- 1Department of Geography and Environmental Sciences, Northumbria University, Newcastle upon Tyne, United Kingdom
- 2Laboratorio de Geología de Llanuras, Facultad de Ciencia y Tecnología, National Scientific and Technical Research Council, Universidad Autónoma de Entre Ríos, Diamante, Argentina
- 3Department of Physics, Earth Science and Space Systems Engineering, Morehead State University, Morehead, KY, United States
- 4Department of Mechanical and Construction Engineering, Northumbria University, Newcastle upon Tyne, United Kingdom
- 5British Geological Survey, Nottingham, United Kingdom
Fossil fungi from periods warmer than modern climates provide unique insights into the future impacts of anthropogenic climate change. Here we report the fossil fungal assemblage from the late Middle Miocene Kenslow Member of central England, associated with climatic conditions warmer than the present-day. The identification of 110 morphotypes, which primarily relate to moist environments and the presence of wood, have been used to develop a new nearest living relative palaeoclimate reconstruction. The fungal assemblage indicates a Köppen–Geiger climate class, represented by temperate conditions, no dry season, and warm summers. This new fungal-based palaeoclimate reconstruction technique holds exciting potential to explore critically important but poorly understood palaeoenvironments, and the resulting qualitative inferences align well with previously published palaeobotanical quantitative estimates of palaeoclimate. These findings show that diverse fungal assemblages can successfully be used to reconstruct past climates for the first time.
Introduction
A lack of long-term and large-scale information on how fungi will respond to climate change prevents determining how ecological and historical factors potentially affect the evolution in space and time of fungal assemblages in different regions (Classen et al., 2015; Willis, 2018). Fungal biogeography has been shown to be controlled by climate, with mycorrhizal taxa having the narrowest climate tolerances (Větrovský et al., 2019). Intriguingly, Větrovský et al. (2019) found that overall fungal diversity was highest in the high northern latitudes, whereas coprophilous fungi and saprotrophs typically exhibit maximum taxonomic richness in the tropics (Richardson, 2001; Hyde et al., 2016). This latitudinal diversity gradient in fungi has also been observed during the Miocene Climatic Optimum [MCO – 17.5–14.5 million years ago (Ma)] (Romero et al., 2021). Observations during the late 20th Century have shown that soil fungal diversity is highly variable, with studies indicating both increased and decreased diversity in relation to warmer temperatures (Melillo et al., 2017; Delgado-Baquerizo et al., 2020; Steidinger et al., 2020). In light of the role fungi play in many ecosystem services, such as carbon sequestration, the lack of information on how warmer than modern climates will modify fungal assemblage composition, distribution and ecology becomes a pressing knowledge gap (Willis, 2018). The presence of fossil fungi in sedimentary successions deposited during warmer-than-modern deep time intervals provides an opportunity to fill this knowledge deficit and inform new predictions of the future response of fungi to warming from anthropogenically driven climate change.
The Miocene epoch (23.03–5.33 Ma) was an interval of geological time that was predominantly warmer than present (Pound et al., 2012a; Holbourn et al., 2018; Steinthorsdottir et al., 2021). The warmest interval of the Miocene was the MCO, during which diverse forests reached the Arctic Circle (Pound et al., 2012a; Steinthorsdottir et al., 2021) and modeled global air temperatures were 5°C warmer-than-present (Henrot et al., 2017; Burls et al., 2021). Following this climatic optimum, there was a progressive cooling toward near-modern sea surface temperatures by around 7 Ma (Herbert et al., 2016). However, this cooling is not recorded in the mid-latitudes of the North Atlantic region, where temperature gradients appear flat and indicate the presence of the warm oceanic current (Super et al., 2020). This warm current was likely responsible for the relatively warm temperatures observed in terrestrial palaeobotanical sites in western Europe (Utescher et al., 2015; Gibson et al., 2022;
McCoy et al., in review). Evidence for Miocene biodiversity, climate, and environments in the United Kingdom (UK) are limited to isolated and poorly dated deposits, of which the Brassington Formation of central England is the most extensive and best dated (Walsh et al., 1987, 1996; Wang et al., 2018; Pound et al., 2012b; Pound and Riding, 2016). The Brassington Formation is present in around 60 karstic hollows and consists of three members: Kirkham, Bees Nest and the uppermost Kenslow Member. Only the uppermost unit, the Kenslow Member, is fossiliferous (Boulter and Chaloner, 1970; Pound et al., 2012b,2019; Pound and Riding, 2016; Walsh et al., 2018; O’Keefe et al., 2020; McCoy et al., in review). The Kenslow member, the focus of this study, is composed of around 6 m of massive grey clay with common plant fragments near the top (Boulter et al., 1971; Walsh et al., 2018). The palaeoenvironment of the Kenslow Member has been interpreted to be a pre-subsidence lake, e.g., a cut-off lake on the Miocene flood plain, a post-subsidence hollow similar to sinkhole lakes or a wetland (Walsh et al., 1972; Pound et al., 2012b; McCoy et al., in review; respectively).
The Kenslow Member, dated to the late-Middle to Late Miocene (Serravallian-Tortonian), has yielded diverse fossil pollen, spore, and palaeobotanical remains that represent a subtropical to warm-temperate mixed forest (Boulter and Chaloner, 1970; Pound et al., 2012b; Pound and Riding, 2016). Climate reconstructions based on the co-existence approach indicate a Mean Annual Temperature (MAT) of 17–18.4°C, with mild winters of > 6.2°C and hot summers of 26.5–28.3°C (Pound and Riding, 2016). However, Gibson et al. (2022) proposed a cooler reconstruction using statistical techniques. In their reconstructions, MAT was reconstructed as 13.7°C ± 0.3°C, with winters of 4.3°C ± 0.8°C and warm summers of 21.9°C ± 0.3°C (Gibson et al., 2022). Both studies proposed higher than modern Mean Annual Precipitation (MAP) of 1096–1562 mm (Pound and Riding, 2016) and 955 mm ± 150 mm (Gibson et al., 2022). However, Pound and Riding (2016) proposed a degree of precipitation seasonality in the Serravallian of the United Kingdom, whereas Gibson et al. (2022) reconstructed a low degree of seasonality. Recent research has provided the first evidence of fossil fungal remains (Pound et al., 2019), and continued study revealed an abundant and diverse fungal assemblage. Given the uncertainty in how modern fungi will respond to anthropogenically driven climate change, the fungal assemblage in the Kenslow Member provides evidence for the diversity of the Dikarya group under a warmer and wetter climate than present-day. The aim of this paper is to document the biodiversity and ecology of the fungi preserved in the Kenslow Member and to provide evidence for how these assemblages change within a developing wetland (McCoy et al., in review) during warmer than present conditions. The fossil fungal assemblage is also used to reconstruct qualitative climate information and this is compared back to previous paleobotanical reconstructions.
Materials and methods
Samples were collected from the Kenslow Member at Bees Nest Pit (NGR SK 1818 6158; 53.09°N; 1.64°W), a disused sand quarry ca. 1 km northeast of Brassington village, near Wirksworth, Derbyshire, United Kingdom (Figure 1). Approximately 50 g of clay were collected from each of the 17 sampling points along a 2.30 m vertical transect that covered the entire exposed Kenslow Member (Figure 2). Samples came from the blue-gray clay and were spread across the entire Kenslow Member, but with concentrations at woody horizons (Figure 2). To maximise the diversity of fungi recovered, additional samples of clay were taken from the cracks in fossil wood recovered from the site. At least 5 g of each sample were prepared using either an acid digestion methodology (following Pound and Riding, 2016; Pound et al., 2021; Riding, 2021) or a non-acid density separation technique (following O’Keefe and Eble, 2012; Caffrey and Horn, 2013). Samples were crushed to < 1 mm prior to processing and sieved through a 250-micrometre top sieve, and where non-acid methods were utilized, a 5-micrometre bottom filter was used to facilitate removal of the remaining clay. Samples were analysed using Leica DM750 and DM2000 microscopes and photographed with Leica ICC50W cameras run by Leica Application Suite® software at both Morehead State University, United States and Northumbria University, United Kingdom. Entire slides were analysed for fungal palynomorphs to ensure maximum identification of taxa (presence) for the palaeoclimate reconstructions technique. NLR techniques rely primarily on presence/absence of taxa, when applied to dispersed remains (Utescher et al., 2014). For this reason and because the number of fungal individuals produced vs. preserved are very poorly understood (Taylor et al., 2015), numbers of individual fungal morphotypes were not counted for this study, rather, morphotype presence-absence was recorded. Z-stacked images were constructed to resolve fine details using Helicon Focus®. Slides prefixed with MPA are archived at the British Geological Survey. Slides with a Wood Sample designation are housed at the Palynology Lab of the Cold and Palaeoenvironments Research Group at Northumbria University. Slides with a numerical-only designation are housed at the OPaL Lab at Morehead State University (Supplementary Table 1).
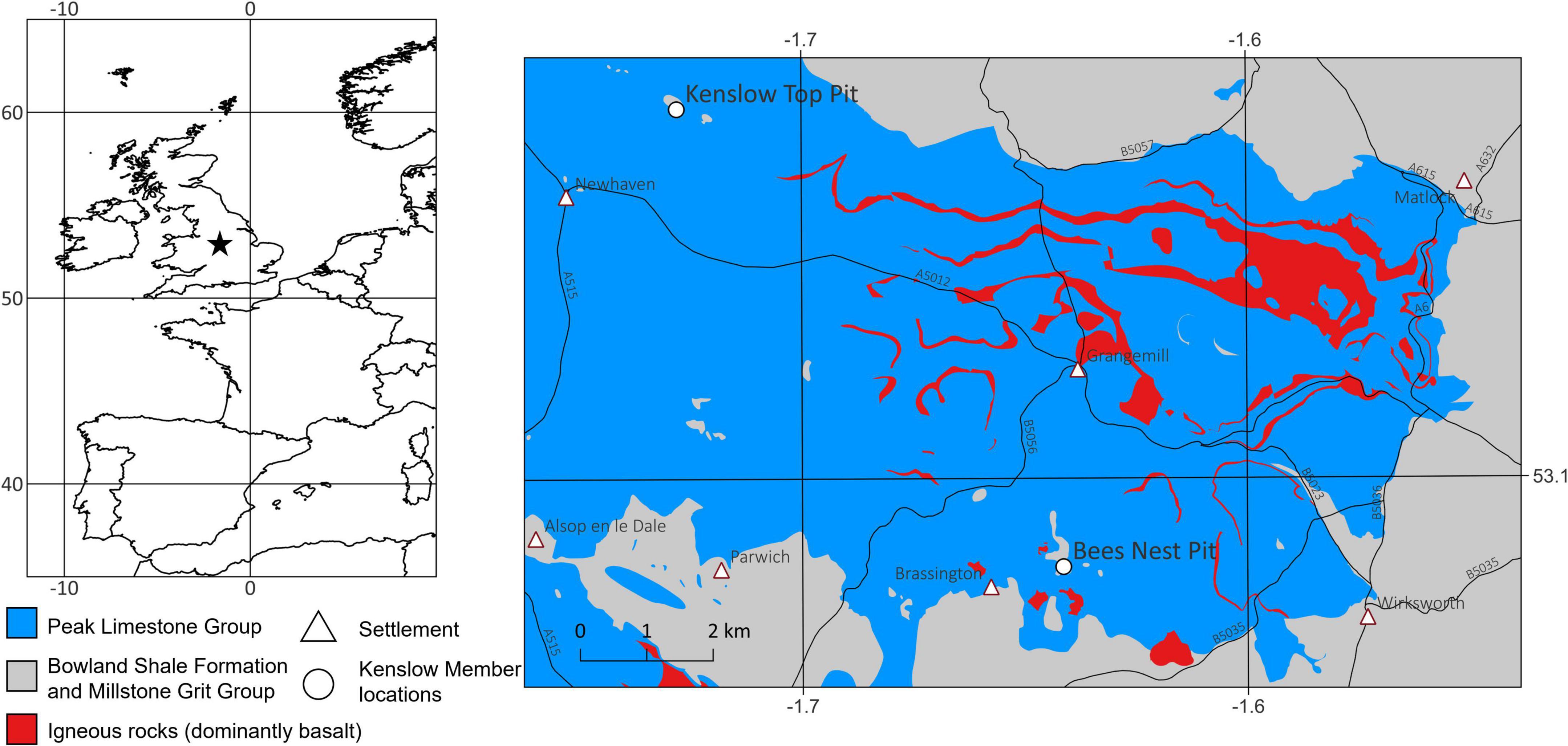
Figure 1. A location map of Bees Nest Pit (and Kenslow Top Pit) in the area around Brassington and Newhaven, Derbyshire, central England, United Kingdom. The (left-hand panel) shows the location of this part of the White Peak, Derbyshire in the context of western Europe. The (right-hand panel) is a simplified geological map of the area around Brassington and Newhaven. The Lower Carboniferous Peak Limestone Group is depicted in the mid-blue ornament; the Brassington Formation is preserved in karstic cavities within this major carbonate unit. The bright red ornament represents igneous rocks, dominantly basalt, within the Peak Limestone Group. The mid gray color represents the Bowland Shale Formation and the Millstone Grit Group (uppermost Lower and Upper Carboniferous). White triangles show the location of settlements and the larger circles show the two remaining pits with the Kenslow Member presence. The map was generated using QGIS and is based upon the British Geological Survey 1:10000 scale digital geological map (Smith, 2013), using the EDINA Geology Digimap Service. The road network is from the Ordnance Survey Open Roads (SHAPE geospatial data), scale 1:25000, updated on 23 September 2021. Downloaded on 2022-04-13 09:54:41.238.
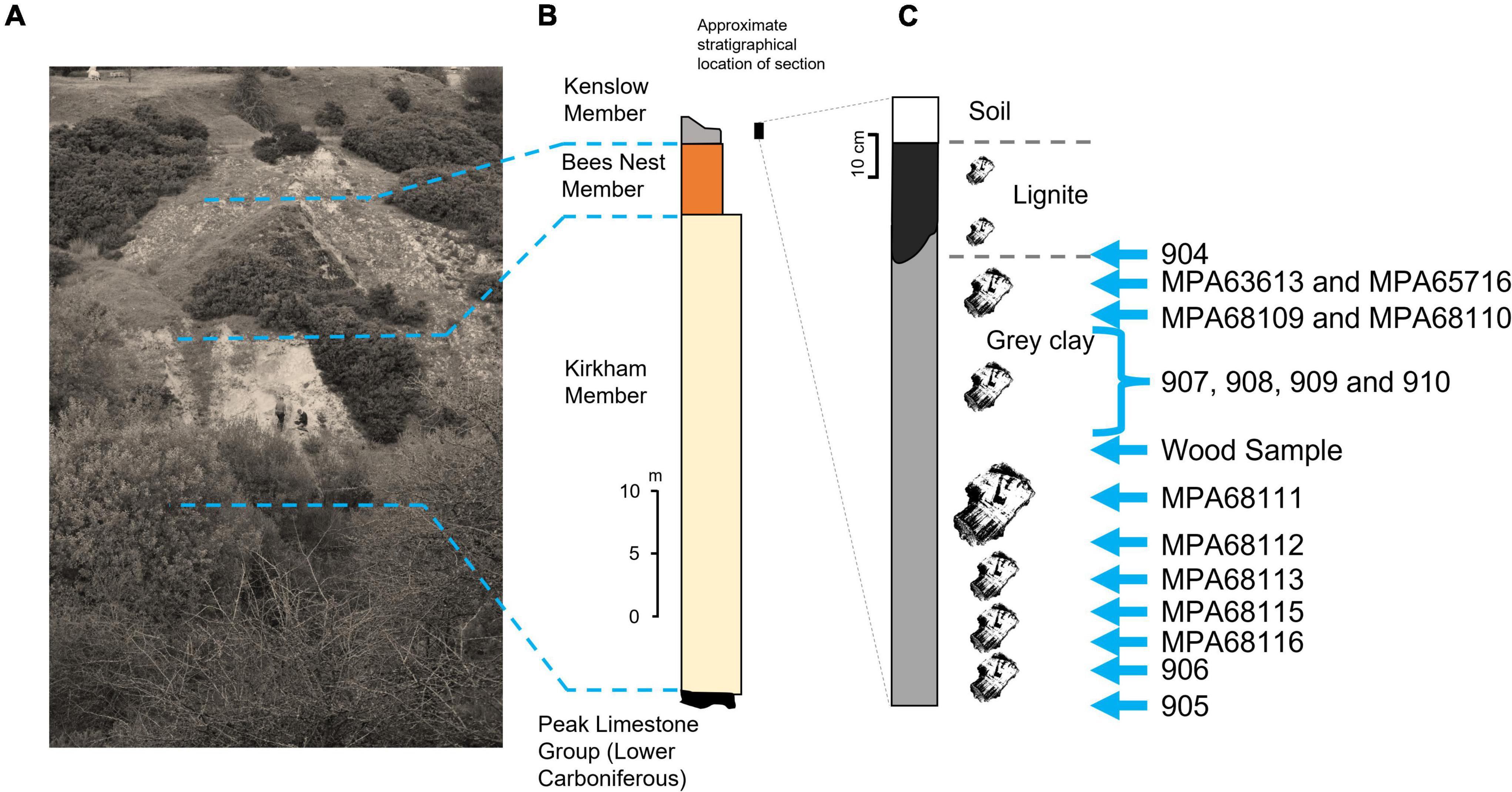
Figure 2. The lithostratigraphy of the Brassington Formation and the samples from the Kenslow Member analysed herein. (A) A field photograph of the exposed section at the central north part of Bees Nest Pit, note people for scale. The dashed lines indicate the boundaries between the three members in this succession, which is the type section of the Brassington Formation. (B) A generalised lithostratigraphy of the Brassington Formation at Bees Nest Pit based on Boulter et al. (1971) and O’Keefe et al. (2020), note the black bar to show the relative location and scale of the section in (C) to (B). (C) A simplified lithological log of the Kenslow Member at Bees Nest Pit. Wood silhouettes indicate the position of fossil wood horizons (the wood fragment sizes reflect the sizes observed in this section), and blue arrows indicate the location of the samples used in this study.
Fossil fungi were first grouped following Nuñez Otaño et al. (2021) and Romero et al. (2021). Taxonomic identification, when possible, was completed using morphometric methods through comparisons with accepted species1,2 based on the primary literature of taxa encountered (Ellis, 1971, 1976; Ellis and Ellis, 1997, 1998; Seifert et al., 2011; Guarro et al., 2012; among many others – see Supplementary Table 2), comparisons with material held in reference collections at Morehead State University and CICyTTP-Prov. ER-CONICET-UADER, Argentina, and those recorded in the John Williams Index of Palaeopalynology (JWIP) (Riding et al., 2012), and the Non-Pollen Palynomorph Image Database (NPP-ID3) (Shumilovskikh et al., 2022). Fungal remains that were not able to be identified were assigned an identifying acronym following O’Keefe et al. (2021); in this case, the acronym BN for Bees Nest and a number were used (e.g., BN-31). Full descriptions of the BN taxa are available in Supplementary Table 1.
Fungal morphological and functional traits along with their geographical distributions were determined through comparison with the primary literature (Supplementary Table 2) and FungalTraits v. 1.2 database (Põlme et al., 2020) using the Nearest Living Relative (NLR) approach. To reconstruct Miocene climate, a simple NLR approach was applied. Once the Kenslow Member fossils were identified, a literature and online database survey was conducted until May 2022 in order to identify known global occurrences of each taxon (full reference list available in Supplementary Table 2; Pound et al., 2022).
After application of the Sorensen Index to the presence-absence data of fungal palynomorphs, a non-metric multidimensional scaling (nMDS) and group cluster analysis were used to ordinate the samples. The Sorensen Index was chosen because sample sizes were adequate and it enables the use of presence-absence data (Schneck and Melo, 2010). The cluster analysis was run with a similarity profile (SIMPROF) test, with 9999 permutations, to identify groups of samples based on their fungal similarities. SIMPROF seeks to identify clusters within a hierarchical cluster analysis whereby no further branching can be identified with increasing similarity and groups can be considered homologous (Clarke et al., 2008). An Analysis of Similarity (ANOSIM) test was used to explore whether sample type (wood versus sediment) or processing method (acid digestion versus non-acid technique) had influenced the results. All analyses were performed in Primer 6 (Plymouth Routines in Multivariate Ecological Research version 6, PRIMER-E Ltd., Plymouth Marine Laboratory, United Kingdom) following a presence/absence pre-treatment in the same software (Clarke and Warwick, 2001; Clarke and Gorley, 2006).
To reconstruct palaeoclimates from fungi, a NLR approach was applied. This branch of palaeoclimate reconstruction techniques builds on the conceptual framework of the present being analogous to the past and is routinely applied to paleobotanical remains (e.g., Utescher et al., 2014; Gibson et al., 2022). For paleobotanical applications, the present-day distribution of a fossil’s NLR is used to derive climatological values. These present-day climate values of each fossil taxon in an assemblage are then analysed in various ways to produce numerical palaeoclimate values (Thompson et al., 2012; Utescher et al., 2014; Gibson et al., 2022). In developing a method to apply to fungal remains, it became apparent that the known present-day distribution of many fungi are not as well documented as plants. To circumvent this limitation, we did not reconstruct numerical values and instead focused on a qualitative reconstruction through the Köppen–Geiger system (Beck et al., 2018). This system uses threshold values of modern climatology to define terrestrial areas into five broad classes (tropical, arid, temperate, continental, and tundra), which are then subdivided into 30 sub-types based on seasonal changes in temperature and precipitation (Beck et al., 2018). Fungal palynomorphs identified to extant genus or below were used in the analysis; those identified to family, fossil taxon, or unnamed (assigned a BN- number) were excluded from the analysis. Published occurrences of the NLR of the identified fossil fungi were then compared to the present-day Köppen–Geiger system maps of Beck et al. (2018). All classes where that taxon has been recorded as occurring were then recorded against that fossil fungus providing a 660-point occurrence dataset (Pound et al., 2022). Köppen–Geiger class occurrences for each fossil fungus were then summed for each sample and the class within which all fossil fungi could have co-existed is presented as the reconstruction. Where multiple classes are reconstructed as being possible, all are presented as equally likely. To test how robust the new fungal-based Köppen–Geiger class reconstructions are, we compared modern climatological data from WorldClim2.1 (Fick and Hijmans, 2017) to the present-day distribution of Köppen–Geiger climate classes and the quantitative palaeoclimate reconstructions of the Kenslow Member that have used three different nearest living techniques (Gibson et al., 2022; McCoy et al., in review).
Results
Fungal assemblages
To date, 110 unique fossil fungal morphotypes (Figures 3–8 and Table 1) have been identified in the Kenslow Member as exposed in Bees Nest pit. Of these, 72 are assigned to extant or known fossil taxa, while an additional 38 have yet to be identified. The assemblages are co-dominated by amerospores (n = 36; Figure 3) and phragmospores (n = 36; Figures 5, 6). Seven taxa represent epiphyllous structures (Figure 8), while all but one of the remainder are reproductive propagules. That one, Balaniopsis sp., is represented by a conidiogenous cell (Figure 7).
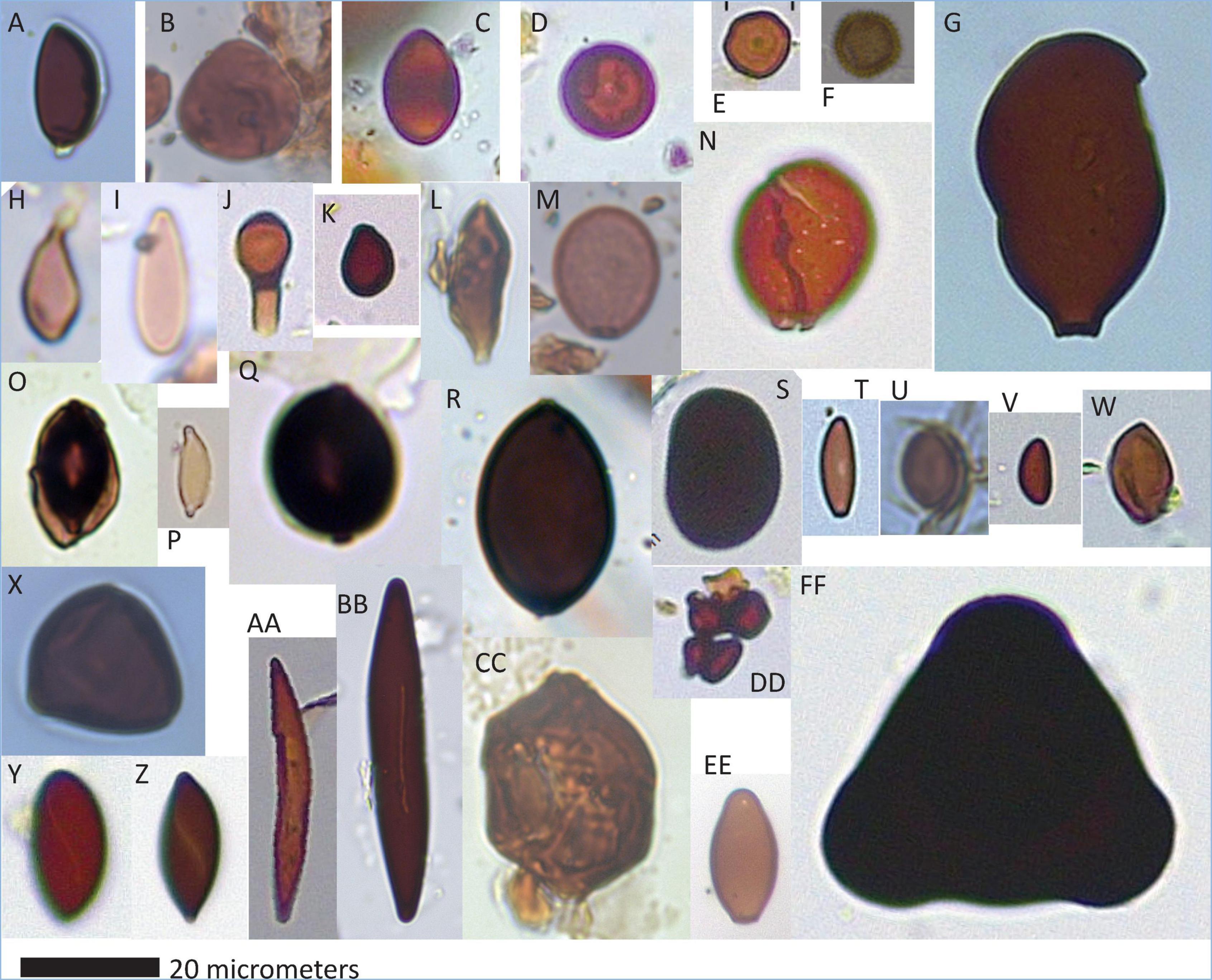
Figure 3. Single-celled fungal remains (amero- types). (A) cf. Acrogenospora, (B) cf. Acrogenospora, (C) BN-2, (D) BN-1, (E) cf. Gilmaniella, (F) cf. Tulostoma, (G) BN-9, (H) BN-3, (I) BN-35, (J) BN-25, (K) BN-5, (L) Juglanconis sp. 1, (M) cf. Periconia, (N) Juglanconis sp. 2, (O) BN-6, (P) BN-4, (Q) BN-34, (R) cf. Podospora, (S) cf. Nigrospora, (T) Annulohypoxylon sp., (U) BN-8, (V) Xylariaceae, (W) Chaetomiaceae, (X) Catenularia elsikii, (Y) Helicogermslita sp., (Z) Rosellinia aff. franciscae, (AA) cf. Rosellinia aff. necatrix, (BB) Kretzchmaria aff. deusta, (CC) BN-11, (DD) BN-37, (EE) cf. Cercophora sp., and (FF) Zopfiella neogenica.
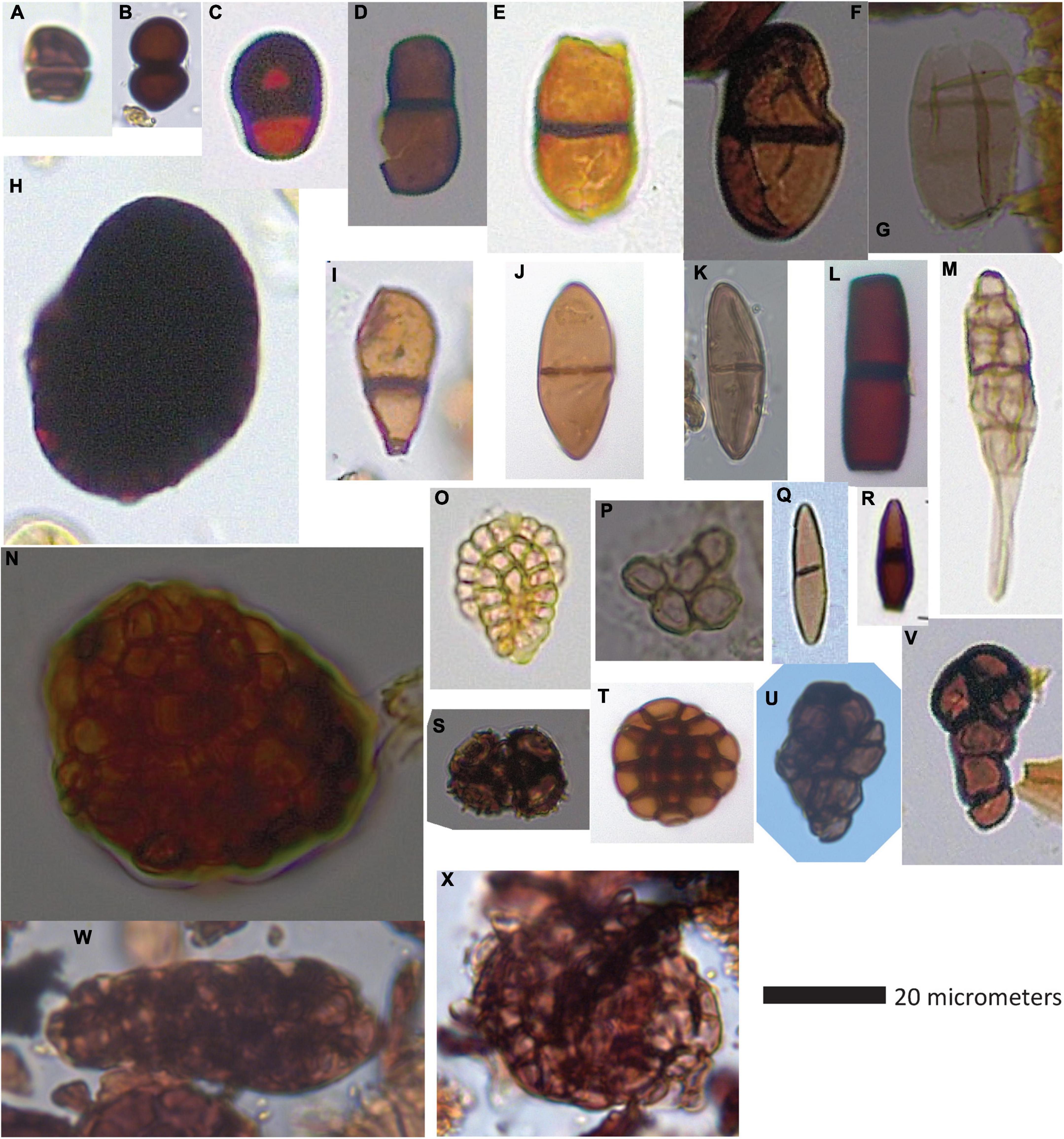
Figure 4. Fungal remains with two or more cells (didymo-, dictyo-, broken phragmo-, stauro-, helico-, and bubil- types). (A) BN-7, (B) BN-32, (C) cf. Endophragmiella, (D) BN-13, (E) cf. Ascotaiwania, (F) BN-36, (G) BN-33, (H) cf. Ascotaiwania, (I) BN-38, (J) cf. Subersisphaeria, (K) Delitschia sp., (L) cf. Sporoschisma uniseptatum, (M) Alternaria sp., (N) Endoconidioma sp., (O) Dictyosporium sp., (P) BN-27, (Q) Amphisphaeria aff. millepunctata, (R) BN-12, (S) Isthmosphora aff. spinosa, (T) Acrodictys sp., (U) Stemphylium sp., (V) cf. Circinoconis, (W) cf. Xenosporium, and (X) BN-26.
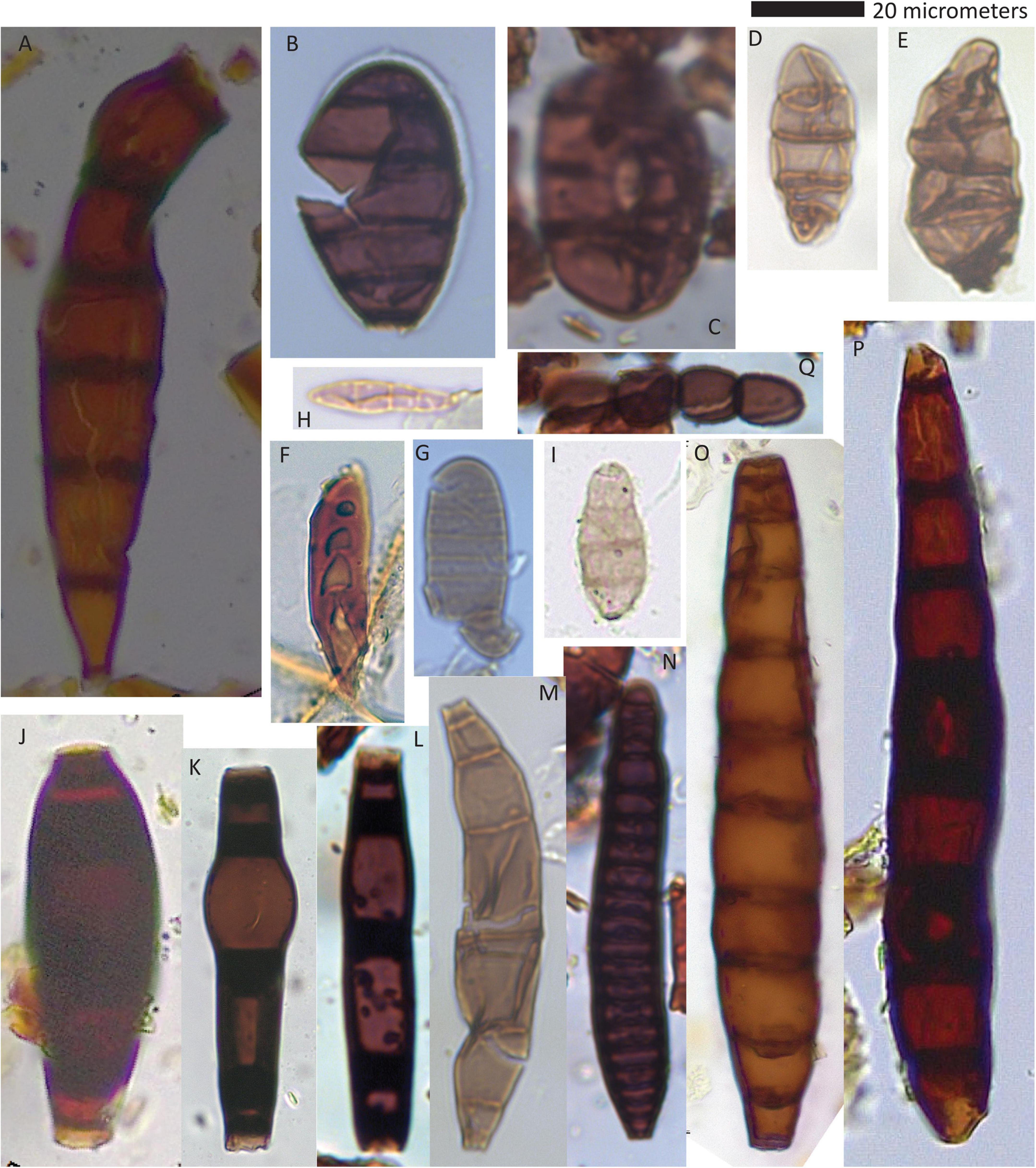
Figure 5. Large elongate fungal remains with two or more cells (phragmo- types). (A) cf. Acrogenotheca, (B) BN-21, (C) BN-19, (D) cf. Lophiostoma, (E) cf. Pithomyces, (F) Dreschlera/Bipolaris, (G) BN-20, (H) BN-17, (I) BN-23, (J) BN-24 (cf. Rhexoampullifera), (K) Rhexoampullifera sufflata, (L) Rhexoampullifera stogieana, (M) Sporidesmium sp. 2, (N) Ellisembia sp., (O) cf. Xylomyces, (P) Lobatopedis sp., and (Q) Preussia/Sporormiella.
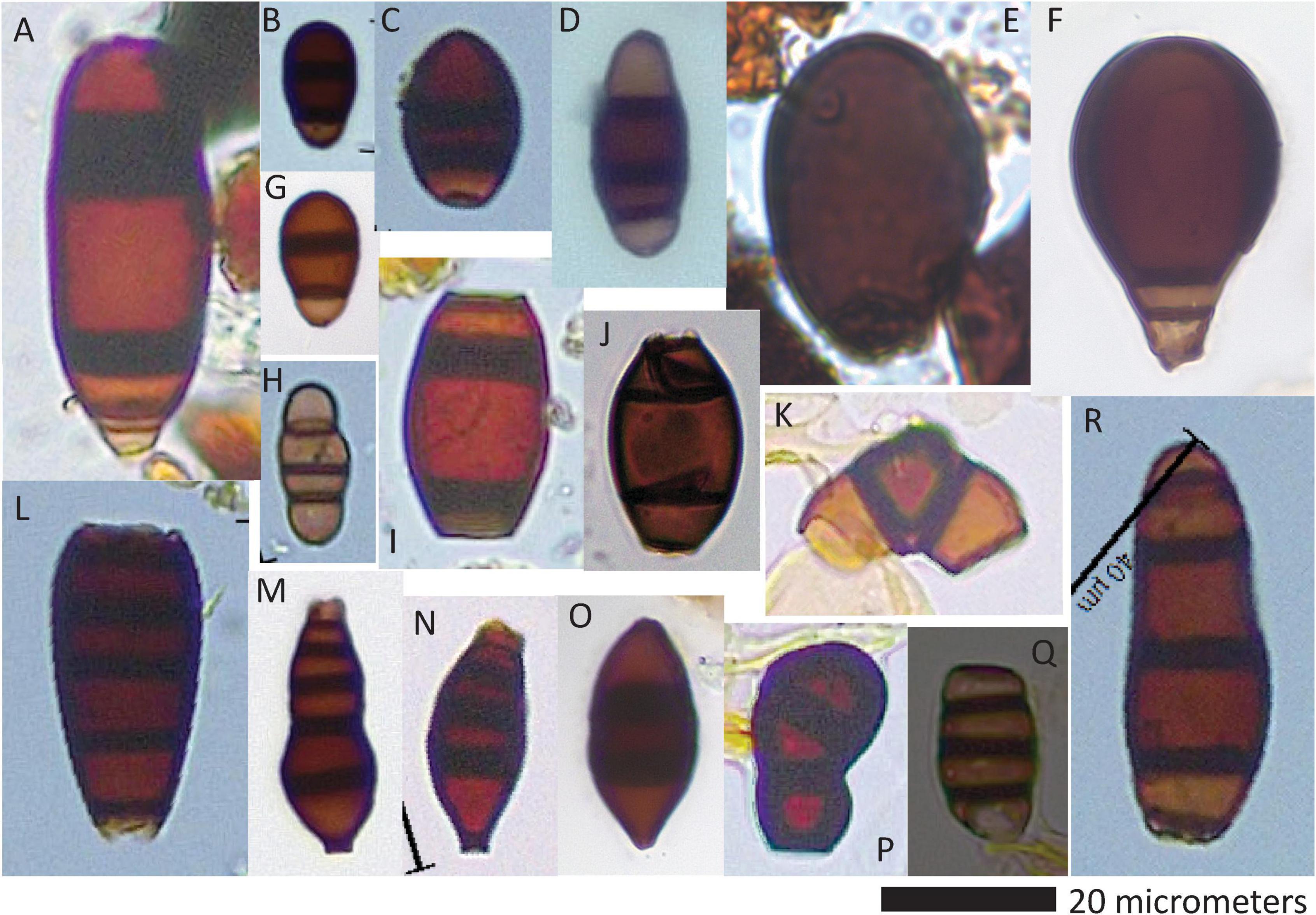
Figure 6. Small and medium elongate fungal remains with two or more cells (phragmo-types). (A) cf. Bactrodesmium aff. abruptum, (B) cf. Bactrodesmium sp. 1, (C) cf. Bactrodesmium sp. 2, (D) cf. Bactrodesmium sp. 3, (E) Brachysporiella sp., (F) Brachysporiella aff. setosa, (G) Spadicoides aff. klotzchii, (H) cf. Melanomma, (I), cf. Endophragmiopsis sp. 2, (J) cf. Endophragmiopsis sp. 1, (K) Curvularia sp., (L) BN-15, (M) Sporidesmium aff. fluminicola, (N) Sporidesmium sp., (O) BN-14, (P) BN-16, (Q) BN-22, and (R) BN-18.
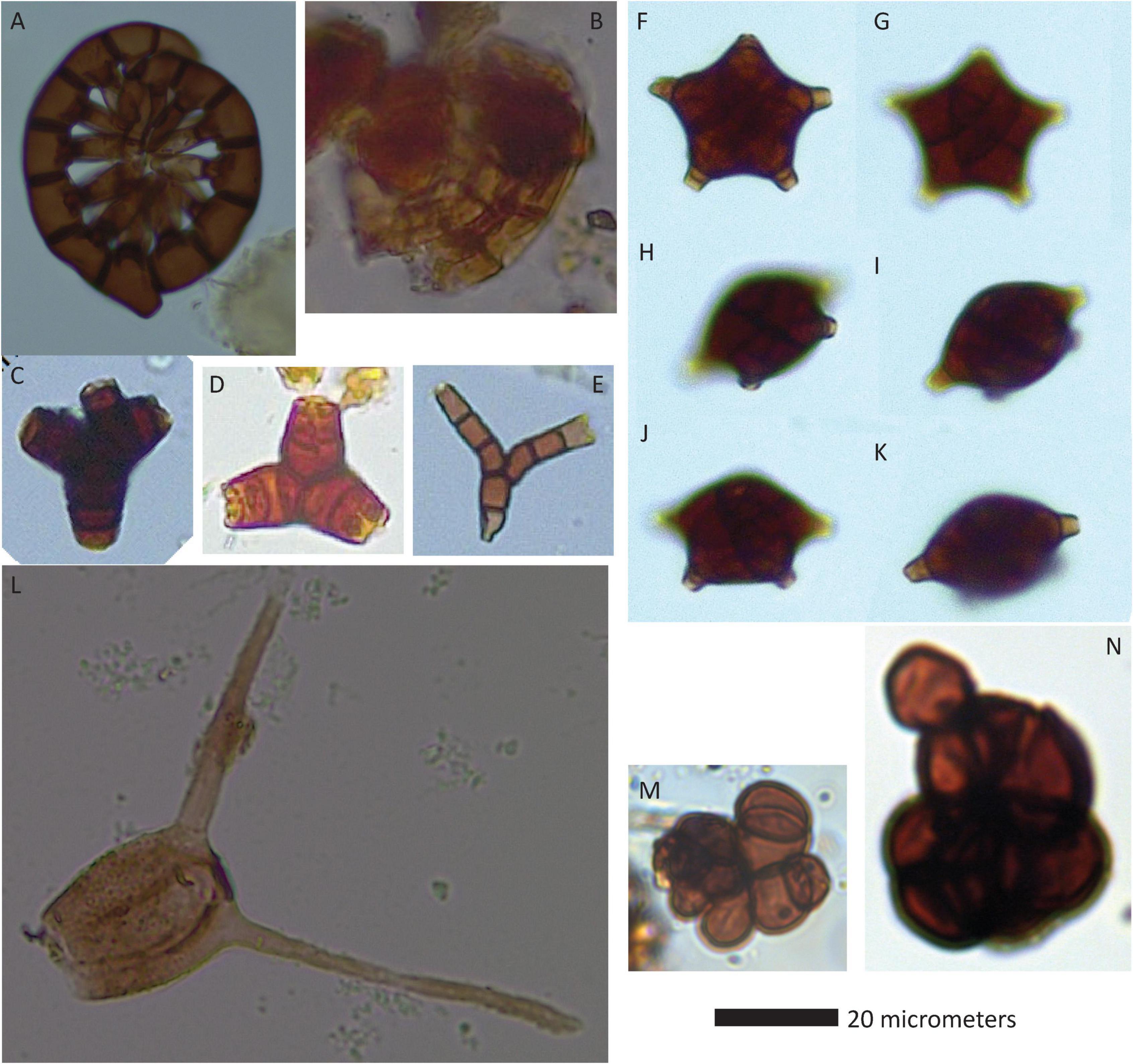
Figure 7. Coiled, star-shaped, and otherwise complex fungal remains (helico-, stauro-, and other types). (A) Pesavis tagluensis, (B) cf. Ctenosporium, (C) Tetrabrunneospora aff. ellisii, (D) cf. Triposporium, (E) Diplocladiella aff. scalaroides, (F–K) successive views of a single conidiogenous cell of cf. Balaniopsis mounted in glycerine, (L) Tetraploa aristata, (M) BN-30, (N) BN-29.
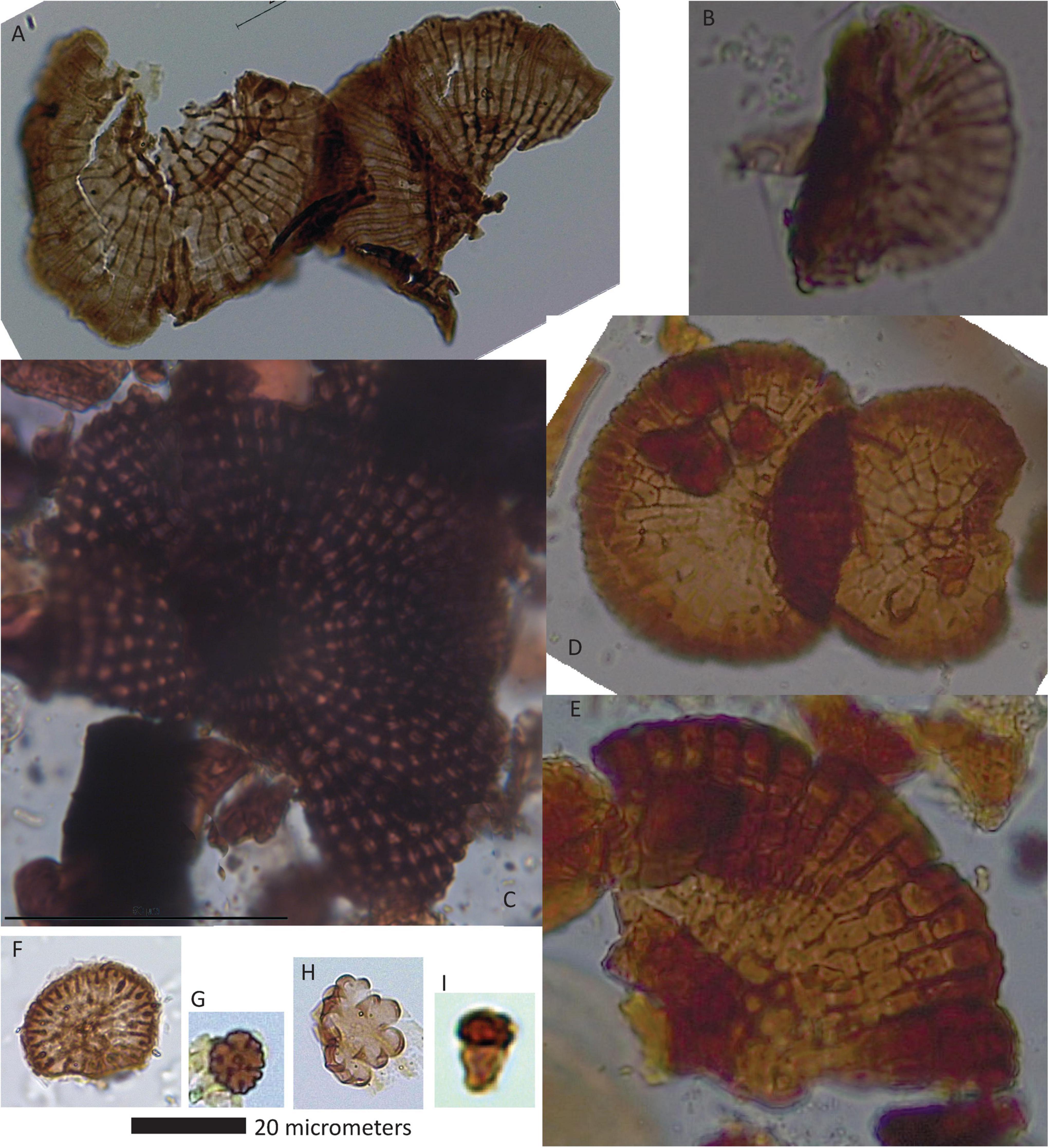
Figure 8. Epiphyllous fungi. (A) Neomycoleptodiscus sp., (B) BN-28, (C) Trichothyrium sp., (D) Microthyrium sp. 2, (E) Microthyrium sp. 1, (F) microthyraceous germling, (G) microthyraceous germling sp. 2, (H) microthyraceous germling sp. 1, and (I) BN-31.
The lowermost 2 cm of the Kenslow Member (sample 906) contains a depauperate palynological assemblage that is dominated by charcoal and burnt (i.e., black) pollen. Within this, only fossil cf. Tulostoma and Zopfiella neogenica were identified. Up section, the variety of Non-Pollen Palynomorphs, especially fungi, in the samples increased significantly beginning with sample MPA 68116. Recovery is highly variable through the lower part of the unit (through sample MPA 68112), and fungi recovered tend to be cosmopolitan saprotrophs, including, Acrodictys sp., Alternaria sp., and cf. Tulostoma.
Sample MPA 68116 contains a higher diversity assemblage, including Ascobolus sp., Rhexoampullifera stogiana, and several indeterminant taxa (Table 1), while sample MPA 68113 contained an exceptionally depauperate assemblage of only one taxon. Approximately midway up section, beginning with sample MPA 68111, diversity increased again. Ordination through cluster analysis showed a grouping that can be interpreted biostratigraphically, even though no stratigraphy was provided to the analysis (Figure 9; Supplementary Figure 1). Samples 906 – MPA68112 were grouped and SIMPROF shows this group to be homologous; MPA68113 is the outgroup due to poor recovery in this sample. All samples above MPA68112 in the sequence were also grouped into a SIMPROF homologous group (Figure 9; Supplementary Figure 1).
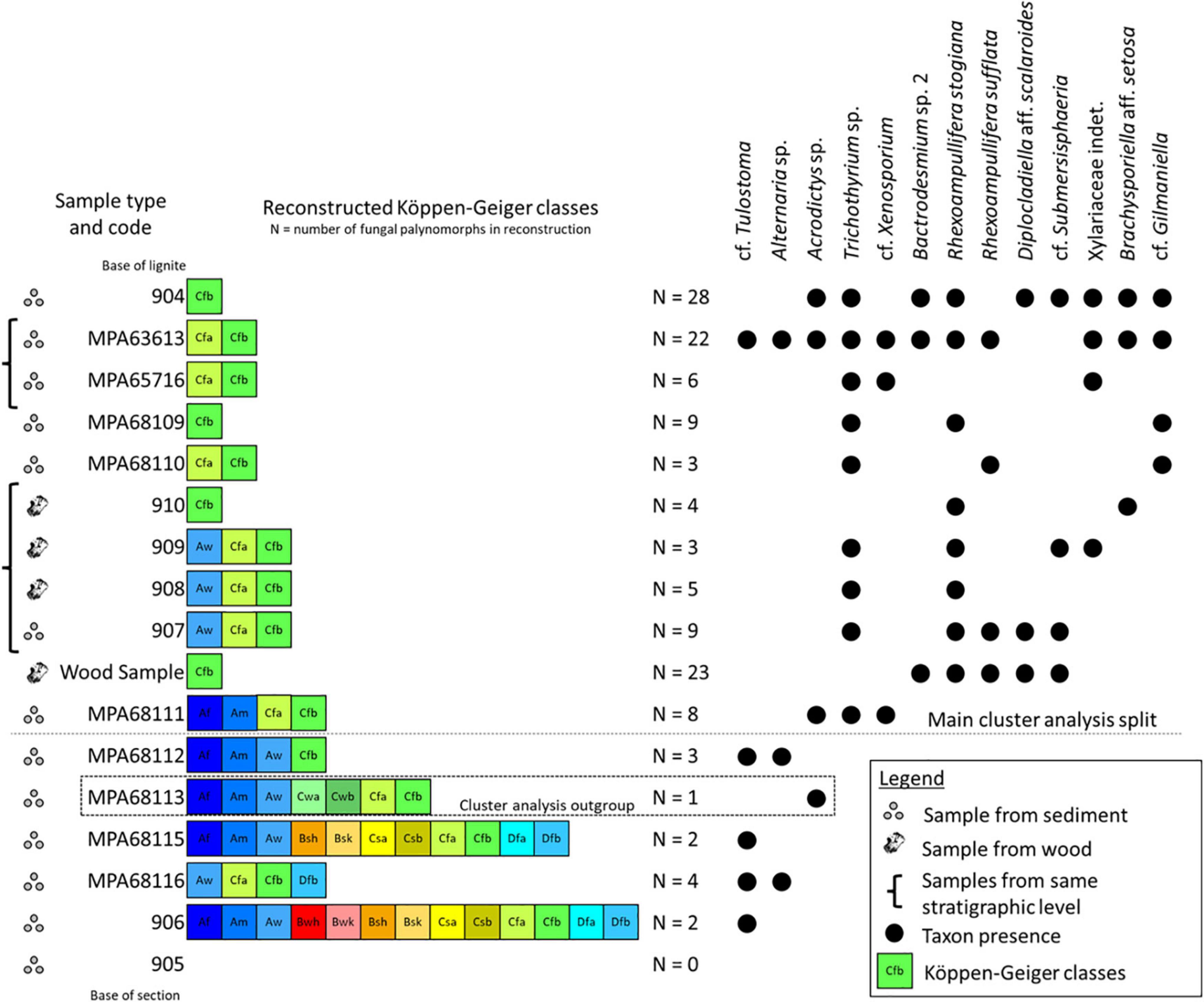
Figure 9. Stratigraphical distribution of reconstructed Köppen–Geiger climate classes and most frequent fungal palynomorphs in the Kenslow Member of Bees Nest Pit. Sample type is indicated by three dots (sediment) or wood silhouette (wood); sample code follows Figure 2. Reconstructed Köppen–Geiger climate classes are presented in the color scheme proposed by Beck et al. (2018) and the number represents the fungal palynomorphs used for the reconstruction. The fungal palynomorphs noted in on the right side of the figure were those that occurred in three or more samples; occurrences are indicated by black dots.
Clay from the so-called wood horizon (Pound et al., 2019; O’Keefe et al., 2020) in the middle of the Kenslow Member (beginning with the Wood Sample) contains abundant fungi, of which 24 were identifiable to modern taxa. These include a number of wet-tolerant taxa, such as cf. Bactrodesmium sp. 2, Diplocladiela aff. scabroides, Rhexoampulifera species, cf. Submerisphaeria sp., and cf. Triposporium. Sample 907, obtained from the clay adjacent to wood samples 908–910, contains an interesting assemblage of 9 identifiable taxa, including conidiogenous cells of cf. Balaniopsis sp., Diplocladiela aff. scabroides, indeterminant stalked microthyraceous fungi (BN-28), Rhexoampulifera stogieana, cf. Submersisphaeria, and Tetraploa aff. artista, as well as the very enigmatic fungus Pesavis tagluensis. Clay from cracks in wood (samples 908–910) recovered from the wood horizon contains a similarly diverse assemblage: abundant members of Rhexoampullifera, mostly R. stogiana, but also R. sufflata and an as-yet un-named form, designated BN-24, and Tetraploa aff. artista as well as epiphyllous fungi, helicosporous conidia, Preussia/Sporormiella, cf. Submersisphaeria, and Zopfiella neogenica, among others (Table 1), although the overall number of named taxa in individual samples is relatively low (n = 3–4, Figure 9).
A metre above the wood horizon (sample 904) and somewhat below that (MPA 63613), fungal diversity again increases, with 25 and 28 identified taxa, respectively (Table 1 and Figure 9). Rhexoampullifera and cf. Submersisphaeria are still common, although not as common as in the wood horizon, cf. Bactrodesmium become increasingly common. Microthyraceous fungi, including germlings of at least two morphologies, and Trichothyrium sp. are very common, as are Diplocladiela aff. scalaroides and other staurospores. The nMDS ordinations showed a grouping of samples processed from sediment associated with fossil wood samples (Supplementary Figure 2). However, the ANOSIM test showed that neither sample type (Global R = 0.062, Significance 29.2%), or processing method – see Supplementary Table 1 (Global R = –0.025, Significance 55%) controlled the similarity of fungal palynomorph assemblages.
Climate reconstruction
The new fungal-based reconstructions show the presence of a Cfb (temperate, no dry season, warm summer) climate during Kenslow Member deposition (Figure 9). For the number of fungal taxa used in the reconstruction, the reader is referred to Figure 9. This class is reconstructed for all assemblages. In the upper part of the section, four of the ten assemblages reconstruct the Cfb class as the only possible class within which all the modern relatives of the fossil fungi could co-occur (Figure 9). Where multiple classes are equally possible (all taxa reconstruct multiple classes) for the other assemblages, these are the Cfb, Cfa (temperate, no dry season, hot summer) and the Aw (Tropical, savannah). In the lower part of the section, as defined by the cluster analysis, all assemblages reconstruct multiple Köppen–Geiger classes, but always contain the Cfb class (Figure 9).
Discussion
Fungi-based palaeoenvironmental reconstruction
The main cluster split noted in Figure 9 and Supplementary Figure 1 appears to roughly correspond to the CONISS-indicated boundary between KM19-1 (lower portion of the section – a low diversity conifer dominated pollen assemblage) and KM19-2 (upper portion of the section – a more diverse mixed forest assemblage) pollen zones of McCoy et al. (in review). This boundary separates more (zone KM19-2) and less (zone KM19-1) diverse plant pollen and spore assemblages that appear to correspond to initiation of what would become a peat-producing wetland system. The change is coupled with increased coalification of preserved wood (O’Keefe et al., 2020) and an increase in freshwater submerged fungal taxa (cf. Bactrodesmium, Brachysporiella aff. setosa, Rhexoampullifera species, and stauro-forms, including Diplocladiella aff. Scalaroides).
Palaeoclimate reconstruction from fossil fungi
The climate reconstruction of Cfb places the Miocene UK in the same Köppen–Geiger climate classification as present-day (Beck et al., 2018). The Cfb class is defined by a hottest month temperature of < 22°C (Peel et al., 2007; Beck et al., 2018). Plant-based co-existence approach reconstructions from the Kenslow Member at Bees Nest Pit of warmest month temperature produce temperatures warmer than this at 26.5–28.3°C (Pound and Riding, 2016), 21.6°–28.1°C (Gibson et al., 2022), and 24.7–27.9°C (McCoy et al., in review). However, statistical techniques produce warmest quarter temperatures in line with anticipated values for a Cfb class – 21.9°C ± 0.3°C and 20.6°C ± 5.8°C (Gibson et al., 2022). That said, by definition, the warmest quarter should be colder than the hottest month. Comparison of the palaeobotanical palaeoclimate reconstructions to the present-day climatology of the Cfb, and warmer Cfa, Köppen–Geiger climate classes shows that the Bees Nest Pit reconstructions are positioned at the upper limits of the Cfb class and lower edge of the Cfa class (Figure 10). The co-existence approach estimate lies within the Cfa Köppen–Geiger climate class (Figure 10), but is known to be estimating warmer than actual temperatures due to the underlying dataset (Gibson et al., 2022). The present-day United Kingdom climate for Derbyshire (based on WorldClim2.1) is positioned toward the cooler end of the Cfb Köppen–Geiger climate class (Figure 10). All previous palaeoclimate reconstructions also show that winter temperatures were above 0°C, helping to support a classification in the Cfb class (Pound and Riding, 2016; Gibson et al., 2022; McCoy et al., in review).
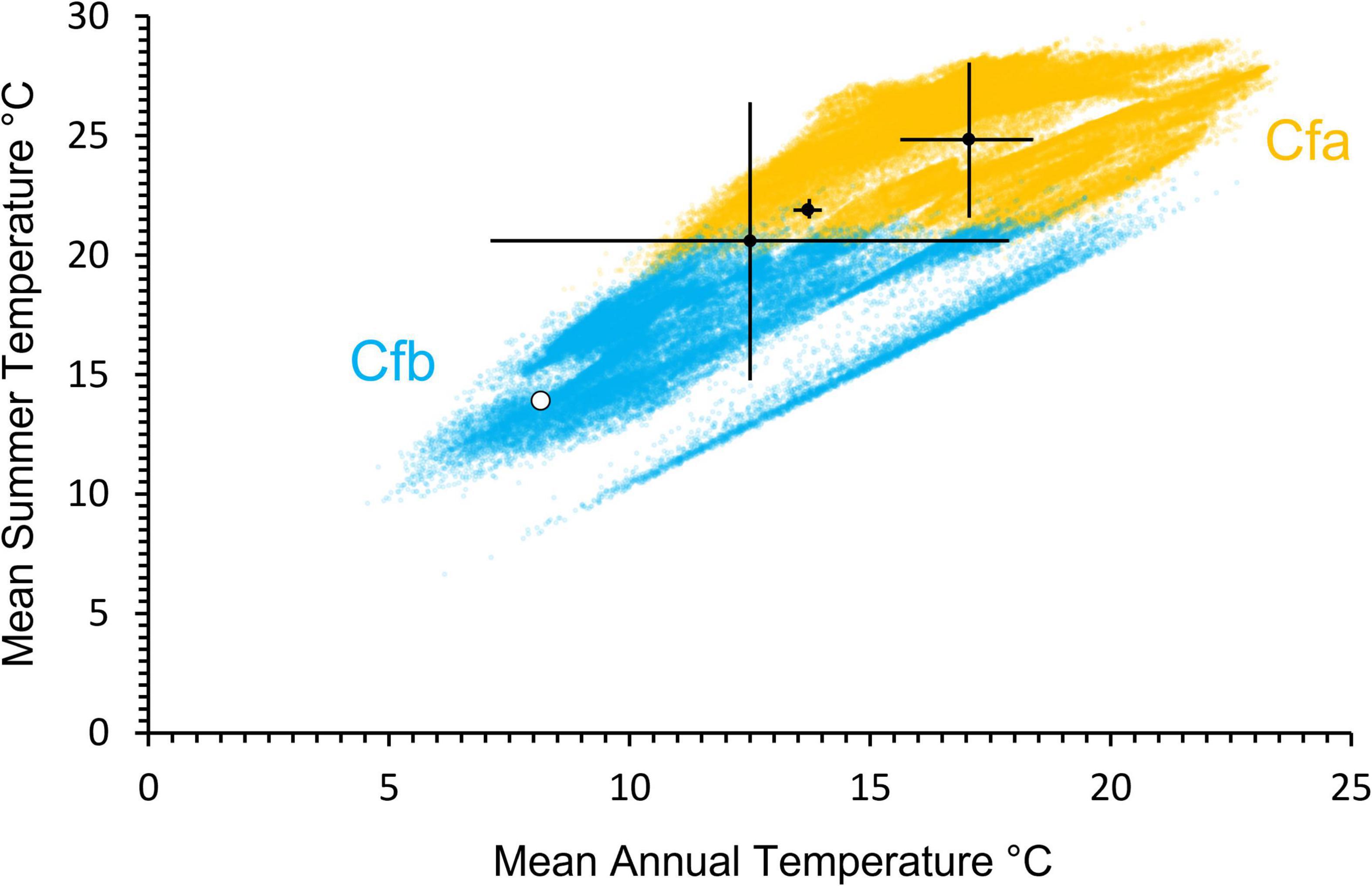
Figure 10. Climatological distribution of the present-day Cfa (yellow) and Cfb (blue) Köppen–Geiger climate classes based upon mean annual temperature (x-axis) and summer temperature (y-axis). The white dot shows the present-day values for Derbyshire, United Kingdom. The black dots with error bars are paleobotanical based reconstructions from Gibson et al. (2022) and McCoy et al. (in review). The present-day dataset comprises 39,646 data points using Köppen–Geiger climate classifications from Beck et al. (2018) and WorldClim2.1 data from Fick and Hijmans (2017).
The fungi-based reconstruction of a Cfb Köppen–Geiger climate classification also implies minimal differences in precipitation seasonality (Beck et al., 2018). Using co-existence approach reconstructions, Pound and Riding (2016) had proposed a degree of seasonality for the Kenslow Member at Bees Nest Pit. However, reconstructions using statistical techniques show relatively low seasonality with high MAP (Gibson et al., 2022). Co-existence approach reconstructions agree with relatively high MAP, with all techniques showing it was likely greater than 1000 mm per year (Pound and Riding, 2016; Gibson et al., 2022; McCoy et al., in review). The seasonality values presented by Gibson et al. (2022) all fall within the values experienced by the present-day Cfb Köppen–Geiger climate class (Fick and Hijmans, 2017; Beck et al., 2018). The consistency between the fungi-based reconstruction and multiple palaeobotanical techniques shows the applicability of fossil fungi to reconstructing qualitative palaeoclimate information.
A Cfb Köppen–Geiger climate classification for the late Middle Miocene of Derbyshire is consistent with reconstructions from plant fossils on Iceland for the Middle to Late Miocene as well (Denk et al., 2013). This has been proposed as showing the presence of the Gulf Stream, which would have also contributed to the warmth and humidity of the United Kingdom during the deposition of the Kenslow Member. Sea surface temperature data points to a flat latitudinal temperature gradient in the North Atlantic during this time interval, that might also indicate a proto-North Atlantic Current (Super et al., 2020). Reconstructed temperatures from the Netherlands, based on branched glycerol dialkyl glycerol tetraethers also show, probably, warm month temperatures of 21.5–25°C (Donders et al., 2009; Wang et al., 2018; Crampton-Flood et al., 2020).
Broader implications and future work
The new fungal assemblage from the Kenslow Member contains two genera with multiple species that facilitates dating constraints on the phylogenetic studies of these. In the phylogeny of Voglmayr et al. (2019) the presence of two species of Juglanconis identified from the Kenslow Member of Bees Nest Pit shows Juglanconis had evolved by this point. There was significant diversification of the Juglans clade during the Miocene (Mu et al., 2020) and Juglans pollen is reported from the same section at Bees Nest Pit (McCoy et al., in review). The presence of both the fungus and the host could be showing co-evolution over the course of the Cenozoic and would be worthwhile investigating fossil Juglanconis co-occurrence with Juglans during earlier time intervals. Species of Rosellinia diverged from those of Kretzschmaria during the Paleogene at around 50 million years ago (Samarakoon et al., 2016). The presence of Rosellinia aff. franciscae and R. aff. necatrix in the 12 Ma Kenslow Member of Bees Nest Pit shows these had originated by this time (Table 1). In the undated phylogeny of Bahl et al. (2005) these two species are on distinct branches, which suggests that this radiation had occurred earlier than the late Middle Miocene.
The presence of Pesavis tagluensis in sediments from the Bees Nest Pit extends the stratigraphic range of this taxon significantly. Pesavis tagluensis is an accepted biostratigraphical indicator for the Maastrichtian to Paleogene (Kalgutkar and Sweet, 1988). This fungal remain has a range of known sizes, with smaller forms (averaging 32 μm) known from the Maastrichtian through middle Paleocene, and larger forms more frequently found in the late Paleocene to Eocene. The species of P. tagluensis from the Kenslow Member is 43.5 μm tall × 38.3 μm wide, placing it in the middle of the range (21–60 μm), congruent with middle-sized Paleocene and Eocene specimens (Kalgutkar and Sweet, 1988). In the United Kingdom, Pesavis has been found previously in the Ardtun Leaf Beds on the Isle of Mull (Selandian), the Reading Beds in Berkshire (Thanetian-earliest Ypresian) and Hordle Cliff in Hampshire (Priabonian) (Smith, 1978; Smith and Crane, 1979; King, 2016; Arting et al., 2018). No other taxon present in the Kenslow Member is suggestive of a Paleogene age; indeed, paleobotanical and plant palynological remains clearly suggest that it is Serravallian (Pound and Riding, 2016), therefore, the range of Pesavis is greater than previously known, extending to at least 12 Ma. Frederiksen et al. (1996) noted that Pesavis in Alaska is associated with wood-rich horizons; it likewise occurs in a wood-rich zone in the Kenslow Member. The Oligocene – Miocene palynology of the United Kingdom is underexplored; it is strongly suggested that fungal palynology be completed as part of all future studies to refine the distribution and ecology of this intriguing group.
Conclusion
A total of 110 unique fossil fungal palynomorph morphotypes were recorded from the Kenslow Member as exposed in Bees Nest Pit, Brassington, Derbyshire, United Kingdom. Samples were co-dominated by amerospores and phragmospores, of which 72 (roughly 2/3) have been identified to extant taxa (Table 1). The majority of taxa encountered occur in association with wood-rich horizons. Neither sediment type nor processing method (mineral-acid vs. acid free) had an appreciable impact on fungal recovery.
Fossil fungal assemblages work for a palaeoclimate proxy. The assemblages throughout the Kenslow Member as exposed at Bees Nest Pit are indicative of deposition in Köppen–Geiger class Cfb – temperate, no dry season, warm summer. The results compare well to previously published quantitative paleobotanical and palynological climate reconstruction for the well-studied Kenslow Member, and together they are suggestive of the upper end of the Cfb climate range. Of note, it is clear from this study that the Serravallian of the United Kingdom was unlikely to be seasonally dry.
This study represents a first step in the utilization of fossil fungal remains as palaeoclimate proxies in the Neogene and lays the foundation for continued work in this area.
Data availability statement
The datasets presented in this study can be found in online repositories. The names of the repository/repositories and accession number(s) can be found below: https://doi.org/10.5281/zenodo.6558518.
Author contributions
MP and JO’K conceived the idea for and designed the study. MP, JO’K, ML, and JR completed the fieldwork and sample collection for the study. JR and MP provided the background information for the study. JO’K and MP generated the data for the study. NN, JO’K, IR, and MP completed the taxonomic identifications. MP developed the NLR technique and determined ecological tolerances and climatological zonations. MP and JO’K completed the data analysis with support of IR. MP and JO’K drafted the figures. MP and JO’K equally led the initial writing with support of NN and contributions from IR and JR. MP, JO’K, and IR led the manuscript editing with supporting editing by all other authors. All authors contributed to the article and approved the submitted version.
Funding
This study was funded by a Geological Society of London William George Fearnsides and Elspeth Matthews funds. NN was in-part supported through a Fulbright Visiting Scholar Award. MP acknowledges funding from The Mathematical Biosciences Institute, Ohio State University, which facilitated the writing of this manuscript. Final data analyses and manuscript revision were completed in the course of work on the joint NSF/Geo- NERC funded project “Fungi in a Warmer World (FiaWW)” (NSF award #2015813 to JO’K and NERC award identifier NE/V01501X/1 to MP).
Acknowledgments
We are grateful to Jayne Spencer, owner of Bees Nest Pit, and Natural England for facilitating access to this site. Maggie Stephenson is thanked for providing JO’K and MP with higher resolution photomicrographs of unusual taxa in 2017 while both were away from their laboratories. The Fuzzy Duck and CoffeeTree Books, Morehead, Kentucky is kindly thanked for fueling the final push to submit the manuscript. We are grateful to the interlibrary loan offices at Morehead State University, especially to Ms. Stacy Scott, and Northumbria University for facilitating rapid loans of primary mycology literature. Initial taxonomic treatment of fossil material was completed with the aid of multiple visits to the John Williams Index of Palaeopalynology (JWIP), housed at the Natural History Museum (London) and Dr. Stephen Stukins. JR publishes with the approval of the Executive Director, British Geological Survey (NERC). We thank EM and AMM for their invaluable comments that have greatly improved the manuscript. IT is thanked for their support and guidance throughout the process.
Conflict of interest
The authors declare that the research was conducted in the absence of any commercial or financial relationships that could be construed as a potential conflict of interest.
Publisher’s note
All claims expressed in this article are solely those of the authors and do not necessarily represent those of their affiliated organizations, or those of the publisher, the editors and the reviewers. Any product that may be evaluated in this article, or claim that may be made by its manufacturer, is not guaranteed or endorsed by the publisher.
Supplementary material
The Supplementary Material for this article can be found online at: https://www.frontiersin.org/articles/10.3389/fevo.2022.947623/full#supplementary-material
Supplementary table 1 | Sample information.
Supplementary table 2 | Descriptions of BN taxa.
Footnotes
References
Arting, U., Condon, D., Riding, J. B., and Riishuus, M. S. (2018). Revised Chronostratigraphy of the Faroe Islands Basalt Group and the British Palaeogene Igneous Province: Implications for Selandan-Thanetian Palynofloral Assemblages and Correlation with the Faroe-Shetland Basin. Nottingham: British Geological Survey.
Bahl, J., Jeewon, R., and Hyde, K. D. (2005). Phylogeny of Rosellinia capetribulensis sp. nov. and its allies (Xylariaceae). Mycologia 97, 1102–1110. doi: 10.3852/mycologia.97.5.1102
Beck, H. E., Zimmermann, N. E., McVicar, T. R., Vergopolan, N., Berg, A., and Wood, E. F. (2018). Present and future Köppen-Geiger climate classification maps at 1-km resolution. Sci. Data 5:180214. doi: 10.1038/sdata.2018.214
Boulter, M. C., and Chaloner, W. G. (1970). Neogene fossil plants from Derbyshire (England). Rev. Palaeobot. Palynol. 10, 61–78. doi: 10.1016/0034-6667(70)90022-9
Boulter, M. C., Ford, T. D., Ijtaba, M., and Walsh, P. T. (1971). Brassington Formation: a newly recognised Tertiary formation in the southern Pennines. Nat. Phys. Sci. 231, 134–136. doi: 10.1038/physci231134a0
Burls, N. J., Bradshaw, C. D., De Boer, A. M., Herold, N., Huber, M., Pound, M., et al. (2021). Simulating Miocene warmth: insights from an opportunistic multi-model ensemble (MioMIP1). Paleoceanogr. Paleoclimatol. 36:e2020A004054. doi: 10.1029/2020PA004054
Caffrey, M. A., and Horn, S. P. (2013). The use of lithium heteropolytungstate in the heavy liquid separation of samples which are sparse in pollen. Palynology 37, 143–150. doi: 10.1080/01916122.2012.736417
Clarke, K. R., Somerfield, P. J., and Gorley, R. N. (2008). Testing of null hypotheses in exploratory community analyses: similarity profiles and biota-environment linkage. J. Exp. Mar. Biol. Ecol. 366, 56–69. doi: 10.1016/j.jembe.2008.07.009
Clarke, K. R., and Warwick, R. M. (2001). Change in Marine Communities: an Approach to Statistical Analysis and Interpretation, 2nd Edn. Plymouth: PRIMER –E.
Classen, A. T., Sundqvist, M. K., Henning, J. A., Newman, G. S., Moore, J. A. M., Cregger, M. A., et al. (2015). Direct and indirect effects of climate change on soil microbial and soil microbial-plant interactions: what lies ahead? Ecosphere 6:art130. doi: 10.1890/ES15-00217.1
Crampton-Flood, E. D., Tierney, J. E., Peterse, F., Kirkels, F. M., and Sinninghe Damsté, J. S. (2020). BayMBT: a Bayesian calibration model for branched glycerol dialkyl glycerol tetraethers in soils and peats. Geochim. Cosmochim. Acta 268, 142–159. doi: 10.1016/j.gca.2019.09.043
Delgado-Baquerizo, M., Guerra, C. A., Cano-Díaz, C., Egidi, E., Wang, J. T., Eisenhauer, N., et al. (2020). The proportion of soil-borne pathogens increases with warming at the global scale. Nat. Clim. Change 10, 550–554. doi: 10.1038/s41558-020-0759-3
Denk, T., Grimm, G. W., Grímsson, F., and Zetter, R. (2013). Evidence from “Köppen signatures” of fossil plant assemblages for effective heat transport of Gulf Stream to subarctic North Atlantic during Miocene cooling. Biogeosciences 10, 7927–7942. doi: 10.5194/bg-10-7927-2013
Donders, T. H., Weijers, J. W. H., Munsterman, D. K., Kloosterboer-van Hoeve, M. L., Buckles, L. K., Pancost, R. D., et al. (2009). Strong climate coupling of terrestrial and marine environments in the Miocene of northwest Europe. Earth Planetary Sci. Lett. 281, 215–225. doi: 10.1016/j.epsl.2009.02.034
Ellis, M. B., and Ellis, J. P. (1997). Microfungi on Land Plants: an Identification Handbook. Slough: Richmond Publishing.
Ellis, M. B., and Ellis, J. P. (1998). Microfungi on Miscellaneous Surfaces: an Identification Handbook. Slough: Richmond Publishing.
Fick, S. E., and Hijmans, R. J. (2017). WorldClim 2: new 1-km spatial resolution climate surfaces for global land areas. Int. J. Climatol. 37, 4302–4315. doi: 10.1002/joc.5086
Frederiksen, N. O., Sheehan, T. P., Ager, T. A., Collett, T. S., Fouch, T. D., Franczyk, K. J., et al. (1996). Palynomorph biostratigraphy of Upper Cretaceous to Eocene samples from the Sagavanirktok Formation in its type region, North Slope of Alaska. U. S. Geol. Surv. Open 96-84, 1–44. doi: 10.3133/ofr9684
Gibson, M. E., McCoy, J., O’Keefe, J. M. K., Nuñez Otaño, N. B., Warny, S., and Pound, M. J. (2022). Reconstructing terrestrial paleoclimates: a comparison of the co-existence approach, Bayesian and probability reconstruction techniques using the UK Neogene. Paleoceanogr. Paleoclimatol. 37:e2021A004358. doi: 10.1029/2021PA004358
Guarro, J. P., Gené, J., Stchigel, A. M., and Figueras, M. J. (2012). Atlas of soil ascomycetes. CBS-KNAW fungal biodiversity centre, Utrecht, the Netherlands. CBS Biodivers. Ser. 10:486.
Henrot, A. J., Utescher, T., Erdei, B., Dury, M., Hamon, N., Ramstein, G., et al. (2017). Middle Miocene climate and vegetation models and their validation with proxy data. Palaeogeogr. Palaeoclimatol. Palaeoecol. 467, 95–119. doi: 10.1016/j.palaeo.2016.05.026
Herbert, T. D., Lawrence, K. T., Tzanova, A., Peterson, L. C., Caballero-Gill, R., and Kelly, C. S. (2016). Late Miocene global cooling and the rise of modern ecosystems. Nat. Geosci. 9, 843–847. doi: 10.1038/ngeo2813
Holbourn, A. E., Kuhnt, W., Clemens, S. C., Kochhann, K. G. D., Jöhnck, J., Lübbers, J., et al. (2018). Late Miocene climate cooling and intensification of Southeast Asian winter monsoon. Nat. Commun. 9:1584. doi: 10.1038/s41467-018-03950-1
Hyde, K. D., Fryar, S., Tian, Q., Bahkali, A. H., and Xu, J. (2016). Lignicolous freshwater fungi along a north–south latitudinal gradient in the Asian/Australian region; can we predict the impact of global warming on biodiversity and function? Fungal Ecol. 19, 190–200. doi: 10.1016/j.funeco.2015.07.002
Kalgutkar, R. M., and Sweet, A. R. (1988). Morphology, taxonomy and phylogeny of the fossil fungal genus Pesavis from Northwestern Canada. Geol. Surv. Canada Bull. 379, 117–133. doi: 10.4095/126976
King, C. (2016). A Revised Correlation of Tertiary Rocks in the British Isles and Adjacent Areas of NW Europe. London: Geological Society of London. doi: 10.1144/SR27
McCoy, J. L., Barrass-Barker, T., Hocking, E. P., O’Keefe, J. M. K., Riding, J. B., and Pound, M. J. (in review). Wetland development in the uppermost Brassington Formation (Middle Miocene) of Derbyshire, central England. Palaeogeogr. Palaeoecol. Palaeoclimatol. doi: 10.31223/X5406X
Melillo, J. M., Frey, S. D., DeAngelis, K. M., Werner, W. J., Bernard, M. J., Bowles, F. P., et al. (2017). Long-term pattern and magnitude of soil carbon feedback to the climate system in a warming world. Science 358, 101–105. doi: 10.1126/science.aan2874
Mu, X. Y., Tong, L., Sun, M., Zhu, Y. X., Wen, J., Lin, Q. W., et al. (2020). Phylogeny and divergence time estimation of the walnut family (Juglandaceae) based on nuclear RAD-Seq and chloroplast genome data. Mol. Phylogenet. Evol. 147:106802. doi: 10.1016/j.ympev.2020.106802
Nuñez Otaño, N. B., Bianchinotti, M. V., and Saparrat, M. C. N. (2021). “Palaeomycology: a modern mycological view on fungal palynomorphs,” in Applications of Non-Pollen Palynomorphs: from Palaeoenvironmental Reconstructions to Biostratigraphy, eds F. Marret, J. M. K. O’Keefe, P. Osterloff, M. J. Pound, and L. Shumilovskikh (London: Geological Society of London). doi: 10.1144/SP511-2020-47
O’Keefe, J. M. K., and Eble, C. F. (2012). A comparison of HF-based and non-HF-based palynology processing techniques in clay-rich lignites from the Claiborne Group, upper Mississippi Embayment, United States. Palynology 36, 116–130. doi: 10.2307/41515104
O’Keefe, J. M. K., Nuñez Otaño, N. B., and Bianchinotti, M. V. (2021). “Nomenclature: how do we designate NPP taxa?,” in Applications of Non-Pollen Palynomorphs: from Palaeoenvironmental Reconstructions to Biostratigraphy, eds F. Marret, J. M. K. O’Keefe, P. Osterloff, M. J. Pound, and L. Shumilovskikh (London: Geological Society of London), 77–89.
O’Keefe, J. M. K., Pound, M. J., Riding, J. B., and Vane, C. H. (2020). Cellular preservation and maceral development in lignite and wood from the Brassington Formation (Miocene), Derbyshire, UK. Int. J. Coal Geol. 222:103452. doi: 10.1016/j.coal.2020.103452
Peel, M. C., Finlayson, B. L., and McMahon, T. A. (2007). Updated world map of the Köppen-Geiger climate classification. Hydrol. Earth Syst. Sci. 11, 1633–1644. doi: 10.5194/hess-11-1633-2007
Põlme, S., Abarenkov, K., Henrik Nilsson, R., Lindahl, B. D., Clemmensen, K. E., Kauserud, H., et al. (2020). FungalTraits: a user-friendly traits database of fungi and fungus-like stramenopiles. Fungal Divers. 105, 1-16. doi: 10.1007/s13225-020-00466-2
Pound, M. J., Haywood, A. M., Salzmann, U., and Riding, J. B. (2012a). Global vegetation dynamics and latitudinal temperature gradients during the Mid to Late Miocene (15.97–5.33Ma). Earth Sci. Rev. 112, 1–22. doi: 10.1016/j.earscirev.2012.02.005
Pound, M. J., Riding, J. B., Donders, T. H., and Daskova, J. (2012b). The palynostratigraphy of the Brassington Formation (Upper Miocene) of the southern Pennines, central England. Palynology 36, 26–37. doi: 10.1080/01916122.2011.643066
Pound, M. J., Nuñez Otaño, N. B., Romero, I. C., Lim, M., Riding, J. B., and O’Keefe, J. M. K. (2022). The fungal ecology of the Brassington Formation (Middle Miocene) of Derbyshire, UK, and a new method for palaeoclimate reconstruction. Dataset Zenodo doi: 10.5281/zenodo.6558518
Pound, M. J., O’Keefe, J. M. K., and Marret, F. (2021). “An overview of techniques applied to the extraction of non-pollen palynomorphs, their known taphonomic issues and recommendations to maximize recovery,” in Applications of Non-Pollen Palynomorphs: from Palaeoenvironmental Reconstructions to Biostratigraphy, eds F. Marret, J. O’Keefe, P. Osterloff, M. Pound, and L. Shumilovskikh (London: Geological Society of London), 63–76.
Pound, M. J., O’Keefe, J. M. K., Nuñez Otaño, N. B., and Riding, J. B. (2019). Three new Miocene fungal palynomorphs from the Brassington Formation, Derbyshire, UK. Palynology 43, 596–607. doi: 10.1080/01916122.2018.1473300
Pound, M. J., and Riding, J. B. (2016). Palaeoenvironment, palaeoclimate and age of the Brassington Formation (Miocene) of Derbyshire, UK. J. Geol. Soc. 173, 306–319. doi: 10.1144/jgs2015-050
Richardson, M. J. (2001). Diversity and occurrence of coprophilous fungi. Mycol. Res. 105, 387–402. doi: 10.1017/S0953756201003884
Riding, J. B. (2021). A guide to preparation protocols in palynology. Palynology 45, 1–110. doi: 10.1080/01916122.2021.1878305
Riding, J. B., Pound, M. P., Hill, T. C. B., Stukins, S., and Feist-Burkhardt, S. (2012). The John Williams Index of Palaeopalynology. Palynology 36, 224–233. doi: 10.1080/01916122.2012.682512
Romero, I. C., Otaño, N. B. N., Gibson, M. E., Spears, T. M., Fairchild, C. J., Tarlton, L., et al. (2021). First record of fungal diversity in the tropical and warm-temperate Middle Miocene Climate Optimum forests of Eurasia. Front. For. Glob. Change 4, 768405. doi: 10.3389/ffgc.2021.768405
Samarakoon, M. C., Hyde, K. D., Promputtha, I., Hongsanan, S., Ariyawansa, H. A., Maharachchikumbura, S. S. N., et al. (2016). Evolution of Xylariomycetidae (Ascomycota: Sordariomycetes). Mycosphere 7, 1746–1761. doi: 10.5943/mycosphere/7/11/9
Schneck, F., and Melo, A. S. (2010). Reliable sample sizes for estimating similarity among macroinvertebrate assemblages in tropical streams. Ann. Limnol. 46, 93–100. doi: 10.1051/limn/2010013
Seifert, K. A., Morgan-Jones, G., Gams, W., and Kendrick, B. (2011). The Genera of Hyphomycetes. Utrecht: CBS-KNAW Fungal Biodiversity Centre.
Shumilovskikh, L. S., Shumilovskikh, E. S., Schlütz, F., and van Geel, B. (2022). NPP-ID: Non-Pollen Palynomorph Image Database as a research and educational platform. Veg. Hist. Archaeobot. 31, 323–328. doi: 10.1007/s00334-021-00849-8
Smith, A. (2013). Digital Geological Map of Great Britain, Information Notes, 2013. Nottingham: British Geological Survey, 1–50.
Smith, P. H. (1978). Fungal spores of the genus Ctenosporites from the early Tertiary of Southern England. Palaeontology 21, 717–722.
Smith, P. H., and Crane, P. R. (1979). Fungal spores of the genus Pesavis from the Lower Tertiary of Britain. Bot. J. Linn. Soc. 79, 243–248.
Steidinger, B. S., Bhatnagar, J. M., Vilgalys, R., Taylor, J. W., Qin, C., Zhu, K., et al. (2020). Ectomycorrhizal fungal diversity predicted to substantially decline due to climate changes in North American Pinaceae forests. J. Biogeogr. 47, 772–782. doi: 10.1111/jbi.13802
Steinthorsdottir, M., Coxall, H. K., de Boer, A. M., Huber, M., Barbolini, N., Bradshaw, C. D., et al. (2021). The Miocene: the future of the past. Paleoceanogr. Paleoclimatol. 36:e2020A004037. doi: 10.1029/2020PA004037
Super, J. R., Thomas, E., Pagani, M., Huber, M., O’Brien, C. L., and Hull, P. M. (2020). Miocene evolution of North Atlantic Sea surface temperature. Paleoceanogr. Paleoclimatol. 35:e2019A003748. doi: 10.1029/2019PA003748
Thompson, R. S., Anderson, K. H., Pelltier, R. T., Strickland, L. E., Bartlein, P. J., and Shafer, S. L. (2012). Quantitative estimation of climatic parameters from vegetation data in North America by the mutual climatic range technique. Quat. Sci. Rev. 51, 18–39. doi: 10.1016/j.quascirev.2012.07.003
Utescher, T., Bondarenko, O. V., and Mosbrugger, V. (2015). The Cenozoic cooling – continental signals from the Atlantic and Pacific side of Eurasia. Earth Planet. Sci. Lett. 415, 121–133. doi: 10.1016/j.epsl.2015.01.019
Utescher, T., Bruch, A. A., Erdei, B., François, L., Ivanov, D., Jacques, F. M. B., et al. (2014). The Coexistence Approach—theoretical background and practical considerations of using plant fossils for climate quantification. Palaeogeogr. Palaeoclimatol. Palaeoecol. 410, 58–73. doi: 10.1016/j.palaeo.2014.05.031
Větrovský, T., Kohout, P., Kopecký, M., Machac, A., Man, M., Bahnmann, B. D., et al. (2019). A meta-analysis of global fungal distribution reveals climate-driven patterns. Nat. Commun. 10:5142. doi: 10.1038/s41467-019-13164-8
Voglmayr, H., Jaklitsch, W. M., Mohammadi, H., and Kazemzadeh Chakusary, M. (2019). The genus Juglanconis (Diaporthales) on Pterocarya. Mycol. Prog. 18, 425–437. doi: 10.1007/s11557-018-01464-0
Walsh, P. T., Atkinson, K., Boulter, M. C., and Shakesby, R. A. (1987). The Oligocene and Miocene outliers of West Cornwall and their bearing on the geomorphological evolution of oldland Britain. Philos. Trans. R. Soc. Lond. A Math. Phys. Sci. 323, 211–245. doi: 10.1098/rsta.1987.0082
Walsh, P. T., Banks, V. J., Jones, P. F., Pound, M. J., and Riding, J. B. (2018). A reassessment of the Brassington Formation (Miocene) of Derbyshire, UK and a review of related hypogene karst suffosion processes. J. Geol. Soc. 175, 443–463. doi: 10.1144/jgs2017-029
Walsh, P. T., Boulter, M. C., Ijtaba, M., and Urbani, D. M. (1972). The preservation of the Neogene Brassington Formation of the southern Pennines and its bearing on the evolution of Upland Britain. J. Geol. Soc. 128, 519–559. doi: 10.1144/gsjgs.128.6.0519
Walsh, P. T., Morawiecka, I., and Skawinaska-Wieser, K. (1996). A Miocene palynoflora preserved by karstic subsidence in Anglesey and the origin of the Menaian Surface. Geol. Mag. 133, 713–719. doi: 10.1017/S0016756800024560
Wang, M., Zong, Y., Zheng, Z., Man, M., Hu, J., and Tian, L. (2018). Utility of brGDGTs as temperature and precipitation proxies in subtropical China. Sci. Rep. 8:194. doi: 10.1038/s41598-017-17964-0
Keywords: wetlands, fungi, biodiversity, nearest living relative, palynology, climate change
Citation: Pound MJ, Nuñez Otaño NB, Romero IC, Lim M, Riding JB and O’Keefe JMK (2022) The fungal ecology of the Brassington Formation (Middle Miocene) of Derbyshire, United Kingdom, and a new method for palaeoclimate reconstruction. Front. Ecol. Evol. 10:947623. doi: 10.3389/fevo.2022.947623
Received: 18 May 2022; Accepted: 06 July 2022;
Published: 04 August 2022.
Edited by:
Irene Tunno, Lawrence Livermore National Laboratory (DOE), United StatesReviewed by:
Anna Maria Mercuri, University of Modena and Reggio Emilia, ItalyEncarni Montoya, University of Liverpool, United Kingdom
Copyright © 2022 Pound, Nuñez Otaño, Romero, Lim, Riding and O’Keefe. This is an open-access article distributed under the terms of the Creative Commons Attribution License (CC BY). The use, distribution or reproduction in other forums is permitted, provided the original author(s) and the copyright owner(s) are credited and that the original publication in this journal is cited, in accordance with accepted academic practice. No use, distribution or reproduction is permitted which does not comply with these terms.
*Correspondence: Matthew J. Pound, bWF0dGhldy5wb3VuZEBub3J0aHVtYnJpYS5hYy51aw==; Jennifer M. K. O’Keefe, ai5va2VlZmVAbW9yZWhlYWRzdGF0ZS5lZHU=
†These authors share first authorship