- 1College of Grassland Agriculture, Northwest A&F University, Yangling, Shaanxi, China
- 2Department of Biology and Geology, University of South Carolina, Aiken, SC, United States
- 3Department of Plant and Environmental Sciences, Edisto Research and Education Center, Clemson University, Blackville, SC, United States
- 4Department of Horticulture, Faculty of Crop and Food Sciences, PMAS Arid Agriculture University, Rawalpindi, Pakistan
Global warming negatively affects plant growth due to the detrimental effects of high temperature-induced heat stress. Rhizobium inoculation (RI) and exogenous melatonin (MT) have shown a positive role in resisting abiotic stress. However, their synergistic effect on avoiding heat-induced damages in Medicago truncatula has not been studied yet. Hence, the objective of the present study was to evaluate the impact of these amendments (RI and MT) to ameliorate the heat damages in Medicago truncatula. The study was comprised of two factors: (1) heat-induced stress: (i) optimum temperature (26 ± 1°C): (23 ± 1°C) (day: night), (ii) moderate heat (35 ± 1°C): (28 ± 1°C), and (iii) severe heat (41 ± 1°C): (35 ± 1°C) for 72 h, and (2) amendments: (i) no RI + no MT (NRI + NMT), (ii) Rhizobium inoculation (RI), (iii) 60 μM melatonin (MT), and (iii) RI + MT. Results showed that the combined application of RI and MT was better than their individual applications, as it prevented heat-induced membrane damages by declining the hydrogen peroxide (34.22% and 29.78%), superoxide anion radical (29.49% and 26.71%), malondialdehyde contents (26.43% and 21.96%), and lipoxygenase activity (44.75% and 25.51%) at both heat stress levels as compared to NRI + NMT. Moreover, RI + MT treated plants showed higher antioxidative and methylglyoxal detoxification enzymes (Gly I and Gly II) activities under heat stress. While, NRI + NMT treated plants showed a higher level of methylglyoxal contents (47.99% and 46.71%) under both levels of heat stress. Relative to NRI + NMT plants, RI + MT pretreated plants exhibited improved heat tolerance as indicated by higher chlorophyll (37.42% and 43.52%), carotenoid contents (32.41% and 47.08%), and photosynthetic rate (42.62% and 64.63%), under moderate and severe heat stress, respectively. Furthermore, RI + MT pretreated plants had considerably higher indole-3 acetic acid and abscisic acid concentrations under moderate (54.02% and 53.92%) and severe (68.36% and 64.61%) heat stress conditions. Similarly, plant dry biomass, NPK uptake, nitric oxide, and nitrate reductase activity were high in RI + MT treated plants, under both levels of stress. Therefore, this study advocates the positive synergistic effect of RI and MT pretreatment against moderate and severe heat-induced stress and for possible maintenance of plant growth under changing scenarios of global warming.
Introduction
Global air temperature is expected to rise at the rate of 0.2°C per decade, which will lead to temperatures of 1.8–4.0°C higher than the current level by 2100 (IPCC, 2007). The global warming-driven rise in temperature has adverse effects on plant growth, mainly due to harmful consequences of heat stress on plant development (Ohama et al., 2017). Rising concerns of heat stress threaten global food security due to its negative impacts on crop growth and productivity (Li et al., 2021; Moghaddam et al., 2021).
Temperatures exceeding the plant-specific optimal range may weaken photosynthetic capacity, leading to a decrease in the photosynthetic area, leaf photochemical efficiency, photosynthetic rate, and ultimately the yield decline in plants (Wassie et al., 2020). Several studies have reported that heat-induced stress damaged cell division, seed germination, root and stem growth, loss of leaf water content, and leaf senescence, resulting in lower plant growth and biomass production (Xu et al., 2016; Jahan et al., 2019). Plants exposed to high temperatures can elevate reactive oxygen species (ROS) accumulation, including hydrogen peroxide (H2O2), singlet oxygen (1O2), and superoxide radicals (), thereby causing damage to carbohydrates, lipids, proteins, and DNA that eventually lead to oxidative stress (Buttar et al., 2020).
Plants have developed over time-efficient various metabolic mechanisms against temperature-induced harmful effects of ROS (Hasanuzzaman et al., 2020). Among many possible strategies, one includes the accumulation of osmolyte that will enable the plant body to maintain cellular turgidity, organize cellular structures, stabilize the proteins, and improve the enzymatic and non-enzymatic antioxidant system to ameliorate the cellular redox balance and homeostasis in response to heat stress (Hasanuzzaman et al., 2020). Several management strategies like pre-treatment of stressed plants with chemicals, microorganisms, and plant hormones have been extensively applied in agriculture to improve stress tolerance in plants (Zhang et al., 2019; Buttar et al., 2020; Irshad et al., 2021a). Similarly, phytohormones including cytokinins, brassinosteroids, jasmonates, gibberellin, salicylic acid, indole-3 acetic acid (IAA), abscisic acid (ABA), and melatonin (MT) also have been reported to play a crucial role in the improvement of antioxidant defense system under abiotic stress thus keeping the damages to the barest minimum level (Wani et al., 2016; Li et al., 2018; Wassie et al., 2020).
Among many plant hormones, melatonin (N-acetyl-5-methoxytryptamine) has recently received ultimate attention owing to its defensive roles and contribution to mitigating various abiotic stresses caused by the sudden fluctuation in temperature (heat and cold), salinity, heavy metals, UV radiation, drought, and oxidative stress (Huang et al., 2019; Buttar et al., 2020; Zahedi et al., 2021). In addition, it has been reported that foliar treatment of MT induces seed germination, flowering, ripening, photosynthetic pigments, modulates antioxidant capacity, and leaf senescence, thus enhancing growth and yield in various crops (Xu et al., 2016; Zhang J. et al., 2017).
Plant growth-promoting rhizobacteria (PGPR) – found near the rhizosphere plane have been reported as a novel growth regulator with the potential to colonize and improve yield and productivity through biological N2 fixation (BNF) (Lindström and Mousavi, 2020). In recent times, PGPR has been reported to improve stress tolerance in several crop species, including but not limited to legumes (Liu et al., 2019; Chea et al., 2021). Similarly, Irshad et al. (2021a) reported that Rhizobium inoculation (RI) showed higher photosynthetic efficiency, better uptake of nutrients, and osmolytes accumulation in Medicago truncatula plants under cold stress. However, information on its response to ameliorate heat stress is still a question.
Legumes play an important role in addressing the world food security challenge and are a major component of managing soil health and fertility in sustainable agricultural systems. Among legumes, Medicago truncatula is an important model forage legume for breeding, genomics, and genetics due to its high nitrogen fixation capacity, self-fertility, diploid, and small genome size (Mollinedo et al., 2016). M. truncatula is widely used to feed livestock as fresh forage and hay in Mediterranean countries and in Australia and is central to ecology and evolution dynamics of forage species in grassland agricultural systems. The optimum growth temperature for M. truncatula ranges from 23 to 28°C with annual rainfall between 275 and 400 mm (Irshad et al., 2021b). The extreme temperature adversely affects the yield and productivity of legumes, including M. truncatula driven by a decrease in soil moisture content, and disruption in soil biogeochemical properties, which altogether negatively impact nutrients uptake, gaseous exchange, photosynthetic efficiency, and plant water relations (Farooq et al., 2017). Numerous studies have reported a severe loss of quality and productivity in many leguminous plants like Pisum sativum (Shahzad et al., 2015), Medicago sativa (Wassie et al., 2019), Glycine max (Campobenedetto et al., 2020), and Vicia faba (Siddiqui et al., 2015) as a result of heat-induced stress.
Individual treatment of RI or MT has been well documented for its positive role in ameliorating oxidative stress in various crops (Lee et al., 2020; Lindström and Mousavi, 2020; Alam et al., 2021; Chea et al., 2021), however, the effect of their combined application is still not clear. Therefore, it was hypothesized that RI and MT can induce thermotolerance against oxidative damage. To the best of our knowledge, the physiological responses of Medicago truncatula against heat stress have not been fully explored. This study sought to evaluate the physiological response of Medicago truncatula against heat-induced stress and the co-application of RI and MT on enhancing thermotolerance by improving antioxidant enzyme activities and scavenging ROS. Antioxidant enzymes, osmolytes, nitro-oxidative homeostasis, endogenous plant hormones, growth, and yield were comprehensively evaluated under heat stress with RI and MT.
Materials and methods
Seed germination and growth conditions
The experiments were conducted at the research facility of Northwest Agriculture and Forestry University (34°18′N, 108°05′E), located in Yangling, China. Medicago truncatula (Jemalong A-17) seeds with more than 90% germination rate were soaked in concentrated H2SO4 for 5-6 min, washed with distilled water, and surface-sterilized in sodium hypochlorite (5%) for 18-20 min for disinfection. The seeds were thoroughly rinsed five to six times using distilled water to remove the chemical residues. After sterilization, the seeds were kept to vernalization on wet filter papers in Petri dishes at 4°C under dark stage photosynthesis to break the seed dormancy. After 48 h, the dishes were transferred to a growth chamber and kept at 23°C for 48 h with relative humidity (RH) of 65-75% for seed germination.
Preliminary experiment
About 7-days before the induction of heat stress, 20 pots of 56-days-old seedlings were treated with variable melatonin (MT) concentrations for selecting the optimal melatonin concentration. The MT concentrations applied were as follows: No melatonin (C), 30 μM melatonin (30-MT), 60 μM melatonin (60-MT), and 90 μM melatonin (90-MT). Seedlings were exposed to (35 ± 1°C): (28 ± 1°C) (light: dark) for 72 h to induce heat stress. For the melatonin treatments, the pots were hand sprayed with 30 ml of 30 μM, 60 μM and 90 μM melatonin per pot, until the whole plants become wet. The non-melatonin treatments involved pretreatment with similar amounts of water. Each treatment contained five replications, and each replicate was comprised of 4 pots of seedlings. The time duration of 72 h was sufficient to induce heat stress as indicated through significant changes in plant dry biomass (g), electrolyte leakage (%), leaf photochemical efficiency (Fv/Fm), and relative water content (%), which were determined following the methods of Irshad et al. (2021a,b). The prolonged duration of heat stress was not chosen in order to avoid permanent wilting.
Main experimental design and treatments
For the main experiment with optimized amendments, young seedlings (five-days-old) were transplanted into a total of 60 pots (12 seedlings/pot) of size 30 × 45 × 10 cm containing 3.5 L Hoagland nutrient solutions (Hoagland and Arnon, 1950; recipe in Supplementary material) and were allowed to grow for 15 days. The nutrient solution was constantly ventilated and renewed at an interval of every two days consecutively until the end of the experiment. A total of 30 pots with uniform seedlings (15 days old) were allowed to grow naturally in Hoagland solution (containing nitrogen), while the remaining 30 pots were treated with Rhizobium meliloti (Dormal strain) in Hoagland solution (nitrogen-free) of 1/4 strength to allow nodule formation; since the presence of nitrogen hinder the nodule development. The seedlings were randomly arranged and subjected to a growth chamber by maintaining a 16:08 h light/dark cycle, (26 ± 1°C): (23 ± 1°C) day: night temperature cycle, with an RH of 65 ± 3%. The seedlings (15 days old) were randomly divided into four treatments: (1) no RI + no MT (NRI + NMT), (2) Rhizobium inoculation (RI); (3) foliar spray with 60 μM of melatonin (MT); and (4) RI + MT. All treatments were exposed to three temperature regimes for 72 h: (1) optimum temperature at (26 ± 1°C): (23 ± 1°C) (light: dark), (2) moderate heat at (35 ± 1°C): (28 ± 1°C), and (3) severe heat at (41 ± 1°C): (35 ± 1°C). Melatonin concentration of 60 μM with moderate heat at (35 ± 1°C): (28 ± 1°C) (light: dark) was based on the preliminary analysis and applied one week prior to heat stress induction (Supplementary Figure 1). There were five biological replicates for each treatment. After the completion of heat stress (72 h), seedlings were instantly harvested and exposed to liquid nitrogen and stored at -80 ± 1°C for further laboratory analyses.
Determination of chlorophyll contents, carotenoids contents, photosynthesis, and leaf photochemical efficiency (Fv/Fm)
For the determination of carotenoids and chlorophyll contents, 0.1 g of fresh plant tissues were macerated in 80% acetone using a sterilized pestle and mortar, followed by centrifugation at 12,300 × g for 11 min. Optical densities (480, 645, and 663 nm) were measured using a spectrophotometer (Irshad et al., 2021b). Leaf net photosynthesis was measured using Li-6400 (LI-COR Inc., USA). Seedlings were kept in the dark for 30 min to determine leaf photochemical efficiency (Fv/Fm) using a fluorometer (MINI version imaging PAM, Walz, Germany) (Irshad et al., 2021b). Measurements were repeated twice for each plant by taking two fully expanded leaves.
Evaluation of oxidative damage and antioxidant enzyme activities
For the evaluation of malondialdehyde content, the thiobarbituric acid method was used, and absorbance was measured at 532 and 600 nm (Irshad et al., 2021a). Hydrogen peroxide was measured by employing the potassium iodide method. For the quantification of H2O2, 0.1 g leaves were homogenized in 0.1% trichloroacetic acid and centrifuged at 11,500 × g for 13 min, and the supernatant was thoroughly mixed with buffer (potassium phosphate; pH 7.0) and potassium iodide (1 ml). After vigorously shaking, optical density was read at 390 nm (Wassie et al., 2019). Elstner’s method was used for the quantification of concentration and optical density was taken at 530 (Liu et al., 2019). Lipoxygenase activity was evaluated as described by Ahanger et al. (2019) with minor modifications. Linoleic acid (an essential fatty acid) was considered as a substrate of lipoxygenase enzyme and an increase in the absorbance was measured at 234 nm.
For the measurement of enzyme activities, 0.5 g of frozen tissues were macerated with potassium phosphate buffer (0.1 M; pH 7.8) in a pre-chilled pestle and mortar. The homogenate was centrifuged at a low temperature at 12,500 × g for 18 min and the final supernatant was further used for the evaluation of enzyme activities. For peroxidase (POD) activity, guaiacol (1-hydroxy-2-methoxybenzene, C7H8O2) was used as an electron donor (Buttar et al., 2020). Superoxide dismutase activity was calculated based on the ability to inhibit the reduction of nitroblue tetrazolium (NBT) by superoxide anion generated by the riboflavin system under 4000 W (light intensity) at 25°C (Liu et al., 2019). Catalase activity was estimated by measuring the conversion rate of H2O2 to water and oxygen molecules (Buttar et al., 2020). Ascorbate peroxidase activity was determined by the method of Ahanger et al. (2019) and H2O2-dependent ascorbate-oxidation was observed at 290 nm for 3 min.
Ascorbate content was determined by homogenizing 0.1 g of frozen leaves in trichloro-acetic acid (6%) and centrifuged at 13,500 × g for 2 min. The supernatant was thoroughly mixed with thiourea (10%) and dinitrophenyl hydrazine (2%). The mixture was incubated in water bath (18 min), followed by ice-cooling. After the samples were cooled, 5 ml of chilled 80% sulfuric acid was added and absorbance was measured at 530 nm (Ahanger and Agarwal, 2017). The estimation of reduced glutathione content was assessed according to Irshad et al. (2021b). Samples (0.1 g) were macerated in phosphate buffer solution (pH 8.0), and the supernatant was treated with 5, 5-dithiobis 2-nitrobenzoic acid, and absorbance was taken at 412 nm.
Measurement of the methylglyoxal detoxification system
Frozen leaf samples were macerated in perchloric acid (5.5%) and centrifuged at 11,200 × g. The supernatant was mixed with sodium dihydrogen phosphate and N-acetyl-L-cysteine, and the reaction mixture was incubated at room temperature for 10 min. Finally, absorbance was measured at 288 nm to determine the methylglyoxal (MG) content (Li et al., 2019). Enzymatic activities of Glyoxalase I and II (Gly I and Gly II) were measured by the methods of Li et al. (2017, 2019).
Determination of compatible solutes and nutrients uptake
Glycine betaine, proline, soluble proteins, and sugars were determined in powdered dry samples (Ahanger et al., 2019), and measurements were recorded at 365 nm, 520 nm, 595 nm, and 620 nm, respectively. Nitrogen contents were determined by using the Kjeldahl method, phosphorous by spectrophotometric method (Zhang R. et al., 2017), and potassium by a flame photometer (microflow analyzer III, Systea, Italy) (Ahanger et al., 2019).
Measurement of nitric oxide and nitrate reductase activity
Homogenates of frozen samples were prepared in a pre-chilled Na-acetate buffer having pH 3.6, following the method of Kaya et al. (2020). Activities of nitrate reductase (NR) were elucidated as suggested by Ahanger et al. (2021), and absorbance was measured at 540 nm.
Measurement of endogenous melatonin, abscisic acid, and indole acetic acid contents
Pape and Lüning method was used for the extraction of endogenous MT in acetone and methanol solution, according to Irshad et al. (2021b). Endogenous MT was calculated according to plant melatonin using an ELISA kit as per the recommendation of the manufacturer (Jiwei Biological Technology Co., Ltd., Shanghai, China). Abscisic acid and acetic acid levels were measured using an ELISA kit (Rapid bio, USA) according to the manufacturer’s instructions. Abscisic acid and acetic acid absorbance were taken at 270 nm using a microplate reader.
Statistical analyses
This experiment was carried out using a complete randomized design, while Tukey’s HSD test – a statistical model of evaluating analysis of variance (ANOVA) was used to test pairwise comparison of the treatments at p ≤ 0.05.
Results
Optimization of melatonin concentration for heat stress tolerance
Heat stress negatively impacted the growth of Medicago truncatula, however, exogenous MT application significantly improved plant dry biomass, relative water content (RWC), electrolyte leakage, and Fv/Fm. Compared to control, the MT applications at 30-MT, 60-MT, and 90-MT increased plant dry biomass and leaf photochemical efficiency by 5.16% and 3.11%, 11.89% and 6.47%, and 1.58% and 1.46%, respectively (Supplementary Figures 1A,B).
Moreover, a substantial increment of 7.60%, 65.07%, and 14.94% were observed in the electrolyte leakage at 30-MT, 60-MT and 90-MT treated plants, respectively. RWC remained consistent in all the treatments regardless of MT rates (Supplementary Figures 1C,D). Bearing in mind the above observation, this preliminary study thus recommended 60-MT as the optimal MT concentration for the investigation of Medicago truncatula heat-induced stress.
Oxidative damage
The generation of hydrogen peroxide (H2O2) was increased by 63.74% and 106.6%, and superoxide anion radical () by 48.63% and 79.45% in NRI + NMT plants under moderate and severe heat stress, thereby, resulting in enhancement of MDA content (31.87% and 50.51%) and lipoxygenase (LOX) activity (90.45% and 136.17%), respectively, compared to plants without stress (Figures 1A–D). On the other hand, RI and MT pretreated plants significantly (p ≤ 0.05) decreased H2O2, , MDA, and LOX contents under moderate and severe heat-stressed conditions. A significant decline was observed in H2O2 (34.23% and 29.79%), (29.49% and 26.72%), MDA (26.43% and 21.96%), and LOX (44.75% and 25.51%) contents in RI + MT treated plants when compared with NRI + NMT plants under moderate and severe heat stress conditions.
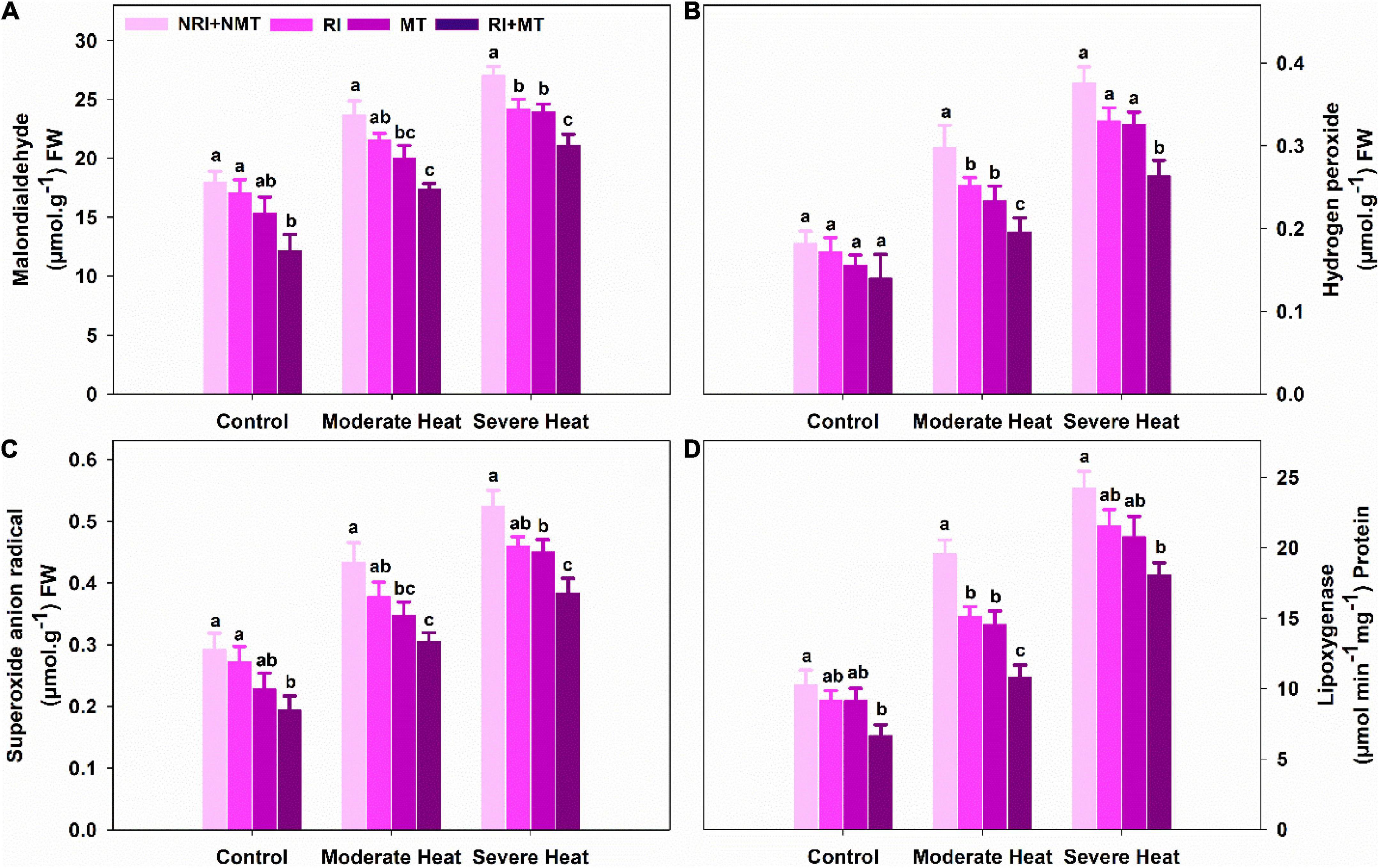
Figure 1. The individual and combined effects of Rhizobium inoculation and exogenous melatonin pretreatment on malondialdehyde (A), hydrogen peroxide (B), superoxide anion radical (C), and lipoxygenase activity (D) in Medicago truncatula under heat stress conditions. Control (26 ± 1°C): (23 ± 1°C) (light: dark), moderate heat (35 ± 1°C): (28 ± 1°C) (light: dark), severe heat (41 ± 1°C): (35 ± 1°C) (light: dark), NRI + NMT (no Rhizobium inoculation, no melatonin), RI (Rhizobium inoculation), MT (pretreated with 60 μM of melatonin), and (4) RI + MT (Rhizobium inoculation and pretreatment with 60 μM of melatonin).
Enzymatic and non-enzymatic antioxidant activities
Relative to control (NRI + NMT), the individual and co-application of RI and MT significantly enhanced the activities of superoxide dismutase (SOD), peroxidase (POD), catalase (CAT), and ascorbate peroxidase (APX) under moderate and severe heat stress (Figures 2A–D). Under control conditions, a significant increase of 14.77% 26.98%, 31.62%, and 30.2% were observed in SOD, POD, CAT, and APX activities, respectively, in RI + MT pretreated plants as compared to NRI + NMT plants. Similarly, the enzymatic activities of SOD, POD, CAT, and APX in NRI + NMT plants were reduced by 58.05%, 10.94%, 10.11%, and 8.96% under severe heat stress conditions, respectively. On the other hand, RI + MT pretreated plants substantially enhanced the enzymatic activities of SOD (36.28% and 14.19%), POD (35.39% and 32.2%), CAT (21.6% and 22.91%), and APX (36.83% and 55.41%) under moderate and severe heat stress as compared to NRI + NMT plants, respectively.
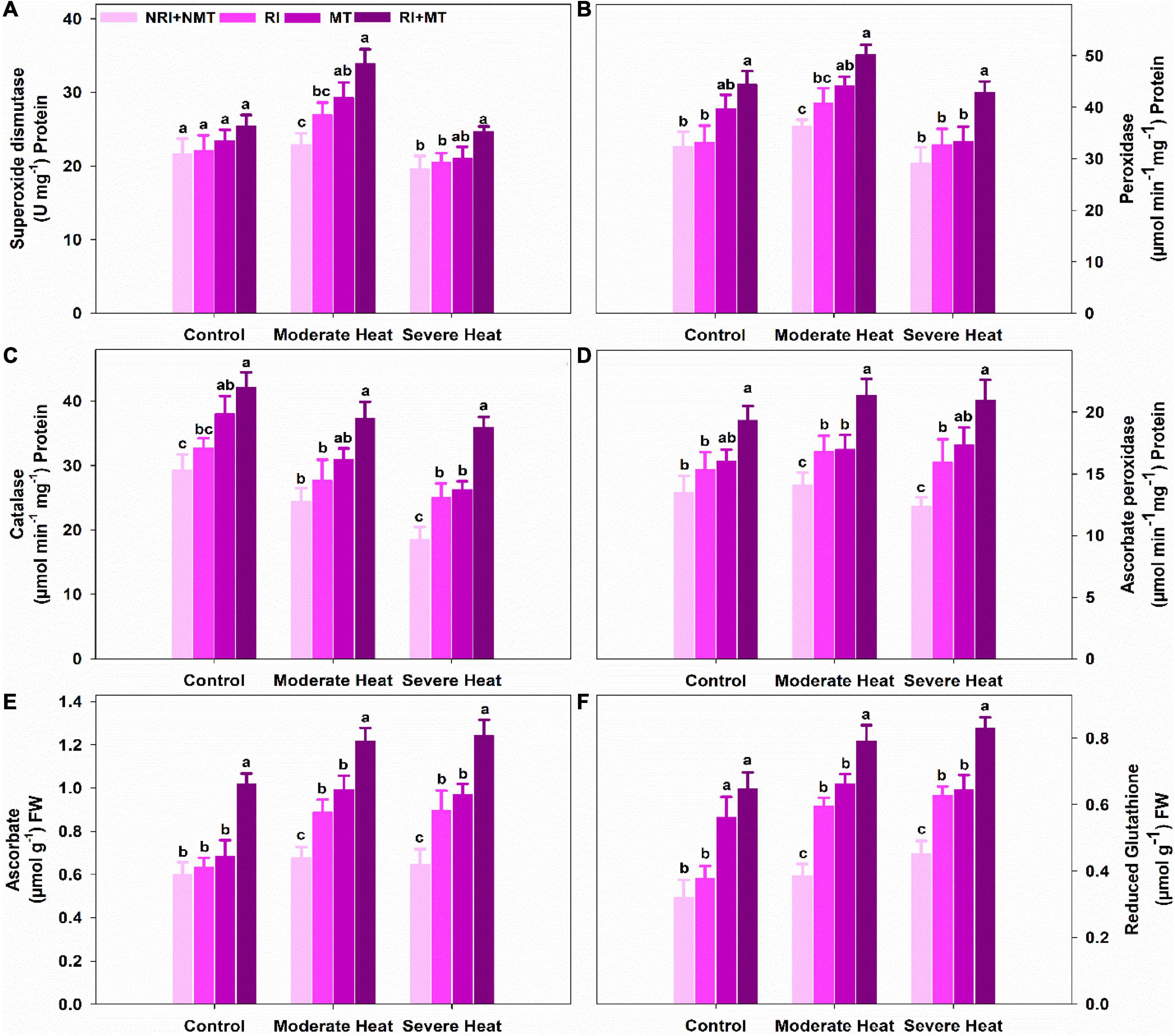
Figure 2. The individual and combined effects of Rhizobium inoculation and exogenous melatonin pretreatment on superoxide dismutase (A), peroxidase (B), catalase (C), ascorbate peroxidase (D), ascorbate (E), and reduced glutathione (F) activities in Medicago truncatula under heat stress conditions. Control (26 ± 1°C): (23 ± 1°C) (light: dark), moderate heat (35 ± 1°C): (28 ± 1°C) (light: dark), severe heat (41 ± 1°C): (35 ± 1°C) (light: dark), NRI + NMT (no Rhizobium inoculation, no melatonin), RI (Rhizobium inoculation), MT (pretreated with 60 μM of melatonin), and (4) RI + MT (Rhizobium inoculation and pretreatment with 60 μM of melatonin).
The ascorbate (AsA) and reduced glutathione (GSH) contents were significantly increased with RI and MT treatments as compared to NRI + NMT. The RI + MT pretreated plants showed maximum AsA and GSH contents which were 5.1− and 6.8-times higher when compared with unstressed NRI + NMT (Figures 2E,F).
Methylglyoxal detoxification system
Decreased activities of Gly I and Gly II were observed under moderate heat stress, while a record low activity was recorded when subjected to severe heat stress. Gly I and Gly II activities were significantly increased in heat-stressed plants supplemented with co-application RI and MT, as compared to control (NRI + NMT) heat-stressed plants (Figures 3A,B). Levels of methylglyoxal were increased by 23.11% and 36.29% following exposure to moderate and severe heat stress when compared with NRI + NMT plants. On the other hand, methylglyoxal levels decreased by 48% and 46.7% in moderate and severe stressed plants following the co-application of RI and MT (Figure 3C).
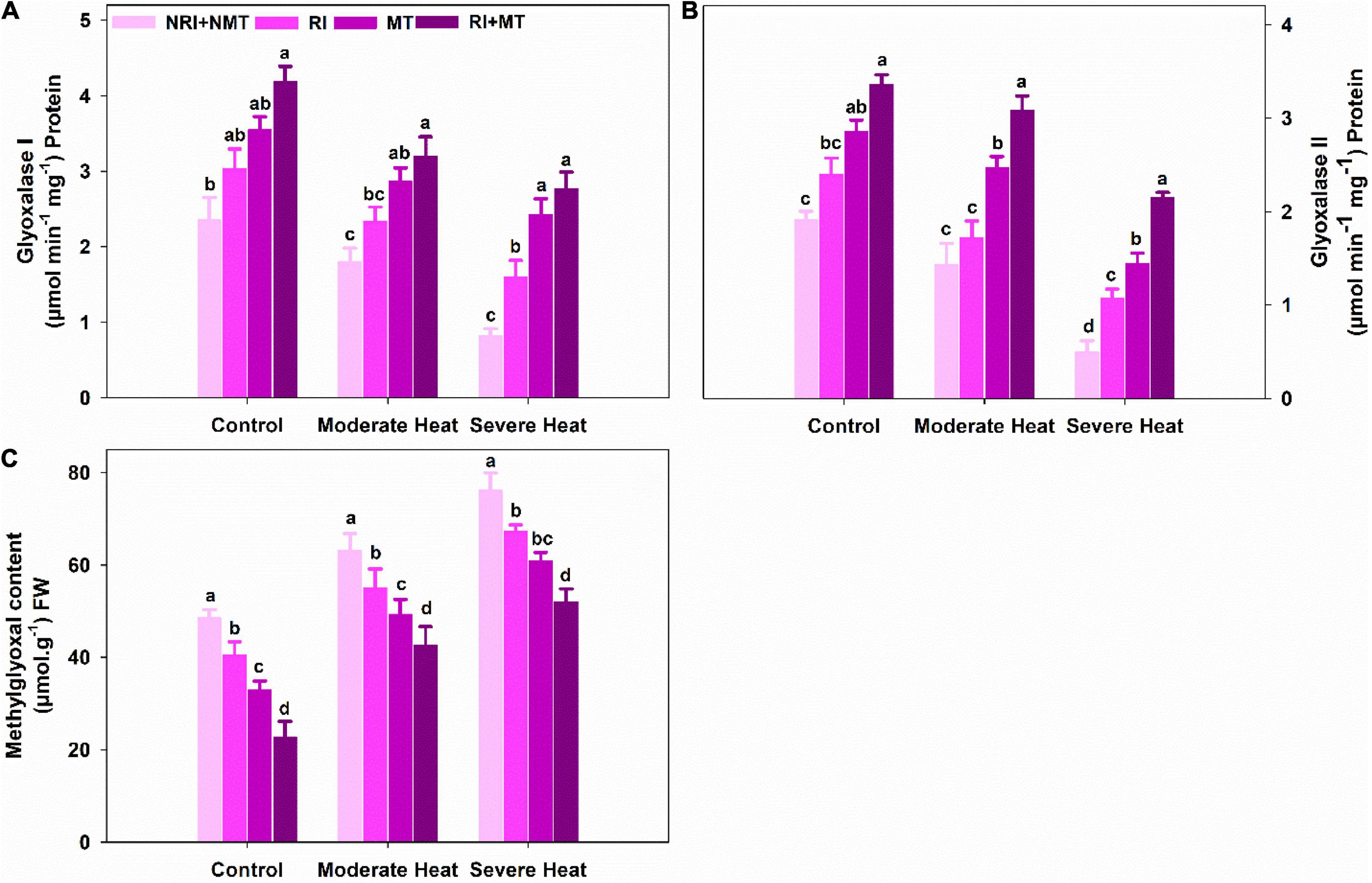
Figure 3. The individual and combined effects of Rhizobium inoculation and exogenous melatonin pretreatment on glyoxalase I (A), glyoxalase II (B), and methylglyoxal content (C) in Medicago truncatula under heat stress conditions. Control (26 ± 1°C): (23 ± 1°C) (light: dark), moderate heat (35 ± 1°C): (28 ± 1°C) (light: dark), severe heat (41 ± 1°C): (35 ± 1°C) (light: dark), NRI + NMT (no Rhizobium inoculation, no melatonin), RI (Rhizobium inoculation), MT (pretreated with 60 μM of melatonin), and (4) RI + MT (Rhizobium inoculation and pretreatment with 60 μM of melatonin).
Osmolytes accumulation
There was no significant difference between the NRI + NMT and RI-treated plants regarding the osmolyte accumulation under control conditions (Supplementary Table 1). In comparison with NRI + NMT plants, proline content was markedly increased by 31.3% in RI + MT treated plants under control conditions (Supplementary Table 1). Overall proline accumulation is affected by heat stress; however, it increased in RI + MT treated plants by 50.27% and 37.98% under moderate and severe heat stress as compared to NRI + NMT treated plants, respectively. Similarly, relative fluctuation in glycine betaine content exhibited an identical trend to proline, and a significant concentration loss was observed in NRI + NMT plants by 39.03% in comparison with RI + MT-treated plants exposed to severe heat stress conditions (Supplementary Table 1). The soluble sugar contents of RI + MT plants were significantly escalated by 51.23% and 36.7% under moderate and severe heat, respectively, as compared to NRI + NMT stressed plants. Likewise, a significant increase was observed in the contents of soluble protein of RI + MT-treated plants under control, moderate, and severe heat stress conditions (Supplementary Table 1).
Photosynthetic pigments and Photosystem II activity
Under control conditions, the co-application of RI and MT pretreatment significantly improved carotenoid and chlorophyll contents by 39.58% and 18.82% compared to NRI + NMT plants, respectively. RI and MT plants subjected to moderate and severe heat showed conspicuous carotenoid and chlorophyll contents due to the individual pretreatments of RI and MT. Meanwhile, RI + MT pretreated plants significantly mitigated the decline in carotenoid and chlorophyll contents under stressed conditions (Figures 4A,B). Photosynthesis rate declined in NRI + NMT plants under moderate and severe heat stress by 62.21% and 421.7% and was significantly increased in RI + MT treated plants by 6.93% and 84.54%, respectively, as compared to NRI + NMT plants (Figure 4C). RI + MT pretreated plants significantly enhanced photosystem II activity (Fv/Fm) relative to NRI + NMT plants and mitigated the decline induced by moderate and severe heat stress. Relative to NRI + NMT plants, RI + MT pretreated plants exhibited a maximum increase of 12.84% in Fv/Fm, under control conditions (Figure 4D).
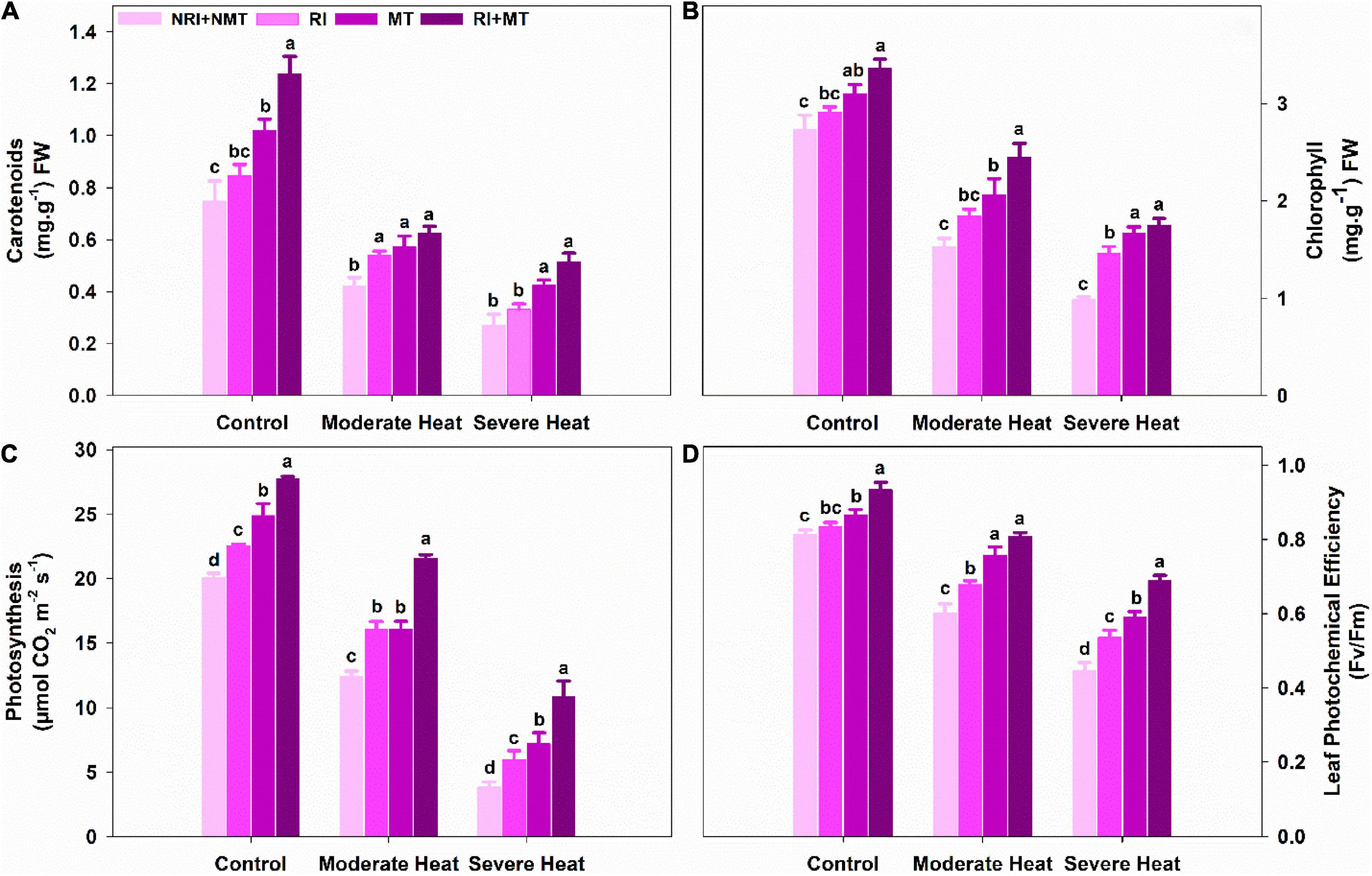
Figure 4. The individual and combined effects of Rhizobium inoculation and exogenous melatonin (MT) pretreatment on carotenoids (A), chlorophyll (B), Photosynthesis (C), and leaf photochemical efficiency (D) in Medicago truncatula under heat stress conditions. Control (26 ± 1°C): (23 ± 1°C) (light: dark), moderate heat (35 ± 1°C): (28 ± 1°C) (light: dark), severe heat (41 ± 1°C): (35 ± 1°C) (light: dark), NRI + NMT (no Rhizobium inoculation, no melatonin), RI (Rhizobium inoculation), MT (pretreated with 60 μM of melatonin), and (4) RI + MT (Rhizobium inoculation and pretreatment with 60 μM of melatonin).
Plant hormones and nutrients uptake
Our results revealed that MT pretreatment had no significant impact on the accumulation of endogenous MT under control conditions (Figure 5A). However, a significant increase was found in RI + MT pretreated by 34.29% and 32.85% over NRI + NMT plants under moderate and severe heat stress, respectively. The IAA levels showed an increasing trend in M. truncatula seedlings under moderate and severe heat stress (Figure 5B). ABA contents increased under moderate heat stress which was further accelerated under severe heat stress (Figure 5C). RI + MT pretreated plants increased the ABA and IAA levels under moderate (68.36% and 64.6%) and severe (54.02% and 53.92%) heat stress, compared to stressed NRI + NMT plants.
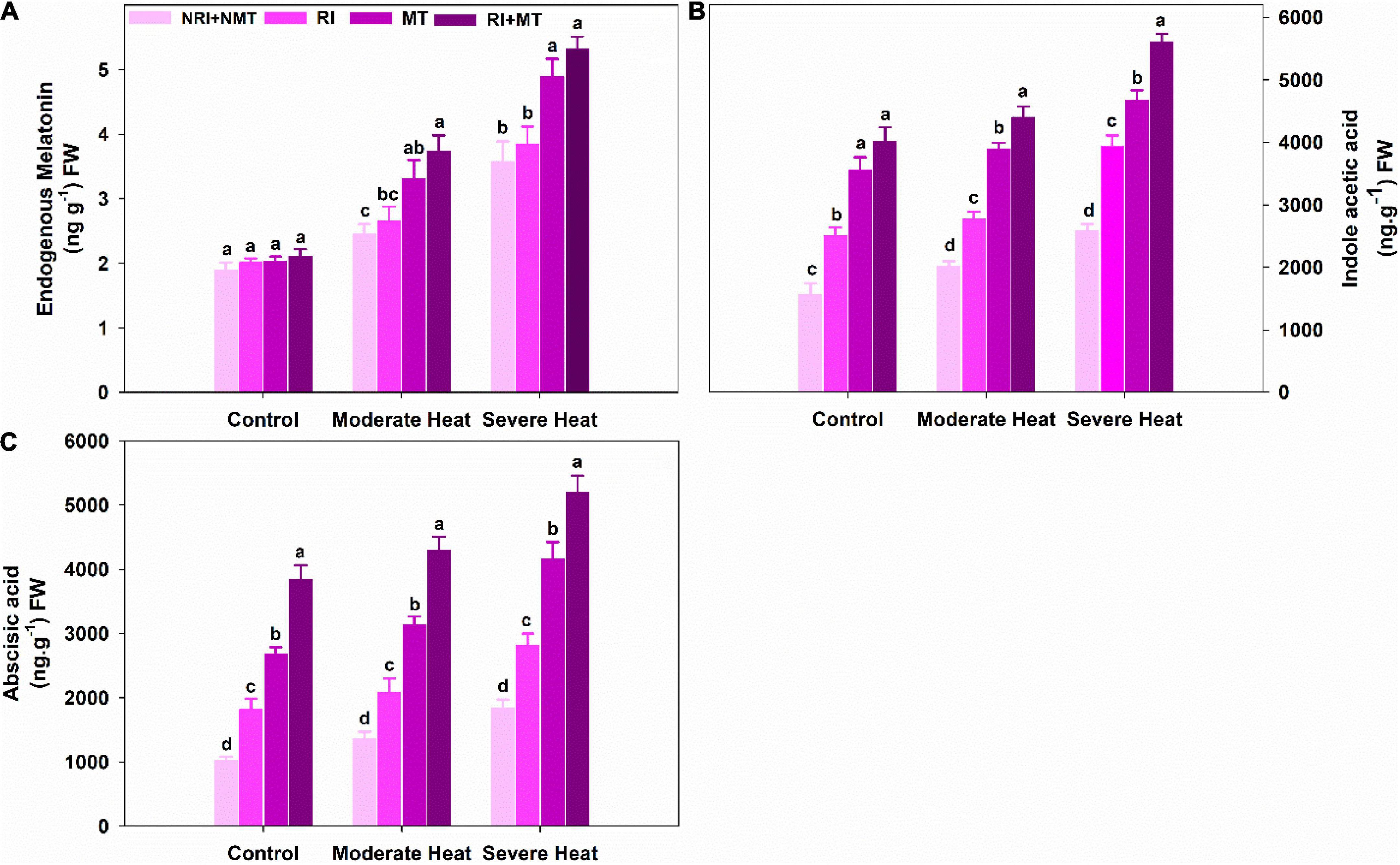
Figure 5. The individual and combined effects of Rhizobium inoculation and exogenous melatonin (MT) pretreatment on endogenous melatonin (A), indole acetic acid (B), and abscisic acid (C) in Medicago truncatula under heat stress conditions. Control (26 ± 1°C): (23 ± 1°C) (light: dark), moderate heat (35 ± 1°C): (28 ± 1°C) (light: dark), severe heat (41 ± 1°C): (35 ± 1°C) (light: dark), NRI + NMT (no Rhizobium inoculation, no melatonin), RI (Rhizobium inoculation), MT (pretreated with 60 μM of melatonin), and (4) RI + MT (Rhizobium inoculation and pretreatment with 60 μM of melatonin).
Plant dry biomass was reduced by 29.26% and 131.7% when exposed to moderate and severe heat stress, respectively, as compared to unstressed NRI + NMT plants. However, the co-application of RI and MT significantly increased plant dry biomass under both levels of heat stress (Figure 6A). Noticeable decreases in NPK at both levels of heat stress were observed while RI + MT treated plants significantly regulated NPK uptake in the heat-stressed plants (Figures 6B–D).
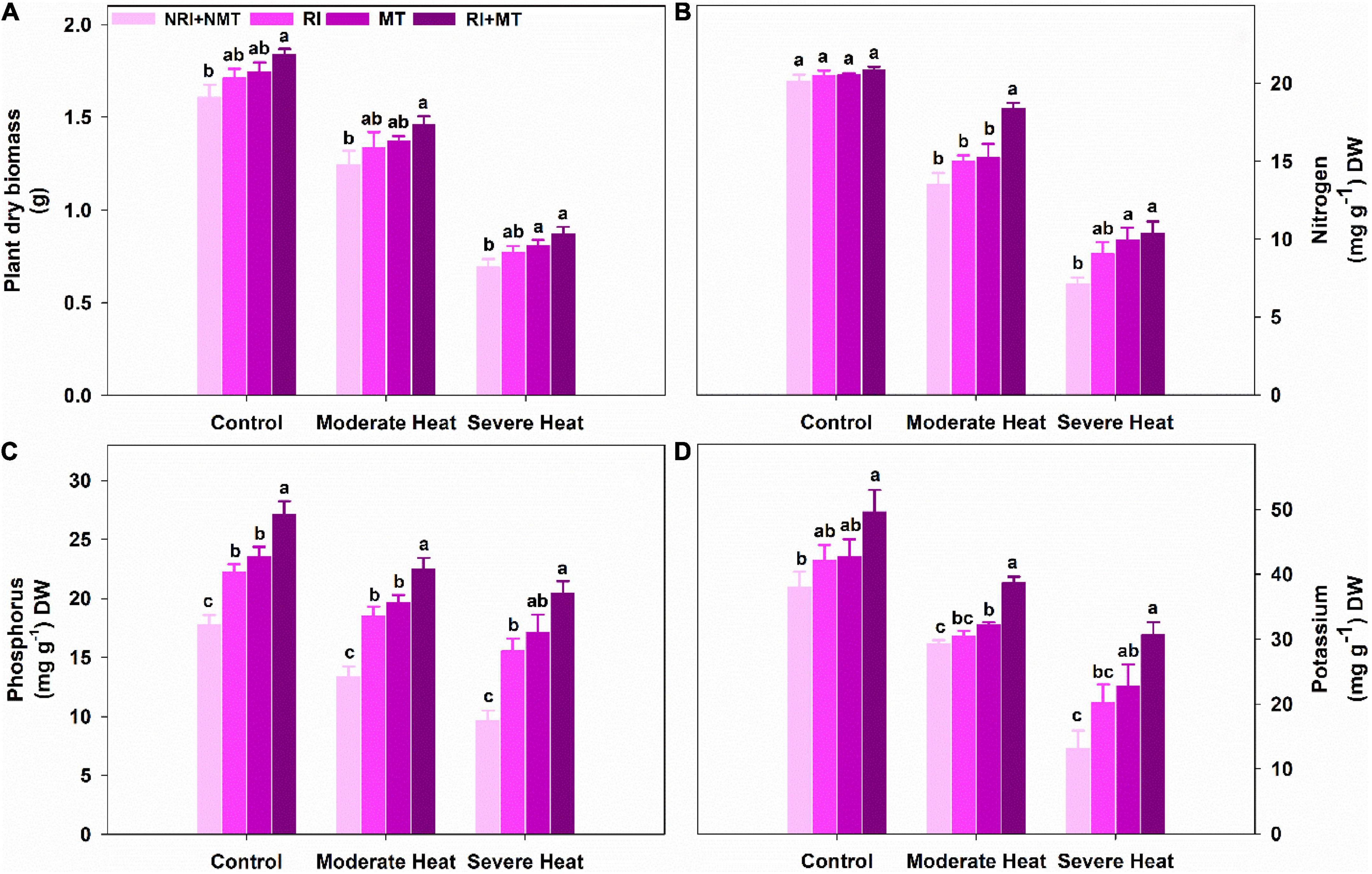
Figure 6. The individual and combined effects of Rhizobium inoculation and exogenous melatonin (MT) pretreatment on plant dry biomass (A), nitrogen (B), phosphorus (C), and potassium (D) in Medicago truncatula under heat stress conditions. Control (26 ± 1°C): (23 ± 1°C) (light: dark), moderate heat (35 ± 1°C): (28 ± 1°C) (light: dark), severe heat (41 ± 1°C): (35 ± 1°C) (light: dark), NRI + NMT (no Rhizobium inoculation, no melatonin), RI (Rhizobium inoculation), MT (pretreated with 60 μM of melatonin), and (4) RI + MT (Rhizobium inoculation and pretreatment with 60 μM of melatonin).
Nitro-oxidative homeostasis
Nitric oxide (NO) was significantly increased in RI treated plants under moderate (25.91%) and severe (15.47%) heat stress, which was further enhanced under combined pretreatment (RI + MT), imparting maximally the NO accumulation of 42.47% (moderate heat) and 34.92% (severe heat) under both heat stress levels NRI + NMT plants (Figure 7A). Under control conditions, RI + MT plants significantly up-regulated the NR activity unlike in NRI + NMT plants. The NR activity of NRI + NMT plants was significantly reduced by 41.36% (moderate heat stress) and 90.97% (severe heat stress) as compared to RI + MT control plants (Figure 7B). On the other hand, RI + MT pretreated plants exhibited significantly higher activity of NR by 31.57% and 37.48% as compared to their respective NRI + NMT plants under stress conditions.
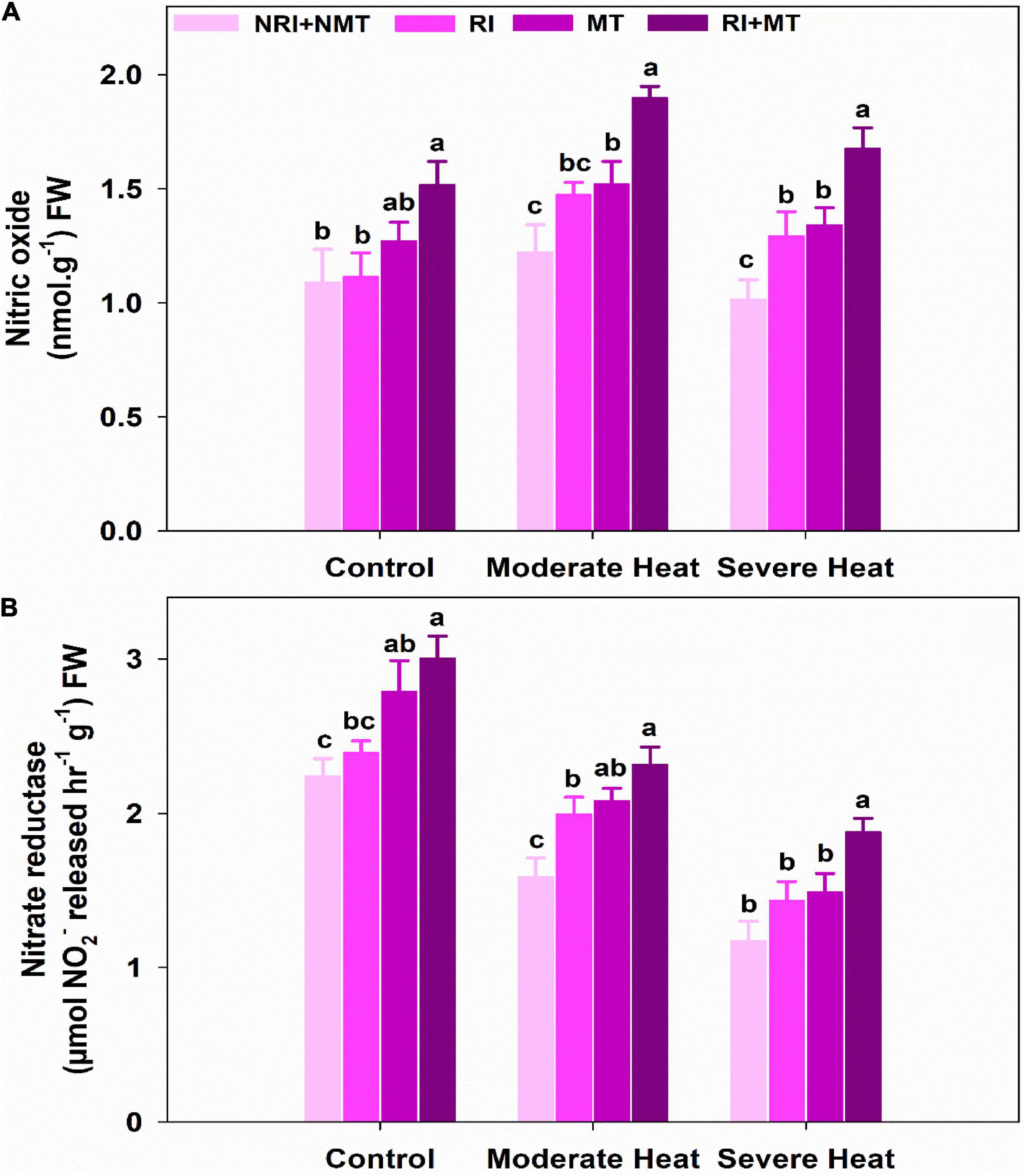
Figure 7. The individual and combined effects of Rhizobium inoculation and exogenous melatonin (MT) pretreatment on nitric oxide (A) and nitrate reductase activity (B) in Medicago truncatula under heat stress conditions. Control (26 ± 1°C): (23 ± 1°C) (light: dark), moderate heat (35 ± 1°C): (28 ± 1°C) (light: dark), severe heat (41 ± 1°C): (35 ± 1°C) (light: dark), NRI + NMT (no Rhizobium inoculation, no melatonin), RI (Rhizobium inoculation), MT (pretreated with 60 μM of melatonin), and (4) RI + MT (Rhizobium inoculation and pretreatment with 60 μM of melatonin).
Discussion
Selection of melatonin concentration under heat stress
Melatonin is actively involved in diverse plant physiological functions and thereby acts as a defensive player to a range of abiotic stressors under capricious environments (Banerjee and Roychoudhury, 2017; Irshad et al., 2021b). Its efficacy in combating abiotic stress varies at different concentrations in different crops (Huang et al., 2019; Jahan et al., 2019; Alam et al., 2021). In this study, variable concentrations of MT (0, 30, 60, and 90 μM) were preliminarily tested in Medicago truncatula plants under heat-stressed conditions and 60 μM was the most effective, showing higher dry biomass, photochemical efficiency (Fv/Fm), and lower electrolyte leakage (Supplementary Figure 1). The higher biomass accumulation with MT might be due to enhancement in carbon assimilation resulting from the improved photosynthetic capability of plants under stress conditions (Liu et al., 2018). Our results confirmed the previous reports on tomato (Xu et al., 2016), wheat (Buttar et al., 2020), and tall fescue (Alam et al., 2021) arguing that MT foliar applications alleviate the effects of heat stress and improved plants growth.
Amelioration of antioxidant enzyme mechanism and heat-induced oxidative damage
The current study demonstrated that heat stress strikingly increased MDA content which is a lipid peroxidation biomarker (Figure 1A). Increased lipid peroxidation level due to heat-induced ROS production has been previously described in wheat and sunflower plants (Buttar et al., 2020; Xing et al., 2021). ROS-induced oxidative damage may occur due to insufficient induction or inhibition of MG and ROS detoxification systems, thereby reducing membrane stability under stressed conditions. Meanwhile, the co-application of RI and MT was more effective in mitigating the negative effects of oxidative stress by reducing MDA, ROS, and MG, as compared to NRI + NMT stressed plants (Figures 1, 3C). Rhizobium inoculation enhanced the production of biomass and decreased ROS and lipid peroxidation under stress conditions as opposed to the non-inoculated plants (Jian et al., 2019; Liu et al., 2019). Rhizobium symbioses protect plants by hindering the ROS production pathways and maintain membranes integrity by protecting the proteins and enzymes (Irshad et al., 2021a). Similarly, MT can also protect against oxidative damages to the cell membrane and decrease the ROS generation in stressed maize plants (Jiang et al., 2016). Therefore, reduction in oxidative damage in RI + MT treated plants can be attributed to the regulation of the antioxidant enzyme system resulting in the removal of excess ROS under stressed conditions.
Plants respond to heat stress by employing an efficient antioxidant defense system through enzymatic and non-enzymatic activities to keep ROS release at a minimum (Wassie et al., 2019). Ascorbate is a key factor of the AsA-GSH pathway that directly or indirectly scavenges the ROS and its products (Anjum et al., 2010). In the present study, the AsA activity decreased in NRI + NMT treated plants which might be as a result of its inefficient restoration of AsA recycling enzymes under both control and stressed conditions (Figure 2E). Glutathione (GSH), however, acts as a cofactor for some enzymes like GST, GPX, and Gly I and thus eliminated free radicals in plants under stressed conditions and participates in the removal of MG, ROS, and other endogenously produced toxic compounds (Buttar et al., 2020). GSH contents were increased in NRI + NMT treated plants under both heat stress levels, while RI + MT treated seedlings had higher GSH levels than the seedlings treated with RI and MT alone under stressed conditions (Figure 2F), which might be due to improved GSH synthesis that was induced by RI and MT. Rhizobium inoculation accelerated the GSH recycling by the regulation of GR activity which is consistent with our recent findings (Irshad et al., 2021a).
The antioxidants AsA, GSH, SOD, POD, CAT, and APX regulated the defense mechanism of plants under abiotic stress cues. As a first-line enzyme in the defense mechanism, SOD is a key antioxidant enzyme that converts to H2O2 (Kaya et al., 2019). This H2O2 is counteracted by POD and CAT at the membranes and cytosol, respectively. APX and GR eliminate H2O2 and in mitochondria and chloroplasts by activating the AsA-GSH cycle (an intriguing pathway) (Ahanger and Agarwal, 2017). RI + MT pretreated plants exhibited higher SOD, POD, CAT, and APX activities as compared to alone treatments (RI and MT) under both stress and control conditions (Figures 2A–D) the same was previously noted in M. truncatula under cold stress conditions (Irshad et al., 2021b). However, Rhizobium inoculation enhances the antioxidant defense mechanism, alleviating abiotic stress-induced oxidative stress (Liu et al., 2019; Irshad et al., 2021a). Melatonin treatment has been considered a crucial antioxidant in plants that effectively quenches free radicals (Wei et al., 2015). Our results corroborated the findings of earlier studies such as tomato (Jahan et al., 2019), alfalfa (Wassie et al., 2020), ryegrass (Zhang J. et al., 2017), and rapeseed (Liu et al., 2018) that MT treatment enhanced the antioxidant enzymes activities in heat-stressed plants.
Followed by the ROS, the accumulation of another cytotoxic compound is MG, and its homeostasis must be regulated by its detoxification system (Gly I and Gly II) and GSH (Hasanuzzaman et al., 2020). As GSH is involved in both AsA-GSH and MG detoxification pathways, GHS may, however, be suggested as a point of interaction between MG detoxification system and antioxidant defense system (Li, 2016). In addition to the activation of antioxidant defense mechanisms by co-application of RI and MT (Figure 2), MG detoxification system (Gly I and Gly II) was found to be improved under control conditions in RI + MT treated plants and maintained their higher activities and GSH level than NRI + NMT plants at both heat stress levels (Figures 2, 3). These activities showed an important adaptive mechanism to heat stress tolerance by decreasing oxidative and MG stress. Our results about enhanced Gly I, Gly II activities and antioxidant defense mechanism to mitigate the ROS, MG, and MDA content confirmed the findings of Li et al. (2019) who reported the similar observations in maize seedlings.
Maintenance of plant growth, nutrient uptake, and nitro-oxidative homeostasis
Heat stress reduced root growth and its biomass, and thus affected the aboveground plants’ growth by restricting the transport of mineral nutrients and water, disturbing hormone production in roots and its supply to shoots (Farooq et al., 2017). The water and nutrients transport are very important in maintaining the structural integrity and up-regulation of physiological processes and functions (Dumlupinar et al., 2007). Numerous studies have reported that PGPR inoculation increased nutrient uptake and provided tolerance against abiotic stress cues (Chea et al., 2021). The growth and yield of leguminous plants are dependent on N fixation by nodules (Lindström and Mousavi, 2020), which is greatly affected by inhibiting the bacterial adherence to root hairs, development of new root hairs, and infection thread formation under heat stress (Alexandre and Oliveira, 2013). Heat stress at both levels decreased nutrients (N, P, and K) uptake, and their acquisition was improved with individual treatments of RI and MT (Figure 6). RI + MT treated plants benefited from the synergistic effect, as depicted by a substantial increase in the uptake of NPK unlike the individual treatment of RI or MT, or NRI + NMT at either level of heat stress. The increases in nutrient uptake might result from the improvement in root structure and size (Postma and Lynch, 2012). Foliar application of MT increased nutrient uptake in apple, wheat, and cucumber seedlings under abiotic stresses (Zhang R. et al., 2017; Liang et al., 2018; Buttar et al., 2020).
The increase in N absorption observed under RI + MT treated plants was a result of the enhanced activity of NR (Figure 7B). NR is a key enzyme and its activity evaluates the status of N content in plants which subsequently participates in the growth and development of plants. Activities of NR convert nitrate into ammonium and up-regulate the biosynthesis of various amino acids, thus it plays a crucial role in N assimilation in plants (Hirel et al., 2011). NR activity significantly reduces under stress conditions (Bashir et al., 2021) that hinder the N uptake in plants. Lee et al. (2020) have observed that PGPR inoculation significantly increased the N assimilation and use of nitrate in wheat and lettuce plants, unlike in non-inoculated plants. Similarly, Zhang R. et al. (2017) suggested that exogenous MT improved NR capacity, ammonium assimilation, and biomass production in cucumber seedlings. It also participates in the synthesis of nitric oxide (NO) in plants cultivated under stress conditions (Kaya et al., 2020). NO maintains cellular homeostasis in stressed plants to repair the negative effects of oxidative damage and thus works as a hallmark of nitrosative stress (Groß et al., 2013). NO acts as a strong antioxidant when the ROS concentration becomes toxic under heat stress (Santisree et al., 2015). RI + MT treated plants strongly increased NO content under both heat stress levels (Figure 7A). The increased NO content in RI + MT treated plants mitigated heat-induced damages probably by two processes: (1) Slowing-down of cellular redox homeostasis by defusing the harmful impacts of ROS; and (2) NO up-regulated the protection mechanisms in the heat-stressed plant by moderating the carotenoid contents (Parankusam et al., 2017). Furthermore, MT treatment alone or in combination with RI-treated plants increased NO content in M. truncatula plants under stress conditions (Figure 7B). It is reasonably possible that MT treatments might generate endogenous NO, which affects electron transport pathways in mitochondria thereby activating the antioxidant defense mechanisms that will improve plants’ stress tolerance (Shi et al., 2015). Iqbal et al. (2022) also reported that NO could enhance heat tolerance in wheat plants by synergistic interaction with other plant hormones and regulate osmolyte content and antioxidant level. Kaya et al. (2019) elucidated that the MT-mediated endogenously produced NO works as an antioxidant to enhance the stress amelioration in wheat. ABA and NO synergistically interact to reduce the heat stress effects on photosynthesis and growth via reducing the content of H2O2.
Improvement in plant hormones and photosynthetic efficiency
Plant hormones, ABA, and IAA play critical roles by triggering plant defense mechanisms against adverse environmental stresses, including heat stress (Abdelrahman et al., 2017; Zhang J. et al., 2017). Under stress conditions, ABA is a key regulator of phytohormone which acts as an endogenous messenger that is involved in the regulation of transcript expression during heat stress (Li et al., 2018). While, IAA is involved in cell elongation, vascular tissue differentiation, and enhances root growth, it also plays an important role in contributing to efficient water and nutrient uptake under salt stress (Liu et al., 2015). In this study, moderate and severe heat stresses increased ABA and IAA accumulations in all plant groups (RI, MT, RI + MT, and NRI + NMT); however, RI + MT treated plants led to higher accumulation of ABA and IAA as compared to other groups of stressed plants (Figures 5B,C). The higher accumulation of ABA in RI + MT treated plants might be correlated to the beneficial impact of both RI and MT, leading to improved photosynthetic activity, photosystem II activity, and photosynthetic pigments, as well as its direct involvement in boosting the antioxidant defense system (Irshad et al., 2021a,b). Castillo et al. (2013) reported that Arabidopsis plants inoculated with A. brasilense produced ABA content two-fold as compared to uninoculated plants. Zahedi et al. (2021) reported that exogenous MT increased the accumulation of IAA and ABA in olive plants under stress conditions. The increase in IAA production in RI + MT treated plants, however, may be a result of the fact that both MT and IAA have structural similarities, common biosynthetic pathways, and use tryptamine as the common precursor (Banerjee and Roychoudhury, 2017). Previously, exogenous MT has been reported to induce a higher accumulation of endogenous IAA in tomato and Brassica juncea plants (Wen et al., 2016). Various PGPRs, including Bradyrhizobium japonicum and Azospirillum brasilense can produce endogenous IAA at sufficient concentrations to induce morphological and physiological fluctuations in young plants (Boiero et al., 2007).
Endogenous MT production is one of the key features of stress responses in plants, with a similar effect on protection against abiotic stress cues as IAA (Nawaz et al., 2021). Our results revealed that individual or combined application of RI and MT pretreatment has no significant effect on the endogenous MT concentration. However, maximum endogenous MT accumulation was observed in plants that were treated with exogenous MT (MT, RI + MT), under both heat stress levels (Figure 5A). This result indicates endogenous MT may offer a potential role in heat stress tolerance in plants thus supporting the findings of Buttar et al. (2020) where exogenous MT was reported to increased tolerance in wheat plants under heat stress. Melatonin is thought to be synthesized and metabolized in mitochondria and chloroplasts of plants. The higher production of MT protected the rate of photosynthesis by maintaining chloroplast integrity (Huang et al., 2019). Chloroplasts are the major sites for the free radical generation which are produced in excess under stress conditions. Hence, it requires a strong protection mechanism against oxidative stress (such as free radicals), which can be partially supported by endogenously produced MT.
Unlike other physiological processes, photosynthesis is the most heat-sensitive photoreaction. Photosynthesis improves plant growth and subsequently promotes stress tolerance capacity under hostile environments (Wassie et al., 2020). Heat stress causes chlorophyll breakdown and reduces chlorophyll biosynthesis because of decreased nutrient uptake (Efeoglu and Terzioglu, 2009), which results in inhibited photosynthesis and photosystem II activity (Buttar et al., 2020) and thus directly impacts plant growth and yield. Heat stress decreased leaf photochemical efficiency (Fv/Fm), net photosynthetic rate, carotenoid, and chlorophyll contents, however, RI + MT treated plants hampered this decline efficiently against moderate and severe heat stresses (Figures 4A–D). This might be due to exogenous MT enhancing the endogenous MT concentration and biosynthesis gene expression, which might regulate the optimal chlorophyll content under heat stress (Jahan et al., 2019). Another reason could be RI, which increases N supply due to N fixation, stimulated the root activity in both treatments (RI and MT), and increased the nutrient uptake (such as N and Mg2+), which are an integral part of chlorophyll (Yousaf et al., 2021). The co-application of RI and MT pretreatment may significantly improve photosystem functioning and mitigate the refrainment of photosynthesis in NRI + NMT plants under moderate and severe heat stress. The damage in Fv/Fm might be due to an increase in ROS production under heat stress (Dolatabadian and Jouneghani, 2009), however, RI + MT treated plants scavenged ROS which in turn cause less damage to chloroplasts and photosystem. These findings are consistent with our recent studies, that the alone or co-application of RI and MT pretreatment in Medicago truncatula enhanced the photosynthesis rate and Fv/Fm under salt and cold stress conditions (Irshad et al., 2021a,b).
Regulation of osmolytes accumulation
Osmolyte accumulation is one of the mechanisms adopted by plants that have a protective role against temperature stress (Sharma et al., 2019). Osmolytes (such as glycine betaine, proline, soluble sugar, and proteins) were enhanced significantly due to individual or co-application of RI and MT pretreatments (Supplementary Table 1). Plants enhanced the accumulation of various osmolytes inside the cell (cytosol) to hamper the harmful impacts of osmotically induced stress (Latef and Miransari, 2014). Maintenance of water potential and turgor pressure in plant cells is a key strategy to mitigate osmotic stress damage by reinforcing the plants’ osmoregulation system (Iqbal et al., 2015). Our results are in line with the findings of Li et al. (2019) who reported that exogenous MT could increase the accumulation of the compatible solutes (proline, total soluble sugar, and trehalose) in maize plants under heat stress. Proline, amino acids, and soluble sugar work as an energy reservoir for immediate stress release and support the functional and structural integrity of major cellular compartments like membranes (Meena et al., 2019). Nitrogen-derived osmolytes such as proline and amino acids were involved in stimulating antioxidant defense mechanisms for stress-bearing in plants (Sharma et al., 2022). Besides this, the accumulation of glycine betaine is associated with the protection of photosynthesis by up-regulating the activities of Rubisco under different kinds of stress (Khan and Hakeem, 2014).
Conclusion
Global warming and the rise in adverse climatic conditions are inevitably degrading crop productivity thereby threatening global food security. Medicago truncatula is a thermo-sensitive crop and its growth is severely affected due to moderate and severe heat stresses. RI + MT treated plants maintained better NPK uptake, NO activity, membrane functioning, and endogenous hormonal levels under heat stress conditions. The surge in redox components and subsequent decreases in oxidative damage (MDA, H2O2, , and LOX) by keeping ROS release at minimum by up-regulating enzymatic activities (POD, CAT, APX, and SOD), modifying methylglyoxal detoxification system and markedly accumulation of osmolytes (proline, glycine betaine, soluble sugar contents, soluble protein contents) protected plants from heat-induced damages and maintained structural and functional stability. These findings provide novel insight into the crosstalk among RI and MT to inhibit the effects of heat stress under rapidly changing global climate and rising temperature. To understand these interactions, further investigation is required to determine how these molecules collectively collaborate to alleviate heat stress-induced oxidative damage.
Data availability statement
The original contributions presented in this study are included in the article/Supplementary material, further inquiries can be directed to the corresponding author.
Author contributions
AI, TH, and PY designed the research. AI performed the experiment. AI, RR, SD, and MK analyzed data and wrote the manuscript. RR, SD, MK, PY, and TH provided ideas and revised the manuscript. All authors read and approved the final manuscript.
Funding
This work was supported by the China Agriculture Research System of MOF and MARA and the Project of Innovation-Driven Plan of Shaanxi Academy of Forestry Sciences (No. SXLK2020-0204).
Acknowledgments
We especially thank Sajjad Raza from Nanjing University of Information Science and Technology for his advice and constructive comments on this manuscript.
Conflict of interest
The authors declare that the research was conducted in the absence of any commercial or financial relationships that could be construed as a potential conflict of interest.
Publisher’s note
All claims expressed in this article are solely those of the authors and do not necessarily represent those of their affiliated organizations, or those of the publisher, the editors and the reviewers. Any product that may be evaluated in this article, or claim that may be made by its manufacturer, is not guaranteed or endorsed by the publisher.
Supplementary material
The Supplementary Material for this article can be found online at: https://www.frontiersin.org/articles/10.3389/fevo.2022.945695/full#supplementary-material
References
Abdelrahman, M., El-Sayed, M., Jogaiah, S., Burritt, D. J., and Tran, L. S. P. (2017). The “STAY-GREEN” trait and phytohormone signaling networks in plants under heat stress. Plant Cell Rep. 36, 1009–1025. doi: 10.1007/s00299-017-2119-y
Ahanger, M. A., and Agarwal, R. M. (2017). Potassium up-regulates antioxidant metabolism and alleviates growth inhibition under water and osmotic stress in wheat (Triticum aestivum L). Protoplasma 254, 1471–1486. doi: 10.1007/s00709-016-1037-0
Ahanger, M. A., Qi, M., Huang, Z., Xu, X., Begum, N., Qin, C., et al. (2021). Improving growth and photosynthetic performance of drought stressed tomato by application of nano-organic fertilizer involves up-regulation of nitrogen, antioxidant and osmolyte metabolism. Ecotoxicol. Environ. Saf. 216:112195. doi: 10.1016/j.ecoenv.2021.112195
Ahanger, M. A., Qin, C., Begum, N., Maodong, Q., Dong, X. X., El-Esawi, M., et al. (2019). Nitrogen availability prevents oxidative effects of salinity on wheat growth and photosynthesis by up-regulating the antioxidants and osmolytes metabolism, and secondary metabolite accumulation. BMC Plant Biol. 19:479. doi: 10.1186/s12870-019-2085-3
Alam, M. N., Yang, L., Yi, X., Wang, Q., and Robin, A. H. K. (2021). Role of melatonin in inducing the physiological and biochemical processes associated with heat stress tolerance in tall fescue (Festuca arundinaceous). J. Plant Growth Regul. 1–10. doi: 10.1007/s00344-021-10472-6
Alexandre, A., and Oliveira, S. (2013). Response to temperature stress in rhizobia. Crit. Rev. Microbiol. 39, 219–228.
Anjum, N. A., Umar, S., and Chan, M. T. (eds) (2010). Ascorbate-glutathione pathway and stress tolerance in plants. Dordrecht: Springer.
Banerjee, A., and Roychoudhury, A. (2017). “Melatonin as a regulator of abiotic stress tolerance in plants,” in Mechanisms Behind Phytohormonal Signaling and Crop Abiotic Stress Tolerance, eds V. P. Singh, S. Singh, and S. M. Prasad (New York, NY: Nova Science Publishers), 47–60.
Bashir, S. S., Hussain, A., Hussain, S. J., Wani, O. A., Nabi, S. Z., Dar, N. A., et al. (2021). Plant drought stress tolerance: Understanding its physiological, biochemical and molecular mechanisms. Biotechnol. Biotechnol. Equip. 35, 1912–1925.
Boiero, L., Perrig, D., Masciarelli, O., Penna, C., Cassán, F., and Luna, V. (2007). Phytohormone production by three strains of Bradyrhizobium japonicum and possible physiological and technological implications. Appl. Microbiol. Biotechnol. 74, 874–880. doi: 10.1007/s00253-006-0731-9
Buttar, Z. A., Wu, S. N., Arnao, M. B., Wang, C., Ullah, I., and Wang, C. (2020). Melatonin suppressed the heat stress-induced damage in wheat seedlings by modulating the antioxidant machinery. Plants 9:809. doi: 10.3390/plants9070809
Campobenedetto, C., Mannino, G., Agliassa, C., Acquadro, A., Contartese, V., Garabello, C., et al. (2020). Transcriptome analyses and antioxidant activity profiling reveal the role of a lignin-derived biostimulant seed treatment in enhancing heat stress tolerance in soybean. Plants 9:1308. doi: 10.3390/plants9101308
Castillo, P., Escalante, M., Gallardo, M., Alemano, S., and Abdala, G. (2013). Effects of bacterial single inoculation and co-inoculation on growth and phytohormone production of sunflower seedlings under water stress. Acta Physiol. Plant. 35, 2299–2309.
Chea, L., Pfeiffer, B., Schneider, D., Daniel, R., Pawelzik, E., and Naumann, M. (2021). Morphological and metabolite responses of potatoes under various phosphorus levels and their amelioration by plant growth-promoting rhizobacteria. Int. J. Mol. Sci. 22:5162. doi: 10.3390/ijms22105162
Dolatabadian, A., and Jouneghani, R. S. (2009). Impact of exogenous ascorbic acid on antioxidant activity and some physiological traits of common bean subjected to salinity stress. Notul. Bot. Horti Agrobot. Cluj Napoca 37, 165–172.
Dumlupinar, R., Demir, F., Budak, G., Karabulut, A., Kadi, N., Karakurt, H., et al. (2007). Determination of replacement of some inorganic elements in pulvinus of bean (Phaseolus vulgaris cv. Gina 2004) at chilling temperature by the WDXRF spectroscopic technique. J. Quant. Spectrosc. Radiat. Transfer. 103, 331–339.
Efeoglu, B., and Terzioglu, S. (2009). Photosynthetic responses of two wheat varieties to high temperature. Eur. J. Biosci. 3, 97–106. doi: 10.1371/journal.pone.0255896
Farooq, M., Nadeem, F., Gogoi, N., Ullah, A., Alghamdi, S. S., Nayyar, H., et al. (2017). Heat stress in grain legumes during reproductive and grain-filling phases. Crop Past. Sci. 68, 985–1005.
Groß, F., Durner, J., and Gaupels, F. (2013). Nitric oxide, antioxidants and prooxidants in plant defence responses. Front. Plant Sci. 4:419. doi: 10.3389/fpls.2013.00419
Hasanuzzaman, M., Bhuyan, M. H. M. B., Parvin, K., Bhuiyan, T. F., Anee, T. I., Nahar, K., et al. (2020). Regulation of ROS metabolism in plants under environmental stress: A review of recent experimental evidence. Int. J. Mol. Sci. 21:8695. doi: 10.3390/ijms21228695
Hirel, B., Tétu, T., Lea, P. J., and Dubois, F. (2011). Improving nitrogen use efficiency in crops for sustainable agriculture. Sustainability 3, 1452–1485.
Hoagland, D. R., and Arnon, D. I. (1950). The water-culture method for growing plants without soil. Circ. Calif. Agric. Exp. Station 347:32.
Huang, B., Chen, Y. E., Zhao, Y. Q., Ding, C. B., Liao, J. Q., Hu, C., et al. (2019). Exogenous melatonin alleviates oxidative damages and protects photosystem II in maize seedlings under drought stress. Front. Plant Sci. 10:677. doi: 10.3389/fpls.2019.00677
IPCC (2007). “Climate change 2007–the physical science basis,” in Contribution of working group I to the fourth assessment report of the intergovernmental panel on climate change, ed. IPCC (Cambridge: Cambridge University Press).
Iqbal, N., Nazar, R., and Khan, N. A. (eds) (2015). Osmolytes and plants acclimation to changing environment: Emerging omics technologies. New Delhi: Springer.
Iqbal, N., Sehar, Z., Fatma, M., Umar, S., Sofo, A., and Khan, N. A. (2022). Nitric Oxide and abscisic acid mediate heat stress tolerance through regulation of osmolytes and antioxidants to protect photosynthesis and growth in wheat plants. Antioxidants 11:372. doi: 10.3390/antiox11020372
Irshad, A., Rehman, R. N. U., Abrar, M. M., Saeed, Q., Sharif, R., and Hu, T. (2021a). Contribution of rhizobium–legume symbiosis in salt stress tolerance in Medicago truncatula evaluated through photosynthesis, antioxidant enzymes, and compatible solutes accumulation. Sustainability 13:3369.
Irshad, A., Rehman, R. N. U., Kareem, H. A., Yang, P., and Hu, T. (2021b). Addressing the challenge of cold stress resilience with the synergistic effect of Rhizobium inoculation and exogenous melatonin application in Medicago truncatula. Ecotoxicol. Environ. Saf. 226:112816. doi: 10.1016/j.ecoenv.2021.112816
Jahan, M. S., Shu, S., Wang, Y., Chen, Z., He, M., Tao, M., et al. (2019). Melatonin alleviates heat-induced damage of tomato seedlings by balancing redox homeostasis and modulating polyamine and nitric oxide biosynthesis. BMC Plant Biol. 19:414. doi: 10.1186/s12870-019-1992-7
Jian, L., Bai, X., Zhang, H., Song, X., and Li, Z. (2019). Promotion of growth and metal accumulation of alfalfa by coinoculation with Sinorhizobium and Agrobacterium under copper and zinc stress. PeerJ 7:e6875. doi: 10.7717/peerj.6875
Jiang, C., Cui, Q., Feng, K., Xu, D., Li, C., and Zheng, Q. (2016). Melatonin improves antioxidant capacity and ion homeostasis and enhances salt tolerance in maize seedlings. Acta Physiol. Plant. 38:82.
Kaya, C., Ashraf, M., Alyemeni, M. N., and Ahmad, P. (2020). The role of nitrate reductase in brassinosteroid-induced endogenous nitric oxide generation to improve cadmium stress tolerance of pepper plants by upregulating the ascorbate-glutathione cycle. Ecotoxicol. Environ. Saf. 196:110483. doi: 10.1016/j.ecoenv.2020.110483
Kaya, C., Okant, M., Ugurlar, F., Alyemeni, M. N., Ashraf, M., and Ahmad, P. (2019). Melatonin-mediated nitric oxide improves tolerance to cadmium toxicity by reducing oxidative stress in wheat plants. Chemosphere 225, 627–638. doi: 10.1016/j.chemosphere.2019.03.026
Khan, F., and Hakeem, K. R. (2014). “Cell signaling during drought and salt stress,” in Plant Signaling: Understanding the Molecular Crosstalk, eds I. Tahir, K. R. Hakeem, and R. Ul Rehman (New Delhi: Springer), 227–239.
Latef, A. A. H. A., and Miransari, M. (2014). “The role of arbuscular mycorrhizal fungi in alleviation of salt stress,” in Use of microbes for the alleviation of soil stresses, (New York, NY: Springer), 23–38.
Lee, S., Trınh, C. S., Lee, W. J., Jeong, C. Y., Truong, H. A., Chung, N., et al. (2020). Bacillus subtilis strain L1 promotes nitrate reductase activity in Arabidopsis and elicits enhanced growth performance in Arabidopsis, lettuce, and wheat. J. Plant Res. 133, 231–244. doi: 10.1007/s10265-019-01160-4
Li, C., Bian, B., Gong, T., and Liao, W. (2018). Comparative proteomic analysis of key proteins during abscisic acid-hydrogen peroxide-induced adventitious rooting in cucumber (Cucumis sativus L.) under drought stress. J. Plant Physiol. 229, 185–194. doi: 10.1016/j.jplph.2018.07.012
Li, N., Euring, D., Cha, J. Y., Lin, Z., Lu, M., Huang, L. J., et al. (2021). Plant hormone-mediated regulation of heat tolerance in response to global climate change. Front. Plant Sci. 11:627969. doi: 10.3389/fpls.2020.627969
Li, Z. G. (2016). Methylglyoxal and glyoxalase system in plants: Old players, new concepts. Bot. Rev. 82, 183–203.
Li, Z. G., Duan, X. Q., Min, X., and Zhou, Z. H. (2017). Methylglyoxal as a novel signal molecule induces the salt tolerance of wheat by regulating the glyoxalase system, the antioxidant system, and osmolytes. Protoplasma 254, 1995–2006. doi: 10.1007/s00709-017-1094-z
Li, Z. G., Xu, Y., Bai, L. K., Zhang, S. Y., and Wang, Y. (2019). Melatonin enhances thermotolerance of maize seedlings (Zea mays L.) by modulating antioxidant defense, methylglyoxal detoxification, and osmoregulation systems. Protoplasma 256, 471–490. doi: 10.1007/s00709-018-1311-4
Liang, B., Ma, C., Zhang, Z., Wei, Z., Gao, T., Zhao, Q., et al. (2018). Long-term exogenous application of melatonin improves nutrient uptake fluxes in apple plants under moderate drought stress. Environ. Exp. Bot. 155, 650–661.
Lindström, K., and Mousavi, S. A. (2020). Effectiveness of nitrogen fixation in rhizobia. Microb. Biotechnol. 13, 1314–1335.
Liu, W., Li, R. J., Han, T. T., Cai, W., Fu, Z. W., and Lu, Y. T. (2015). Salt stress reduces root meristem size by nitric oxide-mediated modulation of auxin accumulation and signaling in Arabidopsis. Plant Physiol. 168, 343–356. doi: 10.1104/pp.15.00030
Liu, Y. S., Geng, J. C., Sha, X. Y., Zhao, Y. X., Hu, T. M., and Yang, P. Z. (2019). Effect of Rhizobium symbiosis on low-temperature tolerance and antioxidant response in alfalfa (Medicago sativa L.). Front. Plant Sci. 10:538. doi: 10.3389/fpls.2019.00538
Liu, Z., Cai, J. S., Li, J. J., Lu, G. Y., Li, C. S., Fu, G. P., et al. (2018). Exogenous application of a low concentration of melatonin enhances salt tolerance in rapeseed (Brassica napus L.) seedlings. J. Integr. Agric. 17, 328–335.
Meena, M., Divyanshu, K., Kumar, S., Swapnil, P., Zehra, A., Shukla, V., et al. (2019). Regulation of L-proline biosynthesis, signal transduction, transport, accumulation and its vital role in plants during variable environmental conditions. Heliyon 5:e02952. doi: 10.1016/j.heliyon.2019.e02952
Moghaddam, S. M., Oladzad, A., Koh, C., Ramsay, L., Hart, J. P., Mamidi, S., et al. (2021). The tepary bean genome provides insight into evolution and domestication under heat stress. Nat. Commun. 12, 1–14. doi: 10.1038/s41467-021-22858-x
Mollinedo, J., Schumacher, T. E., and Chintala, R. (2016). Biochar effects on phenotypic characteristics of “wild” and “sickle” Medicago truncatula genotypes. Plant Soil 400, 1–14.
Nawaz, K., Chaudhary, R., Sarwar, A., Ahmad, B., Gul, A., Hano, C., et al. (2021). Melatonin as master regulator in plant growth, development and stress alleviator for sustainable agricultural production: Current status and future perspectives. Sustainability 13:294.
Ohama, N., Sato, H., Shinozaki, K., and Yamaguchi-Shinozaki, K. (2017). Transcriptional regulatory network of plant heat stress response. Trends Plant Sci. 22, 53–65.
Parankusam, S., Adimulam, S. S., Bhatnagar-Mathur, P., and Sharma, K. K. (2017). Nitric oxide (NO) in plant heat stress tolerance: Current knowledge and perspectives. Front. Plant Sci. 8:1582. doi: 10.3389/fpls.2017.01582
Postma, J. A., and Lynch, J. P. (2012). Complementarity in root architecture for nutrient uptake in ancient maize/bean and maize/bean/squash polycultures. Ann. Bot. 110, 521–534. doi: 10.1093/aob/mcs082
Santisree, P., Bhatnagar-Mathur, P., and Sharma, K. K. (2015). NO to drought-multifunctional role of nitric oxide in plant drought: Do we have all the answers. Plant Sci. 239, 44–55. doi: 10.1016/j.plantsci.2015.07.012
Shahzad, R., Waqas, M., Khan, A. L., Hamayun, M., Kang, S. M., and Lee, I. J. (2015). Foliar application of methyl jasmonate induced physio-hormonal changes in Pisum sativum under diverse temperature regimes. Plant Physiol. Biochem. 96, 406–416. doi: 10.1016/j.plaphy.2015.08.020
Sharma, A., Kapoor, D., Gautam, S., Landi, M., Kandhol, N., Araniti, F., et al. (2022). Heavy metal induced regulation of plant biology: Recent insights. Physiol. Plant. 174:e13688.
Sharma, A., Shahzad, B., Kumar, V., Kohli, S. K., Sidhu, G. P. S., Bali, A. S., et al. (2019). Phytohormones regulate accumulation of osmolytes under abiotic stress. Biomolecules 9:285.
Shi, H., Wang, X., Tan, D. X., Reiter, R. J., and Chan, Z. (2015). Comparative physiological and proteomic analyses reveal the actions of melatonin in the reduction of oxidative stress in Bermuda grass (Cynodon dactylon (L). Pers.). J. Pineal Res. 59, 120–131. doi: 10.1111/jpi.12246
Siddiqui, M. H., Al-Khaishany, M. Y., Al-Qutami, M. A., Al-Whaibi, M. H., Grover, A., Ali, H. M., et al. (2015). Morphological and physiological characterization of different genotypes of faba bean under heat stress. Saudi J. Biol. Sci. 22, 656–663. doi: 10.1016/j.sjbs.2015.06.002
Wani, S. H., Kumar, V., Shriram, V., and Sah, S. K. (2016). Phytohormones and their metabolic engineering for abiotic stress tolerance in crop plants. Crop J. 4, 162–176.
Wassie, M., Zhang, W., Zhang, Q., Ji, K., and Chen, L. (2019). Effect of heat stress on growth and physiological traits of alfalfa (Medicago sativa L.) and a comprehensive evaluation for heat tolerance. Agronomy 9:597.
Wassie, M., Zhang, W., Zhang, Q., Ji, K., Cao, L., and Chen, L. (2020). Exogenous salicylic acid ameliorates heat stress-induced damages and improves growth and photosynthetic efficiency in alfalfa (Medicago sativa L.). Ecotoxicol. Environ. Saf. 191:110206. doi: 10.1016/j.ecoenv.2020.110206
Wei, W., Li, Q. T., Chu, Y. N., Reiter, R. J., Yu, X. M., Zhu, D. H., et al. (2015). Melatonin enhances plant growth and abiotic stress tolerance in soybean plants. J. Exp. Bot. 66, 695–707.
Wen, D., Gong, B., Sun, S., Liu, S., Wang, X., Wei, M., et al. (2016). Promoting roles of melatonin in adventitious root development of Solanum lycopersicum L. by regulating auxin and nitric oxide signaling. Front. Plant Sci. 7:718. doi: 10.3389/fpls.2016.00718
Xing, X., Ding, Y., Jin, J., Song, A., Chen, S., Chen, F., et al. (2021). Physiological and transcripts analyses reveal the mechanism by which melatonin alleviates heat stress in chrysanthemum seedlings. Front. Plant Sci. 12:673236. doi: 10.3389/fpls.2021.673236
Xu, W., Cai, S. Y., Zhang, Y., Wang, Y., Ahammed, G. J., Xia, X. J., et al. (2016). Melatonin enhances thermotolerance by promoting cellular protein protection in tomato plants. J. Pineal Res. 61, 457–469. doi: 10.1111/jpi.12359
Yousaf, M., Bashir, S., Raza, H., Shah, A. N., Iqbal, J., Arif, M., et al. (2021). Role of nitrogen and magnesium for growth, yield and nutritional quality of radish. Saudi J. Biol. Sci. 28, 3021–3030. doi: 10.1016/j.sjbs.2021.02.043
Zahedi, S. M., Hosseini, M. S., Fahadi Hoveizeh, N., Gholami, R., Abdelrahman, M., and Tran, L. S. P. (2021). Exogenous melatonin mitigates salinity-induced damage in olive seedlings by modulating ion homeostasis, antioxidant defense and phytohormone balance. Physiol. Plant. 173, 1682–1694. doi: 10.1111/ppl.13589
Zhang, J., Shi, Y., Zhang, X., Du, H., Xu, B., and Huang, B. (2017). Melatonin suppression of heat-induced leaf senescence involves changes in abscisic acid and cytokinin biosynthesis and signaling pathways in perennial ryegrass (Lolium perenne L.). Environ. Exp. Bot. 138, 36–45.
Zhang, Q., Liu, X., Zhang, Z., Liu, N., Li, D., and Hu, L. (2019). Melatonin improved waterlogging tolerance in alfalfa (Medicago sativa) by reprogramming polyamine and ethylene metabolism. Front. Plant Sci. 10:44. doi: 10.3389/fpls.2019.00044
Keywords: abscisic acid, indole-3-acetic acid, methylglyoxal detoxification system, osmolytes accumulation, oxidative damage
Citation: Irshad A, Rehman RNU, Dubey S, Khan MA, Yang P and Hu T (2022) Rhizobium inoculation and exogenous melatonin synergistically increased thermotolerance by improving antioxidant defense, photosynthetic efficiency, and nitro-oxidative homeostasis in Medicago truncatula. Front. Ecol. Evol. 10:945695. doi: 10.3389/fevo.2022.945695
Received: 16 May 2022; Accepted: 29 August 2022;
Published: 20 September 2022.
Edited by:
Chunliu Zhuo, University of North Texas, United StatesReviewed by:
Md. Mahmudul Hassan, Patuakhali Science and Technology University, BangladeshHayssam M. Ali, King Saud University, Saudi Arabia
Md. Mahadi Hasan, Lanzhou University, China
Copyright © 2022 Irshad, Rehman, Dubey, Khan, Yang and Hu. This is an open-access article distributed under the terms of the Creative Commons Attribution License (CC BY). The use, distribution or reproduction in other forums is permitted, provided the original author(s) and the copyright owner(s) are credited and that the original publication in this journal is cited, in accordance with accepted academic practice. No use, distribution or reproduction is permitted which does not comply with these terms.
*Correspondence: Peizhi Yang, eWFuZ3BlaXpoaUAxMjYuY29t; Tianming Hu, aHV0aWFubWluZ0AxMjYuY29t