf litter and invertebrates from overhanging vegetation (Tanentzap et al., 2017). Aquatic-derived nutrients can be transferred to terrestrial ecosystems via flooding, fish predation, birds, or organisms with an aquatic larval stage, such as emergent aquatic insects (Gladyshev et al., 2013; Richardson and Sato, 2015). During their larval stage, many aquatic insects feed on aquatic primary producers, while later as adult insects (i.e., imago) they emerge from aquatic ecosystems into the surrounding landscape for reproduction. Emerging insects that disperse into adjacent terrestrial ecosystems can become prey for riparian predators, thereby transferring aquatic-based nutrients across ecosystem boundaries (Sanzone et al., 2003). Stable isotope analyses have repeatedly shown the importance of emergent insects for riparian predators, such as birds, bats, lizards, and spiders (Baxter et al., 2005). For example, the abundance of spiders in the riparian zone is strongly linked with the emergence of aquatic insects (Kato et al., 2003; Paetzold et al., 2005; Burdon and Harding, 2008) and some riparian spiders can obtain the majority of their carbon from aquatic-derived sources (Sanzone et al., 2003; Kautza et al., 2016). Freshwater ecosystems typically receive higher subsidies from terrestrial ecosystems than vice versa, but the contribution of subsidies to animal carbon in adjacent habitats is similar, suggesting that aquatic subsidies are of higher quality (Bartels et al., 2012). Recent studies have documented fundamental differences in essential biochemical nutrients, i.e., polyunsaturated fatty acids (PUFA), between aquatic and terrestrial primary producers as well as consumers at multiple trophic levels (e.g., Hixson et al., 2015; Twining et al., 2021a).
The fundamental dichotomy between fatty acid (FA) profiles of aquatic and terrestrial subsidies is driven by the higher abundance of physiologically important long-chain (≥ C20) PUFA (LC-PUFA) in aquatic ecosystems (Hixson et al., 2015). LC-PUFA play a central role in membrane physiology and serve as precursors for a plethora of other bioactive molecules (Harayama and Shimizu, 2020). Beneficial effects of dietary LC-PUFA on performance, growth, and reproduction have been documented for several aquatic (von Elert, 2002; Martin-Creuzburg et al., 2010; Brzeziński and von Elert, 2015; Isanta Navarro et al., 2019) and terrestrial consumers (e.g., Maillet and Weber, 2006; Brenna and Carlson, 2014; Twining et al., 2016; Fritz et al., 2017). In order to satisfy their physiological demands, consumers in both aquatic and terrestrial ecosystems must either take up LC-PUFA from their diet directly, or biosynthesize them from dietary C18-PUFA precursors (Twining et al., 2021a). The dichotomy in FA profiles of aquatic and terrestrial consumers can be traced back to the ability of primary producers to synthesize LC-PUFA (Harwood, 1996). Several algal groups contain high proportions of LC-PUFA, such as arachidonic acid (ARA; 20:4n6), eicosapentaenoic acid (EPA; 20:5n-3), and docosahexaenoic acid (DHA; 22:6n-3; Taipale et al., 2013). In contrast, vascular terrestrial plants typically contain only C18-PUFA, such as linoleic acid (LIN; 18:2n-6) and α-linolenic acid (ALA; 18:3n-3), and lack the ability to synthesize LC-PUFA (Uttaro, 2006; Hixson et al., 2015). This ecosystem-based (i.e., aquatic compared to terrestrial) difference in primary producers’ FA profiles is transferred to higher trophic levels and is also observed between the FA profiles of aquatic and terrestrial insects (Guo et al., 2018; Kowarik et al., 2021; Mathieu-Resuge et al., 2021b; Twining et al., 2021b).
A global meta-analysis comparing multiple trophic levels from aquatic and terrestrial ecosystems demonstrated a statistically significant, but moderate difference between the FA profile of aquatic and terrestrial insects, with differences mainly linked to LC-PUFA content (e.g., EPA; Hixson et al., 2015). Although several insect families were represented in this global dataset, the insect sample size was rather limited (n < 70), which may explain the large variation observed in insect FA profiles. Insects from both ecosystems have also been compared in regard to their quality as prey for insectivorous riparian predators such as birds, spiders, and bats (Lam et al., 2013; Kowarik et al., 2021; Twining et al., 2021b). Aquatic insects have been found to be rich in EPA, while terrestrial insects were found to be higher in ALA (e.g., Hymenoptera and Lepidoptera; Twining et al., 2021c). However, several of these previous studies were limited to the breeding seasons of riparian birds reliant on insects (Twining et al., 2021b,c; Shipley et al., 2022) and thus did not capture additional temporal variation in insect diversity and FA composition. For example, biomass export and community composition of lake emergent insects are both highly variable throughout the year (i.e., temporal variation) and depend on lake morphometry (i.e., spatial variation; Martin-Creuzburg et al., 2017; Mathieu-Resuge et al., 2021a). The relative abundance of terrestrial insects in the riparian zone of rivers also exhibits spatial and seasonal variation (Nsor et al., 2020). Considering the variability in insect communities and their importance as high-quality food for riparian predators, a more extensive comparison of FA profiles of aquatic and terrestrial insects is warranted.
We examined FA profiles of aquatic and terrestrial insects collected using emergence traps deployed on a mid-size, mesotrophic lake from five different water depths and Malaise/window hybrid traps deployed at three distances away from the shoreline of the lake. Unlike previous studies, we sampled aquatic and terrestrial insects within and around a single lake throughout an entire growing season (April to October). We explored (1) differences in FA profiles between aquatic and terrestrial insects, (2) the main FA that drive the potential separation between aquatic and terrestrial insects, and (3) temporal changes in the strength of this FA separation during the growing season. We provide a thorough dataset of aquatic and terrestrial insect FA profiles (FA content per unit biomass), as well as visualize the similarities and/or differences between taxa with respect to 30 quantifiable FA. We predicted that terrestrial insects contain higher contents of C18-PUFA (i.e., ALA and LIN), whereas aquatic insects contain higher contents of ≥ C20-PUFA (i.e., EPA and DHA).
Materials and methods
Study site and sampling
Aquatic and terrestrial insects were, respectively, collected on and around Lake Mindelsee, southwestern Germany (47.754°N, 9.022°E; Supplementary Figure 1). The mid-size lake (1.02 km2) is mesotrophic (total phosphorus: 16.2 μg L–1, total nitrogen: 1,114 μg L–1) with a maximum depth of ∼13 m. The littoral zone is mostly surrounded by reeds, except for the southern portion, which is bordered by an old forest stand dominated by beech and conifers.
Aquatic insects were sampled using floating emergence traps (Figure 1A) that were placed on the water surface in two transects at water depths of 1 (n = 6), 3 (n = 6), 7 (n = 2), 9 (n = 2), and 12 (n = 3) m. Aquatic traps were constructed using four floatable plastic tubes (covering an area of 0.36 m2), smaller plastic pipes covered in extra fine mosquito net (mesh size ∼ 500 μm) forming a pyramid, and a plastic beaker on top with a narrowing opening. The emergence traps were emptied twice a week from April to October 2019 and the insects on the inside of the netting were collected using a self-made vacuum-powered aspirator.
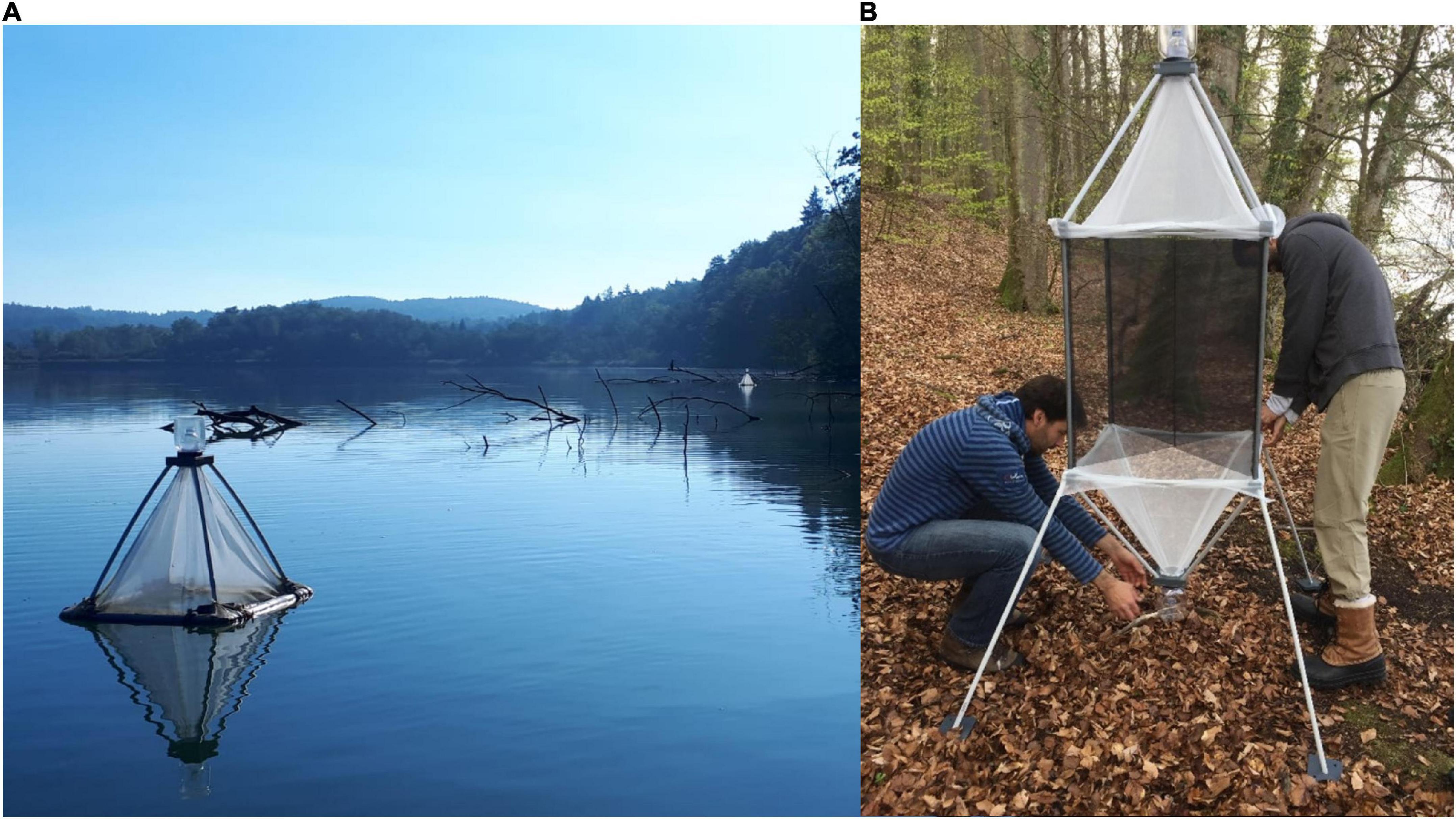
Figure 1. (A) Emergence traps floating on Lake Mindelsee deployed in two transects at the water surface with water depths of 1, 3, 7, 9, and 12 m and (B) Malaise/window hybrid traps deployed in two transects at 1, 100, and 1,000 m from the shoreline.
Terrestrial insects were collected using Malaise/window hybrid traps (Figure 1B). These traps consisted of four extra fine mosquito net (mesh size ∼ 500 μm) “windows” oriented in a cross (equaling a catch area of 1.72 m2) attached to two net-covered pyramids, one leading to a plastic beaker with funnel (to reduce the chances of insects leaving the trap) on top of the catch area and another at the bottom. The design of the trap was intended to catch flying insects that would either fly upwards or drop down when encountering one of the “window” obstacles. The traps were deployed with the catch area one meter above the ground in clear areas without high or hanging vegetation within 1 meter of the trap. The traps were deployed in two transects at distances of 1 m (n = 2), 100 m (n = 2), and 1,000 m (n = 2) from the shore of Lake Mindelsee. Due to logistic constraints, the traps were sampled once a month with the traps deployed for 3 days during non-raining periods between April and October 2019. All insects in the beakers as well as any in the catch area were collected using a vacuum-powered aspirator.
All aquatic and terrestrial insects were collected and transported to the laboratory live, where they were snap-frozen (−20°C), identified using a stereomicroscope, and then stored at −80°C until being freeze-dried, weighed (Mettler Toledo XP2U, ± 0.1 μg), and stored again at −80°C until FA analysis. Insects were classified to order (more specific if possible) and to be of aquatic, semi-aquatic (i.e., emerging from a constantly wet shoreline area), or terrestrial origin. Traps were non-selective and therefore, non-insect arthropod taxa within the traps were also collected and analyzed (Supplementary Table 1).
Fatty acid analysis
Freeze-dried insects were deposited in 5 mL of dichloromethane:methanol (2:1, v:v) and stored overnight at −20°C. Samples were then homogenized using a glass rod, sonicated, and the total lipids were extracted three times with dichloromethane:methanol (2:1, v:v). Pooled lipid extracts were evaporated to dryness under a stream of N2 at 40°C and transesterified with methanolic HCl (3 mole L–1, 60°C, 20 min, Sigma-Aldrich 33050-U). Fatty acid methyl esters (FAME) were extracted three times with isohexane (2 mL). Pooled FAME-containing fractions were evaporated to dryness under N2 at 40°C and resuspended in isohexane; the final volume was between 10 and 100 μL, depending on insect biomass, and subsequently stored at −20°C. FAME were analyzed by gas chromatography (GC) using a 7890B gas chromatograph (Agilent Technologies) equipped with a flame ionization detector (FID) and a DB-225 (J&W Scientific, 30 m × 0.25 mm inner diameter (id) × 0.25 μm film) capillary column. The following temperature gradient was applied: 60°C for 1 min, then increase to 150°C (at a rate of 30°C min–1), continued increase to 170°C (3°C min–1) and final increase to 220°C (2°C min–1) that was held for 10 min. FAME were quantified by comparison to an internal FAME standards (C17:0 and C23:0) of known concentrations (adapted to insect biomass in the sample), using multipoint calibration curves generated using FAME standards (Sigma-Aldrich). FAME were identified by their retention times and their mass spectra, which were recorded with a quadrupole gas chromatograph-mass spectrometer (GC–MS; Agilent Technologies, 5975C inert MSD) equipped with a DB-225MS fused-silica capillary column (J&W Scientific, 30 m × 0.25 mm id × 0.25 μm film); gas chromatographic settings as for FID. Mass spectra were recorded between 50 and 600 m/z in the electron ionization (EI) mode. Both 18:1n-9 and 18:1n-12 coelute and cannot be quantified separately so therefore will be referred to as “18:1” in text. The limit of quantitation was < 10 ng of FA. All biomass and FA contents were reported per dry weight (DW), unless otherwise specified.
Statistical analysis
All analyses were conducted in R 4.1.2 (R Core Team). Individual FA were grouped in saturated fatty acids (SFA), monounsaturated fatty acids (MUFA), and PUFA. Insect dry weight and all FA group comparisons between insects of aquatic, semi-aquatic, and terrestrial origin did not meet assumptions for normal data distribution (Shapiro-Wilk test). Therefore, Kruskal-Wallis (KW) Chi-squared tests (kruskal.test) were used. If KW tests revealed significant differences among insect groups, Dunn’s test for multiple comparisons of groups was conducted to check for differences between the three insect origins (Dinno and Dinno, 2017). Mass fraction values were converted to percentages prior to ordination. Percentage data were arcsine-square-root-transformed for normal distribution approximation and to achieve equal variances. Non-metric multidimensional scaling (NMDS; package vegan) was used to ordinate terrestrial and aquatic insects using rank-order dissimilarities (i.e., Bray-Curtis distances). Convergent solutions were not possible in two dimensions; therefore, data was ordinated in three dimensions but presented in two dimensions using the first two axis. Hierarchical clustering was conducted on average FA mass fractions of insect orders with more than three replicates (hclust; R package stats).
Analysis of similarity (ANOSIM, R package vegan; Oksanen et al., 2013) with 100,000 permutations was used to determine differences between the overall FA profile due to the difference in insect origins. The test statistic, R, is an indicator of similarity where groups can be classified as well separated (R > 0.75), separated but some overlapping (0.5 < R ≤ 0.75), separated but strongly overlapping (0.25 < R ≤ 0.5), and not separated (R < 0.25; Clarke et al., 2014). Similarity percentages (SIMPER; package vegan) with 1,000 permutations were used to quantify the contribution of individual FA to the overall Bray-Curtis dissimilarity between aquatic and terrestrial insects. The FA profile of semi-aquatic insects overlapped with that of both aquatic and terrestrial insects and therefore removed from the SIMPER analysis. Regression tree analysis was conducted using all aquatic and terrestrial insect orders and the 19 FA that were significant in SIMPER analysis and all PUFA of interest (i.e., ALA, LIN, ARA, EPA, and DHA). Explanatory variables were FA percentages of insect taxa. Each split (non-terminal nodes) was labeled with the FA percentage that determined the split, while the leaves (terminal nodes) were labeled with insect order (De’Ath and Fabricius, 2000).
NMDS and similarity percentages breakdown was conducted on aquatic and terrestrial insects sampled in each month (from April to October). Sufficient replicates of semi-aquatic insects were not possible for each month so semi-aquatic insects were removed from the analysis. The within-group monthly separation of aquatic and terrestrial insect FA profile was tested using ANOSIM. PERMANOVA pairwise comparisons (PPC; package vegan) of within-group FA profile differences in aquatic and terrestrial insects revealed little differences in adjacent months (Supplementary Table 3). Therefore, months were grouped into seasons: spring (April, May), summer (June, July, August), and autumn (September and October). SIMPER with 1,000 permutations were used to quantify the contribution of individual FA to the within-group Bray-Curtis dissimilarity between aquatic and terrestrial insects collected in spring, summer, and autumn. The physiologically highly relevant EPA contributed most to the dissimilarity. Therefore, the within-group proportion of EPA was compared between spring, summer, and autumn in aquatic and terrestrial insects, separately using KW Chi-squared tests. Following a significant KW test, Pairwise Wilcoxon Rank Sum comparisons (stats package) were used to test for differences among the three seasons.
Generalized linear models (glm function) were used to assess differences in ALA, LIN, ARA, EPA, and DHA content among the insect and a few other arthropod orders using the “Gaussian” distribution. Specifically, we used insect taxa as a predictor of the response variables: (1) ALA, (2) LIN, (3) ARA, (4) EPA, and (5) DHA. All models were based on a comparison to Psychodidae, semi-aquatic insects that served as a reference for both aquatic and terrestrial insects. All values are reported as means ± standard deviation.
Results
Fatty acid profile of aquatic and terrestrial insects
On average, terrestrial insects (3.5 ± 7.7 mg) weighed significantly more than aquatic (1.6 ± 4.4 mg) insects, which weighed significantly more than semi-aquatic insects (0.2 ± 0.2 mg; KW, χ2 = 52.1, p < 0.0001). Aquatic insects contained significantly more FA in total (64.4 ± 80.9 μg mg–1) than terrestrial (51.3 ± 48.7 μg mg–1) and semi-aquatic insects (43.8 ± 21.7 μg mg–1; KW, χ2 = 29.9, p < 0.0001). Both aquatic (18.5 ± 27.4 μg mg–1) and semi-aquatic insects (15.2 ± 9.9 μg mg–1) contained significantly more SFA than terrestrial insects (13.4 ± 14.7 μg mg–1; KW, χ2 = 54.2, p < 0.0001). No significant differences were observed in MUFA content between aquatic (19.4 ± 31.0 μg mg–1), semi-aquatic (15.0 ± 7.6 μg mg–1), and terrestrial insects (21.1 ± 33.6 μg mg–1; KW, χ2 = 3.0, p > 0.05). Insects of aquatic origins had a significantly higher total PUFA content (26.4 ± 29.6 μg mg–1) than semi-aquatic (13.5 ± 6.9 μg mg–1) and terrestrial insects (16.8 ± 11.8 μg mg–1; KW, χ2 = 172.4, p < 0.0001). Insects of aquatic origin also had significantly higher EPA + DHA contents (10.2 ± 13.8 μg mg–1) than semi-aquatic insects (2.4 ± 1.8 μg mg–1), which in turn had higher EPA + DHA contents than terrestrial insects (1.1 ± 1.5 μg mg–1; KW, χ2 = 514.7, p < 0.0001).
Terrestrial insects (9.8 ± 9.9 μg mg–1) contained more LIN than aquatic (7.1 ± 10.1 μg mg–1) and semi-aquatic insects (5.9 ± 4.7 μg mg–1; KW, χ2 = 37.8, p < 0.0001). In contrast, aquatic insects (4.7 ± 6.5 μg mg–1) contained significantly more ALA than terrestrial (4.1 ± 6.4 μg mg–1) and semi-aquatic insects (2.9 ± 2.5 μg mg–1; KW, χ2 = 56.9, p < 0.0001). The EPA content was significantly higher in aquatic (9.5 ± 11.3 μg mg–1) than in semi-aquatic (2.4 ± 1.8 μg mg–1) and terrestrial insects (1.1 ± 1.5 μg mg–1; KW, χ2 = 514.7, p < 0.0001). Aquatic insects (1.9 ± 3.5 μg mg–1) also had significantly higher ARA content than both semi-aquatic (1.1 ± 1.0 μg mg–1) and terrestrial insects (0.7 ± 0.9 μg mg–1; KW, χ2 = 190.8, p < 0.0001). The DHA content was also significantly higher in aquatic insects (0.8 ± 3.0 μg mg–1) than terrestrial insects (0.03 ± 0.3 μg mg–1; KW, χ2 = 89.1, p < 0.0001).
Multivariate comparisons of similarity revealed a significant and clear difference with some overlapping in FA profiles between aquatic, semi-aquatic, and terrestrial insects (three-group comparison, ANOSIM: R = 0.51, p < 0.0001; Figure 2A) as well as between aquatic and terrestrial insects (two-group comparison ANOSIM: R = 0.54, p < 0.0001). Hierarchal cluster analysis, using Bray-Curtis dissimilarity of mean FA mass fractions, produced three distinct clusters (Figure 2B). One cluster contains only terrestrial insect taxa: Coleoptera, Hymenoptera, Dermaptera, Mecoptera, Neuroptera, Psocoptera, Hemiptera, and Orthoptera. Another cluster contains only aquatic taxa: Chaoborus (order: Diptera), Megaloptera, Chironomidae (order: Diptera), Trichoptera, Ephemeroptera, Simuliidae (order: Diptera), and Plecoptera. A final mixed cluster contains the semi-aquatic Psychodidae, but also aquatic Diptera (Culicidae), and terrestrial insect taxa (i.e., Lepidoptera, Thysanoptera, and Diptera; Figure 2B). The main FA (i.e., FA that contributed > 5% to dissimilarity and revealed significant p-values) that collectively accounted for 70% of the dissimilarity between aquatic and terrestrial insects were (in descending order) EPA, 18:1, LIN, ALA, 18:1n-7, 16:1n-7, 18:4n-3 (stearidonic acid; SDA), ARA, and 14:0 (Table 1). Aquatic insects had higher abundance of EPA, 18:1n-7, SDA, ARA, and 14:0, whereas terrestrial insects had significantly higher abundance of 18:1 and LIN (Table 1). Although DHA was not a significant driver in the global dissimilarity in this dataset, DHA (≥ 1.8%) separated Chaoborus (order: Diptera) from all other terrestrial and aquatic taxa (Figure 3). The majority of aquatic insect taxa (i.e., Chironomidae, Trichoptera, and Ephemeroptera) were separated from terrestrial insect taxa by EPA ≥ 11.1% (Figure 3). Two additional clusters are formed with aquatic, semi-aquatic, and terrestrial insect taxa separated by ARA. Aquatic Hymenoptera, semi-aquatic Psychodidae, and terrestrial Lepidoptera, Hemiptera, and Coleoptera were categorized with ARA < 0.25%. Aquatic Trichoptera and Chironomidae, semi-aquatic Psychodidae, and terrestrial Diptera, Hymenoptera, and Coleoptera were classified with ARA > 0.25% (Figure 3).
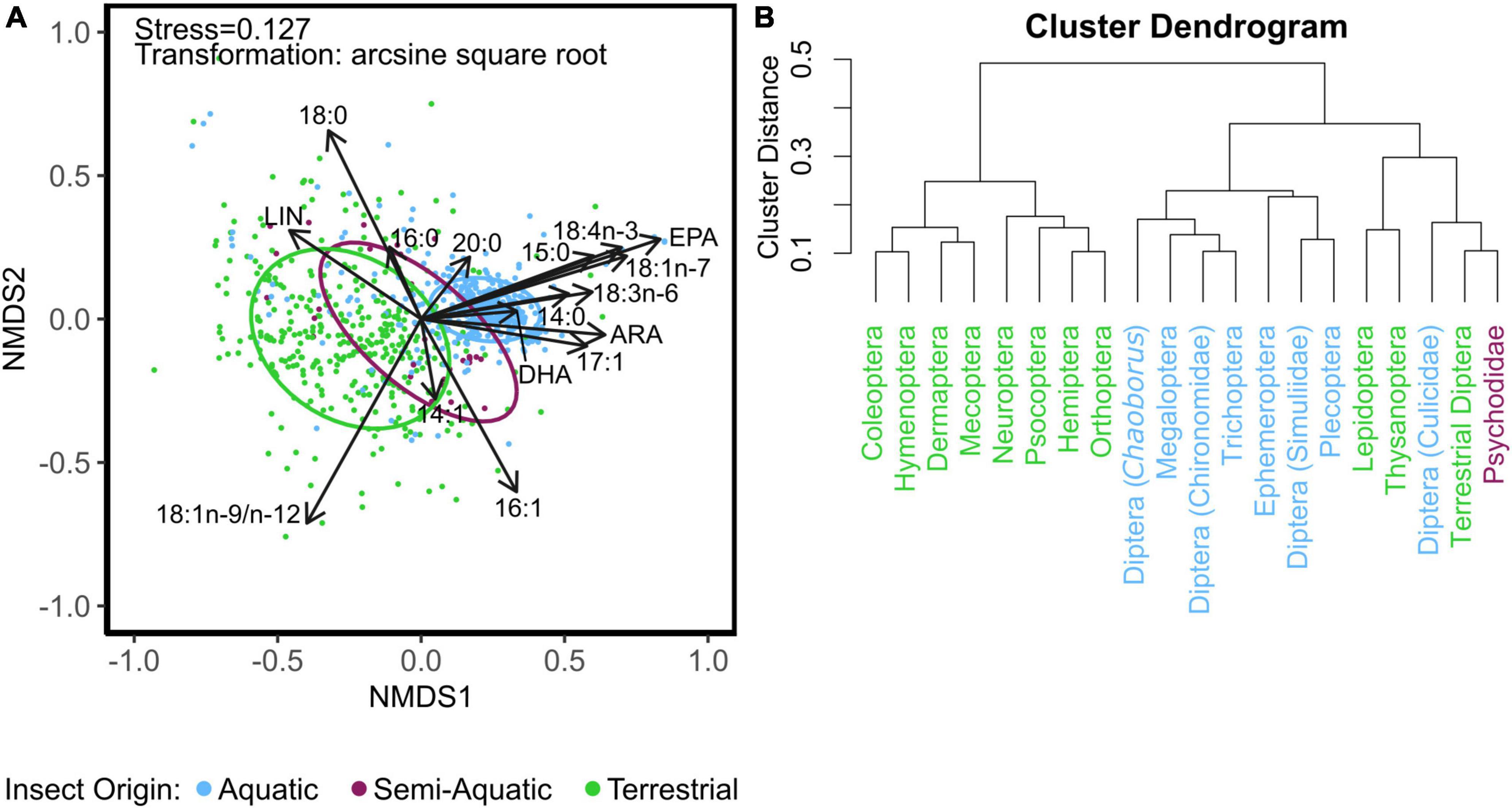
Figure 2. (A) Non-metric multidimensional scaling (NMDS) of arcsine-square-root-transformed fatty acid fractions (% of total fatty acids) of aquatic, semi-aquatic, and terrestrial insects. Vectors represent fatty acids that had a significant regression value (p < 0.001) and a vector length of 0.33 or greater. Ellipses represent 75% confidence intervals. (B) Hierarchal cluster analysis of mean fatty acid content (mg mg–1 DW). Fatty abbreviations: linoleic acid (LIN; 18:2n-6), arachidonic acid (ARA; 20:4n-6), eicosapentaenoic acid (EPA; 20:5n-3), and docosahexaenoic acid (DHA; 22:6n-3).
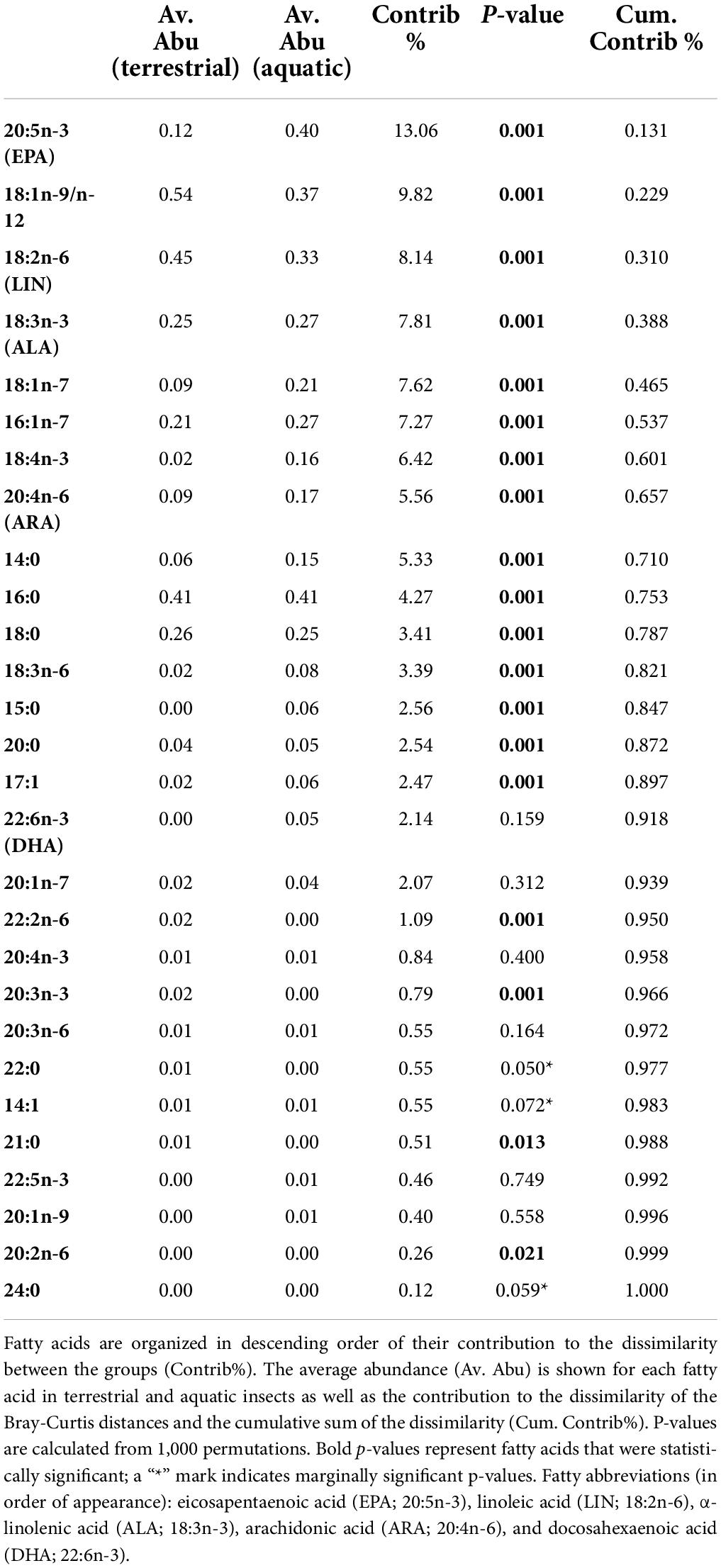
Table 1. Similarity percentages (SIMPER) analysis of fatty acid profiles (%) of aquatic and terrestrial insects.
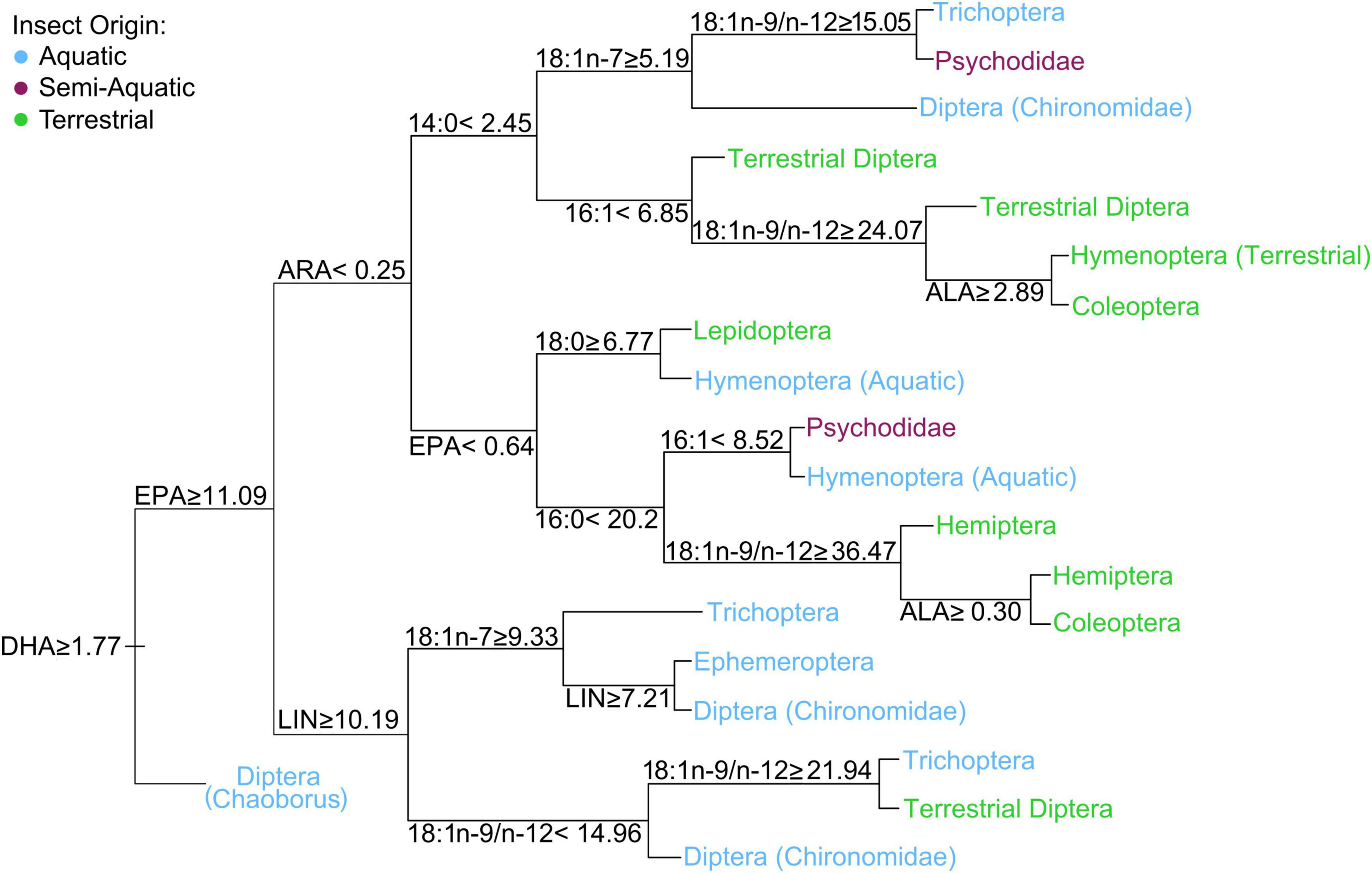
Figure 3. Regression tree analysis constructed with individual fatty acids (% of total FA) that were significant in the similarity percentages (SIMPER) analysis. All insect orders were considered, however, only those separated by the selected FA are shown. The condition set at each node is true for the divide going downwards and the opposite condition is true for the divide going upwards. Fatty abbreviations: linoleic acid (LIN; 18:2n-6), α-linolenic acid (ALA; 18:3n-3), arachidonic acid (ARA; 20:4n-6), eicosapentaenoic acid (EPA; 20:5n-3), and docosahexaenoic acid (DHA; 22:6n-3).
Seasonality of the aquatic/terrestrial insect fatty acid profile dissimilarity
Multivariate comparisons of similarity for each sampling month revealed a significant difference in FA profiles between aquatic and terrestrial insects (ANOSIM, p < 0.0001; Figures 4A–G). However, the similarity (i.e., ANOSIM) differed throughout the year (Figure 4H). April, May, and July had similar ANOSIM values compared to the ANOSIM value of the entire dataset (Figure 4H). June and August had higher ANOSIM value suggesting a greater dissimilarity between aquatic and terrestrial insect FA profiles, whereas September and October had lower ANOSIM values, suggesting greater similarity between aquatic and terrestrial insect FA profiles (Figure 4H). In all months, EPA was a significant driver of the FA dichotomy between aquatic and terrestrial taxa, with the highest contribution to the dissimilarity (SIMPER, p < 0.001; Supplementary Table 2).
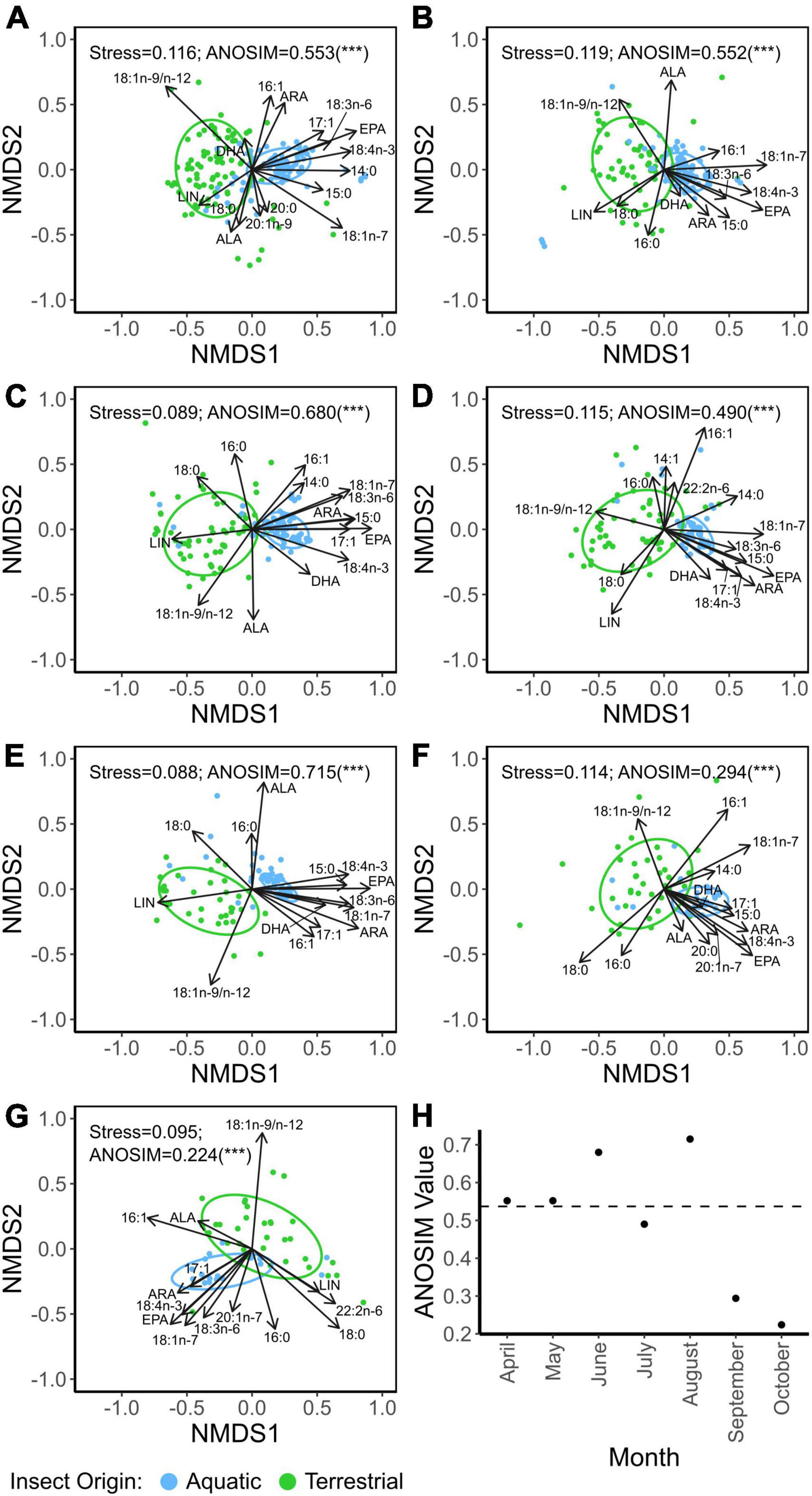
Figure 4. Non-metric multidimensional scaling (NMDS) of arcsine-square-root-transformed fatty acid fractions (% of total fatty acids) of aquatic and terrestrial insects of April (A), May (B), June (C), July (D), August (E), September (F), and October (G). Asterisk represent significant ANOSIM (Analysis of Similarities) comparisons; “***” represent p < 0.0001. (H) ANOSIM value representing the similarity between aquatic and terrestrial insects (i.e., higher value means greater separation between groups) for each sampling month. Dashed line represents the ANOSIM value for the entire dataset. Fatty abbreviations: linoleic acid (LIN; 18:2n-6), α-linolenic acid (ALA; 18:3n-3), arachidonic acid (ARA; 20:4n-6), eicosapentaenoic acid (EPA; 20:5n-3), and docosahexaenoic acid (DHA; 22:6n-3).
FA profiles of aquatic (ANOSIM: R = 0.121, p < 0.001; Supplementary Figure 2A) and terrestrial insects (ANOSIM: R = 0.047, p < 0.001; Supplementary Figure 2B) were significantly separated based on the season, albeit less pronounced than the separation between aquatic and terrestrial insect FA. Season had a statistically significant effect on the FA profile of aquatic insects (PPC, p < 0.01). The FA profiles of terrestrial insects were not significantly different between summer and autumn but were significantly different between spring and summer (PPC, p < 0.01) and between spring and autumn (PPC, p < 0.05). EPA was the significant main driver of within-group differences between the comparison of spring/autumn and summer/autumn aquatic insects (SIMPER; Supplementary Table 4). LIN, ARA, and DHA were significant drivers of differences between spring and summer aquatic insects (SIMPER; Supplementary Table 4). EPA proportion significantly differed in aquatic insects between seasons (KW, χ2 = 35.2, p = 0.0001), i.e., the proportion of EPA was significantly higher in spring and summer than in autumn, whereas the proportion of EPA did not differ between spring and summer (Supplementary Figure 2C). No significant seasonal differences were observed in terrestrial insects EPA proportions (KW, χ2 = 4.95, p > 0.05; Supplementary Figure 2C).
Fatty acid profiles of different insect taxa
Multivariate comparisons of similarity also revealed a significant difference in FA profiles between insect taxa (ANOSIM: R = 0.48, p < 0.0001). Dermaptera, Simuliidae (order: Diptera), Hymenoptera, Lepidoptera, Thysanoptera had significantly higher ALA content; Dermaptera Hemiptera and Trichoptera had marginally significant higher ALA content when compared to the semi-aquatic reference (i.e., Psychodidae; Figure 5A and Supplementary Table 5). Dermaptera, Hymenoptera, Orthoptera had significantly higher LIN content; Hemiptera and Neuroptera had marginally significant higher LIN content when compared to Psychodidae (Figure 5C and Supplementary Table 6). Chaoborus (Diptera) and Ephemeroptera had significantly higher EPA content; Chironomidae (order: Diptera) and Trichoptera had marginally significant higher EPA content than Psychodidae (Figure 5B and Supplementary Table 7). Chaoborus (order: Diptera) and Collembola had significantly higher ARA content than Psychodidae (Figure 5D and Supplementary Table 8). DHA was significantly higher in Chaoborus (order: Diptera; Supplementary Table 9) than in all other taxa, where only traces of DHA were detected if at all (Supplementary Figure 3). A complete table of all orders and their respective fatty acid profiles is available in Supplementary Table 1.
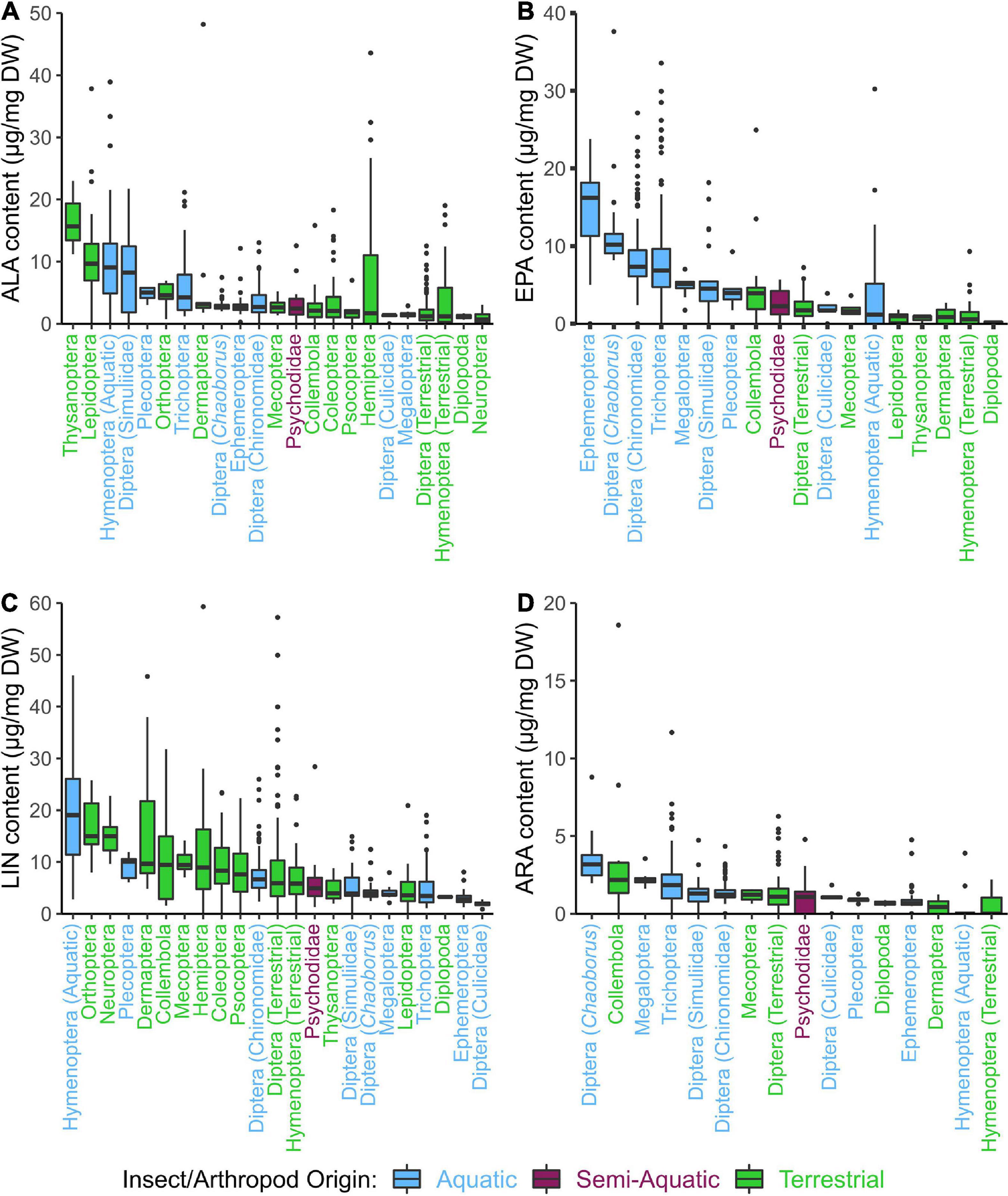
Figure 5. Contents (μg mg–1 DW) of selected PUFA of aquatic, semi-aquatic, and terrestrial arthropod orders: (A) α-linolenic acid (ALA; 18:3n-3), (B) eicosapentaenoic acid (EPA; 20:5n-3), (C) linoleic acid (LIN; 18:2n-6), (D) arachidonic acid (ARA; 20:4n-6). The box represents the first quartile to the third quartile with a line representing the median value, and the whiskers represent the minimum and maximum value. Only taxonomic groups with a minimum of 3 samples for boxplot statistics were presented.
Discussion
Our data show a clear dichotomy between the FA profiles of aquatic and terrestrial insects with the separation being mainly driven by higher proportions of C20-PUFA (i.e., EPA and ARA) in aquatic insects and higher proportions of C18-PUFA (i.e., LIN) in terrestrial insects, which is in line with our predictions. Aquatic insect taxa separated from terrestrial insect taxa by higher proportions of mainly EPA and ARA, while Chaoborus separated from all insect taxa due to the presence of DHA. Although the separation in aquatic and terrestrial insects was evident, the strength of this separation changed over the growing season. The dissimilarity between aquatic and terrestrial insect FA profiles was greater in spring and summer than in autumn, highlighting the importance of aquatic insects as a source of LC-PUFA when most riparian predators have their young.
Differences in FA profiles between emerging aquatic and terrestrial insects have been demonstrated previously (e.g., Hixson et al., 2015). Here, we explored this dichotomy in more detail, considering multiple aquatic and terrestrial insect orders as well as seasonal changes in the strength of FA separation between aquatic and terrestrial insects in a lake ecosystem. Emergent aquatic insects had higher mean PUFA content, specifically ALA, EPA, ARA, and DHA, than terrestrial insects, which is in line with previous studies on both river (Twining et al., 2019; Kowarik et al., 2021) and lake ecosystems (Mathieu-Resuge et al., 2021b; Twining et al., 2021b). Twining et al. (2019) found higher ALA proportions in terrestrial Hymenoptera and Lepidoptera, but higher EPA proportions in aquatic insect taxa near streams in the Eastern United States during the bird breeding season in May and June. In contrast, Twining et al. (2021b) found higher ALA, as well as EPA, ARA, and DHA contents in aquatic insects, but no difference in LIN content between aquatic and terrestrial insects in April and May around Lake Mindelsee in southern Germany (same lake as in current study) during the Blue Tit (Cyanistes caeruleus) breeding season. In the current study, considering the entire growing season, we found that terrestrial insects contain, on average, more LIN than aquatic insects. This significant LIN comparison may be a product of the additional sampling from June to October, including Orthoptera as well as a higher proportion of Dermaptera and Neuroptera to the analysis, all of which having significantly higher LIN content. Although Mathieu-Resuge et al. (2021b) found no differences in the total PUFA content between aquatic and terrestrial insects around Lake Lunz (Austria), similar to the current study, ALA and EPA were higher in aquatic insects while LIN was higher in terrestrial insects.
Although comparisons of mean individual PUFA content and ratios between aquatic and terrestrial insects provide insight into the dichotomy between aquatic and terrestrial insect, they do not quantify the dissimilarity between aquatic and terrestrial insect FA. To our knowledge, only Hixson et al. (2015) attempted to quantify the dissimilarity between aquatic and terrestrial insect FA profiles so far. In their global meta-analysis, the FA profiles of aquatic and terrestrial insects were different but overlapped strongly (ANOSIM R = 0.34; see Hixson et al., 2015). In our dataset, aquatic and terrestrial insect FA profiles were clearly separated with only some overlapping (ANOSIM R = 0.54). The higher dissimilarity observed in the current study may be due to sampling in a single system with replicates for each insect taxa and thus reduced variability associated with comparing insect FA profiles collected from various climate zones. Sampling in one ecosystem also revealed temporal differences in the dissimilarity between aquatic and terrestrial insect FA profiles, potentially impacting riparian predators that can feed on both aquatic and terrestrial prey. Consumers must either take up LC-PUFA from their prey or biosynthesize them from dietary C18-PUFA precursors in order to satisfy their physiological demands (Twining et al., 2021b). The high dissimilarity between aquatic and terrestrial insect FA profiles observed in spring and summer (e.g., May to August) emphasizes the importance of aquatic insects in providing LC-PUFA to riparian predators during the breeding season. Twining et al. (2021b) showed that spiders collected in April and May along the shore of Lake Mindelsee (∼10 m from shore) had higher EPA content than spiders collected 500 and 1,000 m away from the shore. Mixing models revealed that emergent aquatic insects comprised 74% of riparian spider diet, whereas spiders at 500 and 1,000 m distance from Lake Mindelsee hardly consumed any aquatic insects (Twining et al., 2021b). Along a river ecosystem, the diet of web-building spiders has been demonstrated to contain higher proportions of aquatic-derived material in spring and summer than in autumn, based on analysis of similarity and mixing models (Kowarik et al., 2021). The temporal variability in the dissimilarity between aquatic and terrestrial insects FA profiles may be linked with the strong temporal variation in their respective biomass and community composition (Martin-Creuzburg et al., 2017; Nsor et al., 2020; Mathieu-Resuge et al., 2021a). Lower biodiversity of both aquatic and terrestrial insects in autumn may increase the similarity between FA profiles. The reliance of riparian predators on aquatic-derived LC-PUFA may depend on their capability to biosynthesize LC-PUFA (Twining et al., 2021b). However, bird chicks raised on a high LC-PUFA diet had higher growth rates and improved overall body condition (Twining et al., 2016, 2019), highlighting the importance of emergent aquatic insects early in the season for providing PUFA to riparian predators.
As expected, based on the higher EPA content of aquatic insects, EPA contributed most to the dissimilarity between aquatic and terrestrial insects and was the major driver for aquatic insect grouping. In addition to EPA, aquatic insects were characterized by higher abundances of 18:1n-7, SDA, ARA, and 14:0, while terrestrial insects were mainly characterized by higher abundances of 18:1 and LIN. LIN can be found in high proportions in many terrestrial plants (Hixson et al., 2015) and therefore, the high LIN content observed in terrestrial insects is most likely related to feeding on terrestrial plant material. In consumers, LIN plays a crucial role in maintaining vital membrane properties and as precursor for ARA and thus eicosanoids (Stanley and Kim, 2019). A high body LIN content may be linked with the high proportion of LIN in the insect fat body, where LIN is stored as an important energy source, especially during reproduction (Thomas, 1974; Sayah et al., 1997). Why the LIN content tends to be higher in terrestrial than aquatic insects remains unclear. The higher PUFA (i.e., ALA, EPA, and ARA) content of emergent aquatic insects appears to be related to feeding on PUFA-containing algae during the larval stage. An ecosystem-based difference in insect PUFA content is likely transferred from this difference in PUFA availability at the basal food web. FA profiles of insect larvae closely mirror the SDA (Kowarik et al., 2021) and EPA (Guo et al., 2018) content of periphyton, suggesting that these PUFA are selectively retained in aquatic consumers.
Grouping insect taxa based on mean FA profiles produced three distinct hierarchical clusters. The two clusters containing only aquatic or terrestrial taxa, respectively, reflect the general dissimilarity between aquatic and terrestrial insects in FA profiles. A third cluster, containing Lepidoptera, Thysanoptera, Culicidae (order: Diptera), terrestrial Diptera, and Psychodidae, separates the insect taxa causing the FA profile similarities between the two ecosystems, i.e., the observed overlap of aquatic and terrestrial FA profiles. Insect larvae that emerge from wet soil along the lake shore (i.e., Psychodidae and Culicidae) may still have consumed LC-PUFA containing algae (Sushchik et al., 2013). However, the separation of Psychodidae in our cluster analysis reflects the dissimilarity in FA availability between wet soil and lake algal communities. PUFA that contribute to the overall dissimilarity between aquatic and terrestrial insects can also be used to separate insect orders. DHA, for instance, separates Chaoborus (order: Diptera) from all other insect taxa. DHA has been reported already to occur in Chaoborus larvae (Goedkoop et al., 2000; Lau et al., 2014) as well as in emergent adults (Martin-Creuzburg et al., 2017; Twining et al., 2021b). Chaoborus larvae are predatory and may prey on DHA-rich zooplankton, such as copepods (Persson and Vrede, 2006; Smyntek et al., 2008). The capability of Chaoborus larvae to biosynthesize LC-PUFA is currently unknown. However, other aquatic Diptera (i.e., Chironomus) have been shown to elongate and desaturate dietary C18-PUFA precursors to EPA and ARA in the absence of dietary C20-PUFA (Strandberg et al., 2021), indicating that at least some aquatic insects have the capability to biosynthesize LC-PUFA to meet their physiological needs. Collembola also contained moderate amounts of C20-PUFA (i.e., EPA and ARA). As an exception among terrestrial invertebrates, Collembola contain and synthesize C20-PUFA (Chamberlain and Black, 2005; Chamberlain et al., 2005). For example, Collembola collected from a woodland had ARA values between 1.2 and 8.6% and EPA values between 0.9 and 12.7% of total FA, depending on the species (Chamberlain and Black, 2005). Collembola from the current study had ARA and EPA contents of 4.6 ± 2.9 and 7.9 ± 4.7%, respectively. Our study provides detailed FA profiles for multiple insect taxa as well as a few other non-insect arthropod taxa collected with our traps (see Supplementary Table 1).
The between-group and within-group dissimilarity of aquatic and terrestrial insects changing throughout the growing season clearly suggests that riparian predators have varying access to aquatic-derived LC-PUFA throughout the year. The overall dichotomy between aquatic and terrestrial insect FA profiles exists despite seasonal changes in taxonomic diversity within aquatic and terrestrial insect communities. Seasonal differences in FA profiles within aquatic insects can be explained by higher EPA proportions in spring and summer insects. Higher emergence of EPA-rich taxa such as Ephemeroptera, Chaoborus, Trichoptera during spring and summer (Martin-Creuzburg et al., 2017) explains the within-group variation in aquatic insect FA profiles. Emergent insect biomass and biodiversity is also typically higher in spring and summer (Martin-Creuzburg et al., 2017). Combined with evidence of greater dissimilarity of FA profile between aquatic and terrestrial insects in spring and summer, this highlights the importance of early aquatic insect emergence for riparian predators when they have their young.
In addition, climate change is predicted to create a phenological mismatch where riparian predators have their young later than the peak aquatic insect emergence, reducing the availability of aquatic LC-PUFA for riparian predators and their young (Shipley et al., 2022). The potential consequences of this phenological mismatch on riparian predators would heavily depend on their internal bioconversion capability. For example, Tree Swallows (Tachycineta bicolor) chicks can modestly convert C18-PUFA precursors (i.e., ALA) to C20-PUFA (i.e., EPA and DHA; Twining et al., 2018a). However, a reduction of aquatic insect prey would also reduce dietary ALA, besides C20-PUFA (i.e., ARA, EPA, and DHA). Direct impacts of lower dietary PUFA intake may include reduced growth, immune function, performance, and reproductive output (Twining et al., 2016, 2018b; Fritz et al., 2017), while additional performance costs may arise from the energetic cost of biosynthesizing LC-PUFA from dietary precursors. On the contrary, Blue Tit chicks seem to readily convert dietary ALA into DHA, even in riparian habitats with high availability of aquatic insects (Twining et al., 2021c). Our field study highlights the importance of emergent aquatic insects as critical cross-ecosystem conveyors of LC-PUFA to terrestrial riparian predators. Future studies aiming to quantify the LC-PUFA biosynthesis capability of riparian predators may help to understand how changes in aquatic insect availability may affect the transfer of aquatic nutrients to terrestrial food webs.
Data availability statement
The original contributions presented in this study are included in the article/Supplementary material, further inquiries can be directed to the corresponding author.
Ethics statement
Written informed consent was obtained from the individual(s) for the publication of any potentially identifiable images or data included in this article.
Author contributions
TP and DM-C designed the study. DM-C and MK acquired funding. TP, AK, and DM-C conducted fieldwork and conducted laboratory analyses. TP, AK, and MM-R conducted data analysis and visualization. TP led writing of the manuscript. All authors contributed to the manuscript drafts and gave final approval for publication.
Funding
This study was funded by the German Research Foundation (DFG; MA 5005/8-1) and Austrian Science Fund (FWF; I 3855-B25) within the framework of the DACH collaboration (project “AquaTerr”). CT was supported by a postdoctoral fellowship from the Alexander von Humboldt Foundation.
Acknowledgments
We thank Kai-Steffen Frank and Verena Medinger (BUND) for their great support and access to sampling sites. We would also like to thank Martin Wolf for help designing and building traps and to Petra Merkel for help with the FA analysis. We further thank Marie Huchler, Anna-Caterina Eberhard, Hannah Bodmer, Johanna Roller, Luca Kehrt, and Frauke Schmidt for their field and/or laboratory assistance. We would also like to thank the handling editor, Jeff Wesner, and the three reviewers for their thoughtful comments and efforts towards improving our manuscript.
Conflict of interest
The authors declare that the research was conducted in the absence of any commercial or financial relationships that could be construed as a potential conflict of interest.
The handling editor declared a past co-authorship with one of the author, CT.
Publisher’s note
All claims expressed in this article are solely those of the authors and do not necessarily represent those of their affiliated organizations, or those of the publisher, the editors and the reviewers. Any product that may be evaluated in this article, or claim that may be made by its manufacturer, is not guaranteed or endorsed by the publisher.
Supplementary material
The Supplementary Material for this article can be found online at: https://www.frontiersin.org/articles/10.3389/fevo.2022.952292/full#supplementary-material
References
Bartels, P., Cucherousset, J., Steger, K., Eklov, P., Tranvik, L. J., and Hillebrand, H. (2012). Reciprocal subsidies between freshwater and terrestrial ecosystems structure consumer resource dynamics. Ecology 93, 1173–1182. doi: 10.1890/11-1210.1
Baxter, C. V., Fausch, K. D., and Saunders, W. C. (2005). Tangled webs: reciprocal flows of invertebrate prey link streams and riparian zones. Freshw. Biol. 50, 201–220. doi: 10.1111/j.1365-2427.2004.01328.x
Brenna, J. T., and Carlson, S. E. (2014). Docosahexaenoic acid and human brain development: evidence that adietary supply is needed for optimal development. J. Hum. Evol. 77, 99–106. doi: 10.1016/j.jhevol.2014.02.017
Brzeziński, T., and von Elert, E. (2015). Predator evasion in zooplankton is suppressed by polyunsaturated fatty acid limitation. Oecologia 179, 687–697. doi: 10.1007/s00442-015-3405-4
Burdon, F. J., and Harding, J. S. (2008). The linkage between riparian predators and aquatic insects across a stream-resource spectrum. Freshw. Biol. 53, 330–346. doi: 10.1111/j.1365-2427.2007.01897.x
Chamberlain, P. M., and Black, H. I. J. (2005). Fatty acid compositions of Collembola: unusually high proportions of C20 polyunsaturated fatty acids in a terrestrial invertebrate. Comp. Biochem. Physiol. Part B Biochem. Mol. Biol. 140, 299–307. doi: 10.1016/j.cbpc.2004.10.016
Chamberlain, P. M., Bull, I. D., Black, H. I. J., Ineson, P., and Evershed, R. P. (2005). Fatty acid composition and change in Collembola fed differing diets: identification of trophic biomarkers. Soil Biol. Biochem. 37, 1608–1624.
Clarke, K. R., Gorley, R. N., Somerfield, P. J., and Warwick, R. M. (2014). Change in marine communities: an approach to statistical analysis and interpretation. Plymouth: Primer-E.
De’Ath, G., and Fabricius, K. E. (2000). Classification and regression trees: a powerful yet simple technique for ecological data analysis. Ecology 81, 3178–3192.
Dinno, A., and Dinno, M. A. (2017). Package ‘dunn. test.’. Available online at: https://cran.r-project.org/web/packages/dunn.test/dunn.test.pdf (accessed January 28, 2022).
Fritz, K. A., Kirschman, L. J., McCay, S. D., Trushenski, J. T., Warne, R. W., and Whiles, M. R. (2017). Subsidies of essential nutrients from aquatic environments correlate with immune function in terrestrial consumers. Freshw. Sci. 36, 893–900. doi: 10.1086/694451
Gladyshev, M. I., Sushchik, N. N., and Makhutova, O. N. (2013). Production of EPA and DHA in aquatic ecosystems and their transfer to the land. Prostaglandins Other Lipid Mediat. 107, 117–126. doi: 10.1016/j.prostaglandins.2013.03.002
Goedkoop, W., Sonesten, L., Ahlgren, G., and Boberg, M. (2000). Fatty acids in profundal benthic invertebrates and their major food resources in Lake Erken, Sweden: seasonal variation and trophic indications. Can. J. Fish. Aquat. Sci. 57, 2267–2279.
Guo, F., Bunn, S. E., Brett, M. T., Fry, B., Hager, H., Ouyang, X., et al. (2018). Feeding strategies for the acquisition of high−quality food sources in stream macroinvertebrates: collecting, integrating, and mixed feeding. Limnol. Oceanogr. 63, 1964–1978. doi: 10.1002/lno.10818
Harayama, T., and Shimizu, T. (2020). Roles of polyunsaturated fatty acids, from mediators to membranes. J. Lipid Res. 61, 1150–1160. doi: 10.1194/JLR.R120000800
Harwood, J. L. (1996). Recent advances in the biosynthesis of plant fatty acids. Biochim. Biophys. 1301, 7–56. doi: 10.1016/0005-2760(95)00242-1
Hixson, S. M., Sharma, B., Kainz, M. J., Wacker, A., and Arts, M. T. (2015). Production, distribution, and abundance of long-chain omega-3 polyunsaturated fatty acids: a fundamental dichotomy between freshwater and terrestrial ecosystems. Environ. Rev. 23, 414–424.
Isanta Navarro, J., Fromherz, M., Dietz, M., Zeis, B., Schwarzenberger, A., and Martin-Creuzburg, D. (2019). Dietary polyunsaturated fatty acid supply improves Daphnia performance at fluctuating temperatures, simulating diel vertical migration. Freshw. Biol. 64, 1859–1866. doi: 10.1111/fwb.13377
Kato, C., Iwata, T., Nakano, S., and Kishi, D. (2003). Dynamics of aquatic insect flux affects distribution of riparian web-building spiders. Oikos 103, 113–120. doi: 10.1034/j.1600-0706.2003.12477.x
Kautza, A., Mažeika, S., and Sullivan, P. (2016). The energetic contributions of aquatic primary producers to terrestrial food webs in a mid-size river system. Ecology 97, 694–705. doi: 10.1890/15-1095
Kowarik, C., Martin-Creuzburg, D., and Robinson, C. T. (2021). Cross-ecosystem linkages: transfer of polyunsaturated fatty acids from streams to riparian spiders via emergent insects. Front. Ecol. Evol. 9:707570. doi: 10.3389/fevo.2021.707570
Lam, M., Martin-Creuzburg, D., Rothhaupt, K. O., Safi, K., Yohannes, E., and Salvarina, I. (2013). Tracking diet preferences of bats using stable isotope and fatty acid signatures of faeces. PLoS One 8:e83452. doi: 10.1371/journal.pone.0083452
Lau, D., Sundh, I., Vrede, T., Pickova, J., and Goedkoop, W. (2014). Autochthonous resources are the main driver of consumer production in dystrophic boreal lakes. Ecology 95, 1506–1519. doi: 10.1890/13-1141.1
Maillet, D., and Weber, J. M. (2006). Performance-enhancing role of dietary fatty acids in a long-distance migrant shorebird: the semipalmated sandpiper. J. Exp. Biol. 209, 2686–2695. doi: 10.1242/jeb.02299
Martin-Creuzburg, D., Kowarik, C., and Straile, D. (2017). Cross-ecosystem fluxes: export of polyunsaturated fatty acids from aquatic to terrestrial ecosystems via emerging insects. Sci. Total Environ. 577, 174–182. doi: 10.1016/j.scitotenv.2016.10.156
Martin-Creuzburg, D., Wacker, A., and Basen, T. (2010). Interactions between limiting nutrients: consequences for somatic and population growth of Daphnia magna. Limnol. Oceanogr. 55, 2597–2607. doi: 10.4319/lo.2010.55.6.2597
Mathieu-Resuge, M., Pilecky, M., Twining, C. W., Martin-Creuzburg, D., Parmar, T. P., Vitecek, S., et al. (2021b). Dietary availability determines metabolic conversion of long-chain polyunsaturated fatty acids in spiders: a dual compound-specific stable isotope approach. Oikos 2022, 1–12. doi: 10.1111/oik.08513
Mathieu-Resuge, M., Martin-Creuzburg, D., Twining, C. W., Parmar, T. P., Hager, H. H., and Kainz, M. J. (2021a). Taxonomic composition and lake bathymetry influence fatty acid export via emergent insects. Freshw. Biol. 66, 2199–2209. doi: 10.1111/fwb.13819
Nakano, S., and Murakami, M. (2001). Reciprocal subsidies?: dynamic interdependence. PNAS 98, 166–170. doi: 10.1073/pnas.98.1.166
Nsor, C. A., Oppong, S. K., Danquah, E., Ochem, M., and Antobre, O. O. (2020). Spatiotemporal dynamics of terrestrial invertebrate assemblages in the riparian zone of the Wewe river, Ashanti region, Ghana. Open Life Sci. 15, 331–345. doi: 10.1515/biol-2020-0037
Oksanen, J., Blanchet, F. G., Kindt, R., Legendre, P., Minchin, P. R., O’hara, R. B., et al. (2013). Package ‘vegan.’. Community Ecology Package. Vienna: R Core Team.
Paetzold, A., Schubert, C. J., and Tockner, K. (2005). Aquatic terrestrial linkages along a braided-river: riparian arthropods feeding on aquatic insects. Ecosystems 8, 748–759. doi: 10.1007/s10021-005-0004-y
Persson, J., and Vrede, T. (2006). Polyunsaturated fatty acids in zooplankton: variation due to taxonomy and trophic position. Freshw. Biol. 51, 887–900.
Polis, G. A., and Hurd, S. D. (1996). Linking marine and terrestrial food webs: allochthonous input from the ocean supports high secondary productivity on small islands and coastal land communities. Am. Nat. 147, 396–423. doi: 10.1086/285858
Richardson, J. S., and Sato, T. (2015). Resource subsidy flows across freshwater-terrestrial boundaries and influence on processes linking adjacent ecosystems. Ecohydrology 8, 406–415. doi: 10.1002/eco.1488
Sanzone, D. M., Meyer, J. L., Marti, E., Gardiner, E. P., Tank, J. L., and Grimm, N. B. (2003). Carbon and nitrogen transfer from a desert stream to riparian predators. Oecologia 134, 238–250. doi: 10.1007/s00442-002-1113-3
Sayah, F., Karlinsky, A., and Breuzet, M. (1997). Lipid and fatty acid composition of the fat body during the female reproductive cycle of Labidura riparia (Insecta Dermaptera). J. Comp. Physiol. B 167, 502–507.
Shipley, J. R., Twining, C. W., Mathieu-Resuge, M., Parmar, T. P., Kainz, M., Martin-Creuzburg, D., et al. (2022). Climate change shifts the timing of nutritional flux from aquatic insects. Curr. Biol. 1–8. doi: 10.1016/j.cub.2022.01.057
Smyntek, P. M., Teece, M. A., Schulz, K. L., and Storch, A. J. (2008). Taxonomic differences in the essential fatty acid composition of groups of freshwater zooplankton relate to reproductive demands and generation time. Freshw. Biol. 53, 1768–1782. doi: 10.1111/j.1365-2427.2008.02001.x
Stanley, D., and Kim, Y. (2019). Prostaglandins and other eicosanoids in insects: biosynthesis and biological actions. Front. Physiol. 10:1927. doi: 10.3389/fphys.2018.01927
Strandberg, U., Ilo, T., Akkanen, J., and Kankaala, P. (2021). Warming decreases bioconversion of polyunsaturated fatty acids in chironomid larvae maintained on cyanobacterium microcystis. Biomolecules 11:1326. doi: 10.3390/biom11091326
Sushchik, N. N., Yurchenko, Y. A., Gladyshev, M. I., Belevich, O. E., Kalachova, G. S., and Kolmakova, A. A. (2013). Comparison of fatty acid contents and composition in major lipid classes of larvae and adults of mosquitoes (Diptera: Culicidae) from a steppe region. Insect Sci. 20, 585–600. doi: 10.1111/j.1744-7917.2012.01582.x
Taipale, S., Strandberg, U., Peltomaa, E., Galloway, A. W. E., Ojala, A., and Brett, M. T. (2013). Fatty acid composition as biomarkers of freshwater microalgae: analysis of 37 strains of microalgae in 22 genera and in seven classes. Aquat. Microb. Ecol. 71, 165–178. doi: 10.3354/ame01671
Tanentzap, A. J., Kielstra, B. W., Wilkinson, G. M., Berggren, M., Craig, N., Del Giorgio, P. A., et al. (2017). Terrestrial support of lake food webs: synthesis reveals controls over cross-ecosystem resource use. Sci. Adv. 3:e1601765. doi: 10.1126/sciadv.1601765
Thomas, K. K. (1974). Lipid composition of the fat body and haemolymph and its relation to lipid release in Oncopeltus fasciatus. J. Insect Physiol. 20, 845–858. doi: 10.1016/0022-1910(74)90174-7
Twining, C. W., Bernhardt, J. R., Derry, A. M., Hudson, C. M., Ishikawa, A., Kabeya, N., et al. (2021a). The evolutionary ecology of fatty-acid variation: implications for consumer adaptation and diversification. Ecol. Lett. 24, 1709–1731. doi: 10.1111/ele.13771
Twining, C. W., Parmar, T. P., Mathieu-Resuge, M., Kainz, M. J., Shipley, J. R., and Martin-Creuzburg, D. (2021b). Use of fatty acids from aquatic prey varies with foraging strategy. Front. Ecol. Evol. 9:735350. doi: 10.3389/fevo.2021.735350
Twining, C. W., Razavi, N. R., Brenna, J. T., Dzielski, S. A., Gonzalez, S. T., Lawrence, P., et al. (2021c). Emergent freshwater insects serve as subsidies of methylmercury and beneficial fatty acids for riparian predators across an agricultural gradient. Environ. Sci. Technol. 55, 5868–5877. doi: 10.1021/acs.est.0c07683
Twining, C. W., Brenna, J. T., Lawrence, P., Shipley, J. R., Tollefson, T. N., and Winkler, D. W. (2016). Omega-3 long-chain polyunsaturated fatty acids support aerial insectivore performance more than food quantity. Proc. Natl. Acad. Sci. U.S.A. 113, 10920–10925.
Twining, C. W., Brenna, J. T., Lawrence, P., Winkler, D. W., Flecker, A. S., and Hairston, N. G. (2019). Aquatic and terrestrial resources are not nutritionally reciprocal for consumers. Funct. Ecol. 33, 2042–2052. doi: 10.1111/1365-2435.13401
Twining, C. W., Lawrence, P., Winkler, D. W., Flecker, A. S., and Brenna, J. T. (2018a). Conversion efficiency of α-linolenic acid to omega-3 highly unsaturated fatty acids in aerial insectivore chicks. J. Exp. Biol. 221:jeb165373. doi: 10.1242/jeb.165373
Twining, C. W., Shipley, J. R., and Winkler, D. W. (2018b). Aquatic insects rich in omega-3 fatty acids drive breeding success in a widespread bird. Ecol. Lett. 21, 1812–1820. doi: 10.1111/ele.13156
Uttaro, A. D. (2006). Biosynthesis of polyunsaturated fatty acids in lower eukaryotes. IUBMB Life 58, 563–571. doi: 10.1080/15216540600920899
Keywords: alpha-linolenic acid, aquatic subsidies, arachidonic acid, Diptera, eicosapentaenoic acid, insect emergence, seasonality
Citation: Parmar TP, Kindinger AL, Mathieu-Resuge M, Twining CW, Shipley JR, Kainz MJ and Martin-Creuzburg D (2022) Fatty acid composition differs between emergent aquatic and terrestrial insects—A detailed single system approach. Front. Ecol. Evol. 10:952292. doi: 10.3389/fevo.2022.952292
Received: 24 May 2022; Accepted: 27 July 2022;
Published: 16 August 2022.
Edited by:
Jeff Wesner, University of South Dakota, United StatesReviewed by:
Gonzalo Gajardo, University of Los Lagos, ChileDavid L. Swanson, University of South Dakota, United States
Sydney Moyo, Rhodes College, United States
Copyright © 2022 Parmar, Kindinger, Mathieu-Resuge, Twining, Shipley, Kainz and Martin-Creuzburg. This is an open-access article distributed under the terms of the Creative Commons Attribution License (CC BY). The use, distribution or reproduction in other forums is permitted, provided the original author(s) and the copyright owner(s) are credited and that the original publication in this journal is cited, in accordance with accepted academic practice. No use, distribution or reproduction is permitted which does not comply with these terms.
*Correspondence: Tarn Preet Parmar, dGFybnByZWV0LnBhcm1hckBnbWFpbC5jb20=