- 1Department of Biology, University of North Carolina at Chapel Hill, Chapel Hill, NC, United States
- 2Environment, Ecology, and Energy Program, University of North Carolina at Chapel Hill, Chapel Hill, NC, United States
In response to environmental change, species may decrease or increase in population size across their range, expand or contract their range limits, or alter how sites are occupied within their existing range. Shifts in range limits and widespread changes in population size have been documented in birds especially in response to changes in climate. Range occupancy, or how patchily or continuously a species is distributed within their range, has been studied less in the context of anthropogenic changes but may be expected to decrease with range-wide population size if abundance-occupancy relationships are generally positive. Determining which properties of species are related to range expansion or contraction or increased range occupancy or decreased range occupancy is useful in developing an understanding of which species become “winners” or “losers” under global change. Species with broader climatic niches may be more likely to successfully expand to new sites as climate changes. Range occupancy can be related to habitat preferences of species, and habitat specialization may predict how species fill in sites within their range. To examine how species niche breadth may explain changes in species distributions, we modeled how changes in range-wide population size, range extent, and range occupancy from 1976 to 2016 were predicted by species’ climate, habitat, and diet niche breadth for 77 North American breeding bird species. We found that climate generalists were more likely to be increasing in range area, while species with declining population trends were likely to be contracting in range area and in occupancy within their range. Understanding how different dimensions of specialization relate to shifts in species distributions may improve predictions of which species are expected to benefit from or be vulnerable to anthropogenic change.
Introduction
Globally, species are experiencing multiple sources of change to their environments driven by human activity, including but not limited to land use and climate change (Walther et al., 2002; Parmesan and Yohe, 2003). While the direct impacts of many of these changes are felt locally, these local shifts can scale up to measurable changes at the level of a species range within a region or continent. Many species distributions may shift toward poles or move upslope to higher elevations to maintain consistent climatic niches (Mason et al., 2015). Broad, continental-scale population trends have also been observed for many groups (Rosenberg et al., 2019). Considering multiple dimensions of species responses in their distribution at continental scales can help clarify the processes and mechanisms by which species are impacted by anthropogenic change at macroecological scales.
At the level of a species range, species may increase or decrease in population size across their range, expand or contract their range limits, or alter how sites are occupied within their existing range. Shifts in range limits and widespread changes in population size have been documented in response to changes in climate that may impact species occurrence due to their physiological tolerance or through interactions with other species (Tingley et al., 2009; Illán et al., 2014). Range occupancy, or how patchily or continuously a species is distributed within their range (Hurlbert and White, 2007), has been studied less in the context of anthropogenic changes such as climate and land use change. Range occupancy may be expected to decrease with range-wide population size if abundance-occupancy relationships are generally positive (Gaston et al., 2000).
The distributions of North American breeding bird species have been well-studied. Widespread population declines, especially in very abundant species, have been observed over the past several decades (Rosenberg et al., 2019). Declines have also been observed particularly among long-distance migrants and insectivores (Bregman et al., 2014; Zurell et al., 2018). Upslope distribution shifts to track changing climate conditions have also been observed (Tingley et al., 2009), as well as northward shifts in the range limits of some species (La Sorte and Thompson, 2007; Townsend Peterson and Martínez-Meyer, 2009; Zuckerberg et al., 2009). A few species have also undergone rapid range expansion in the past half-century, including Northern Cardinal (Cardinalis cardinalis) (Halkin and Linville, 1999), Eurasian Collared-Dove (Streptopelia decaocto) (Scheidt and Hurlbert, 2014), and Wild Turkey (Meleagris gallopavo) (Foster et al., 2002). Exploring distribution shifts across many species may help to understand if there are species characteristics related to expansion or contraction.
One aspect of species that may drive how they respond to anthropogenic change is niche specialization. In some communities, global change has led to biotic homogenization driven by replacement of specialist with generalist species (La Sorte and McKinney, 2007; Zwiener et al., 2017; Finderup Nielsen et al., 2019). Because species can be generalists on certain niche axes and specialists on others, considering multiple dimensions of species niche specialization may help in developing an understanding of which species become “winners” or “losers,” or species that benefit from or are vulnerable to anthropogenic change (Dornelas et al., 2019). This may be particularly important in determining species range responses. Changes in range extent may be driven by climatic tolerances, for example, in studies of invasive bird species geographical range size is strongly correlated with introduction success (Blackburn and Duncan, 2001). We may expect species with broader climatic niches to also be more likely to successfully expand to new sites as climate changes (Di Marco and Santini, 2015). However, range occupancy is a result of local process related to habitat preferences of species (Lawton and Woodroffe, 1991), and we might expect habitat niche breadth to better predict changes in range occupancy, especially in areas of increasing human influence. Niches may be conceptualized by the resources utilized by species (MacArthur and Levins, 1967), or alternatively defined by where populations of a species persist (Chase and Leibold, 2003). In this study, we consider dimensions of species niches that draw from both of these different concepts (species diet describing resource utilization, while habitat specialization and climatic tolerance are based on areas of population persistence).
Here, we use annual observations and trend estimates of 77 breeding bird species from the North American Breeding Bird Survey (Pardieck et al., 2018) to examine shifts in species distributions over the previous half-century. We measure changes in species range extent, range occupancy, and population trend over time to characterize shifts in species ranges through multiple dimensions. We then use structural equation modeling to investigate how species niche breadth along multiple axes (climate, diet, and habitat) may explain species range changes over the time period. Finally, we contrast species niche breadth with other variables that might explain range shifts such as body size and migratory distance, to determine which species characteristics are most important in determining range shifts across species.
Materials and methods
Data sources
We obtained occurrence records for North American breeding bird species from the Breeding Bird Survey (BBS; Pardieck et al., 2018), which consists of point count observations made by trained volunteers across the United States and Canada annually during the breeding season (typically May, June, or July). On each survey route, volunteers conduct 50 3-min point counts evenly spaced along a 40-km track, recording all species they see or hear during the survey time period. To measure changes in species ranges over multiple decades, we retained BBS routes that were surveyed in two five-year time windows: 1976–1980 and 2013–2017. We selected these time windows in order to capture as long a time series as possible without sacrificing too much geographic coverage of routes, especially in the early time period. Each survey route had to be surveyed at least three of the five years in both time windows to be included in the analysis. There were 655 BBS routes that fit these criteria and were included in the analysis.
We only included bird species in the analysis for which >50% of the breeding range (based on range maps from BirdLife International1) fell within the BBS survey area (based on a convex hull of BBS routes that met criteria specified above). We did this because we are unable to accurately characterize range-wide shifts of species that occur primarily in regions outside of the BBS survey area. For each species included in the analysis, we obtained body size data from Dunning (1993), and calculated migratory distance as the great circle distance between the centroids of the wintering and breeding ranges. When a species is a year-round resident throughout its range, migratory distance is 0. There were a total of 16 year-round resident species in our species pool.
Measuring species niche breadth
To measure diet niche, we obtained categorical diet data from EltonTraits (Wilman et al., 2014), and calculated the Shannon evenness index (Shannon, 1948) of the proportion of diet in each category for each species as done in previous studies (e.g., Santini et al., 2019; Huang et al., 2021). Diet categories include invertebrates, endotherms, ecotherms, fish, scavenging, nectar, seeds, and plants.
To measure climate niche breadth, we calculated a hypervolume for each species using warmest quarter temperature and precipitation variables at 1-km resolution from BioClim (Fick and Hijmans, 2017) within each species’ breeding range from the BirdLife range maps using the hypervolume package in R (Blonder et al., 2014). This method captures the range of climatic conditions species occur at in multiple dimensions simultaneously (for example, this measure of niche breadth incorporates information about temperature and precipitation means and variability into a single metric). In practice, climate niche breadth as measured here is not always correlated with range size, especially for species with distributions that span strong precipitation gradients especially in the western United States.
To measure habitat niche breadth, we obtained 3-km occupancy rasters for each species from eBird Status and Trends (Strimas-Mackey et al., 2021), and combined these data with 30-m land cover class data for North America in 2015.2 For each species, we calculated the average occupancy by land cover class for the set of land cover classes occurring within that species’ breeding range, and then calculated a species specialization index (SSI) following Barnagaud et al. (2011) as the standard deviation of habitat-specific occupancy values divided by the mean occupancy across land cover classes. For example, a species with high occupancy in one or two habitat types but low occupancy elsewhere would have a high SSI value, while a species with even occupancy across many habitat types would have a low SSI value. In order for the SSI to have the same interpretability in model coefficients as our measures of diet and climate niche breadth (high values indicating broader niches), we multiplied SSI by –1.
Estimating range responses
Species’ population trend estimates across North America were obtained from published estimates based on BBS data (Pardieck et al., 2018). To estimate shifts in range occupancy and range area, we first mapped BBS survey routes to a 1° by 1° grid, randomly sampling one BBS route per grid cell (e.g., Figure 1A). We did this to account for the increase in BBS route density over the study period, and also from west to east on the continent. We explored various thresholds for routes per grid cell, but found that requiring more than one route per grid cell drastically reduces the number of grid cells with sufficient BBS sampling in western North America. For each species, we measured range occupancy in the first and last time periods as the number of occupied grid cells divided by the total number of grid cells within the breeding range. We measured change in range occupancy as the difference in the proportion of occupied grid cells in the late time period minus the early time period (Figure 1A). Because range occupancy is a proportion of each species’ range as measured by occupied grid cells, it ranges from 0 to 1 for each species regardless of differences in range size.
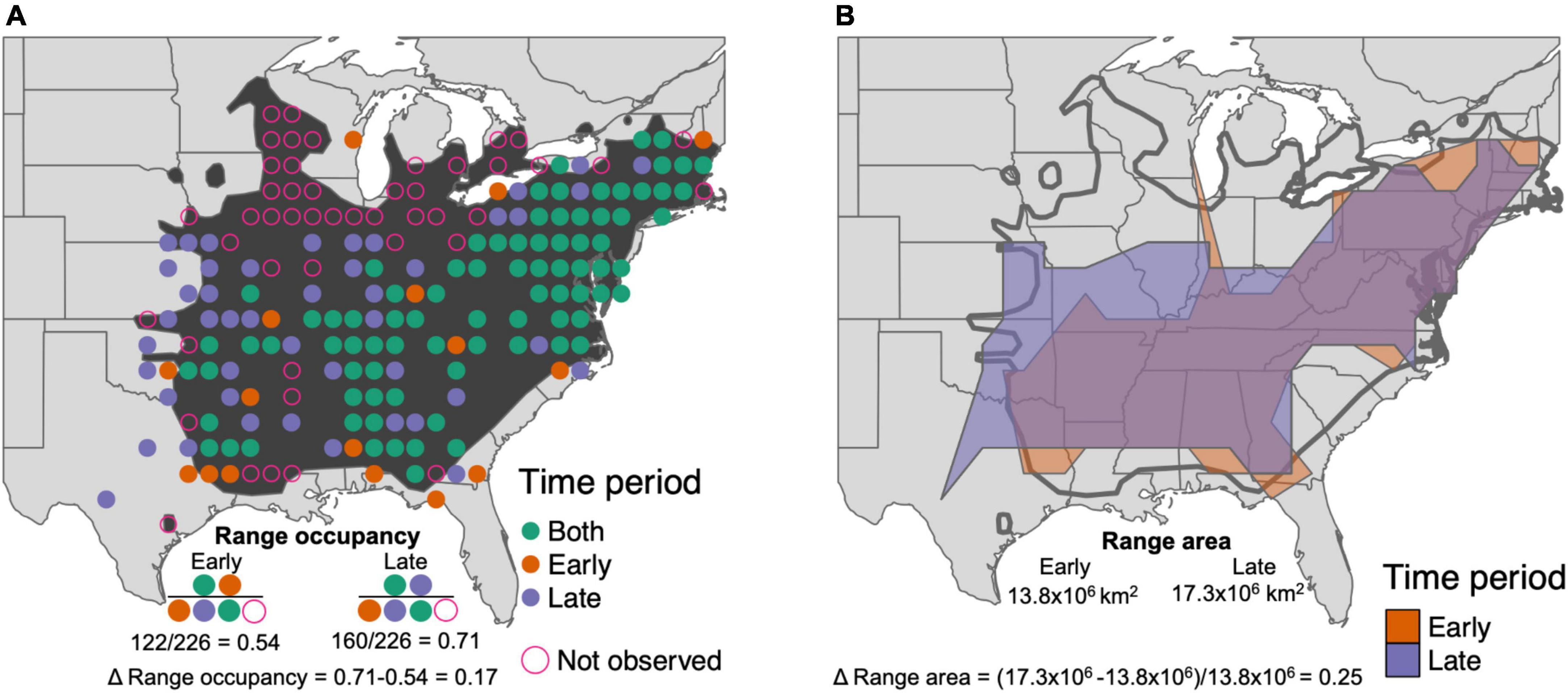
Figure 1. Measuring changes in the range of Louisiana Waterthrush (Parkesia motacilla). (A) Dark shaded polygon shows the breeding range of Louisiana Waterthrush. Circles indicate grid cells with Breeding Bird Survey (BBS) locations. Solid circles indicate locations where Louisiana Waterthrush was observed, in both (green) time periods, just the first time period (orange), or just the later time period (purple). Open pink circles are BBS routes within the breeding range where Louisiana Waterthrush was not observed during either time period. In each time period, the range occupancy of Louisiana Waterthrush is the number of occupied circles over the total number of circles within the breeding range, and the change in range occupancy is the difference between them. (B) Shaded polygons show estimates of Louisiana Waterthrush range area in early (orange) and late (purple) time periods from a concave hull of gridded BBS occurrences. The change in range area is the difference between the later time period area and the early time period area, divided by the early time period area to compare across species with different range sizes. Dark outline shows breeding range.
To measure range area, we first fit a concave hull to grid cell occurrences during each five-year window for species occupying at least ten grid cells (using R function concaveman with concavity = 2; Gombin et al., 2020; Figure 1B). We explored how robust changes in range area were to this concavity parameter, and redid the following analyses with two higher concavity values and two lower concavity values (Supplementary Figure 1). Results were consistent across a majority of concavity values, except for the most extreme cases tested (Supplementary Figure 2). We present results for an intermediate level of concavity, which is flexible enough to resolve species ranges without being too sensitive to individual occupied grid cells in estimating range shapes. We then cropped each concave hull to the species breeding range, to eliminate cases where the concave hull drew unrealistic shapes based on the species range (e.g., showing range area in the Great Plains for a species with a distribution in northern boreal forests that extends into the Appalachian Mountains). Because there were still some cases where species were present outside their breeding range polygon, we checked each cropped region of the concave hulls, and if they contained at least two occupied BBS grid cells, we retained those cropped sections of the concave hull. Following this same procedure for the early and late time window, we then calculated the area of those concave hulls to represent range area. The relative change in range area was then the difference between the area in time two minus time one, divided by the area at time one to facilitate comparison between species with breeding range sizes that vary by many orders of magnitude.
Because the estimation of range occupancy and range area may depend on which BBS route within a grid cell was chosen as representative, we repeated these range occupancy and range area calculations for 500 iterations, randomly sampling one route per grid cell for each iteration. For each iteration, the same set of routes was used for comparing between the two time periods. We used the average change in range occupancy and average change in range area over the 500 iterations, as well as the standard deviation in those metrics, in later analyses.
There are inherent limitations in our methods using BBS observations to capture changes in range area and range occupancy of a species, because the BBS does not encompass the entire range of some species (although as mentioned above it does encompass the majority of the breeding range for all species here), and because uneven sampling throughout the continent may result in patchier estimates of species ranges than the true species distribution. However, the methods we have used to grid BBS records, sample variation in survey occurrences, and estimate range area using varying concave hull algorithms help alleviate some of these issues, and allow us to make use of a uniquely valuable dataset for its spatial and temporal scope.
Statistical analyses
We examined the correlations of each of the three niche breadth measurements and three range response measurements prior to model fitting using the Pearson correlation coefficient.
To estimate the effects of niche specialization on changes in species ranges, we used piecewise structural equation modeling (implemented in the “piecewiseSEM” R package; Lefcheck, 2016). We elected to use SEM because we wanted to explore the relative explanatory power of different niche axes on describing species range responses, but at the same time account for the causal structure likely present between our range response variables (i.e., that species with declining population trends are expected to also have declining range occupancy and range area). We used linear generalized least squares models, considering pathways between each niche specialization measure and each range shift measure, as well as considering the effect of changes in population trend on changes in range occupancy and range area. Because niche specialization may be conserved between more closely related species (this was true especially of habitat specialization, see Supplementary Figure 3), we also included a fixed correlation structure using phylogenetic distances between species. We calculated the model correlation matrix from a consensus (function consensus.tree in R package phytools; Revell, 2022) of 100 phylogenetic trees of the species used in this study obtained from birdtree.org (Jetz et al., 2012), following the method in Lefcheck (2016). To account for uncertainty in response variables, we also included weights based on the inverse of 95% confidence interval widths around estimates of change in range occupancy and range area obtained from our sampling procedure described above, and the inverse of 95% credible interval widths provided for abundance trend indices from the BBS.
We compared the variance explained in range shift metrics by niche specialization compared to other commonly examined traits, in particular body size and migratory distance, using variance partitioning of linear regressions (Legendre and Legendre, 2012) as implemented in the R package vegan (Oksanen et al., 2020). We compared the unique variance explained by body size, migratory distance, and the particular niche specialization variable that explained the most variance in range shifts in the SEM. These analyses were performed subsequent to and separate from the SEM to avoid overfitting the model given the sample size of species included.
Results
The different measures of niche breadth were weakly positively correlated with each other across species, with the highest correlation between climate niche breadth and habitat niche breadth (r = 0.30), and the lowest between diet niche breadth and climate niche breadth (r = –0.02; Figure 2). Diet specialist species were generally insectivores or granivores, and the most generalist species were from Corvidae. Many of the species with the narrowest climate niche breadths were from Parulidae, while the species with the broadest climate niches were two species that occur in the western United States across steep precipitation gradients, Lesser Goldfinch (Spinus psaltria) and Violet-green Swallow (Tachycineta thalassina). The species with the narrowest habitat niche was Lark Bunting (Calamospiza melanocorys), while species with broad habitat niches included American Robin (Turdus migratorius) and Tree Swallow (Tachycineta bicolor).
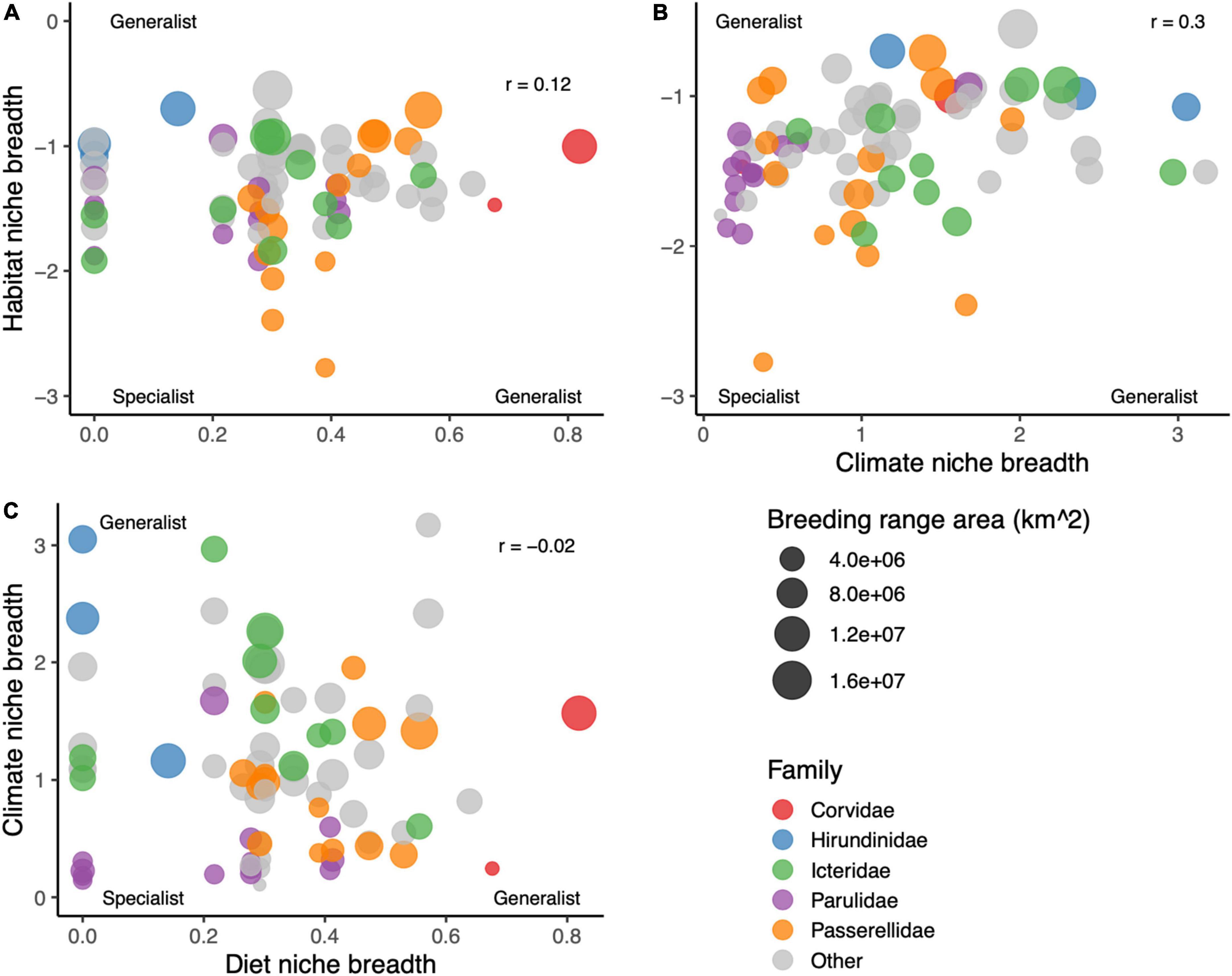
Figure 2. Cross-correlations among the three measures of species niche breadth: (A) habitat versus diet niche breadth, (B) habitat versus climate niche breadth, and (C) climate versus diet niche breadth. Each species’ dot is sized by the breeding range area of the species, and colored by family.
Change in range occupancy, change in range area, and population trend were all positively correlated with each other (0.34 < r < 0.56, all p < 0.05; Figure 3). The distributions of changes in range occupancy and range area were centered close to zero, while the median population trend of the species in this study was –0.52%/year (Figure 3).
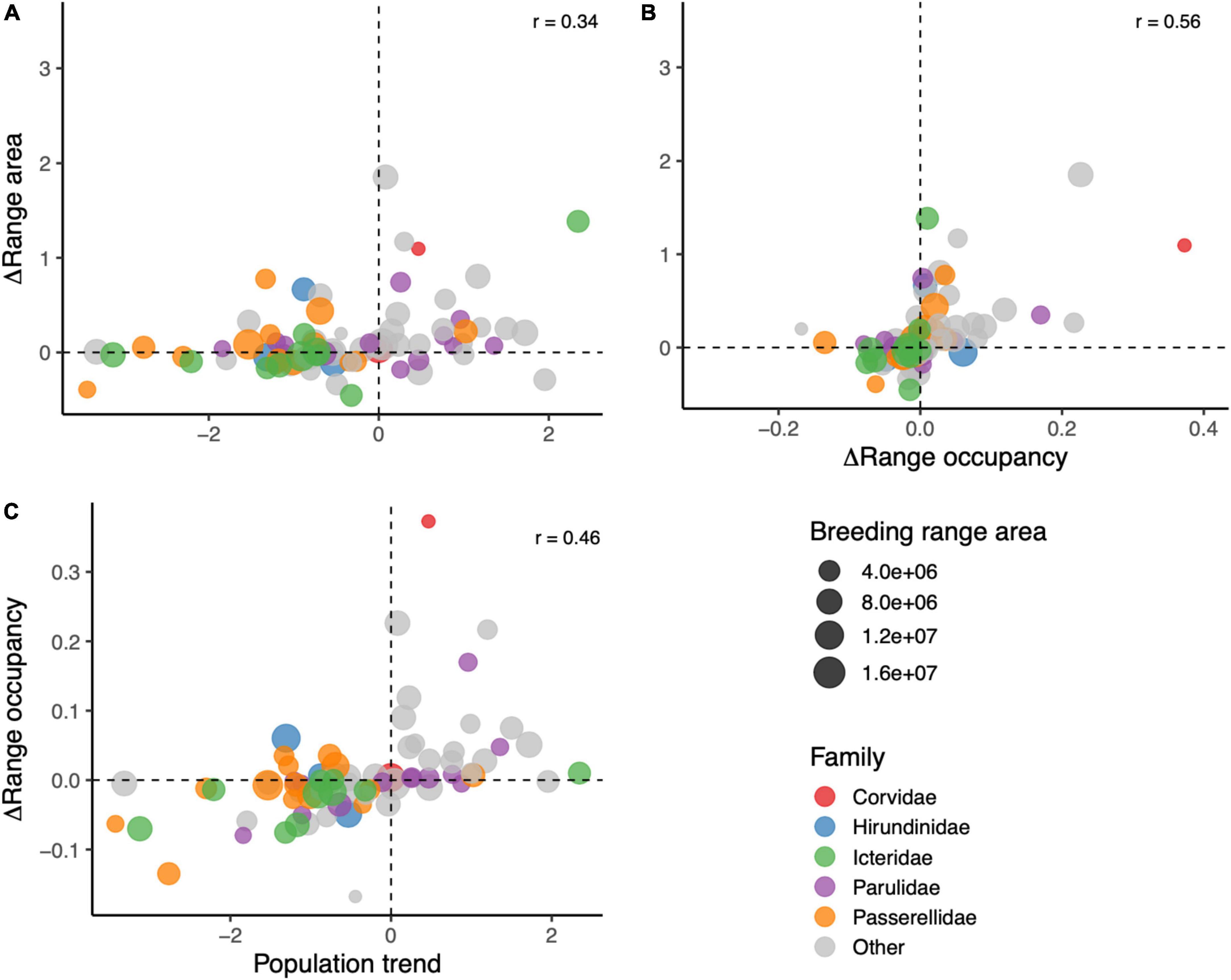
Figure 3. Cross-correlations of different measurements of changes in species distributions over time. (A) Compares relative change in range area and population trend (%/year), (B) compares relative change in range area and absolute change in range occupancy, and (C) compares absolute change in range occupancy and population trend. Each species dot is sized by the size of the species breeding range, and colored by family.
The SEM provides estimates of the relative path strengths among our various niche breadth and range response variables, as well as the total variation explained in each range response. Out of the three response variables, these predictor variables collectively explained the most variation in change in range occupancy (R2 = 0.33), while the predictor variables explained 23% of the variation in change in range occupancy and 19% of the variation in population trend (Figure 4). Climatic generalist species and species with positive population trends showed the strongest increases in range area (p = 0.029 and p < 0.001, respectively), and species with positive population trends also showed strong increases in range occupancy (p < 0.001; Figure 4). We also found that population trend increased with increasing diet niche breadth (p < 0.001; Figure 4).
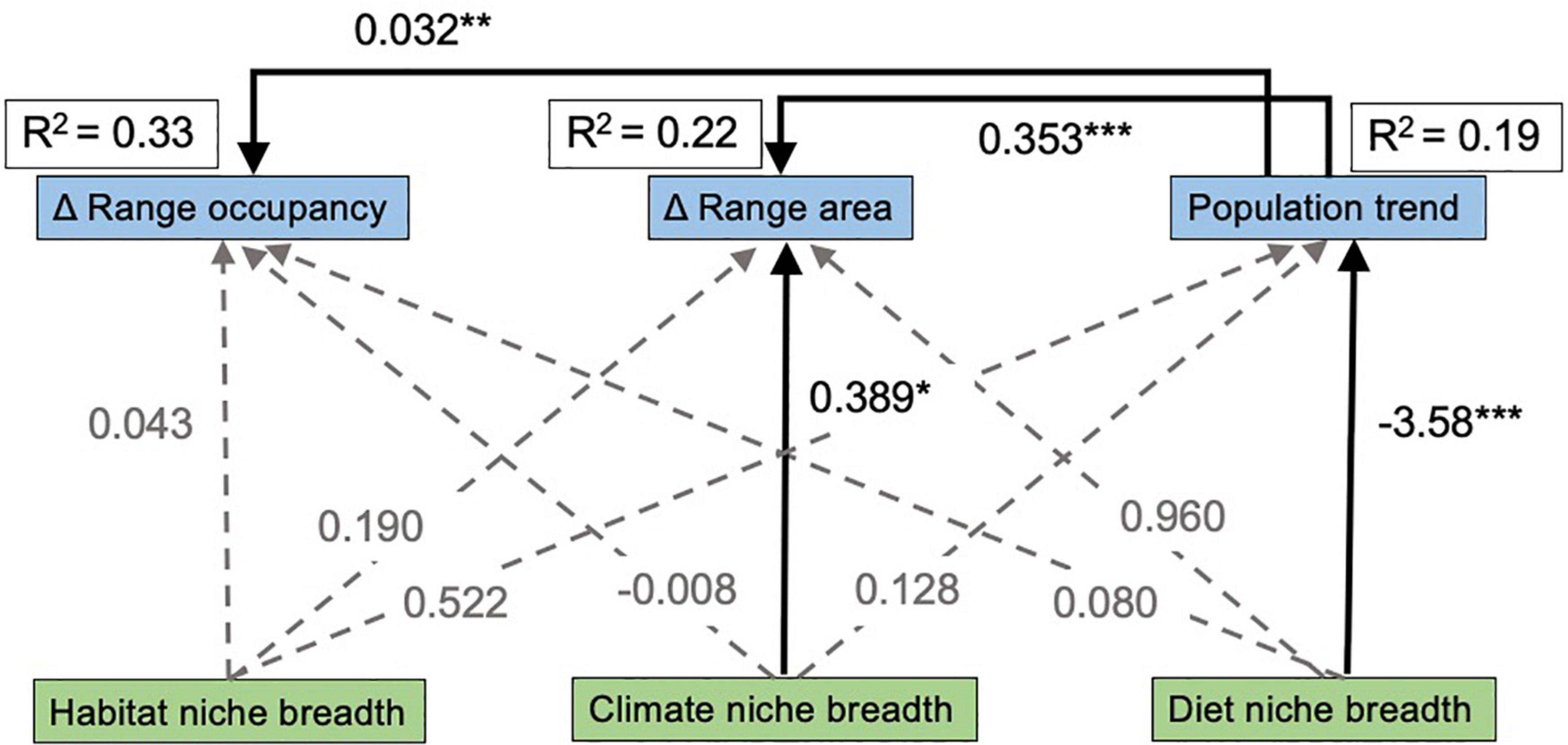
Figure 4. Path diagram showing results of structural equation model relating species niche breadth to changes in species ranges. Black, solid lines indicate significant relationships, while gray, dotted lines indicate no support for a causal path between those variables. Point estimates of the effect size for each directional relationship are next to each line, with significance levels indicated: p < 0.05 *, p < 0.01 **, p < 0.001 ***. The overall R2 for each response variable is outlined in a box next to each variable name.
Relative to migratory distance and body size, the best performing niche breadth measure explained more variation in change in range area, however, migratory distance explained more variation in change in population trend than diet niche breadth and explained only slightly less variation in the change in range occupancy than habitat niche breadth (Figure 5). Longer migratory distance was associated with stronger declines in population trend. There was little shared variance between migratory distance, body size, and species niche breadth in explaining the response variables. Overall R2 estimates presented here are lower than R2 estimates from the SEM analysis because these models do not incorporate phylogenetic signal, and negative values are possible due to correlations between variables. Notably, population trend was least well explained by these species traits, while the most variation in change in range area was explained by these predictors (Figure 5).
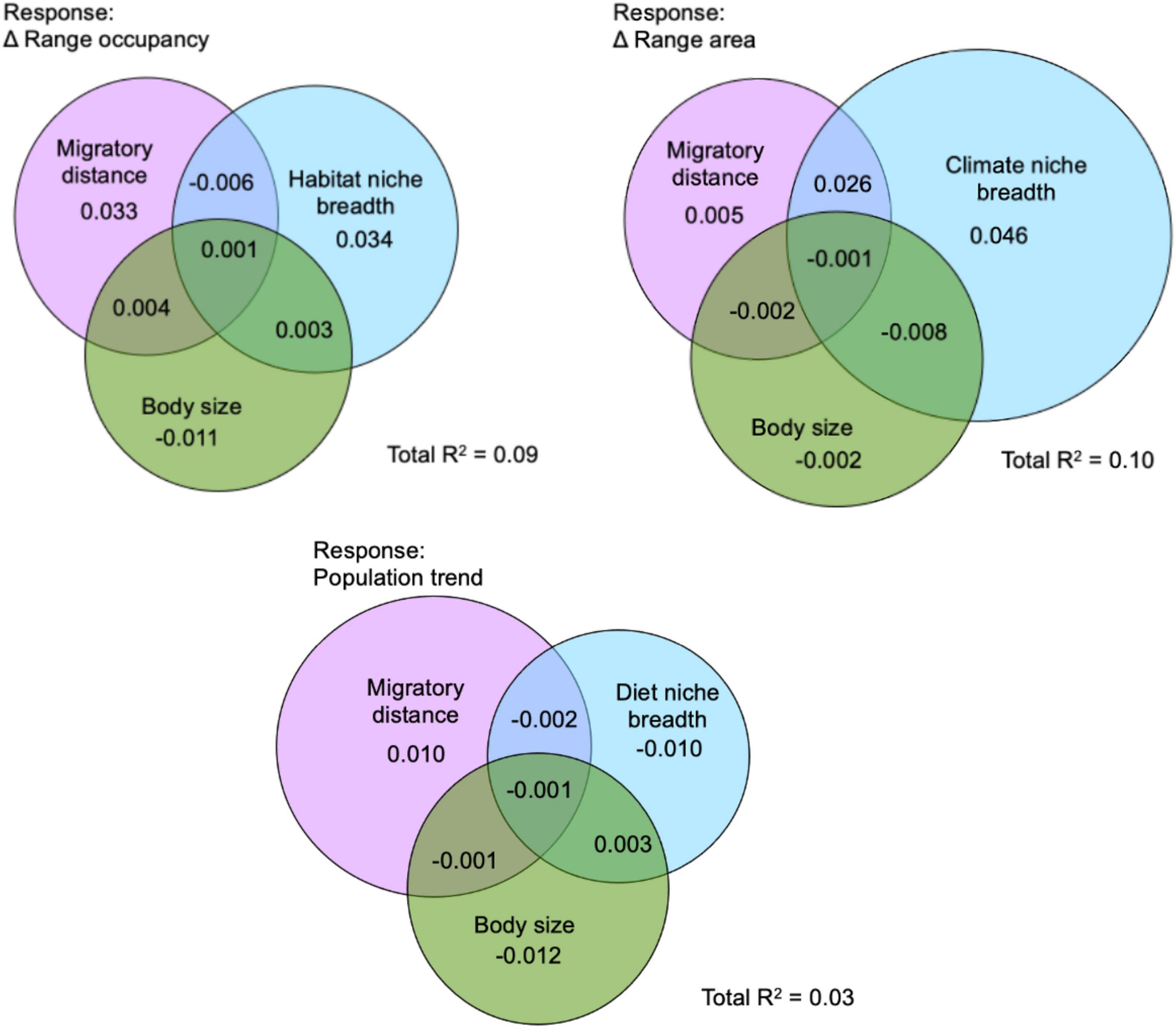
Figure 5. Variance partitioning of species range shifts explained by species migratory distance, body mass, and the niche breadth axis which showed the strongest effect on the three response variables in a structural equation model. For population trend, diet niche breadth was used. For change in range area, climate niche breadth was used. For change in range occupancy, habitat niche breadth was used. The Venn diagrams indicate unique variance in the responses explained by each of the predictor variables, with overlapping regions showing shared variances. Negative variance partitions can result from correlated variables.
Discussion
We characterized three different dimensions by which species have shifted in distribution or abundance over the past half century, and found that change in range area and population trend could be explained by some measure of niche breadth. In line with predictions, climate specialists were more likely to decline in overall range area. Species with declining population trends also showed declines in range area and range occupancy, an example of local scale population processes scaling up to distributional shifts. Additionally, diet generalists were more likely to experience population declines than diet specialists. Climate niche breadth explained more variation in change in range area than body size or migratory distance, while migratory distance explained more variation in population trend and change in range occupancy than niche breadth or body size, indicating divergences between longer and shorter distance migrants and resident species. These two aspects of species may be useful in predicting winner or loser species from anthropogenic change.
Our results indicating range contractions in climate specialist species is in line with prior work indicating that specialist species in particular are threatened by global anthropogenic change (Gilchrist, 1995; Clavel et al., 2011). Species with broader climate niches may be more likely to persist in areas with changing climates, and be able to colonize new areas within their range of climate tolerance. As a result of climate change, specialist species may decline due to an inability to persist in areas of their range that are no longer climatically suitable. However, as this study did not explore particular drivers of environmental change and cannot distinguish between different sources of anthropogenic change.
While our finding that diet generalist species were more likely to be declining in population was unexpected given declines in foliage-gleaning insectivores (Jones et al., 2003), the species with the strongest declining population trends driving this result are Savannah Sparrow (Passerculus sandwichensis) and Purple Finch (Haemorhous purpureus). However, these species may be declining for reasons not directly related to diet, such as interspecific competition between Purple Finches and House Finches (Haemorhous mexicanus) (Wootton, 1987) and decreases in suitable open, grassy habitats for Savannah Sparrow (Rockwell et al., 2003). Furthermore, the diet data available to characterize diet niche was somewhat coarse (e.g., a single “invertebrates” category for all insectivorous birds despite the complexity within this guild), and characterizations of the diet niche will hopefully improve as more detailed data becomes available (Hurlbert et al., 2021).
An association between declining population trends and shrinking range area is expected when population declines are widespread throughout a species range. Species undergoing population declines may also retract first from range edges if those marginal regions are areas where environmental conditions are the least favorable (Martínez-Meyer et al., 2013; de Medeiros et al., 2018). We also found some species increasing in population trend and increasing in range area, including two species with southerly distributions, Great-Tailed Grackle (Quiscalus mexicanus) and Fish Crow (Corvus ossifragus). Our finding that population trend positively predicts changes in range occupancy also suggests the importance of the scaling of local population processes up to infilling of species ranges at broader scales, and is consistent with expectations based on positive abundance-occupancy relationships (Gaston et al., 2000).
While we found that niche breadth explained more variance in change in range area than body mass or migratory distance, there was substantial variance unexplained by all three of these species characteristics. Some research has hypothesized that species traits are bad predictors of range shifts generally (Beissinger and Riddell, 2021), which might limit their utility in predicting winner or loser species more broadly. Our analysis is also limited by the coarse way in which we characterized species ranges (at 1° by 1° latitude-longitude cells) due to data availability. This precludes our study from examining finer scale shifts in species distributions at range margins, and from understanding very fine scale shifts in range occupancy which happen at the scale of local sites. Land use change may impact species most at local scales by impacting availability of suitable habitats (Di Cecco and Hurlbert, 2022), however, the sensitivity of these analyses to detect local scale patterns was likely limited by the spatial resolution, and future work focused at a finer scale could provide a more robust investigation into whether habitat specialization impacts range occupancy. Because of our thresholds for data availability for each species, our species pool is also biased toward more common, larger ranged species and underrepresents boreal species, which may impact the generalizability of these results.
Our study highlights the associations between niche specialization and declines in range area and occupancy, particularly for climatic and habitat specialist species. While we found that niche breadth was important in explaining species range changes, other processes not included in these analyses undoubtedly play a major role as well, such as specific matches between species habitat preferences and land use change regimes (Di Cecco and Hurlbert, 2022), biotic interactions (Snell Taylor et al., 2020), or habitat and climate change on the wintering grounds for migratory species. Future work in this area may expand on these analyses to describe species range dynamics at finer resolutions, particularly changes in range occupancy and at range margins. Further exploration of the importance of different traits may also provide insights into “winner” and “loser” species under global change.
Data availability statement
The original results presented in the study are included in the article/Supplementary material. Code and data used to generate figures are archived at doi: 10.5281/zenodo.6977295. Further inquiries can be directed to the corresponding author.
Author contributions
GD and AH conceptualized the study. GD conducted the analyses and wrote the first draft of the manuscript. Both authors contributed substantially to manuscript revision.
Funding
GD was supported by a dissertation completion fellowship from the UNC Chapel Hill Graduate School. This project was also funded by the National Science Foundation (grant no. 1702708 to AH).
Acknowledgments
We thank the thousands of skilled observers who have contributed to the Breeding Bird Survey and eBird, without whom this work would not have been possible.
Conflict of interest
The authors declare that the research was conducted in the absence of any commercial or financial relationships that could be construed as a potential conflict of interest.
Publisher’s note
All claims expressed in this article are solely those of the authors and do not necessarily represent those of their affiliated organizations, or those of the publisher, the editors and the reviewers. Any product that may be evaluated in this article, or claim that may be made by its manufacturer, is not guaranteed or endorsed by the publisher.
Supplementary material
The Supplementary Material for this article can be found online at: https://www.frontiersin.org/articles/10.3389/fevo.2022.921480/full#supplementary-material
Footnotes
- ^ www.birdlife.org
- ^ http://www.cec.org/north-american-environmental-atlas/land-cover-30m-2015-landsat-and-rapideye/
References
Barnagaud, J. Y., Devictor, V., Jiguet, F., and Archaux, F. (2011). When species become generalists: On-going large-scale changes in bird habitat specialization. Glob. Ecol. Biogeogr. 20, 630–640. doi: 10.1111/j.1466-8238.2010.00629.x
Beissinger, S. R., and Riddell, E. A. (2021). Why are species’ traits weak predictors of range shifts? Annu. Rev. Ecol. Evol. Syst. 52, 47–66. doi: 10.1146/annurev-ecolsys-012021-092849
Blackburn, T. M., and Duncan, R. P. (2001). Determinants of establishment success in introduced birds. Nature 414, 195–197. doi: 10.1038/35102557
Blonder, B., Lamanna, C., Violle, C., and Enquist, B. J. (2014). The n-dimensional hypervolume. Glob. Ecol. Biogeogr. 23, 595–609. doi: 10.1111/geb.12146
Bregman, T. P., Sekercioglu, C. H., and Tobias, J. A. (2014). Global patterns and predictors of bird species responses to forest fragmentation: Implications for ecosystem function and conservation. Biol. Conserv. 169, 372–383. doi: 10.1016/j.biocon.2013.11.024
Chase, J. M., and Leibold, M. A. (2003). Ecological niches: Linking classical and contemporary approaches. Chicago, IL: University of Chicago Press. doi: 10.7208/chicago/9780226101811.001.0001
Clavel, J., Julliard, R., and Devictor, V. (2011). Worldwide decline of specialist species: Toward a global functional homogenization? Front. Ecol. Environ. 9, 222–228. doi: 10.1890/080216
de Medeiros, C. M., Hernández-Lambraño, R. E., Ribeiro, K. A. F., and Sánchez Agudo, J. Á (2018). Living on the edge: Do central and marginal populations of plants differ in habitat suitability? Plant Ecol. 219, 1029–1043. doi: 10.1007/s11258-018-0855-x
Di Cecco, G. J., and Hurlbert, A. H. (2022). Anthropogenic drivers of avian community turnover from local to regional scales. Glob. Change Biol. 28, 770–781. doi: 10.1111/gcb.15967
Di Marco, M., and Santini, L. (2015). Human pressures predict species’ geographic range size better than biological traits. Glob. Change Biol. 21, 2169–2178. doi: 10.1111/gcb.12834
Dornelas, M., Gotelli, N. J., Shimadzu, H., Moyes, F., Magurran, A. E., and McGill, B. J. (2019). A balance of winners and losers in the Anthropocene. Ecol. Lett. 22, 847–854. doi: 10.1111/ele.13242
Fick, S. E., and Hijmans, R. J. (2017). Worldclim 2: New 1-km spatial resolution climate surfaces for global land areas. Int. J. Climatol. 37, 4302–4315. doi: 10.1002/joc.5086
Finderup Nielsen, T., Sand-Jensen, K., Dornelas, M., and Bruun, H. H. (2019). More is less: Net gain in species richness, but biotic homogenization over 140 years. Ecol. Lett. 51, 1650–1657. doi: 10.1111/ele.13361
Foster, D. R., Motzkin, G., Bernardos, D., and Cardoza, J. (2002). Wildlife dynamics in the changing New England landscape. J. Biogeogr. 29, 1337–1357. doi: 10.1046/j.1365-2699.2002.00759.x
Gaston, K. J., Blackburn, T. M., Greenwood, J. J. D., Gregory, R. D., Quinn, R. M., and Lawton, J. H. (2000). Abundance-occupancy relationships. J. Appl. Ecol. 37, 39–59. doi: 10.1046/j.1365-2664.2000.00485.x
Gilchrist, G. W. (1995). Specialists and generalists in changing environments. I. Fitness landscapes of thermal sensitivity. Am. Nat. 146, 252–270. doi: 10.1086/285797
Gombin, J., Vaidyanathan, R., Agafonkin, V., and Mapbox. (2020). Package ‘concaveman’ v 1.1.0. Available online at: https://cran.r-project.org/web/packages/concaveman/concaveman.pdf (accessed July 22, 2022).
Halkin, S. L., and Linville, S. U. (1999). Northern Cardinal (Cardinalis cardinalis): The birds of North America. Acad. Nat. Sci., 1999. 440:32. doi: 10.2173/tbna.440.p
Huang, S., Tucker, M. A., Hertel, A. G., Eyres, A. and Albrecht, J. (2021). Scale-dependent effects of niche specialisation: the disconnect between individual and species ranges. Ecol. Lett. 24, 1408–1419. doi: 10.1111/ele.13759
Hurlbert, A. H., Olsen, A. M., Sawyer, M. M., and Winner, P. M. (2021). The avian diet database as a source of quantitative information on bird diets. Sci. Data 8, 1–5. doi: 10.1038/s41597-021-01049-9
Hurlbert, A. H., and White, E. P. (2007). Ecological correlates of geographical range occupancy in North American birds. Glob. Ecol. Biogeogr. 16, 764–773. doi: 10.1111/j.1466-8238.2007.00335.x
Illán, J. G., Thomas, C. D., Jones, J. A., Wong, W. K., Shirley, S. M., and Betts, M. G. (2014). Precipitation and winter temperature predict long-term range-scale abundance changes in Western North American birds. Glob. Change Biol. 20, 3351–3364. doi: 10.1111/gcb.12642
Jetz, W., Thomas, G. H., Joy, J. B., Hartmann, K., and Mooers, A. O. (2012). The global diversity of birds in space and time. Nature 491, 444–448. doi: 10.1038/nature11631
Jones, J., Doran, P. J., and Holmes, R. T. (2003). Climate and food synchronize regional forest bird abundances. Ecology 84, 3024–3032. doi: 10.1890/02-0639
La Sorte, F. A., and McKinney, M. L. (2007). Compositional changes over space and time along an occurrence-abundance continuum: Anthropogenic homogenization of the North American avifauna. J. Biogeogr. 34, 2159–2167. doi: 10.1111/j.1365-2699.2007.01761.x
La Sorte, F. A., and Thompson, F. R. III (2007). Poleward shifts in winter ranges of North American birds. Ecology 88, 1803–1812. doi: 10.1890/06-1072.1
Lawton, J. H., and Woodroffe, G. L. (1991). Habitat and the distribution of water voles: Why are there Gaps in a Species’. Range? Br. Ecol. Soc. 60, 79–91. doi: 10.2307/5446
Lefcheck, J. S. (2016). piecewiseSEM: Piecewise structural equation modelling in r for ecology, evolution, and systematics. Methods Ecol. Evol. 7, 573–579. doi: 10.1111/2041-210X.12512
MacArthur, R., and Levins, R. (1967). Divergence of coexisting species. Am. Nat. 101, 377–385. doi: 10.1086/282505
Martínez-Meyer, E., Díaz-Porras, D., Peterson, A. T., and Yáñez-Arenas, C. (2013). Ecological niche structure and rangewide abundance patterns of species. Biol. Lett. 9:20120637. doi: 10.1098/rsbl.2012.0637
Mason, S. C., Palmer, G., Fox, R., Gillings, S., Hill, J. K., Thomas, C. D., et al. (2015). Geographical range margins of many taxonomic groups continue to shift polewards. Biol. J. Linnean Soc. 115, 586–597. doi: 10.1111/bij.12574
Oksanen, J., Blanchet, F. G., Friendly, M., Kindt, R., Legendre, P., Mcglinn, D. J., et al. (2020). Package “vegan”: Community ecology package, version 2. Available online at: https://cran.r-project.org/package=vegan (accessed July 22, 2022).
Pardieck, K. L., Ziolkowski, D. J. Jr., Lutmerding, M., and Hudson, M.-A. R. (2018). North American breeding bird survey dataset 1966-2017, version 2017.0.
Parmesan, C., and Yohe, G. (2003). A globally coherent fingerprint of climate change impacts across natural systems. Nature 421, 37–42. doi: 10.1038/nature01286
Revell, M. L. J. (2022). Package “phytools” v 1.0-3. Available online at: https://cran.r-project.org/web/packages/phytools/phytools.pdf (accessed July 22, 2022).
Rockwell, R. F., Witte, C. R., Jefferies, R., and Weatherhead, P. J. (2003). Response of nesting savannah sparrows to 25 years of habitat change in a snow goose colony. Écoscience 10, 33–37. doi: 10.1080/11956860.2003.11682747
Rosenberg, K. V., Dokter, A. M., Blancher, P. J., Sauer, J. R., Smith, A. C., Smith, P. A., et al. (2019). Decline of the North American avifauna. Science 366, 120–124. doi: 10.1126/science.aaw1313
Santini, L., González-Suárez, M., Russo, D., Gonzalez-Voyer, A., von Hardenberg, A. and Ancillotto, L. (2019). One strategy does not fit all: determinants of urban adaptation in mammals. Ecol. Lett. 22:365–376. doi: 10.1111/ele.13199
Scheidt, S. N., and Hurlbert, A. H. (2014). Range expansion and population dynamics of an invasive species: The Eurasian collared-dove (Streptopelia decaocto). PLoS One 9:e111510. doi: 10.1371/journal.pone.0111510
Shannon, C. E. (1948). A mathematical theory of communication. Bell Syst. Techn. J. 27, 623–656. doi: 10.1002/j.1538-7305.1948.tb00917.x
Snell Taylor, S., Umbanhowar, J., and Hurlbert, A. H. (2020). The relative importance of biotic and abiotic determinants of temporal occupancy for avian species in North America. Glob. Ecol. Biogeogr. 29, 736–747. doi: 10.1111/geb.13064
Strimas-Mackey, M., Ligocki, S., Auer, T., and Fink, D. (2021). ebirdst: Tools for loading, plotting, mapping and analysis of eBird status and trends data products. Available online at: https://cornelllabofornithology.github.io/ebirdst/ (accessed July 22, 2022).
Tingley, M. W., Monahan, W. B., Beissinger, S. R., and Moritz, C. (2009). Birds track their Grinnellian niche through a century of climate change. Proc. Natl Acad. Sci. U.S.A. 106, 19637–19643. doi: 10.1073/pnas.0901562106
Townsend Peterson, A., and Martínez-Meyer, E. (2009). Pervasive poleward shifts among North American bird species. Biodiversity 9, 114–116. doi: 10.1080/14888386.2008.9712915
Walther, G.-R., Post, E., Convey, P., Menzel, A., Parmesan, C., Beebee, T. J. C., et al. (2002). Ecological responses to recent climate change. Nature 416, 389–395. doi: 10.1038/416389a
Wilman, H., Belmaker, J., Simpson, J., de la Rosa, S., and Rivadeneira, M. M. (2014). EltonTraits 1.0?: Species-level foraging attributes of the world’s birds and mammals. Ecology 95:2027. doi: 10.1890/13-1917.1
Wootton, J. T. (1987). Interspecific competition between introduced house finch populations and two associated passerine species. Oecologia 71, 325–331. doi: 10.1007/BF00378703
Zuckerberg, B., Woods, A. M., and Porter, W. F. (2009). Poleward shifts in breeding bird distributions in New York state. Glob. Change Biol. 15, 1866–1883. doi: 10.1111/j.1365-2486.2009.01878.x
Zurell, D., Graham, C. H., Gallien, L., Thuiller, W., and Zimmermann, N. E. (2018). Long-distance migratory birds threatened by multiple independent risks from global change. Nat. Clim. Change. 8, 992–996. doi: 10.1038/s41558-018-0312-9
Keywords: niche specialization, birds (Aves), range occupancy, range extent, population trends
Citation: Di Cecco GJ and Hurlbert AH (2022) Multiple dimensions of niche specialization explain changes in species’ range area, occupancy, and population size. Front. Ecol. Evol. 10:921480. doi: 10.3389/fevo.2022.921480
Received: 15 April 2022; Accepted: 29 July 2022;
Published: 30 August 2022.
Edited by:
Frederico Mestre, University of Évora, PortugalReviewed by:
Vinicius Bastazini, University of Evora, PortugalJosé M. Herrera, University of Evora, Portugal
Nuria Galiana Ibáñez, The Centre for Ecological Research and Forestry Applications (CREAF), Spain
Copyright © 2022 Di Cecco and Hurlbert. This is an open-access article distributed under the terms of the Creative Commons Attribution License (CC BY). The use, distribution or reproduction in other forums is permitted, provided the original author(s) and the copyright owner(s) are credited and that the original publication in this journal is cited, in accordance with accepted academic practice. No use, distribution or reproduction is permitted which does not comply with these terms.
*Correspondence: Grace J. Di Cecco, gracedicecco11@gmail.com