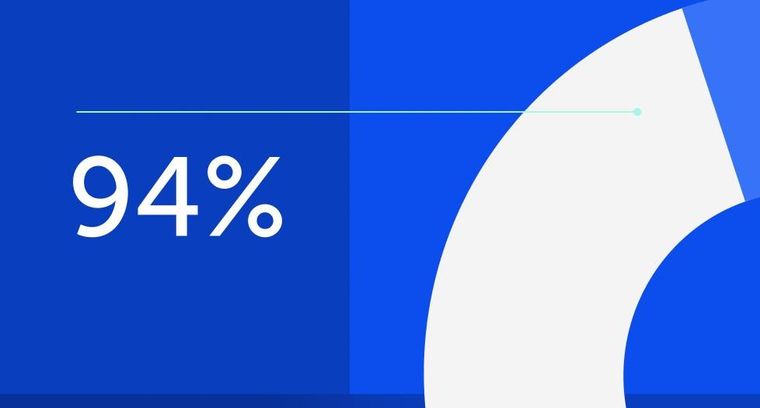
94% of researchers rate our articles as excellent or good
Learn more about the work of our research integrity team to safeguard the quality of each article we publish.
Find out more
ORIGINAL RESEARCH article
Front. Ecol. Evol., 30 June 2022
Sec. Population, Community, and Ecosystem Dynamics
Volume 10 - 2022 | https://doi.org/10.3389/fevo.2022.915199
This article is part of the Research TopicFood Webs and Stable Isotopes, volume IIView all 13 articles
Even in areas as remote as the Southern Ocean, marine organisms are exposed to contaminants that arrive through long-range atmospheric transport, such as mercury (Hg), a highly toxic metal. In previous studies in the Southern Ocean, inter-specific differences in Hg contamination in seabirds was generally related to their distribution and trophic position. However, the Blue Petrel (Halobaena caerulea) was a notable exception among small seabirds, with higher Hg levels than expected. In this study, we compared the Hg contamination of Blue Petrels and Thin-billed Prions (Pachyptila belcheri), which both spend the non-breeding season in polar waters, with that of Antarctic Prions (Pachyptila desolata), which spend the winter in subtropical waters. We collected body feathers and blood samples, representing exposure during different time-frames. Hg concentrations in feathers, which reflect contamination throughout the annual cycle, were related to δ13C values, and varied with ocean basin and species. Blue Petrels from breeding colonies in the southeast Pacific Ocean had much higher feather Hg concentrations than expected after accounting for latitude and their low trophic positions. Both Hg concentrations and δ15N in blood samples of Blue Petrels were much lower at the end than at the start of the breeding period, indicating a marked decline in Hg contamination and trophic positions, and the carry-over of Hg burdens between the wintering and breeding periods. Elevated Hg levels may reflect greater reliance on myctophids or foraging in sea-ice environments. Our study underlines that carry-over of Hg concentrations in prey consumed in winter may determine body Hg burdens well into the breeding season.
Seabirds are often used as bioindicators of marine pollution (Van den Steen et al., 2011; Becker et al., 2016; Thébault et al., 2021). They are long-lived animals, feed at high trophic levels, and thus integrate and bioaccumulate contaminants from the food webs on which they rely (Albert et al., 2019). Often, seabirds nest in accessible breeding colonies, but roam over vast areas of ocean that can thus be monitored. Our knowledge of their diets and at-sea distribution has greatly increased in the last years with the advances in biologging methods that are now suitable for the smallest seabird species (Quillfeldt et al., 2015), trophic tracers such as compound-specific stable isotope analyses (Lorrain et al., 2009; Quillfeldt and Masello, 2020), and metabarcoding from faecal samples (Kleinschmidt et al., 2019).
Among the contaminants that increase in the marine environment due to human activities, mercury (Hg) is a highly toxic non-essential metal that has deleterious effects on the behaviour, neurology, endocrinology and development of wildlife (Scheuhammer et al., 2007; Tan et al., 2009). Released from both natural and anthropogenic sources, Hg reaches remote polar and sub-polar regions through long-range atmospheric transport (Fitzgerald et al., 1998). In seabirds, Hg is incorporated from the food and accumulates in soft tissues such as liver and muscle (Bearhop et al., 2000a; Carravieri et al., 2014a). Birds can excrete up to 90% of the Hg accumulated since the previous moult in the new growing feathers and thus, feathers – which can be sampled non-destructively – are an archive of year-round Hg contamination (Thompson et al., 1998; Albert et al., 2019). Birds may also show a substantial carry-over of Hg among seasons, and slow changes in Hg over time. For example, Double-Crested Cormorants (Phalacrocorax auritus) and Caspian Terns (Hydroprogne caspia) with high Hg exposure in winter still had elevated blood Hg values in summer (Lavoie et al., 2014).
Among seabirds, species with high trophic position in marine food webs have elevated Hg concentrations due to the biomagnification of methylmercury (MeHg), the most bioavailable form of Hg in marine ecosystems (Seco et al., 2021). This pattern has been shown in the seabird community of the subantarctic Kerguelen Islands (Blévin et al., 2013; Carravieri et al., 2014a). In particular, species feeding in colder waters to the south had lower Hg concentrations than species feeding in northern, warmer waters. At the scale of the Southern Hemisphere, such a pattern (higher Hg concentrations in birds feeding in subtropical and subantarctic waters) has been confirmed for diverse species, including penguins, skuas, and albatrosses (Carravieri et al., 2014b,2016, 2017, 2020; Cherel et al., 2018). However, the Blue Petrel (Halobaena caerulea) seems to be a marked exception to this general pattern, as Hg concentrations in tissues are one order of magnitude higher than in other species of small petrels (Bocher et al., 2003).
Blue Petrels and Prions, Pachyptila spp. are a similar size (∼200 g). The largest breeding populations of Blue Petrels are at Diego Ramírez Islands, Chile in the southeast Pacific Ocean (>2 million individuals or ∼1.35 million pairs; Schlatter and Riveros, 1997; Lawton et al., 2006), Kerguelen Islands in the southern Indian Ocean (100,000–200,000 pairs; Weimerskirch et al., 1989) and Marion Island in the Indian Ocean (110,000–180,000 pairs; Dilley et al., 2017). Muscle tissue sampled from Blue Petrels breeding at Kerguelen Islands contains far higher Hg concentrations than expected, given the relatively low Hg levels in epipelagic fish and crustaceans in the same region (Bocher et al., 2003). Proposed explanations include the relative longevity of Blue Petrels (up to 20 years) and thus, Hg bioaccumulation over the long term, and from their consumption of mesopelagic fish (Cherel et al., 2002b), which contain high Hg concentrations (Bustamante et al., 2003; Cipro et al., 2018; Seco et al., 2020). Blue Petrels at Marion Island in the southern Indian Ocean showed the highest feather Hg concentrations reported for the species so far (Becker et al., 2016). At South Georgia, studies reported either relatively high Hg concentrations in feathers of Blue Petrels (Becker et al., 2002) or Hg levels in a similar range to Antarctic Prions (Pachyptila desolata) and Diving Petrels, Pelecanoides spp. (Anderson et al., 2009).
Although Hg in Southern Ocean seabirds has received considerable attention (Anderson et al., 2009; Blévin et al., 2013; Becker et al., 2016; Carravieri et al., 2020), the influence of sea ice on Hg dynamics has not yet been explored. Recent studies identified bacteria of the genus Nitrospina as a potential Hg methylator within sea ice and brine, and proposed that Antarctic waters associated with sea ice can harbour a microbial source of MeHg in the Southern Ocean (Gionfriddo et al., 2016). Thus, total Hg (i.e., inorganic Hg and MeHg) and methylated Hg (MeHg) concentrations are elevated in these zones, related to high atmospheric Hg deposition and subsequent in situ methylation (Gionfriddo et al., 2016). A study of the Hg species distribution suggested that the Southern Ocean Hg cycle is characterized by a net atmospheric Hg deposition on surface waters near the ice edge, and Hg enrichment in brine during sea-ice formation (Cossa et al., 2011). Studies in coastal Antarctica have shown greatly enhanced total Hg concentrations in surface snow at the sea-ice edge adjacent to the freezing ocean surface (McMurdo/Ross Sea region: Brooks et al., 2008; Casey station/East Antarctica: Cossa et al., 2011). The Hg concentrations found in fast ice near Casey station were three orders of magnitude above the concentrations in surface water in the Southern Ocean (Cossa et al., 2011). A seasonal study of elemental and total Hg concentrations in the Antarctic sea-ice environment (Nerentorp Mastromonaco et al., 2016) found that the concentration of total Hg in sea ice halved from winter to spring (average 9.7 ng/l to 4.7 ng/l). A recent analysis has related high winter Hg concentrations to the frequency of katabatic winds, bringing Hg from the Antarctic ice sheet to coastal waters (Yu et al., 2021).
In the present study, we compared Hg concentrations in blood and feathers of Blue Petrels, Antarctic Prions, and Thin-billed Prions (P. belcheri), each at their largest colonies in widely separated oceans. Of the three species, Blue Petrels spend the non-breeding season at the most southerly latitudes (Quillfeldt et al., 2013, 2015; Navarro et al., 2015), and have disproportionately high Hg values (Bocher et al., 2003). We therefore used tracking data to examine if exposure to sea ice may play a part in explaining variability in Hg concentrations. We used stable isotope analyses to determine trophic positions and distributions (water mass) used by each species. In the Southern Ocean, δ13C values in seabird tissues correspond to the location of their foraging habitats (Phillips et al., 2009; Jaeger et al., 2010; Quillfeldt et al., 2010b) and δ15N values increase with trophic position (Cherel et al., 2010). As novel questions, we aimed to test (1) if foraging close to sea-ice-covered polar waters results in higher exposure to Hg, and (2) if there is carry-over of Hg between wintering and breeding grounds.
Blue Petrels, Thin-billed Prions, and Antarctic Prions have wide distributions in the Southern Ocean. We sampled breeding populations in the south-west Atlantic Ocean (Falkland Islands for Thin-billed Prions; South Georgia for Blue Petrels and Antarctic Prions) and in the Indian Ocean (Kerguelen Islands, all three species) (Figure 1). In addition, a population of Blue Petrels was sampled on Diego Ramírez Islands, Chile, southeast Pacific Ocean. In total, we sampled seven populations (Figure 1). Thin-billed Prions breed mainly on the Falkland and Kerguelen Islands. New Island, in the Falkland Islands, is the most important known breeding site for Thin-billed Prions with an estimated two million breeding pairs. South Georgia and Kerguelen are the most important breeding sites (with populations > 1 million) of Antarctic Prions.
Figure 1. Distribution of Blue Petrels, Thin-billed Prions, and Antarctic Prions during primary moult (i.e., the core moult area). Colony sites: Diego Ramirez (DR), South Georgia (SG), Falkland Islands (Malvinas) (FLK), and Kerguelen (KER). Blue Petrels moulting in February between 71°S, 119°W and 67°S, 78°W are most likely birds from the large Diego Ramirez colony (Ryan et al., 2020). Moult takes place around the time of the minimum sea-ice extent (February–April) in Blue Petrels and Thin-billed Prions, and during August–October in Antarctic Prions.
These three petrel species migrate away from their breeding grounds during the non-breeding season, where they segregate latitudinally (Navarro et al., 2015; Quillfeldt et al., 2015). Antarctic Prions migrate to subtropical waters, and Thin-billed Prions and Blue Petrels moult in polar waters (Quillfeldt et al., 2013, 2015; Navarro et al., 2015). The species also show breeding allochrony, with Blue Petrels arriving at colonies in September, Thin-billed Prions in October and Antarctic Prions in November to early December (Quillfeldt et al., 2020). After several days of pair formation, the birds leave on a pre-laying exodus, and return ready for egg-laying and incubation, with the mean start of the first trip by the female in incubation at Kerguelen of 28 October (Blue Petrel), 19 November (Thin-billed Prion) and 26 December (Antarctic Prion) (Quillfeldt et al., 2020).
Differences in habitat use in the breeding season are less pronounced than in winter, and diets largely overlap. The three species are zooplanktivorous, with a preference for crustaceans (Prince, 1980; Cherel et al., 2002a,b; Quillfeldt et al., 2010a), and forage on the surface or up to depths of 5–7 m (Chastel and Bried, 1996; Cherel et al., 2002a; Navarro et al., 2013).
Adult Blue Petrels and the two species of Prions were trapped either at the burrow or by mist net. Fieldwork at Kerguelen was carried out in colonies of Thin-billed Prions and Blue Petrels at Île Mayès (49°28′S, 69°57′E) during incubation, late chick-rearing or post-moult periods (when Blue Petrels return to clean out their burrows) of five breeding seasons (Tables 1, 2). Sampling in 2010/11 was carried out as part of the POLARTOP project (Carravieri et al., 2014a,b) and in 2011/12, blood and feather samples were collected during the deployment and retrieval of geolocator-immersion loggers (Quillfeldt et al., 2015). Antarctic Prions were sampled at Île Verte (49°30′S, 70°02′E; n = 10) in 2011/12. Mist netting of Blue Petrels was carried out at Isla Gonzalo, Diego Ramírez Islands (56°29′S, 68°44′W) in December 2010 to January 2011. Thin-billed Prions were sampled at New Island, Falkland/Malvinas Islands (51°43′S, 61°18′W) in 2006/07 and 2017/18. Blue Petrels and Antarctic Prions were sampled at Bird Island, South Georgia (54°00′S, 38°03′W) in burrows during the austral summer 2010/11, when the incubation period overlaps between the two species, and feathers were also collected from Blue Petrels when geolocators were retrieved in austral summer 2011/12.
Table 1. Summary of stable isotope and mercury data of Blue Petrels (mean ± standard deviation), as well as trophic position (TP) estimates based on linear models (TPLM) or compound-specific isotope analyses of amino acids (TPCSIA).
Table 2. Summary of stable isotope and mercury data of Thin-billed Prions (mean ± standard deviation), as well as trophic position (TP) estimates based on linear models (TPLM) or compound-specific isotope analyses of amino acids (TPCSIA).
We sampled two different tissue types, body feathers and blood. Body feathers, moulted annually, represent Hg accumulated over the annual cycle (Albert et al., 2019). To assess seasonal changes in Hg exposure, we sampled blood at different stages in the breeding season as blood reflects the contamination for the 1−2 previous months (half-life of 30 days in Great Skuas Stercorarius skua: Bearhop et al., 2000a; 40–65 days in Cory’s Shearwaters Calonectris borealis: Monteiro and Furness, 2001). For sample times and sizes see Tables 1–3.
Table 3. Summary of stable isotope and mercury data of Antarctic Prions (mean ± standard deviation), as well as trophic position (TP) estimates based on linear models (TPLM).
Feather samples (body feathers) were stored in individual Ziploc bags. Antarctic Prions moult their primaries towards the end of the non-breeding season, and Blue Petrels and Thin-billed Prions directly after the breeding season (Cherel et al., 2016). Less is known about body feather moult, but this is thought to occur over a longer period. Blue Petrels collected in January (i.e., likely non-breeders or failed breeders) had extensive body moult coinciding with primary and secondary feather moult (Bierman and Voous, 1950), but very few Blue Petrels moult body feathers in winter (Brown et al., 1986). Blue Petrels return to the colony after their moult, mostly in May (Brooke, 2004; own observations from tracking data).
Feathers were cleaned in a chloroform:methanol solution (2:1, v/v) in an ultrasonic bath and rinsed two times in methanol. After 48 h drying at 45°C in an oven, they were cut into tiny fragments with stainless steel scissors. Blood (0.2–0.4 ml) was sampled by puncture of the wing vein and collected using heparinized capillaries, or syringes. Blood was stored in ethanol (Diego Ramírez, Kerguelen 2012/13), or separated by centrifugation, and the pellet of red blood cells was frozen (Kerguelen 2010/11 and 2018/19, Falkland Islands, and South Georgia). Both whole blood and blood cells were freeze-dried and ground to powder for Hg and stable isotope analyses. As Hg from whole blood is mainly found in red blood cells (>95%), it is equivalent to analyse one or the other, when referring to dry mass.
The half-life of isotope turnover for avian red blood cells was 29.8 days in American Crows (Corvus brachyrhynchos) (Hobson and Clark, 1993). For this, blood samples collected from petrels therefore likely represented the diet ingested ca. 2–4 weeks before sampling. After return from the wintering areas, stable isotope ratios in blood quite quickly reach values characteristic of the summer habitat and diet (Cherel et al., 2014; Lavoie et al., 2014). In contrast, there can be substantial carry-over of Hg among seasons and slow changes in the body pool of Hg over time, especially for individuals with high Hg exposure in winter (Lavoie et al., 2014). This suggests a slow depuration rate and storage in internal tissues, such that levels in the blood reflect both recent and past exposure. Renal excretion of MeHg is low and bile excretion is followed by intestinal reabsorption, thus retaining Hg in the organism. Hence, Hg values in blood at a given time may be influenced by previous exposure at distant locations.
Mercury concentrations were determined on aliquots with an Advanced Mercury Analyser spectrophotometer Altec AMA-254 [aliquots: blood ∼2 mg dry weight (dw), feathers ∼1 mg dw] as described in Bustamante et al. (2006). AMA measures total Hg but bird blood and feathers contain virtually 100% methylmercury (Thompson and Furness, 1989; Renedo et al., 2017; Manceau et al., 2021). Measurements were repeated two to three times for each sample, until the relative standard deviation (RSD) was <10%. For each set of samples, accuracy and reproducibility of the results were tested by preparing analytical blanks and performing replicate measurements of certified reference materials (TORT-2: lobster hepatopancreas, certified concentration: 0.27 ± 0.06 μg/g dw; DOLT-5: dogfish liver, certified concentration: 0.44 ± 0.18 μg/g dw; National Research Council of Canada). Measured Hg concentrations for the certified reference materials were: 0.26 ± 0.02 μg/g dw (n = 18) and 0.42 ± 0.01 μg/g dw (n = 15) for TORT-2 and DOLT-5, respectively, corresponding to a recovery rate of 96 ± 2% for TORT-2 and 96 ± 1% for DOLT-5. The limit of detection (LOD) was 0.005 μg/g dw. Hg concentrations are expressed in μg/g dw.
To perform bulk stable isotope analyses, 0.2–0.4 mg of sample was weighed into tin cups. δ13C and δ15N values were determined with a continuous-flow mass spectrometer (Thermo Scientific Delta V Advantage) coupled to an elemental analyser (Thermo Scientific Flash EA 1112). Results are expressed in parts per thousand (‰) in the usual δ notation, relative to Vienna Pee Dee Belemnite for δ13C and atmospheric N2 for δ15N, following the formula:
where R is 13C/12C or 15N/14N, respectively. Measurements of internal laboratory standards were conducted using acetanilide and peptone and indicated an experimental precision of ±0.15 ‰ for both elements.
Compound-specific isotope analyses of amino acids (CSIA-AA) data can provide a good estimate of the trophic position of marine organisms even from temporally and spatially variable environments. CSIA-AA were performed at the UC Davis Stable Isotope facility (United States), as described previously (Quillfeldt and Masello, 2020). Trophic positions (TP) were calculated from the δ15N values of glutamic acid (Glx) and phenylalanine (Phe), using a stepwise trophic discrimination factor (multi-TDFGlx–Phe, for detailed discussion, see Quillfeldt and Masello, 2020), with the following equations:
Due to high analytical costs, only small sample sizes were analysed with CSIA-AA. For Blue Petrels (Table 1), we analysed 10 blood samples and 10 feathers (five from Kerguelen and five from Diego Ramírez, respectively). For Thin-billed Prions (Table 2), we included 20 blood samples (5 from Kerguelen and 15 from New Island: 5 each in 2 years and 2 parts of the season), and 21 feathers (5 from Kerguelen and 16 from New Island: 5 from 2017 to 2018, and 11 from 2006 to 2007).
Trophic positions were calculated as described in Thébault et al. (2021). In the Southern Hemisphere, a latitudinal enrichment in δ15N baseline values occurs from Antarctic to subtropical waters (Jaeger et al., 2010; Quillfeldt et al., 2010b). To correct for this latitudinal effect, we calculated the trophic positions of the birds by applying linear regression models to the relationship between TPCSIA and bulk stable isotope values (δ13C and δ15N). Trophic positions calculated with linear models are referred as TPLM.
Linear regression models were used to test relationships between TPCSIA and bulk stable isotope values (δ13C and δ15N). Models were applied separately for blood samples, both reflecting short-term food intake and with similar TDF – 4.0 ‰ (Quillfeldt and Masello, 2020) and 4.1 ‰ (Hebert et al., 2016), respectively, and feather samples (which reflect trophic ecology at the time of moult). For feathers, the linear regression model was statistically significant (R2 = 0.58, F28,2 = 19.1, p < 0.001), and the following equation was used to calculate trophic positions from bulk stable isotope values:
However, the linear regression model was not statistically significant for blood TPCSIA values (R2 = 0.05, F22,2 = 0.5, p = 0.596). Thus, we did not calculate trophic positions from bulk stable isotope values for blood.
Moulting times and distributions were determined using three steps, as described previously in Cherel et al. (2016): using the information recorded by the geolocator-immersion loggers (i) extraction of daily data on activity using the ACTAVE tool (Mattern et al., 2015), (ii) fitting a Generalized Additive Model (GAM) to the variable ‘on-water’ (i.e., the total time spent on water) separately for each individual, and (iii) calculating the dates when the fitted ‘on-water’ value exceeded 75% of the maximum (which indicates the core moult area; Cherel et al., 2016).
We defined habitat zones following Cherel et al. (2018), based on feather δ13C isoscapes (Jaeger et al., 2010), as Subtropical Zone (STZ): δ13C > −18.3 ‰, Subantarctic Zone (SAZ): δ13C values of −21.2 to −18.3 ‰, and Antarctic Zone (AZ): δ13C < −21.2 ‰. Likewise, in blood, habitat was derived from δ13C as Subtropical Zone (STZ): δ13C > −20.1 ‰, Subantarctic Zone (SAZ): δ13C values of −22.9 to −20.1 ‰, and Antarctic Zone (AZ): δ13C < −22.9 ‰ (Jaeger et al., 2010).
The populations were assigned to the ocean basin where they spend most of their annual cycle. Thus, although Blue Petrels from Kerguelen moult in the Atlantic, and Blue Petrels from South Georgia spend 2 months in winter in the Pacific, they were assigned to the ocean basin of their breeding colony, i.e., Indian and Atlantic Ocean, respectively.
Using geolocator data, we calculated an index of sea-ice concentrations used by tracked birds, obtained through the Environmental Data Automated Track Annotation System (Env-DATA) on Movebank1. Sea-ice values (ECMWF Interim Full Daily SFC Sea Ice Cover, scale 0–1) for each location were summarized by individual and month. From these, we calculated the maximum value and mean annual sea-ice concentration. The maximum values were reached in the weeks before the breeding season, and we tested for a relationship with Hg values in blood collected in the early breeding season. An exception was the Thin-billed Prions from New Island, where the sea-ice maximum was reached earlier in the winter; however, this population was excluded from analyses as Hg was not measured in feathers and blood of tracked animals. As body feathers integrate the Hg contamination over the year, we tested for a relationship with the mean annual sea-ice values of tracked birds during the breeding and non-breeding season.
Data were analysed in R4.1.0., and visualized in R and in ArcGIS 10.2.2. Normality was tested using Shapiro tests and QQ plots. Stable isotopes and Hg values were not normally distributed, and univariate statistics were carried out using non-parametric tests, while the data were successfully transformed using transform Tukey in the R package “rcompanion” before carrying out multivariate statistics such as linear models. A comparison of the model outputs did not show any large differences between models using transformed and untransformed data. Thus, effect plots are given from models of untransformed data to enhance readability, i.e., showing the actual scale of the data.
As Hg concentrations differed among the species and did not show a linear relationship with stable isotope values, we ran GAMs in the R package “mgcv”, separately for the species. As proxies for the trophic position, we included either δ15N or the estimated trophic position based on the linear regression of feather δ15N and δ13C values (TPLM). As proxies for distribution, we included either δ13C or the distribution zone. We checked all GAMs for model convergence and random distribution of residuals, and reported statistics (effective degrees of freedom and p-values) for the GAMs run separately for each parameter.
We further ran a model selection separately for each species with the dredge function in the R package MuMIn on the full models for feathers: gam(THg.feathers ∼ s(TP_est) + s(δ13C.feathers) + s(δ15N.feathers) + habitat + ocean), and for blood: gam(THg.blood ∼ s(δ13C.blood) + s(δ15N.blood) + season + habitat + ocean). For the selected best models, we report the coefficients and, as a measure of effect size, calculated eta squared values (η2) obtained with the EtaSq function in the R package “DescTools”. Unless indicated otherwise, mean values are given ± SD.
The three species and their different populations had distinct moulting sites and winter distributions (Figures 1, 2). Blue Petrels and Thin-billed Prions moulted south of the Antarctic Polar Front, and Antarctic Prions to its north. In all three species, birds from Kerguelen started the core period of moult later than birds from the south-west Atlantic colonies (mean 12 days, 11 days, and 28 days later in Blue Petrels, Thin-billed Prions, and Antarctic Prions, respectively: Supplementary Figure 1 and Supplementary Table 1). Blue Petrels from South Georgia and Kerguelen moulted in the Southern Ocean between 20°W and 30°E, overlapping between 20°W and 10°E (Figure 1). Based on the immersion data, the core moult phase took place on average between early February and late March in Blue Petrels from South Georgia, and between mid-February and early April in Blue Petrels from Kerguelen (Supplementary Figure 1 and Supplementary Table 1). The latitudes during the breeding and moulting period differed only slightly for Blue Petrels from Kerguelen and South Georgia (Supplementary Figure 2), whereas ship-based observations indicate that Blue Petrels from Diego Ramírez moult at higher latitudes (c. 70°S; Ryan et al., 2020). Blue Petrels from Kerguelen and South Georgia spent the mid-winter mostly south of 55°S (Figure 2 and Supplementary Figure 2). Although both populations moulted in the Atlantic Ocean, subsequent longitudinal movements were in opposite directions; birds from Kerguelen returned to the Indian Ocean, whereas those from South Georgia entered the Pacific Ocean in mid-winter (July–August) (Supplementary Figure 2).
Figure 2. (A) Latitudinal distribution of geolocator tracked Blue Petrels, Thin-billed Prions, and Antarctic Prions during the mid-winter months (June to August), and a mercury transect shown for comparison: (B) total mercury and (C) methyl mercury concentrations along a transect from Hobart to the Antarctic (adapted from Figures 3 and 6 in Cossa et al., 2011). STZ, Subtropical Zone; STF, Subtropical Front; SAZ, Subantarctic Zone; SAF, Subantarctic Front; PFZ, Polar Frontal Zone; SPF, Polar Front; AZ, Antarctic Zone; SACCF, Southern Antarctic Circumpolar Current Front; SZ, Southern Zone. Note that the position of the fronts changes longitudinally (e.g., see Polar Front in Figure 1).
The moulting areas of Thin-billed Prions were southeast and southwest of the Falkland Islands, and most birds from the Falklands and Kerguelen moulted in waters between 25°W and 30°E, overlapping between 0° and 30°E (Figure 1). The core moult period was between late February and early April in Thin-billed Prions from the Falkland Islands, and between early March and late April in Thin-billed Prions from Kerguelen (Supplementary Figure 1 and Supplementary Table 1). The year-round latitudinal distribution was very similar for Thin-billed Prions from both colonies (Supplementary Figure 3), whereas longitudinal movements were in opposite directions (Supplementary Figure 3). Thin-billed Prions spent the mid-winter mostly between 45°S and 55°S, intermediate between the other two species (Figure 2 and Supplementary Figure 3).
Antarctic Prions generally moulted north of the Antarctic Polar Front, and the moult areas of the birds from South Georgia and Kerguelen did not overlap (Figure 1). The core moult took place in the pre-breeding period, between late July and mid-October in Antarctic Prions from South Georgia, and between early August and late October in Antarctic Prions from Kerguelen (Supplementary Figure 1 and Supplementary Table 1). The latitudes during the breeding period were slightly lower, and those in the winter and moult periods slightly higher, for Antarctic Prions from Kerguelen (Figure 2 and Supplementary Figure 4), and longitudinal movements were relatively short in this species (Supplementary Figure 4). Antarctic Prions spent the mid-winter mostly north of 45°S (Figure 2 and Supplementary Figure 4), and moulted during this time. Antarctic Prions had longer core-moult periods (71 and 88 days) than the other two species (43–53 days, Supplementary Table 1).
Stable isotope values of feathers differed among species (Tables 1–3 and Supplementary Figure 5), with the δ13C and δ15N values increasing from Blue Petrels to Thin-billed Prions to Antarctic Prions (Kruskal–Wallis tests; for δ13C: χ2 = 100.2, d.f. = 2, p < 0.001, post-hoc Dunn-tests: all p < 0.001, for δ15N: χ2 = 27.6, d.f. = 2, p < 0.001, post-hoc Dunn-tests: Blue Petrels vs. Thin-billed Prions p = 0.292, all other p < 0.001). Trophic positions based on the subset of feathers analysed for CSIA from Thin-billed Prions and Blue Petrels ranged from 3.0 to 4.3. A linear model detected no significant difference in trophic positions between the species (ANOVA tests; F1,26 = 1.04, p = 0.316, η2 = 0.045), whereas differences among the oceans were significant (F2,26 = 3.82, p = 0.035, η2 = 0.227), as were differences among feathers from AZ and SAZ distributions (F = 46.9, p < 0.001, η2 = 0.511; Supplementary Figure 6). Trophic positions were higher in the Pacific population, and birds with more northerly distributions (Figure 3 and Supplementary Figure 6).
Figure 3. Mercury and trophic position in feathers of Blue Petrels, Thin-billed Prions, and Antarctic Prions (BP, TBP, and AP, respectively). The mercury (A) and trophic position (B) values are shown for the seven populations, separately for habitat zones. Habitat was derived from δ13C following Cherel et al. (2018), as Subtropical Zone (STZ): δ13C > –18.3 ‰, Subantarctic Zone (SAZ): δ13C values of –21.2 to –18.3 ‰, and Antarctic Zone (AZ): δ13C < –21.2 ‰.
Across species, the trophic positions determined from feathers using linear models (TPLM), ranged from 3.2 to 3.9. Using this larger data set, we detected moderate differences in TPLM among species (F2,206 = 109.4, p < 0.001, η2 = 0.148) and oceans (F2,206 = 23.2, p < 0.001, η2 = 0.184), and strong differences among distributions (F2,206 = 263.0, p < 0.001, η2 = 0.574). Trophic positions were elevated and highly variable in the Pacific population, and birds with more northerly distributions (Figure 4).
Figure 4. Species-specific Generalized Additive Model (GAM) model fits for mercury values in feathers of Antarctic Prions, Blue Petrels, and Thin-billed Prions. Estimated smoothing curves for mercury values in feathers in relation to δ13C and trophic position (derived from δ15N and δ13C values, with 95% confidence intervals) are given where statistically significant. For GAM statistics, see Table 4.
Mercury concentrations in feathers differed among species (Kruskal–Wallis ANOVA: χ2 = 85.5, d.f. = 2, p < 0.001, post-hoc Dunn-tests: Blue Petrels vs. Antarctic Prions p = 0.265, all other p < 0.001). The highest mean Hg concentrations were in Blue Petrels (2.17 ± 1.94 μg/g), followed by Antarctic Prions (1.85 ± 0.75 μg/g), and Thin-billed Prions (1.14 ± 0.69 μg/g). Of the seven populations, Blue Petrels from Diego Ramírez (Pacific Ocean) had much higher Hg concentrations than predicted by their latitudinal distribution and trophic positions (Figure 4 and Supplementary Figures 7–9).
Generalized Additive Models (Figure 4 and Table 4) showed a significant effect of ocean basin in all three species, with the most elevated Hg values in the Pacific Ocean and the lowest in the Atlantic Ocean (Figure 5). In Blue Petrels and Thin-billed Prions, distribution (δ13C, habitat zone) as well as trophic position (δ15N, TPLM) influenced Hg values (Figure 4 and Table 4). Model selection retained only ocean basin for Antarctic Prions (Figure 6), but all parameters except habitat zone for Blue Petrels and Thin-billed Prions. Coefficients for the effect of trophic position (δ15N, TPLM) on feather Hg indicated a strong positive relationship for Blue Petrels, a weaker, negative relationship for Thin-billed Prions, and no influence for Antarctic Prions (Figure 6).
Table 4. Generalized Additive Model (GAM) results for feather mercury values, separately for the species, as a function of distribution (δ13C, habitat), trophic position (δ15N, TPLM), and ocean basin (Atlantic, Indian, or Pacific).
Figure 5. Mercury values in feathers of Antarctic Prions, Blue Petrels, and Thin-billed Prions from different ocean basins. Boxplots showing medians, interquartile ranges, and outliers.
Figure 6. Summary of GAM model selection (dredge function in the R package MuMIn) on the full models for feathers (A–C): gam(THg.feathers ∼ s(TP_est) + s(δ13C.feathers) + s(δ15N.feathers) + habitat + ocean), and for blood (D–F): gam(THg.blood ∼ s(δ13C.blood) + s(δ15N.blood) + season + habitat + ocean). The selected best models are outlined, and their AICs given on the right. Blue cells note the retained parameter, with coefficients reported in white letters.
Mean blood δ13C values were lowest in Blue Petrels (−24.4 ± 1.4 ‰), and higher in Thin-billed Prions (−21.4 ± 2.6 ‰) and Antarctic Prions (−22.5 ± 1.3 ‰, Kruskal–Wallis ANOVA: χ2 = 90.3, d.f. = 2, p < 0.001), with no significant difference between the last two species (post-hoc Dunn-tests: Thin-billed vs. Antarctic Prions p = 0.474, all other p < 0.001). Blood δ15N values differed among species, and were lowest in Antarctic Prions (8.3 ± 0.3 ‰), intermediate in Blue Petrels (9.0 ± 0.8 ‰), and highest in Thin-billed Prions (10.1 ± 1.9 ‰, Kruskal–Wallis ANOVA: χ2 = 38.5, d.f. = 2, p < 0.001, post-hoc Dunn-tests: all p < 0.001).
The trophic positions based on the subset of blood samples analysed for CSIA ranged from 3.3 to 4.0 in Thin-billed Prions (3.5 ± 0.1) and Blue Petrels (3.5 ± 0.2). According to TPCSIA values, the trophic positions of the two species did not differ significantly (t-test, t = −0.7, d.f. = 11.6, p = 0.480).
Mean Hg concentrations in blood differed among species (Kruskal–Wallis ANOVA: χ2 = 124.0, d.f. = 2, p < 0.001, post-hoc Dunn-tests: all p < 0.001), with the highest concentrations in Blue Petrels (2.99 ± 1.97 μg/g), then Thin-billed Prions (0.99 ± 0.41 μg/g), and Antarctic Prions (0.51 ± 0.22 μg/g).
Species-specific GAMs showed a significant effect of latitudinal distribution (δ13C, habitat zone) in all three species (Table 5 and Figures 7, 8). However, this was only clearly positive in Blue Petrels (Figures 7, 8 and Supplementary Figure 10). The trophic position (δ15N) influenced Hg values in Blue Petrels and Thin-billed Prions (Table 5), with a clear increase only in Blue Petrels (Figure 8). There was a significant effect of ocean basin for Antarctic and Thin-billed Prions (Table 5). Changes in Hg and stable isotope values over the season were apparent in blood of Blue Petrels and, to a lesser extent, of Thin-billed Prions (Table 5 and Figure 7). There was a decrease of an order of magnitude in Hg concentrations in blood of Blue Petrels, which were sampled from arrival in September to the post-moult visit to the colony in April (Figure 9).
Table 5. Generalized Additive Model (GAM) results for blood mercury values, separately for the species, as a function of distribution (δ13C, habitat), trophic position (δ15N), period (early = arrival to incubation vs. late = chick-rearing), and ocean basin (Atlantic, Indian, or Pacific).
Figure 7. Blood mercury concentrations in Blue Petrels, Thin-billed Prions, and Antarctic Prions, with effects of distribution (A) and the breeding season (B).
Figure 8. Species-specific Generalized Additive Model (GAM) model fits for mercury values in blood of Antarctic Prions, Blue Petrels, and Thin-billed Prions. Estimated smoothing curves for mercury values in blood in relation to δ13C and δ15N are given with 95% confidence intervals where statistically significant. For GAM statistics, see Table 5.
Figure 9. Temporal changes in mercury concentrations in blood of Blue Petrels from different breeding locations over the breeding season.
For blood Hg, all parameters except δ15N and habitat were retained in the best models for Antarctic Prions (Figure 6D). Habitat was also excluded for Blue Petrels (Figure 6E), and in three of four best models for Thin-billed Prions (Figure 6F). Coefficients for the effect of trophic position (δ15N) on the feather Hg indicated a strong positive relationship for Blue Petrels, but values close to zero for Thin-billed Prions and Antarctic Prions (Figure 6).
The year-round sea-ice concentration in areas used by tracked Blue Petrels, Thin-billed Prions and Antarctic Prions (Figure 10) from the Atlantic and Indian Ocean showed two annual peaks: in April for Blue Petrels and Thin-billed Prions from Atlantic colonies, and again in August–September for Blue Petrels. Blue Petrels from the Indian Ocean had higher sea-ice overlap than birds from the Atlantic in April to August (Figure 10). Blue Petrels from Diego Ramírez have not yet been tracked (but see distribution in Figure 1). The highest exposure to sea ice was in September for all populations except Thin-billed Prions from New Island (Falklands) (Figure 10).
Figure 10. Year-round percentage of sea-ice concentration (mean ± SD, and smooth lines with 95% confidence intervals) of geolocator tracked Blue Petrels, Thin-billed Prions, and Antarctic Prions.
During the period of wing moult (Supplementary Figure 1 and Supplementary Table 1), sea-ice exposure was low (<0.01) for all populations.
Matching data on blood Hg and sea-ice exposure were available for tracked individuals from four populations (Figure 11A), and on feather Hg and sea-ice exposure for five populations (Figure 11B). Model selection suggested that species differences were sufficient to explain differences in blood Hg values, but when analysing the dataset across species, a GAM suggested that blood Hg values increased with maximum sea-ice exposure (Figure 11A and Table 6). In contrast, feather Hg concentrations were not related to mean annual sea-ice exposure (Figure 11B and Table 6).
Figure 11. Mercury concentrations in relation to the year-round sea-ice exposure of tracked Blue Petrels, Thin-billed Prions, and Antarctic Prions, shown for blood samples (A) and body feathers (B). Grey shaded areas show GAM smoothed 95% confidence intervals, obtained in the R package “ggplot2”, across the whole dataset.
Table 6. Generalized Additive Model (GAM) results for tracked individuals, separately for blood and feathers, as a function of species and sea ice cover.
In the present study, we examined temporal and spatial effects on stable isotope values and Hg concentrations in seven populations of three species of small petrels in widely separated oceans. We found evidence that higher trophic level and the distribution may result in higher exposure to Hg. We also found a carry-over effect of Hg exposure between wintering and breeding grounds.
We found interspecific differences in Hg concentrations in both blood and feathers, with the highest value for both tissues in Blue Petrels. In the literature, differences among species in Hg concentrations are mostly discussed in relation to biomagnification processes and thus, trophic position (e.g., Becker et al., 2002; Anderson et al., 2009; Blévin et al., 2013; Gatt et al., 2020). However, we here compared three small-bodied petrel species of similar trophic positions, according to δ15N values in feathers and blood samples. We found that similar trophic position in different water masses did not lead to the same degree of Hg biomagnification. For example, Thin-billed Prions had the highest trophic positions relative to their distribution, but lower Hg concentrations than Blue Petrels. This result does not agree with the suggestion that the trophic position is the most important factor explaining variation in Hg concentrations in Southern Ocean seabirds (Becker et al., 2002). Likewise, in tunas trophic effects (i.e., geographical changes in foraging ecology) had a limited influence on the spatial variability of tissue Hg concentrations (Médieu et al., 2022).
Despite generally low trophic positions, dietary differences exist among the species, especially in the relative importance of fish. At South Georgia, crustaceans, and particularly Antarctic krill (Euphausia superba), predominated in Antarctic Prion and Blue Petrel diets, but fish was considerably more important for the Blue Petrels (Prince, 1980). In Blue Petrels at Marion Island (Steele and Klages, 1986), the diet consisted of 60% crustaceans, 21% myctophid fish and 16% squid by mass. In Blue Petrels at Kerguelen, however, the contribution of fish was higher (57%, Cherel et al., 2002b). Compared to King Penguins (Aptenodytes patagonicus) at Kerguelen which have a diet consisting of primarily (>90%) myctophids, Blue Petrels at the same island group have only slightly lower feather Hg values (Table 1, King Penguins = 2.2 ± 0.5 μg/g; Carravieri et al., 2013). In comparison, the proportion of fish taken by Thin-billed Prions and Antarctic Prions is very low both in Kerguelen (Cherel et al., 2002a) and the Falkland Islands (Quillfeldt et al., 2010a). The hyperiid amphipod Themisto gaudichaudii was consistently the dominant prey item for Thin-billed Prions. These predatory pelagic crustaceans may be responsible for the relatively high trophic position of Thin-billed Prions (Figure 3), but result in little Hg take-up. At Kerguelen, Hg concentrations were higher in myctophid fish (up to 0.424 μg/g dw) and, to a lesser extent, squid (up to 0.270 μg/g dw) compared to crustaceans (up to 0.034 in amphipods, 0.074 in copepods and 0.125 in euphasiids) (Cipro et al., 2018), and fish in the diet was suggested to be the most important driver of elevated Hg values in seabirds (Bocher et al., 2003).
While Blue Petrels are the most piscivorous of the species in the present study, they also use the most southerly habitats over the non-breeding season (Quillfeldt et al., 2015; Figure 2). Blue Petrels from Kerguelen spent the winter in waters with >10% sea-ice (Figure 10), and all Blue Petrels spent time in waters with 30–40% sea-ice before the start of the breeding season in August–September (Figure 10). Observations off west Antarctica suggested that Blue Petrels avoided areas with dense pack ice, but were found just outside the marginal ice zone, at sea surface temperatures of −0.7 to 0.9°C (Ryan et al., 2020). Mercury measurements along a transect from Hobart to the Antarctic (Cossa et al., 2011; see Figure 2) identified two zones of elevated dissolved Hg concentrations: in the Southern Zone where it is caused by processes in the ice-atmosphere-ocean interface like brine formation, and south of the Antarctic Polar Front (Cossa et al., 2011). In the Southern Zone, there is further a build-up of MeHg-enriched surface waters during winter months, when the sea-ice extent increases and the sea surface is protected from the UV and, thus, from MeHg photo-reduction (Cossa et al., 2011). However, the MeHg concentration was highest close to the Southern Antarctic Circumpolar Current Front, due to upwelling of waters from the minimum oxygen zone (Cossa et al., 2011; see Figure 2).
Some Antarctic seabirds have a strong affinity to the sea-ice environment, in particular Snow Petrels (Pagodroma nivea), Antarctic Petrels (Thalassoica antarctica), Adélie Penguins (Pygoscelis adeliae), and Emperor Penguins (Aptenodytes forsteri). As Procellariiformes (albatrosses, shearwaters, petrels, and storm-petrels) tend to have higher feather Hg concentrations than other species owing to their protracted moulting periods (Braune and Gaskin, 1987; Stewart et al., 1999), their Hg concentrations are particularly relevant here. However, a comparison with these species shows no particularly elevated Hg concentrations. In Snow Petrels from Adélie Land, the blood Hg concentration averaged 2.7 ± 1.1 (range: 1.0–5.3) μg/g dw in the pre-laying season (Tartu et al., 2014), lower than the values in our study for Blue Petrels in the early breeding season (Figure 9). Likewise, Antarctic Petrels had moderate mean Hg concentrations in feathers (2.41 ± 0.83 μg/g dw) and blood cells (1.38 ± 0.43 μg/g dw; Carravieri et al., 2021). Similarly, Adélie Penguins and Emperor Penguins from the Ross Sea had low feather Hg concentrations (0.592 ± 0.015 μg/g and 1.351 ± 0.058 μg/g, respectively; Pilcher et al., 2020). Therefore, the high values observed for Blue Petrels are unlikely to be explained directly by foraging in southern waters with up to 40% sea-ice concentration, but might have a connection with fish that migrate to the surface from the oxygen minimum layer, and with the elevated MeHg concentration close to the Southern Antarctic Circumpolar Current Front and, thus, in waters from the minimum oxygen zone (Cossa et al., 2011; see Figure 2). Further research should be dedicated to test this hypothesis.
Ivory Gulls (Pagophila eburnean) have the highest Hg concentrations in their eggs of any Arctic bird (Miljeteig et al., 2009; Bond et al., 2015). They consume ice-associated marine fish and scavenge on marine mammal carcasses. While the trophic position remained unchanged between 1877 and 2007 in ivory gulls from Arctic Canada and western Greenland (Bond et al., 2015), their feather Hg concentration increased by a factor of 45 (from 0.09 to 4.11 μg/g). Due to human activities such as coal and oil combustion, cement production, waste incineration, mining, smelting, and other industrial processes, the total and bioavailable amounts of Hg have dramatically increased in the environment since the industrial revolution (Pirrone et al., 2010; Arctic Monitoring and Assessment Programme [AMAP], 2019). The concentration of Hg that causes deleterious effects in birds depends on different factors, including diet composition, moult duration and the ability to demethylate Hg in the liver (Heinz et al., 2009), and has been given as 5–40 μg/g in feathers in general (Burger and Gochfeld, 1997), or 10–15 μg/g in piscivorous divers (Evers et al., 2014). All values observed here were below 10 μg/g, but the highest values in Blue Petrels approached this concentration (Figure 4), warranting further monitoring in the future.
We found temporal differences in Hg concentrations in blood samples, which were most pronounced in Blue Petrels. The highest concentrations were noted in September, after arrival from the wintering grounds, indicating that the adults arrived from Hg contaminated water masses or after feeding on prey with high Hg levels, but then switched to less contaminated prey. Hg in blood then decreased continually over the breeding season, and reached very low levels in birds sampled after returning to the colony post-moult. This was paralleled by a decline in trophic position, as indicated by δ15N values. Results of a previous study showed that mean δ15N values in adult Blue Petrels at Kerguelen decreased continuously throughout the annual cycle, from 9.5 ± 1.1 ‰ on arrival in the colony in September, to 7.3 ± 0.5 ‰ in the immediate post-breeding period in April to May (Cherel et al., 2014). In the present study, we measured a similar decrease from means of 10.3 ± 0.8 ‰ on arrival in the colony in September to 7.9 ± 0.4 ‰ at the post-nuptial stage in April (Table 1). The very low levels in April can be directly related to the Hg reset after Hg depuration in feathers, at a time when δ15N values are also very low (most likely indicating feeding on Antarctic krill).
Antarctic Prions did not show a pronounced seasonal trend in Hg concentrations, but had low values throughout the breeding season. In both Thin-billed Prion populations and all years, the blood Hg values were somewhat higher early in the breeding season. Diet composition of Thin-billed Prions at the Falkland Islands changes during the breeding season: from 60% squid and 35% amphipods during incubation to more equal proportions of amphipods, krill and squid during chick rearing (Quillfeldt et al., 2010a).
Mercury in blood represents two components: Hg incorporated from the diet during blood formation, and Hg stored in other tissues, such as the liver, kidneys and muscles, since the last feather moult. Residual Hg in other tissues is thought to equilibrate with levels in muscle (especially MeHg; Renedo et al., 2021) and liver, which act as the main storage organs for Hg between moults (Bearhop et al., 2000b). It has been shown that a carry-over of Hg can occur from remote places, such that high exposure in winter may lead to elevated blood Hg values until late in the summer (Lavoie et al., 2014). Especially for individuals with high winter exposure to Hg, slow changes in blood Hg over time were reported, suggesting a fast uptake rate and slow depuration (Lavoie et al., 2014). Carry-over of Hg among seasons would also explain the temporal patterns observed in Blue Petrels in our study.
In the Blue Petrels, the population of Diego Ramírez most likely moulted off west Antarctica, i.e., in the Pacific Ocean sector of the Southern Ocean, from 67 to 71°S and 78 to 119°W (Ryan et al., 2020). Ryan et al. (2020) observed large numbers of moulting Blue Petrels sitting on the water in dense flocks in mid-February, which is in line with the 10.7 ± 2.5 h per day spent sitting on the water by Blue Petrels from Kerguelen during moult (Cherel et al., 2016). Ryan et al. (2020) suggested that most of the birds observed in west Antarctica probably breed at Diego Ramírez, and this is also suggested by a comparison with distribution data of Blue Petrels from other colonies. Blue Petrels from both Kerguelen and South Georgia were found in the Atlantic sector of the Southern Ocean (20°W to 30°E) in March, during the core moulting period, and thus far away from the moulting aggregations observed off west Antarctica (Ryan et al., 2020).
Latitudinal differences in distribution influence Hg exposure, with lower Hg in Antarctic waters compared to subantarctic waters, a trend reported in previous studies (e.g., Carravieri et al., 2014b,2016, 2017, 2020; Cherel et al., 2018). We found no further increase towards subtropical waters. The trophic position also increased from polar to subantarctic waters, but continued to increase to subtropical waters. Thus, differences in trophic position would not fully explain the observed patterns. Indeed, a more detailed analysis revealed that not all populations show an increase in blood Hg concentrations associated with δ13C values. Differences in prey as well as carry-over effects of Hg may mask spatial differences.
Further spatial differences in Hg values were observed when comparing populations from different ocean sectors. Values were lowest in the Atlantic Ocean, intermediate in the Indian Ocean, and highest in the Pacific Ocean, although this was only based on one population. That population, Blue Petrels from Diego Ramírez, had high feather Hg concentrations (4.42 ± 2.72 μg/g dw), comparable with Blue Petrels on Marion Island, Indian Ocean (4.62 ± 4.11 μg/g dw, Supplementary Table 2). Tracking and dietary data are still lacking from both populations.
A difference in Hg has also been observed for other organisms such as Marbled Rockcod (Notothenia rossii), where mean muscle Hg concentrations of fish in waters around Kerguelen (0.255 μg/g dw; Bustamante et al., 2003) were three times higher than in the South Shetland Islands in the Atlantic sector of the Southern Ocean (0.077 μg/g dw; Cipro et al., 2017). Such differences may be due to differences in Hg sources and oceanographic features.
In line with previous studies, we found high Hg concentrations in Blue Petrels. As a novel result, we further found important population differences. We highlight that Blue Petrels did not have a northerly distribution or high trophic position, which usually account for elevated Hg concentrations in Southern Ocean seabirds. Instead, they have the most southern winter distribution of our three study species, and feed mainly on crustaceans, except on Kerguelen where myctophid fish constitute a substantial proportion of the diet. As other seabirds exposed to high Hg levels in winter, they have a notable temporal carry-over of high blood Hg values into the breeding season.
While the Kerguelen population of Blue Petrels has been tracked recently (e.g., Quillfeldt et al., 2015, 2020; Cherel et al., 2016), there are no diet or tracking data from the major population at Diego Ramírez. Our study suggests that this population has particularly high exposure to Hg (e.g., Figure 3 and Supplementary Figure 8), which can be an additional stressor and impact reproduction and survival in birds (Goutte et al., 2014; Mills et al., 2020). Further study of their movements and foraging ecology are therefore required, in particular to confirm if the high Hg concentrations in feathers are related to differences in diet or sea-ice exposure. Additionally, a comparison of Hg in flight feathers, and of spatial and temporal variation in Hg concentrations of their crustacean and fish prey in relation to biogeography and ecology would help reveal the factors driving differences among seabird species in terms of Hg exposure and contamination. A combination of ship-based and tracking studies could address the question of how the foraging and movement ecology of predators and spatial differences interact to produce the patterns in Hg burdens observed in these and other wildlife in the Southern Ocean.
The raw data supporting the conclusions of this article will be made available by the authors, without undue reservation.
The study involved wild individuals and was carried out under permits from the Falkland Islands Government (Environmental Planning: R21.2012), and the Animal Ethic Committee of the IPEV. All work conducted at Bird Island was approved by the Ethics Committee of the British Antarctic Survey and carried out under permit from the Government of South Georgia and the South Sandwich Islands. Seabird work in Diego Ramírez was approved by Res. N° 959 and N° 6093, Servicio Agrícola y Ganadero (Agriculture and Livestock Service), Chile.
PQ and PB conceived and designed the study. PQ, JM, JN, RP, and CS carried out the fieldwork. YC and PB carried out the lab work. PQ, JN, RP, KD, and YC carried out the data curation. PQ carried out the data analyses. PQ and PB drafted the manuscript. All authors reviewed the final draft of the manuscript.
This study was funded by the Deutsche Forschungsgemeinschaft (DFG) in the framework of the priority programme SPP1154 “Antarctic Research with comparative investigations in Arctic ice areas” (Grant No. Qu148/18). The fieldwork at Kerguelen was supported financially and logistically by the Institut Polaire Français Paul Emile Victor (IPEV, Programme N°109, C. Barbraud) and the Terres Australes et Antarctiques Françaises. We are grateful to the Contrat de Projet Etat-Région (CPER) and the Fonds Européen de Développement Régional (FEDER) for funding the Advanced Mercury Analyzer and the isotope-ratio mass spectrometers of LIENSs laboratory. The Institut Universitaire de France (IUF) is acknowledged for its support to PB as a Senior Member. This study represents a contribution to the Ecosystems component of the British Antarctic Survey Polar Science for Planet Earth Programme, funded by NERC. Logistical support in Bird Island was provided by the Collaborative Gearing Scheme of the Natural Environment Research Council Antarctic Funding Initiative (AFI-NERC).
The authors declare that the research was conducted in the absence of any commercial or financial relationships that could be construed as a potential conflict of interest.
All claims expressed in this article are solely those of the authors and do not necessarily represent those of their affiliated organizations, or those of the publisher, the editors and the reviewers. Any product that may be evaluated in this article, or claim that may be made by its manufacturer, is not guaranteed or endorsed by the publisher.
We thank the New Island Conservation Trust. Justine Thébault collected samples in 2018–19 and prepared samples for analysis. We are grateful to C. Churlaud and M. Brault-Favrou from the “Plateforme Analyses Elémentaires” of LIENSs for their assistance during mercury analysis and to G. Guillou from the “Plateforme analyses isotopiques” of LIENSs for running stable isotope analyses. We thank A. Corbeau, J. Ferrer-Obiol, M. Passerault, and T. Lacombe for fieldwork assistance in Kerguelen. We thank Jaime A. Cursach who assisted with sample collection and the III Naval Zone - Chilean Navy - for all their logistical and personnel support during our fieldwork in the Diego Ramírez Archipelago, Chile.
The Supplementary Material for this article can be found online at: https://www.frontiersin.org/articles/10.3389/fevo.2022.915199/full#supplementary-material
Albert, C., Renedo, M., Bustamante, P., and Fort, J. (2019). Using blood and feathers to investigate large-scale Hg contamination in Arctic seabirds: a review. Environ. Res. 177:108588. doi: 10.1016/j.envres.2019.108588
Anderson, O. R. J., Phillips, R. A., McDonald, R. A., Shore, R. F., McGill, R. A. R., and Bearhop, S. (2009). Influence of trophic position and foraging range on mercury levels within a seabird community. Mar. Ecol. Prog. Ser. 375, 277–288.
Arctic Monitoring and Assessment Programme [AMAP] (2019). Technical Background Report for the Global Mercury Assessment 2018. Oslo: Arctic Monitoring and Assessment Programme.
Bearhop, S., Phillips, R. A., Thompson, D. R., Waldron, S., and Furness, R. W. (2000a). Variability in mercury concentrations of great skuas Catharacta skua: the influence of colony, diet and trophic status inferred from stable isotope signatures. Mar. Ecol. Prog. Ser. 195, 261–268.
Bearhop, S., Ruxton, G. D., and Furness, R. W. (2000b). Dynamics of mercury in blood and feathers of great skuas. Environ. Toxicol. Chem. 19, 1638–1643.
Becker, P. H., González-Solís, J., Behrends, B., and Croxall, J. (2002). Feather mercury levels in seabirds at South Georgia: influence of trophic position, sex and age. Mar. Ecol. Prog. Ser. 243, 261–269.
Becker, P. H., Goutner, V., Ryan, P. G., and González-Solís, J. (2016). Feather mercury concentrations in Southern Ocean seabirds: variation by species, site and time. Environ. Pollut. 216, 253–263. doi: 10.1016/j.envpol.2016.05.061
Bierman, W. H., and Voous, K. H. (1950). Birds observed and collected during the whaling expeditions of the ‘Willem Barendsz’ in the Antarctic, 1946–1947 and 1947–1948. Ardea 37, 1–121.
Blévin, P., Carravieri, A., Jaeger, A., Chastel, O., Bustamante, P., and Cherel, Y. (2013). Wide range of mercury contamination in chicks of Southern Ocean seabirds. PLoS One 8:e54508. doi: 10.1371/journal.pone.0054508
Bocher, P., Caurant, F., Cherel, Y., Miramand, P., and Bustamante, P. (2003). Influence of the diet on the bioaccumulation of heavy metals in zooplankton-eating petrels at Kerguelen archipelago, Southern Indian Ocean. Polar Biol. 26, 759–767.
Bond, A. L., Hobson, K. A., and Branfireun, B. A. (2015). Rapidly increasing methyl mercury in endangered ivory gull (Pagophila eburnea) feathers over a 130 year record. Proc. R. Soc. B 282:20150032. doi: 10.1098/rspb.2015.0032
Braune, B. M., and Gaskin, D. E. (1987). Mercury levels in Bonaparte’s gulls (Larus philadelphia) during autumn molt in the Quoddy region, New Brunswick, Canada. Arch. Environ. Contam. Toxicol. 16, 539–549.
Brooks, S., Lindberg, S., Southworth, G., and Arimoto, R. (2008). Springtime atmospheric mercury speciation in the McMurdo, Antarctica coastal region. Atmos. Environ. 42, 2885–2893.
Brown, R. S., Norman, F. I., and Eades, D. W. (1986). Notes on Blue and Kerguelen petrels found beach-washed in Victoria, 1984. Emu 86, 228–238.
Burger, J., and Gochfeld, M. (1997). Risk, mercury levels, and birds: relating adverse laboratory effects to field biomonitoring. Environ. Res. 75, 160–172. doi: 10.1006/enrs.1997.3778
Bustamante, P., Bocher, P., Cherel, Y., Miramand, P., and Caurant, F. (2003). Distribution of trace elements in the tissues of benthic and pelagic fish from the Kerguelen Islands. Sci. Total Environ. 313, 25–39. doi: 10.1016/S0048-9697(03)00265-1
Bustamante, P., Lahaye, V., Durnez, C., Churlaud, C., and Caurant, F. (2006). Total and organic Hg concentrations in cephalopods from the North East Atlantic waters: influence of geographical origin and feeding ecology. Sci. Total Environ. 368, 585–596.
Carravieri, A., Bustamante, P., Churlaud, C., and Cherel, Y. (2013). Penguins as bioindicators of mercury contamination in the Southern Ocean: birds from the Kerguelen Islands as a case study. Sci. Total Environ. 454, 141–148. doi: 10.1016/j.scitotenv.2013.02.060
Carravieri, A., Bustamante, P., Labadie, P., Budzinski, H., Chastel, O., and Cherel, Y. (2020). Trace elements and persistent organic pollutants in chicks of 13 seabird species from Antarctica to the subtropics. Environ. Int. 134:105225. doi: 10.1016/j.envint.2019.105225
Carravieri, A., Cherel, Y., Blévin, P., Brault-Favrou, M., Chastel, O., and Bustamante, P. (2014a). Mercury exposure in a large subantarctic avian community. Environ. Pollut. 190, 51–57. doi: 10.1016/j.envpol.2014.03.017
Carravieri, A., Bustamante, P., Tartu, S., Meillère, A., Labadie, P., Budzinski, H., et al. (2014b). Wandering albatrosses document latitudinal variations in the transfer of persistent organic pollutants and mercury to southern ocean predators. Environ. Sci. Technol. 48, 14746–14755. doi: 10.1021/es504601m
Carravieri, A., Cherel, Y., Brault-Favrou, M., Churlaud, C., Peluhet, L., Labadie, P., et al. (2017). From Antarctica to the subtropics: contrasted geographical concentrations of selenium, mercury, and persistent organic pollutants in skua chicks (Catharacta spp.). Environ. Pollut. 228, 464–473. doi: 10.1016/j.envpol.2017.05.053
Carravieri, A., Cherel, Y., Jaeger, A., Churlaud, C., and Bustamante, P. (2016). Penguins as bioindicators of mercury contamination in the southern Indian Ocean: geographical and temporal trends. Environ. Pollut. 213, 195–205. doi: 10.1016/j.envpol.2016.02.010
Carravieri, A., Warner, N. A., Herzke, D., Brault-Favrou, M., Tarroux, A., Fort, J., et al. (2021). Trophic and fitness correlates of mercury and organochlorine compound residues in egg-laying Antarctic petrels. Environ. Res. 193:110518. doi: 10.1016/j.envres.2020.110518
Chastel, O., and Bried, J. (1996). Diving ability of Blue Petrels and Thin-billed Prions. Condor 98, 627–629. doi: 10.1242/jeb.00286
Cherel, Y., Barbraud, C., Lahournat, M., Jaeger, A., Jaquemet, S., Wanless, R. M., et al. (2018). Accumulate or eliminate? Seasonal mercury dynamics in albatrosses, the most contaminated family of birds. Environ. Pollut. 241, 124–135. doi: 10.1016/j.envpol.2018.05.048
Cherel, Y., Bocher, P., Trouvé, C., and Weimerskirch, H. (2002b). Diet and feeding ecology of Blue Petrels Halobaena caerulea at Iles Kerguelen, Southern Indian Ocean. Mar. Ecol. Prog. Ser. 228, 283–299.
Cherel, Y., Bocher, P., de Broyer, C., and Hobson, K. A. (2002a). Food and feeding ecology of the sympatric Thin-billed Pachyptila belcheri and Antarctic P. desolata Prions at Iles Kerguelen, Southern Indian Ocean. Mar. Ecol. Prog. Ser. 228, 263–281.
Cherel, Y., Connan, M., Jaeger, A., and Richard, P. (2014). Seabird year-round and historical feeding ecology: blood and feather δ13C and δ15N values document foraging plasticity of small sympatric petrels. Mar. Ecol. Prog. Ser. 505, 267–280.
Cherel, Y., Fontaine, C., Richard, P., and Labat, J. P. (2010). Isotopic niches and trophic levels of myctophid fishes and their predators in the Southern Ocean. Limnol. Oceanogr. 55, 324–332.
Cherel, Y., Quillfeldt, P., Delord, K., and Weimerskirch, H. (2016). Combination of at-sea activity, geolocation and feather stable isotopes documents where and when seabirds molt. Front. Ecol. Evol. 4:3. doi: 10.3389/fevo.2016.00003
Cipro, C. V. Z., Cherel, Y., Bocher, P., Caurant, F., Miramand, P., and Bustamante, P. (2018). Trace elements in invertebrates and fish communities off the Kerguelen Islands. Polar Biol. 41, 175–191.
Cipro, C. V. Z., Montone, R. C., and Bustamante, P. (2017). Mercury in the ecosystem of Admiralty Bay, King George Island, Antarctica: occurrence and trophic distribution. Mar. Pollut. Bull. 114, 564–570. doi: 10.1016/j.marpolbul.2016.09.024
Cossa, D., Heimbürger, L. E., Lannuzel, D., Rintoul, S. R., Butler, E. C., Bowie, A. R., et al. (2011). Mercury in the southern ocean. Geochim. Cosmochim. Acta 75, 4037–4052.
Dilley, B. J., Davies, D., Schramm, M., Connan, M., and Ryan, P. G. (2017). The distribution and abundance of Blue Petrels (Halobaena caerulea) breeding at subantarctic Marion Island. Emu 117, 222–232.
Evers, D. C., Schmutz, J. A., Basu, N., DeSorbo, C. R., Fair, J., Gray, C. E., et al. (2014). Historic and contemporary mercury exposure and potential risk to yellow-billed loons (Gavia adamsii) breeding in Alaska and Canada. Waterbirds 37, 147–159.
Fitzgerald, W. F., Engstrom, D. R., Mason, R. P., and Nater, E. A. (1998). The case for atmospheric mercury contamination in remote areas. Environ. Sci. Technol. 32, 1–7. doi: 10.1007/s10661-005-9180-7
Gatt, M. C., Reis, B., Granadeiro, J. P., Pereira, E., and Catry, P. (2020). Generalist seabirds as biomonitors of ocean mercury: the importance of accurate trophic position assignment. Sci. Total Environ. 740:140159. doi: 10.1016/j.scitotenv.2020.140159
Gionfriddo, C. M., Tate, M. T., Wick, R. R., Schultz, M. B., Zemla, A., Thelen, M. P., et al. (2016). Microbial mercury methylation in Antarctic sea ice. Nat. Microbiol. 1:16127. doi: 10.1038/nmicrobiol.2016.127
Goutte, A., Bustamante, P., Barbraud, C., Delord, K., Weimeskirch, H., and Chastel, O. (2014). Demographic responses to mercury exposure in two closely-related Antarctic top predators. Ecology 95, 1075–1086. doi: 10.1890/13-1229.1
Hebert, C. E., Popp, B. N., Fernie, K. J., Ka’apu-Lyons, C., Rattner, B. A., and Wallsgrove, N. (2016). Amino acid specific stable nitrogen isotope values in avian tissues: insights from captive American kestrels and wild herring gulls. Environ. Sci. Technol. 50, 12928–12937. doi: 10.1021/acs.est.6b04407
Heinz, G. H., Hoffman, D. J., Klimstra, J. D., Stebbins, K. R., Kondrad, S. L., and Erwin, C. A. (2009). Species differences in the sensitivity of avian embryos to methylmercury. Arch. Environ. Contam. Toxicol. 56, 129–138. doi: 10.1007/s00244-008-9160-3
Hobson, K. A., and Clark, R. G. (1993). Turnover of 13C cellular and plasma reactions of blood: implications for non-destructive sampling in avian dietary studies. Auk 110, 638–641.
Jaeger, A., Lecomte, V. J., Weimerskirch, H., Richard, P., and Cherel, Y. (2010). Seabird satellite tracking validates the use of latitudinal isoscapes to depict predators’foraging areas in the Southern Ocean. Rapid Commun. Mass Spectrom. 24, 3456–3460. doi: 10.1002/rcm.4792
Kleinschmidt, B., Burger, C., Dorsch, M., Nehls, G., Heinänen, S., Morkûnas, J., et al. (2019). The diet of red-throated divers (Gavia stellata) overwintering in the German Bight (North Sea) analysed using molecular diagnostics. Mar. Biol. 166:77.
Lavoie, R. A., Baird, C. J., King, L. E., Kyser, T. K., Friesen, V. L., and Campbell, L. M. (2014). Contamination of mercury during the wintering period influences concentrations at breeding sites in two migratory piscivorous birds. Environ. Sci. Technol. 48, 13694–13702. doi: 10.1021/es502746z
Lawton, K., Robertson, G., Kirkwood, R., Valencia, J., Schlatter, R., and Smith, D. (2006). An estimate of population sizes of burrowing seabirds at the Diego Ramirez archipelago, Chile, using distance sampling and burrow-scoping. Polar Biol. 29, 229–238.
Lorrain, A., Graham, B., Ménard, F., Popp, B., Bouillon, S., van Breugel, P., et al. (2009). Nitrogen and carbon isotope values of individual amino acids: a tool to study foraging ecology of penguins in the Southern Ocean. Mar. Ecol. Prog. Ser. 391, 293–306.
Manceau, A., Gaillot, A. C., Glatzel, P., Cherel, Y., and Bustamante, P. (2021). In vivo formation of HgSe nanoparticles and Hg-tetraselenolate complex from methylmercury in seabird – Implications for the Hg-Se antagonism. Environ. Sci. Technol. 55, 1515–1526. doi: 10.1021/acs.est.0c06269
Mattern, T., Masello, J. F., Ellenberg, U., and Quillfeldt, P. (2015). Actave.net - a web-based tool for the analysis of seabird activity patterns from saltwater immersion geolocators. Methods Ecol. Evol. 6, 859–864.
Médieu, A., Point, D., Itai, T., Angot, H., Buchanan, P. J., Allain, V., et al. (2022). Evidence that Pacific tuna mercury levels are driven by marine methylmercury production and anthropogenic inputs. Proc. Nat. Acad. Sci. U.S.A. 119:e2113032119. doi: 10.1073/pnas.2113032119
Miljeteig, C., Strøm, H., Gavrilo, M. V., Volkov, A., Jennsen, B. M., and Gabrielsen, G. W. (2009). High levels of contaminants in ivory gull Pagophila eburnean eggs from the Russian and Norwegian Arctic. Environ. Sci. Technol. 43, 5521–5528. doi: 10.1021/es900490n
Mills, W. F., Bustamante, P., McGill, R. A. R., Anderson, O. R. J., Bearhop, S., Cherel, Y., et al. (2020). Mercury exposure in an endangered seabird: long-term changes and relationships with trophic ecology and breeding success. Proc. R. Soc. B 287:20202683. doi: 10.1098/rspb.2020.2683
Monteiro, L. R., and Furness, R. W. (2001). Kinetics, Dose-Response, and excretion of methylmercury in free-living adult Cory’s shearwaters. Environ. Sci. Technol. 35, 739–746. doi: 10.1021/es000114a
Navarro, J., Cardador, L., Brown, R., and Phillips, R. A. (2015). Spatial distribution and ecological niches of non-breeding planktivorous petrels. Sci. Rep. 5:12164.
Navarro, J., Votier, S. C., Aguzzi, J., Chiesa, J. J., Forero, M. G., and Phillips, R. A. (2013). Ecological segregation in space, time and trophic niche of sympatric planktivorous petrels. PLoS One 8:e62897. doi: 10.1371/journal.pone.0062897
Nerentorp Mastromonaco, M. G., Gårdfeldt, K., Langer, S., and Dommergue, A. (2016). Seasonal study of mercury species in the Antarctic sea ice environment. Environ. Sci. Technol. 50, 12705–12712. doi: 10.1021/acs.est.6b02700
Phillips, R. A., Bearhop, S., McGill, R. A. R., and Dawson, D. A. (2009). Stable isotopes reveal individual variation in migration strategies and habitat preferences in a suite of seabirds during the nonbreeding period. Oecologia 160, 795–806. doi: 10.1007/s00442-009-1342-9
Pilcher, N., Gaw, S., Eisert, R., Horton, T. W., Gormley, A. M., Cole, T. L., et al. (2020). Latitudinal, sex and inter-specific differences in mercury and other trace metal concentrations in Adélie and Emperor penguins in the Ross Sea, Antarctica. Mar. Pollut. Bull. 154:111047. doi: 10.1016/j.marpolbul.2020.111047
Pirrone, N., Cinnirella, S., Feng, X., Finkelman, R. B., Friedli, H. R., Leaner, J., et al. (2010). Global mercury emissions to the atmosphere from anthropogenic and natural sources. Atmos. Chem. Phys. 10, 5951–5964.
Prince, P. A. (1980). The food and feeding ecology of Blue petrel (Halobaena caerulea) and dove Prion (Pachyptila desolata). J. Zool. 190, 59–76.
Quillfeldt, P., and Masello, J. F. (2020). Compound-specific stable isotope analyses in Falkland Islands seabirds reveal seasonal changes in trophic positions. BMC Ecol. 20:21. doi: 10.1186/s12898-020-00288-5
Quillfeldt, P., Cherel, Y., Delord, K., and Weimerkirch, H. (2015). Cool, cold or colder? Spatial segregation of Prions and Blue Petrels is explained by differences in preferred sea surface temperatures. Biol. Lett. 11:20141090. doi: 10.1098/rsbl.2014.1090
Quillfeldt, P., Masello, J. F., McGill, R. A., Adams, M., and Furness, R. W. (2010b). Moving polewards in winter: a recent change in the migratory strategy of a pelagic seabird? Front. Zool. 7:15. doi: 10.1186/1742-9994-7-15
Quillfeldt, P., Michalik, A., Veit-Köhler, G., Strange, I. J., and Masello, J. F. (2010a). Inter-annual changes in diet and foraging trip lengths in a small pelagic seabird, the Thin-billed Prion Pachyptila belcheri. Mar. Biol. 157, 2043–2050.
Quillfeldt, P., Masello, J. F., Navarro, J., and Phillips, R. A. (2013). Year-round distribution suggests spatial segregation of two small petrel species in the South Atlantic. J. Biogeogr. 40, 430–441.
Quillfeldt, P., Weimerskirch, H., Delord, K., and Cherel, Y. (2020). Niche switching and leapfrog foraging: movement ecology of sympatric petrels during the early breeding season. Mov. Ecol. 8, 1–14. doi: 10.1186/s40462-020-00212-y
Renedo, M., Bustamante, P., Tessier, E., Pedrero, Z., Cherel, Y., and Amouroux, D. (2017). Assessment of mercury speciation in feathers using species-specific isotope dilution analysis. Talanta 174, 100–110. doi: 10.1016/j.talanta.2017.05.081
Renedo, M., Pedrero, Z., Amouroux, D., Cherel, Y., and Bustamante, P. (2021). Mercury isotopes of key tissues document mercury metabolic processes in seabirds. Chemosphere 263:127777. doi: 10.1016/j.chemosphere.2020.127777
Ryan, P. G., Lee, J. R., and Le Bouard, F. (2020). Moult intensity in Blue Petrels and a key moult site off West Antarctica. Antarct. Sci. 32, 1–9.
Scheuhammer, A. M., Meyer, M. W., Sandheinrich, M. B., and Murray, M. W. (2007). Effects of environmental methylmercury on the health of wild birds, mammals, and fish. AMBIO 36, 12–19. doi: 10.1579/0044-7447(2007)36[12:eoemot]2.0.co;2
Schlatter, R. P., and Riveros, G. M. (1997). Historia natural del Archipiélago Diego Ramírez, Chile. Serie Científica INACH 47, 87–112
Seco, J., Aparício, S., Brierley, A. S., Bustamante, P., Coelho, J. P., Philips, R., et al. (2021). Mercury biomagnification in a Southern Ocean food web. Environ. Pollut. 275:116620. doi: 10.1016/j.envpol.2021.116620
Seco, J., Xavier, J. C., Bustamante, P., Coelho, J. P., Saunders, R. A., Ferreira, N., et al. (2020). Main drivers of mercury levels in Southern Ocean Lantern fish Myctophidae. Environ. Pollut. 264:114711. doi: 10.1016/j.envpol.2020.114711
Steele, W. K., and Klages, N. T. (1986). Diet of the Blue petrel at sub-Antarctic Marion Island. Afr. Zool. 21, 253–256.
Stewart, F. M., Phillips, R. A., Bartle, J. A., Craig, J., and Shooter, D. (1999). Influence of phylogeny, diet, moult schedule and sex on heavy metal concentrations in New Zealand Procellariiformes. Mar. Ecol. Prog. Ser. 178, 295–305.
Tan, S. W., Meiller, J. C., and Mahaffey, K. R. (2009). The endocrine effects of mercury in humans and wildlife. Crit. Rev. Toxicol. 39, 228–269. doi: 10.1080/10408440802233259
Tartu, S., Bustamante, P., Goutte, A., Cherel, Y., Weimerskirch, H., and Bustnes, J. O. (2014). Age-Related Mercury Contamination and Relationship with Luteinizing Hormone in a Long-Lived Antarctic Bird. PLoS One 9:e103642. doi: 10.1371/journal.pone.0103642
Thébault, J., Bustamante, P., Massaro, M., Taylor, G., and Quillfeldt, P. (2021). Influence of species-specific feeding ecology on mercury concentrations in seabirds breeding on the Chatham Islands, New Zealand. Environ. Toxicol. Chem. 40, 454–472. doi: 10.1002/etc.4933
Thompson, D. R., and Furness, R. W. (1989). The chemical form of mercury stored in South Atlantic seabirds. Environ. Pollut. 60, 305–317. doi: 10.1016/0269-7491(89)90111-5
Thompson, D. R., Bearhop, S., Speakman, J. R., and Furness, R. W. (1998). Feathers as a means of monitoring mercury in seabirds: insights from stable isotope analysis. Environ. Pollut. 101, 193–200. doi: 10.1016/s0269-7491(98)00078-5
Van den Steen, E., Poisbleau, M., Demongin, L., Covaci, A., Dirtu, A. C., Pinxten, R., et al. (2011). Organohalogenated contaminants in eggs of rockhopper penguins (Eudyptes chrysocome) and imperial shags (Phalacrocorax atriceps) from the Falkland Islands. Sci. Total Environ. 409, 2838–2844. doi: 10.1016/j.scitotenv.2011.04.002
Weimerskirch, H., Zotier, R., and Jouventin, P. (1989). The avifauna of the Kerguelen Islands. Emu 89, 15–29.
Keywords: distribution, mercury, petrels, stable isotopes, trophic position
Citation: Quillfeldt P, Cherel Y, Navarro J, Phillips RA, Masello JF, Suazo CG, Delord K and Bustamante P (2022) Variation Among Species and Populations, and Carry-Over Effects of Winter Exposure on Mercury Accumulation in Small Petrels. Front. Ecol. Evol. 10:915199. doi: 10.3389/fevo.2022.915199
Received: 07 April 2022; Accepted: 06 June 2022;
Published: 30 June 2022.
Edited by:
Jason Newton, University of Glasgow, United KingdomReviewed by:
Nathan Wolf, Alaska Pacific University, United StatesCopyright © 2022 Quillfeldt, Cherel, Navarro, Phillips, Masello, Suazo, Delord and Bustamante. This is an open-access article distributed under the terms of the Creative Commons Attribution License (CC BY). The use, distribution or reproduction in other forums is permitted, provided the original author(s) and the copyright owner(s) are credited and that the original publication in this journal is cited, in accordance with accepted academic practice. No use, distribution or reproduction is permitted which does not comply with these terms.
*Correspondence: Petra Quillfeldt, cGV0cmEucXVpbGxmZWxkdEBiaW8udW5pLWdpZXNzZW4uZGU=
Disclaimer: All claims expressed in this article are solely those of the authors and do not necessarily represent those of their affiliated organizations, or those of the publisher, the editors and the reviewers. Any product that may be evaluated in this article or claim that may be made by its manufacturer is not guaranteed or endorsed by the publisher.
Research integrity at Frontiers
Learn more about the work of our research integrity team to safeguard the quality of each article we publish.