- Department of Entomology and Nematology, Fort Lauderdale Research and Education Center, Institute of Food and Agricultural Sciences, University of Florida, Fort Lauderdale, FL, United States
A eusocial insect colony represents a complex biological entity that must ensure degrees of perennity once it reaches maturity (production of dispersing imagoes over many successive years) to optimize its reproductive success. It is known that a subterranean termite colony invests differentially in different castes over time and adjusts colony functions depending on colony internal and external conditions over many years of activity. However, the current study demonstrates that Coptotermes formosanus Shiraki field mature colonies go through dramatic demographic changes and breeding structure shifts, even many years after they have reached reproductive success. By analyzing the changes in age demography of C. formosanus colonies from four field sites, we here provide a new perspective on how a colony may function over decades, which reveals that each colony demographic trajectory is unique. In a way, throughout its life, a termite colony displays its own “demographic individuality” that drives its growth, its foraging ability, its competitiveness, its age demography, its senescence and ultimately its death. This study is therefore a narrated story of the life -and death- of different C. formosanus field colonies over decades of observation.
Introduction
Animal societies have the ability to form complex groups of cooperating individuals, where a distinct degree of organization was reached in eusocial taxa (often referred to as “superorganisms”) (Wheeler, 1911; Emerson, 1939; Seeley, 1989; Hölldobler and Wilson, 2009; Boomsma and Gawne, 2018). Eusociality involves a reproductive division of labor within the social context of overlapping generations and cooperative brood care (Wilson, 1971). This major transition has evolved multiple times with remarkable degrees of evolutionary convergence among independent taxa (Mueller et al., 2005; Howard and Thorne, 2010; Harrison et al., 2018). Eusocial insects are important ecological drivers, with ants and termites combined representing more than half of the terrestrial arthropod biomass (Eggleton, 2020), while displaying remarkable degrees of biodiversity and phenotypic innovations (Hölldobler and Wilson, 2009; Chouvenc et al., 2021).
In the life cycle of superorganisms, eusocial insects such as ants and termite colonies have decoupled the default linear reproductive process of organisms (birth → juvenile development → adult maturation → reproduction), where the production of sterile individual helpers during colony growth is analogous to the development of the soma line, while the emergence of reproductively competent imagoes is limited to matured colonies as an analogy to the germ line (Oster and Wilson, 1978; Boomsma and Gawne, 2018; Ramsay et al., 2021). The establishment of the reproductive division of labor is therefore tied to two distinct types of reproductive investments playing at two different degrees of organization and at different times within the colony life cycle, especially for taxa relying on independent colony foundation strategies (Cronin et al., 2013). First, is the internal reproductive cycle of individuals in the colony aiming at producing sterile helper castes that contribute to the growth and defense of the colony. Second, is the external reproductive cycle of the colony aiming at producing reproductively competent alates that disperse and spread their genetic material to the next generation outside of the colony (Emerson, 1939; Moritz and Southwick, 2012; Shik et al., 2012; Boomsma and Gawne, 2018). Thus, independently from the life cycle of each individual within a colony, eusocial insect colonies must go through their own life cycle from birth ( = colony foundation) → juvenile development ( = colony ergonomic growth) → adult maturation ( = production of dispersing reproductive caste) → to sexual reproduction ( = dispersal flight events) (Nutting, 1969; Oster and Wilson, 1978; Porter and Tschinkel, 1985; Chouvenc and Su, 2014; Nalepa, 2015).
The theoretical framework of the ergonomic optimization of eusocial insect colony growth and their reproductive perennity provided a comprehensive background to explain the differential resource investment into castes throughout the life cycle of a social insect colony (Oster and Wilson, 1978; Houston et al., 1988). The demography of a social insect colony may change upon its variable intrinsic metabolic needs and extrinsic environmental fluctuations (Porter and Tschinkel, 1986; Kaspari and Vargo, 1995; Hou et al., 2010; Cook et al., 2011; Chouvenc and Su, 2014; Fewell and Harrison, 2016). In addition, many eusocial taxa can display diverse and plastic breeding strategies (Bourke and Ratnieks, 1999; Heinze and Keller, 2000; Vargo et al., 2003; Vargo, 2019; Eyer and Vargo, 2021). Such characteristics explain why Oster and Wilson (1978) considered eusocial insect colonies as “highly coordinated growth machines” that are optimized through selective pressures to maximize colony fitness outcomes.
Notably, the study of social insect colony demography has primarily been driven by the determination of colony size (i.e., the number of individuals in the colony), as it often defines the colony ability to acquire resources and thrive in a competitive environment (Michener, 1964; Su and Scheffrahn, 1988a; Haagsma and Rust, 1995; Tschinkel et al., 1995; Naug, 2009). Reciprocally, the optimization of resource acquisition and utilization through colony ergonomic growth and colony reproductive output influences colony size in a feedback loop (Oster and Wilson, 1978; Dornhaus et al., 2012). Therefore, acquiring demographic data about social insect colonies can provide a unique insight about its reproductive status, its breeding system, its ecological impact, its competitive ability, and other aspects that would inform how a eusocial insect colony functions as a superorganism (Oster and Wilson, 1978; Tschinkel, 1991; Schmid-Hempel, 1992; Vargo and Husseneder, 2009). However, the determination of colony size and other demographic variables from field populations is inherently challenging often because of its destructive nature and the resulting inability to follow demographic changes over time (Darlington, 1984; Tschinkel, 1998), which often results in the reliance on theoretical models to explain long term ecological patterns (Oster and Wilson, 1978; Shik et al., 2012; Su, 2013).
In subterranean termites (Rhinotermitidae), colony access is limited owing to their cryptic lifestyle and their widely spread underground foraging territory (King and Spink, 1969), which have presented additional challenges for identifying general patterns about colony demography and ecology. Such limitation has resulted in a reliance on mark-recapture protocols, with limited predictive power from the inference process (Su and Scheffrahn, 1988a; Grace, 1990; Thorne et al., 1996; Evans et al., 1998; Su and Scherer, 2003; Arab et al., 2005; Su, 2013). The quest for subterranean termite colony size estimate initially aimed at establishing termite control protocols (Su et al., 1991; Su and Scheffrahn, 1998; Su, 2001, 2019), which left aside a rather important aspect of termite colony functionality: their inherent age cohorts, their caste demographic composition, and their reproductive status over time. In theory, such colony demography must be highly variable throughout the life of the colony (Oster and Wilson, 1978). Unfortunately, to our knowledge, no study was able to gather data with comprehensive information about termite colony age demographic changes over time, except for a limited observation from Grace et al. (1995).
Termite colony age demography is a direct result of two opposite factors: (1) colony natality rate, which relies on queen(s) oviposition and quality of brood care, and (2) colony mortality rate, which depends on environmental threats on foraging individuals and the inevitable individual senescence (Nakajima et al., 1963; Šobotník et al., 2012; Chouvenc and Su, 2014; Du et al., 2017). Similar to human demographic studies of countries that use age pyramids to determine how natality and mortality shape the age structure of a population, which impact economic trends (Goldstein, 2009), an analogous investigation of individual age composition in termite colonies can directly reflect on the statuses of their general demography, health, reproductivity and resource management efficiency. To our knowledge, such study has not been done because of the need to follow the population age structure over the lifetime of a colony in the field. Therefore, making any interpretation about the demography of large perennial eusocial insect colonies remains primarily speculative or theoretical (Oster and Wilson, 1978; Su, 2013).
Arguably, the seminal work of Oster and Wilson (1978) established the foundation for understanding the fundamental shifts required in colony demography throughout the life cycle of the colony (foundation, ergonomic growth, reproduction) for fitness maximization, but they omitted to discuss the phase that eventually all social insect colonies have to go through: colony senescence that involves the loss of critical colony functions, which ultimately leads to colony death. Paradoxically, individual senescence (or lack thereof) in social insects has been extensively investigated in the context of the superorganism (Rueppell et al., 2007; Kramer and Schaible, 2013; Giraldo and Traniello, 2014; de Verge and Nehring, 2016; Kramer et al., 2021), where sterile individuals are disposable and often not as long-lived as reproductive castes (Keller and Genoud, 1997; Lemanski and Fefferman, 2018; Giraldo et al., 2021). However, the longevity of individuals directly impacts their ability to maintain colony functions (resource acquisition, colony maintenance, brood care) as a high return on investment for the colony (Moret and Schmid-Hempel, 2009; Dornhaus et al., 2012; Chouvenc et al., 2015a; Lemanski and Fefferman, 2018; Bernadou et al., 2021). Conversely, beyond honey bee colony collapse research (Ellis et al., 2010; Dainat et al., 2012; Evans and Chen, 2021), colony senescence is a poorly studied aspect of the life of eusocial insects, especially in termites (Lepage and Darlington, 2000; Chouvenc et al., 2013a).
Often, observations of “natural” termite colony death in the field may arise from accidental events such as flooding, droughts, predation, or inherent colony senescence (Collins, 1981; Lepage and Darlington, 2000). It has been suggested that colony senescence could be initiated by a reduction or loss of reproduction, which leads to an absence of brood ultimately resulting in the accumulation of old individuals within the colony (Bodot, 1969; Darlington and Dransfield, 1987; Darlington, 1991; Grace et al., 1995; Chouvenc and Su, 2014). Studies on the use of chitin synthesis inhibitor (CSI) baits against subterranean termite colonies implied that the rapid death of the brood and the accumulation of old workers was analogous to an accelerated senescence of the colony, combined with a progressive starvation of all dependent castes (Chouvenc, 2018, 2020; Kakkar et al., 2018; Chouvenc and Lee, 2021). This recent research has provided clues on what the end of colony life may look like from a demographic perspective. However, beyond such limited observations, information on colony demographic changes over time and colony senescence in subterranean termites in the field remains fragmentary.
Finally, the colony life cycle and the inherent molting processes of subterranean termites add another layer of complexity when it comes to how to interpret demographic changes over time (Grace et al., 1995; Chouvenc and Su, 2014). Subterranean termites molt periodically until they die from a series of factors (age, injury, predation, disease, competition, resource limitation). Each time a worker molts, it grows slightly bigger which means that when comparing two groups of termites, the relative weight of individuals can be used as a proxy for the determination of the relative age of the two groups (Nakajima et al., 1963; Chouvenc and Su, 2014; Su et al., 2017). Similarly, high reproduction would lead to an influx of young individuals, while low reproduction would lead to an accumulation of old individuals over time. Therefore, if one could monitor the changes in the average weight of individuals throughout the life of a subterranean termite colony, it can be used as proxy to estimate the relative age of a termite group, which in turn could inform the natality and mortality rate for the entire colony over time.
In 1985, the University of Florida Ft Lauderdale Research and Education Center subterranean termite laboratory was established by NYS to address the rising problems resulting from the recent establishment of Coptotermes formosanus Shiraki (Blattodea:Rhinotermitidae) in Florida (Koehler, 1980), an invasive termite species in the United States, with a major structural pest status (Lax and Osbrink, 2003; Rust and Su, 2012; Chouvenc et al., 2016; Evans, 2021). To study the foraging behavior of this invasive species, field ground traps were established on properties with active termite infestation and were monitored by PMB. In addition, such traps were used to collect large numbers of termites to provide biological material for laboratory experiments. As a result, more than 250 studies were published using such source material between 1986 and 2009 from this laboratory. However, as termites were collected, a series of measurements were recorded, resulting in the acquisition of a vast database from more than 15,000 field traps over the span of 24 years from more than 120 different sites. This database was digitized between 2009 and 2014, and it was recently partially curated by TC. Therefore, as a result of the existence of this dataset, it is now possible to look into the fundamental demographic changes throughout the life of subterranean termite colonies and reveal aspects of their ecology that were previously unsuspected. The current study is the first of an upcoming series of demographic studies that will use this subterranean termite dataset to answer a set of questions that emerged during its initial analysis. In this first study, we focused on age demography of colonies over time (using worker, soldier and nymph average weights as proxies), and highlighted the senescence processes of large mature colonies, with a multidecade monitoring approach of four distinct field sites with C. formosanus activity.
Materials and Methods
Termite Collection and Processing Protocols
Termite ground traps (often referred in the termite literature as “bucket trap”) were installed at locations with known C. formosanus activity, following the protocol established by Su and Scheffrahn (1986). However, this protocol was modified over the years to minimize potential disturbances and increase the number of termites collected, while streamlining the termite processing method when brought back to the laboratory. Paradoxically, while this modified termite trapping method was used for more than 20 years of termite collection, it was never fully redescribed properly. We here provide an updated overview of this procedure.
Wooden stakes (Picea sp.) were installed in the ground of properties with known C. formosanus activity and monitored periodically (1–3 months). When foraging termites were found feeding on a wooden stake, a hole around the stake was dug in the ground, a bottomless bucket (18 cm diam × 20 cm height) was installed, a bundle of wood was placed at the bottom of the hole and a lid was placed on top of the plastic container. A bundle of wood consists of five 15 × 10 × 0.3 cm pieces of wood (Picea sp.), each separated by two 8 cm plastic straws, and bundled all together by two plastic-coated metal wires tied around it. By twist-tying the wires, the straws compressed to the point of creating a ∼2 mm gap between each wood layer. The wood for each bundle was oven-dried at 73°C for 3 days and weighted prior to assembly. A serial number was attributed to each bundle using a 2 × 4 × 0.2 cm plastic label stappled on one of the sides of the wood bundle. Periodically, the bundles were collected from the field traps, and replaced with new bundles for ongoing monitoring. The retrieved bundles were then individually processed in the laboratory using the Tamashiro et al. (1973) method, which uses moist wooden tong-depressors as ramps for termites to sort themselves out from debris, toward a clean wooden slab. It allows for a rapid processing of a large numbers of termites while limiting injury levels. The remaining wood material from the bundle was cleaned up, oven-dried for 3 days and weighted again to determine net wood consumption. The rationale behind this overall standardized protocol using wood bundles was to create a space (provided by the compressed straws) that favors termite aggregation and access to feeding surfaces when in the bucket ( = “termite space,” Chouvenc et al., 2011), to maximize the number of termites collected, but also to expedite termite processing and minimize damage done to termites in the laboratory by simply cutting the two wires and sorting wood debris and straws from termites.
Between 1986 and 2009, a total of 15,016 wood bundle samples from ground monitoring traps located at more than 120 independent sites were collected and processed. In the current study, data from a selection of four distinct sites located in Hallandale, FL were used, here referred as sites Cf1, Cf2, Cf3, and Cf4, for a total of 3,491 cumulated traps. Here, “site” refers to a delineated monitored area, but depending on the context, may reflect the demographic changes of a single colony, or in some cases, a succession of multiple colonies, as explained in the results. These four sites were selected from the rest of the dataset because of their relatively long sampling timeline compared to all other sites (10, 11, 22, and 24 years respectively). The rest of the unused data will be used in follow-up studies that will focus on aspects of termite demographics not discussed here-in. Over the years of monitoring, new wooden stakes were regularly placed on surveyed sites in order to maintain ongoing observation of termite colonies from multiple trap locations, in case of temporary or definitive loss of foraging termite activity from one or more traps. Wood bundles were collected and replaced from field traps periodically (frequency varied greatly, see below), resulting in a large collection of groups of termites from the field. With few exceptions of triple mark-recapture procedures for colony size estimations as the ones performed in Su and Scheffrahn (1988a) and to confirm that different traps at a site were accessed by a single colony, termites were never returned to their colony of origin, but were instead used for laboratory experiments for over two decades. Such protocol implies that from the 10–24 years of monitoring, millions of termites were removed from established colonies. While this protocol inherently altered colony demographics each time termites were collected, we here argue that its impact may have been negligeable, as it was estimated that less than 0.5% of the overall termite colony population were sampled on a monthly basis (Su and Scheffrahn, 1988a; Chouvenc and Su, 2014).
Data Acquisition
The termite population obtained from a single wood bundle sample represents a snapshot of its foraging demographics at a given foraging location. By accumulating several snapshots over many years, it provided a trend in foraging population dynamic of a given colony at any given time. Each time a wood bundle was brought back from a field trap, it was processed in the laboratory, all castes were separated to individual containers, and a series of variables were recorded: Site of origin, trap #ID at the site, date placed in the ground, date collected from the ground, initial wood weight, final wood weight, and the number and the total mass of workers, soldiers, nymph, alates and secondary reproductives, if any. Data about the presence of a brood (eggs/larvae) was not found in the trap records, but it is unknown if the presence of a brood was overlooked or it was never found at foraging sites [although, the brood in Coptotermes is expected to be found at the central part of the nest, not at foraging sites, according to Kakkar et al. (2017), who used laboratory colonies with primary reproductives only].
Workers represented the largest proportion of the individuals collected, so in order to determine the number of workers without having to count them individually, a subsampling protocol was established to estimate the total number of workers from the processed wood bundle. Five groups of 10 workers were randomly subsampled from the pooled workers and the total weight of each group of 10 workers was noted to calculate the average worker weight from each subsample. Then, the average weight of an individual worker from a given wood bundle was determined by averaging the five worker weight subsample averages, indirectly providing information (standard deviation = weight heterogeneity within a given trap) on the potential variability of worker weights within a given foraging trap. Once the average worker weight was determined, the total worker mass was then used to estimate the total number of workers from a wood bundle. For all other castes, all individuals were counted, and the average individual weight was inferred from the total mass of each caste.
Data Curation
Data acquisition quality was variable over the 24 years of trap collection. While the protocol was standardized in the early days of termite trap collection, the manual labor to process the traps and record the variables were partially performed by many different temporary technicians over the years. As a result, some variables were acquired inconsistently, weight calculations were sometimes inaccurate, and notes sometimes lack context to make sense of it. In addition, as the initial records were manually collected in notebooks, the subsequent digitalization process possessed inherent typos. Therefore, by cross-referencing all resources available, the dataset was carefully curated to ensure the reliability of the data obtained and outliers were all checked for validity. A majority of suspicious outliers resulted from a simple misplaced decimal or from typing an adjacent number on the keypad. By checking back on the original written records, each of them was corrected accordingly. However, despite a thorough curation process, many trap records were unusable owing to their lack of reliability (undecipherable handwriting, orders of magnitude outliers with no clue on how wrong the calculations were made, entry too incomplete to make sense out of it, etc.). Thus, out of the 3,491 wood bundles collected from traps at the four sites of interest, 3,067 trap records could be used with confidence in this study, while 424 unreliable trap records were removed from the analysis. Finally, the wood consumption data only was partially recorded over the years, explaining some of the discrepancies in the number of traps used across variables in the results.
It is here important to emphasize that the data was acquired with the initial purpose of informing on the quality of the termites collected from the field prior to using them for experimentation in the laboratory (1986–2009). There was no initial tangible intent to compile all entries from all these years and to perform the analysis presented in the current study. Therefore, while data collection could have been more rigorous in hindsight, additional variables could also have been acquired (worker instar composition, dry weight, molting frequency, sex determination of individuals, distances between traps, etc.), but because of limited time and resources, the need to focus on the experiments at that time, and no plan for a multidecade analysis, we are limited to what was collected.
Variables of Interest
The average weight of individual castes was used as a proxy to determine the relative developmental stage of the foraging population at the time of the sampling, which directly reflects on which instars were present, and by extension, reflected on the general demography of the colony (Grace et al., 1995; Chouvenc and Su, 2014; Su et al., 2017, see “development pathway context” overview below). Therefore, the variability of the age composition of individuals over time was the primary focus of the study.
While colony size could have been an important variable to obtain, the number of termites collected at each sampling event cannot be used as a proxy to estimate the actual population size of colonies. Coptotermes formosanus colonies can reach populations of several millions, can fluctuate extensively over time, and colony size estimation protocols for a single point in time is resource and time consuming, which prohibits the scaling of the process to repeated measurement (King and Spink, 1969; Su and Scheffrahn, 1988a; Su, 2013). In addition, the overall data on caste ratios, total number of termites collected, feeding activity over time, and on other aspects this dataset can inform on termite colony functioning will be discussed in future papers. However, in special “demographic transitions” described in the current study, some of these variables were inspected to provide additional elements for interpretations.
Developmental Pathway Context to Interpret Colony Age Demographics
A background about developmental pathways in Coptotermes is first necessary to place age demography data in a relevant context (Figure 1). In Coptotermes, eggs (E) hatch into first instar larvae (L1), which then molt into a second instar larva (L2). Here, despite hemimetabolous development, the use of the “larva” term follows the analogous function of immature individuals as found in social Hymenoptera, while “nymph” refers to an individual on the imaginal line (wing buds present), as per Thorne (1996). Second instar larvae (L2) can then molt into a first instar worker (W1 = nutritionally competent individual that start performing various tasks in the nest), which then successively molt into older worker instars (→ W2 → W3 → …) until they die of old age (W6+), and workers progressively grow bigger as they molt into older instars (Shimizu, 1962; Higa, 1981; Du et al., 2016). Thus, a group of workers with a relatively small average weight indicates a group of relatively young workers, and vice versa. Also, weight fluctuation may have nutritional and moisture components, but we assume it to be negligeable in the context of this study and was not determined at the time of sampling. It was also shown that foraging termites display degrees of age polyethism, as older workers tend to forage further away from the central part of the nest than younger workers (Du et al., 2017; Su et al., 2017; Lee et al., 2021). It therefore explains why the demographic profile of a colony may vary among traps on any given sampling date, as the age cohort of foragers are not homogeneously distributed throughout the colony foraging territory (Lee et al., 2022). For a given colony with multiple monitored foraging sites, it can therefore be inferred that a trap with a majority of old workers is located further away from the central part of the nest than a trap with a majority of relatively young workers (Shimizu, 1962; Su et al., 2017; Lee et al., 2022). In mature colonies of C. formosanus, soldiers (S) are produced from various worker pathways through a presoldier stage (PS), which depends on the availability of workers at the time they initiate differentiation into soldiers: W1 → PS2 → S2, W2 → PS3 → S3, and W3 → PS4 → S4. Contrary to workers, the weight of soldiers cannot be used as a proxy for their relative age, as the soldier caste is final and no longer molts. Instead, the later the soldier pathway, the bigger the soldier, so the average weight of soldiers indirectly informs on the relative worker demographics at the time the individuals engaged into soldier development (Park and Raina, 2003; Chouvenc and Su, 2014). Nymphs (N) are produced seasonally toward the production of imagoes (alates) for dispersal flight events (Chouvenc et al., 2017). In Coptotermes, nymphs are produced from second instar larva (L2 → N1 → N2 → … → N6 → Alate) and can be found at foraging sites (Albino and Costa-Leonardo, 2011). Late instar nymphs may also be the source for the production of secondary reproductives (Chouvenc and Su, 2014). Relatively few alates were collected in this study, as it is possible that alates move above ground (trees) prior to their dispersal flights and may only be present in traps for a short period.
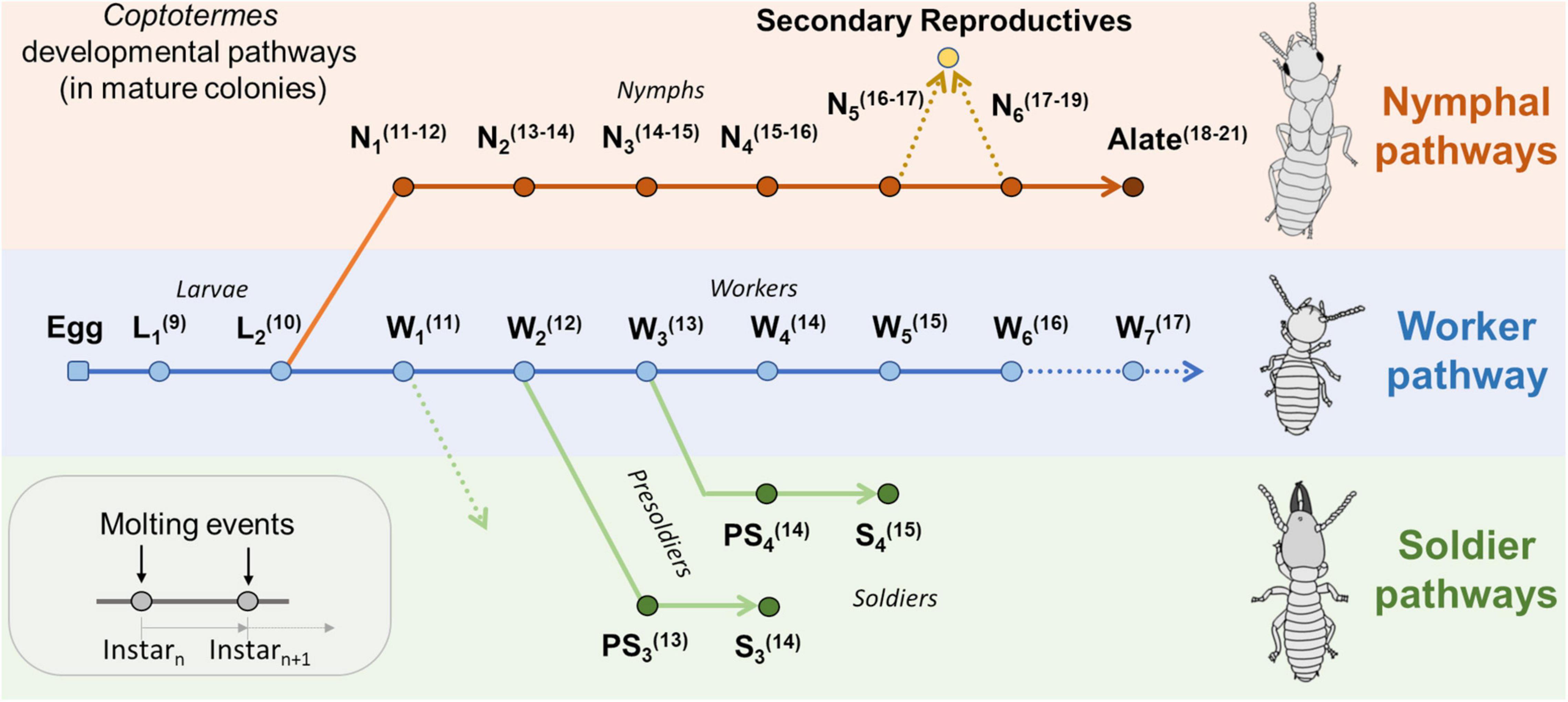
Figure 1. Developmental pathways expected in mature colonies of Coptotermes formosanus, modified from Chouvenc and Su (2014). Ln, Larva instar; Wn, Worker instar; Nn, Nymph instar; PSn, Presoldier type, and Sn, Soldier type. Values in parenthesis(n) indicate the expected number of antennomeres for each given instar.
Statistical Analysis
For all monitored sites (Cf1, Cf2, Cf3, and Cf4), the worker average weight, the soldier average weight, and the nymph average weight were scatter-plotted over time, where one datapoint represents the average weight of one trap. For each caste, an average weight trend was generated using a rolling average function (period = 3, i.e., previous, current, next sampling event) to soften sharp fluctuations and outliers over time. The generated figures are purely descriptive of the demographic changes that occurred at these four foraging sites over time. Next, for each site, the relationship between the average weight of workers and the weight of soldiers and nymphs, respectively, were all positively correlated (Pearson correlation, p < 0.001 for all), so a simple linear model (lm, linear) was used in R v 4.1.0 (R Core Team, 2021) using the default and ggplot2 packages.
Then, the seasonal fluctuations of caste weights were investigated by pooling the data from several years with cyclical trends into a single combined annual dataset (1989–1993 for Cf1, 1993–1996 for Cf2, 1997–2008 for Cf3, and 1988–1997 for Cf4). From these combined annual datasets, for workers and soldiers, weights followed sinusoidal seasonal function (lm, sin). However, nymph weight followed a different seasonal pattern, progressively increasing from May to January, but then plateauing or decreasing from January to May as they eventually go through imaginal molt. A cubic polynomial function was therefore applied (lm, poly3) on the combined nymph datasets (best fit).
To investigate the demographic changes during critical transitions, additional parameters were produced. The standard deviation ( = heterogeneity of age cohorts within a given trap) associated the average worker weight values (obtained from five subsamples of 10 workers) was scatter-plotted with the average worker weight, and 95% ellipses were generated with ggplot2, using pre-transition, transition, and post-transition values as factors of the ellipses for visual representation. This analysis allowed us to determine if there was a strong heterogeneity of worker weight among subsamples during a demographic transition, implying a potential colony fusion event, a territorial takeover from a neighboring colony, or a long hiatus in oviposition activity. Finally, when comparing two different time periods, data from each period were independently pooled and compared using t-tests or ANOVA, depending on the number of factors.
Notes About Data Interpretation
In this paper, our ability to interpret demographic events from the dataset fundamentally relies on the established literature about Coptotermes foraging ability (Shimizu, 1962; Nakajima et al., 1963; King and Spink, 1969; Su and Scheffrahn, 1988a; Grace et al., 1995), on the recent advancements in our understanding of Coptotermes biology at the colony level (Messenger et al., 2005; Chouvenc and Su, 2014; Du et al., 2016, 2017; Su et al., 2017; Kakkar et al., 2018; Chouvenc, 2019) and on our direct observation of large mature colonies reared in our laboratory for a decade (Chouvenc, 2022). However, to our knowledge, the dataset provided in this study is beyond the timescale and the population scale of any previous study on any social insects. The complexity of the demographic variation of colonies over time maintains degrees of challenges on potential interpretative scenarios. In addition, the data acquired through field traps are reflecting a demographic fraction of the foraging populations at the time of collection. These data represent a temporary window within the life of termite colonies and remains mostly fragmentary. Therefore, there is an inherent speculative approach to result interpretation in the current study. However, they are all rooted in our own experience of rearing subterranean laboratory colonies to maturity (Chouvenc, 2022), and in the extensive literature about Coptotermes foraging ability in the field and in the laboratory. Ironically, a voluminous section of such literature was produced over several decades by using the termites referred to in the current study (virtually everything published from Su and colleagues between 1986 and 2009).
Results and Discussion
Sampling Metadata
Sampling frequency varied greatly among sites and across time. A summary of the overall data acquired for each of the four sites provides an overview of the sampling effort and the resulting termite activity profile of each surveyed site (Table 1).
Demographic Observations Over Time
Each site displayed a unique demographic profile in the population structure of foraging termites over time. Such observations provide a novel understanding about age demographic succession within single colonies, as the average age or developmental status directly depends on the mortality and natality rates of individuals. The following section provides comments on the observations made for each site, which reflects on how colonies display unique demographic profiles over time. However, the sites are described in order of interpretative complexity, where the information provided from the first site informs aspects of the second site, etc. Finally, the interpretation of demographic changes is provided linearly through the chronology of events for each colony, so it is highly recommended to refer to the figures when indicated throughout the following sections, as the visual representation of the data is indispensable to follow our interpretations.
Site Cf1, a Simple Perennial Trajectory With Final Senescence
This site apparently contained a single termite colony during the time of observation (1988–1997). The worker average weight (Figure 2A) was relatively stable between 1988 and 1993, with noticeable seasonal fluctuations that followed a sinusoidal function (F2,307 = 90.73, p < 0.001, R2 = 0.372) (Figure 2B). Such seasonality was previously noticed in Coptotermes (Waller and La Fage, 1987a) where workers tend to be bigger in winter months than in spring-summer months. In 1993–1994, a drop in worker’s weight reflected a relatively progressive change in the age structure of foragers, with the presence of many younger workers, potentially indicating an increase of either egg production from primary reproductives, an addition of secondary reproductives, and/or the progressive death of many old individuals, resulting in an overall rejuvenation of the population. However, starting in 1995, the colony progressively accumulated old workers until 1997 when the colony finally collapsed and died (as a result, monitoring was terminated), supporting the observations by Grace et al. (1995) and Chouvenc (2018) that senescent colonies primarily contain old individuals. Such observation reveals that reproduction stopped relatively suddenly, with the putative death of the queen(s) in late 1994, as it was previously observed that in absence of reproduction, Coptotermes workers and soldiers can survive for 3–4 years in laboratory and then die of old age (Chouvenc et al., 2013b). Alternatively, queens may stop laying eggs several months prior to their death (Bess 1970), implying that reproduction may have stopped while the presence of the live queen could have temporarily inhibited the production of secondary reproductives (Costa-Leonardo et al., 2004; Chouvenc and Su, 2014), although the socio-physiological processes involved in the emergence of replacement or supplementary reproduction in Coptotermes remain poorly understood.
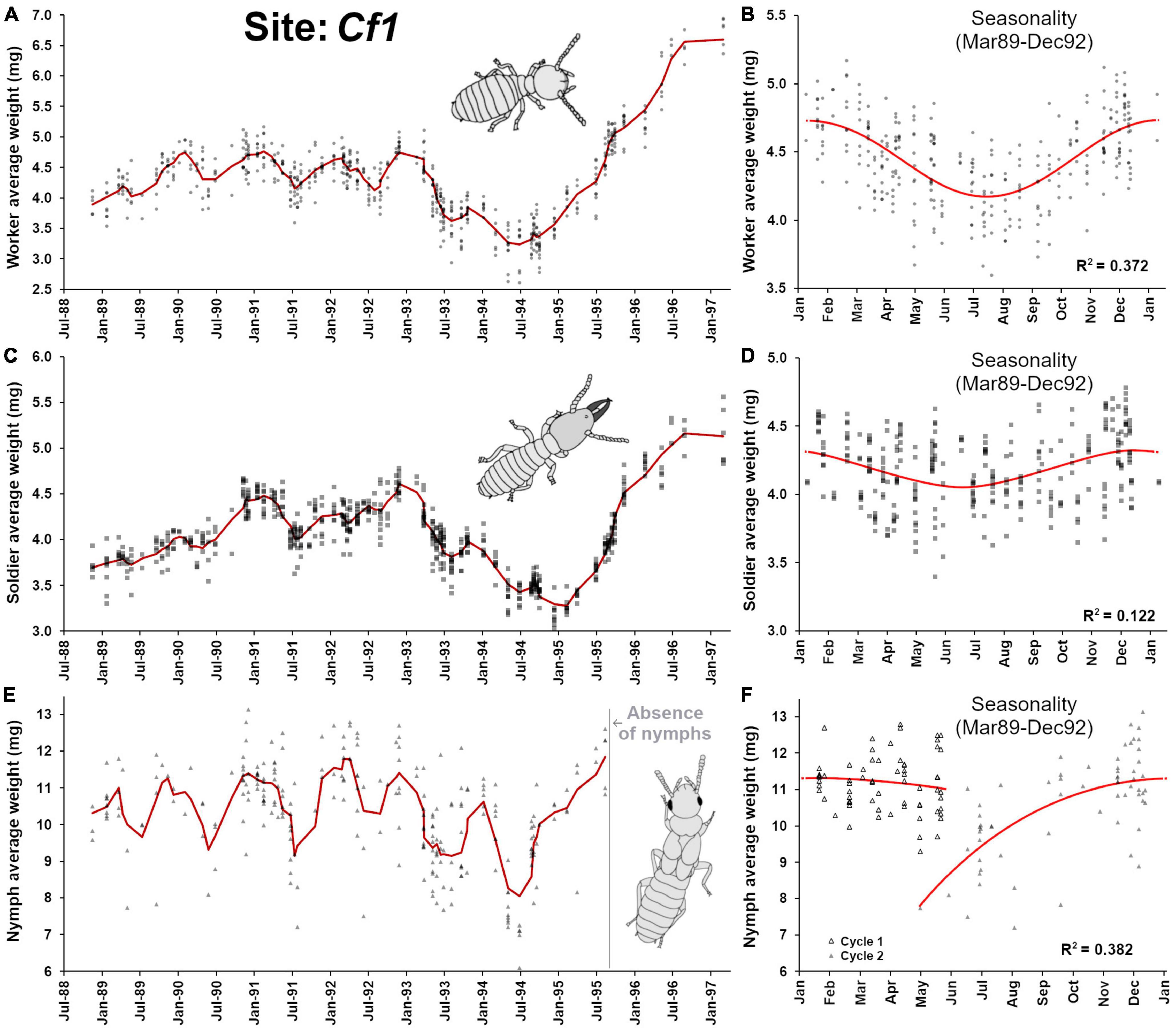
Figure 2. Colony demographic changes for Coptotermes formosanus at site Cf1. Each data point represents an average caste weight from a field trap. For the 1988–1997 demographic trends of each caste, the red line represents the rolling average function (period = 3) (A) Workers’ average weight between 1988 and 1997. (B) Workers’ average weight seasonality, following an annual sinusoidal function (F2,307 = 90.73, p < 0.001, R2 = 0.372). (C) Soldiers’ average weight between 1988 and 1997. (D) Soldiers’ average weight seasonality, following an annual sinusoidal function (F2,307 = 21.41, p < 0.001, R2 = 0.122). (E) Nymphs’ average weight between 1988 and 1997. (F) Nymphs’ average weight seasonality, with an annual growth that follows a polynomial function (poly3, F3,128 = 26.37, p < 0.001, R2 = 0.382). Note the absence of nymphs at foraging sites after the 1995 dispersal event and throughout the colony senescence.
The weight profiles of soldiers followed closely the ones from workers throughout the observation (Figures 2A,C), including some degree of seasonal fluctuations (F2,307 = 21.41, p < 0.001, R2 = 0.122) (Figure 2D) but less pronounced than the one observed in workers. Notably, the magnitude of the final increase of weight in soldiers after 1995–1997 was not as marked as the one observed in workers [worker average weight/soldier average weight ratio before January 1995 = 1.04 ± 0.06 (n = 69), after January 1995 = 1.21 ± 0.04 (n = 13), t = 8.82, df = 80, p < 0.001]. This reflects that as the termites were aging in the absence of population renewal during colony senescence, W5 and higher worker instar accumulated in the colony and as soldier replacement is presumably limited to pathways initiating from W3 maximum (Chouvenc and Su, 2014), the production of “bigger” soldiers was not possible through W4 and higher. Therefore, toward the end of colony senescence, the production of soldiers may be terminated by default (absence of < W5), preventing the ∼1:1 average weight ratio between the two castes observed throughout the rest of the senescence of the termite colony, because of the progressive accumulation of old workers. Nymphs were produced by the colony at the Cf1 site between 1988 and 1995 (Figure 2E), confirming that by the time the observation started, the colony already reached maturity and was at least 5 years-old (Chouvenc and Su, 2014). Nymph weight followed a seasonality, with relatively small nymphs being observed at foraging sites around May of each year. These nymphs progressively increased their weight as they went through successive molts to mature, until reaching a plateau between Jan and May of the following year pattern (Figure 2F, F3,128 = 26.37, p < 0.001, R2 = 0.382) as they eventually turned into alates and exited the colony via dispersal flights (April-June). Our observation confirms that in Coptotermes mature colonies, nymphs can be found at foraging sites year-round (Albino and Costa-Leonardo, 2011). Remarkably, nymphs were absent from the colony from July 1995 until the death of the colony in 1997. This nymph absence highlights that with the lack of reproduction starting around late 1994, the brood that initiated nymphal development in 1994 was able to proceed to maturity into imago in 1995. However, the subsequent absence of eggs and larvae in 1995 shut down possibilities for the nymphal pathway to occur in the following years (Raina, 2006), ultimately resulting in a nymphless colony throughout senescence.
A strong positive relationship (lm, F1,620 = 879.0, p < 0.001, R2 = 0.586) (Figure 3A) was found between the weight of worker and soldier throughout the period of observation. A weaker relationship was found between workers’ weight and nymphs’ weight (lm, F1,258 = 151.1, p < 0.001, R2 = 0.369) (Figure 3A). This discrepancy can be explained by the fact that soldiers are produced through the pool of available workers and both their developmental status are inherently tied. In addition, the changes of their demographic representation at foraging site partially depends on a sinusoidal seasonal fluctuation, while being continuously produced throughout the year through overlapping generations. On the contrary, nymphs are initiated yearly during a limited period of time and then progressively matured throughout the following year. During this time, many larvae that would have contributed to the production of workers are instead initiating a nymphal developmental pathway, reducing the pool of individuals that would otherwise sustain the production of workers and soldiers. Therefore, nymph initial production (L2 → N1) may directly contribute to a temporary increase in the average age of workers in the following months while nymphs continue to mature. It is therefore possible that the seasonality fluctuations in the workers age demographic are partially the result of temporary larval investment into nymphal production and not just from environmental fluctuations (Waller and La Fage, 1987b).
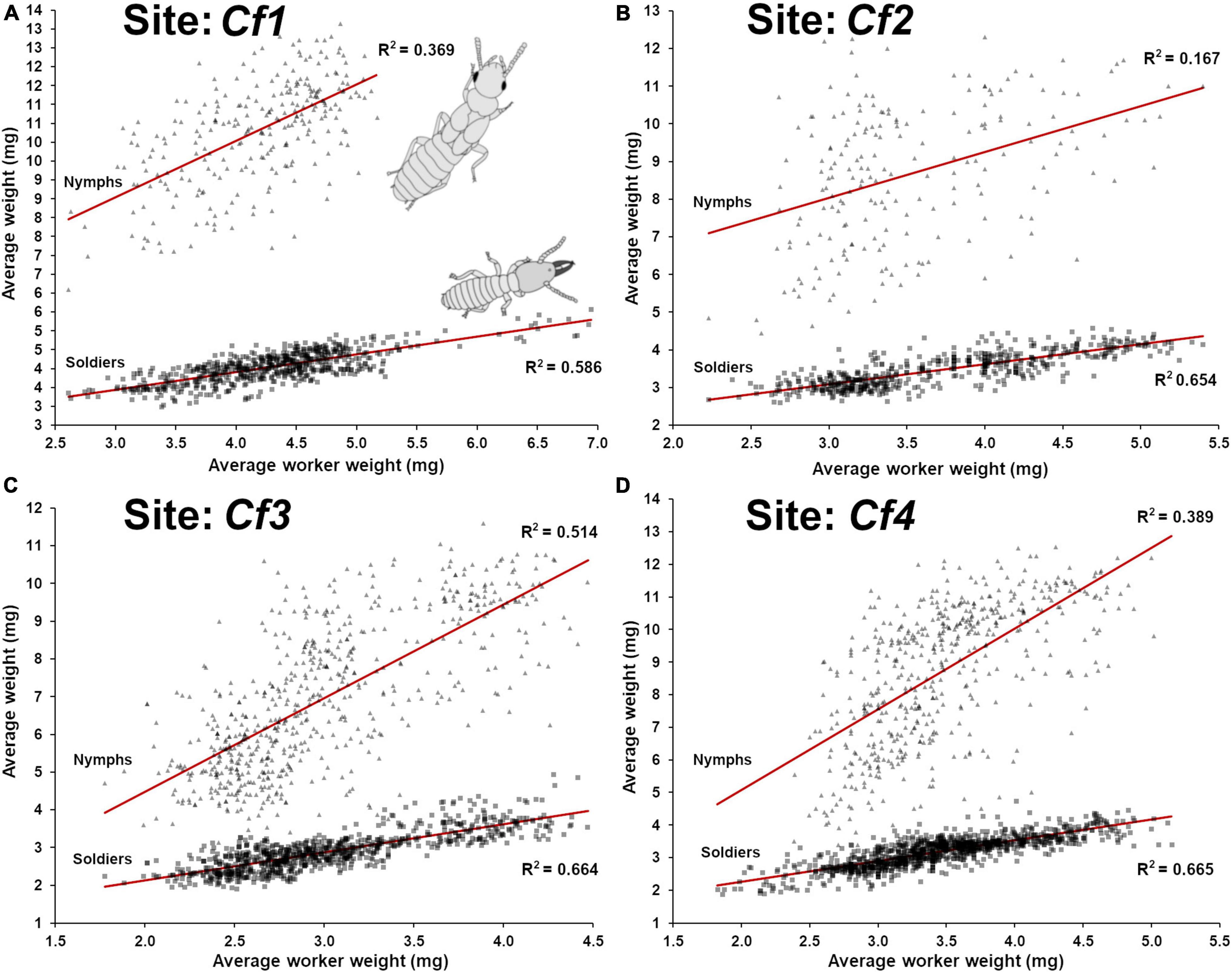
Figure 3. Relationship between the average weight of workers and the average weight of soldiers and nymphs in field traps throughout the time of a site observation. (A) Site Cf1 (1988–1997), worker-soldier (lm, F1,620 = 879.0, p < 0.001, R2 = 0.586) and worker-nymph (lm, F1,258 = 151.1, p < 0.001, R2 = 0.369) relationships. (B) Site Cf2 (1986–1996), worker-soldier (lm, F1,523 = 989.6, p < 0.001, R2 = 0.654) and worker-nymph (lm, F1,197 = 38.6, p < 0.001, R2 = 0.167) relationships. (C) Site Cf3 (1988–2009), worker-soldier (lm, F1,852 = 1,687, p < 0.001, R2 = 0.664) and worker-nymph (lm, F1,611 = 646.7, p < 0.001, R2 = 0.514) relationships. (D) Site Cf4 (1986–2009), worker-soldier (lm, F1,1064 = 2,116, p < 0.001, R2 = 0.665) and worker-nymph (lm, F1,508 = 323.2, p < 0.001, R2 = 0.389) relationships.
In addition, Coptotermes late instar nymphs were suggested to be a non-dependent caste because of their ability to directly feed on wood at foraging sites (Crossland and Su, 2006; Albino and Costa-Leonardo, 2011). We here also argue that their presence at foraging site is an efficient strategy for the colony to limit the cost of the inherent nutritional requirements for alate production, by bringing the developing nymphs directly to where the resources are located. Some of the relatively young nymphs show up in May at foraging sites at 6–9 mg, already as advanced nymphal instars. It implies that the initial development of nymphs most likely starts much earlier, safe around the central area of the nest, while later instar nymphs move to foraging sites. Therefore, it establishes that the developmental process from N1 to alate takes much more than a year to complete, implying that the alate production cycles for two consecutive years largely overlap.
Finally, only 8 secondary reproductives were haphazardly collected as singletons at site Cf1 between 1989 and 1994. Their presence was not visibly associated with any demographic events but their relative rarity cannot exclude that other secondary reproductives may have been present in the colony at the central part of the nest or other non-monitored foraging sites. Notably, no secondary reproductive was found in the colony after 1995 when the colony accumulated extremely large worker and soldier individuals (Figures 2A,E, 3A), reinforcing the argument that in the absence of larvae, the nymphal pathway necessary to produce secondary reproductive is shut to the senescent colony, sealing its final demise (Nakajima et al., 1963; Lenz et al., 1988; Myles, 1999; Raina, 2006; Chouvenc and Su, 2014).
Site Cf2, a Perennial Trajectory With a Reproductive Shift
This site was monitored for 11 years, from 1986 to 1996 (Figure 4A), but sampling events between 1986 and 1996 were irregular, providing a relatively poor resolution in workers’ demographic changes. However, in 1991–1992, old workers progressively accumulated in the colony, followed by a sudden rejuvenation of the worker pool in 1993 (here referred as “transition A”). Following this transition, a drastic drop in worker’s weight was observed until 1996, with weak sinusoidal seasonal patterns (Figure 4B, F2,201 = 4.89, p = 0.008, R2 = 0.047). As seen in Cf1, the soldier weight closely followed the weight variation observed in workers (Figure 4C), although an apparent delay of ∼3 months can be seen in variation patterns. Again, this reflects that soldier production and replacement are driven by the worker pool available at the time of individual workers initiating soldier differentiation (Park and Raina, 2003; Liu et al., 2005; Chouvenc and Su, 2014), for which additional time is needed for the full presoldier-soldier differentiation. Contrary to workers, the seasonality of the change in soldier weight was strongly marked (Figure 4D, F2,201 = 161.4, p < 0.001, R2 = 0.616). Nymphs were haphazardly collected from traps from 1987 to 1992, with a notable absence of nymphs throughout 1991, but their presence could then reliably be confirmed after transition A (Figure 4E), with a distinct seasonal production (Figure 4F, F3,115 = 87.2, p < 0.001, R2 = 0.695), as observed in Cf1. Finally, similar as Cf1, the trends of relationships between weight of workers and the weight and soldiers (R2 = 0.654) and of nymphs (R2 = 0.167). It confirms that larvae are engaging in the nymphal developmental pathway within a narrow window of time and mature over the following year, while worker and soldier developments are tied and partially depend on the pool of larvae available at the time of their differentiation. Note that contrary to Cf1, no final senescence and colony death was observed in Cf2, and nymphs were associated even in traps with relatively old workers (Figure 3B).
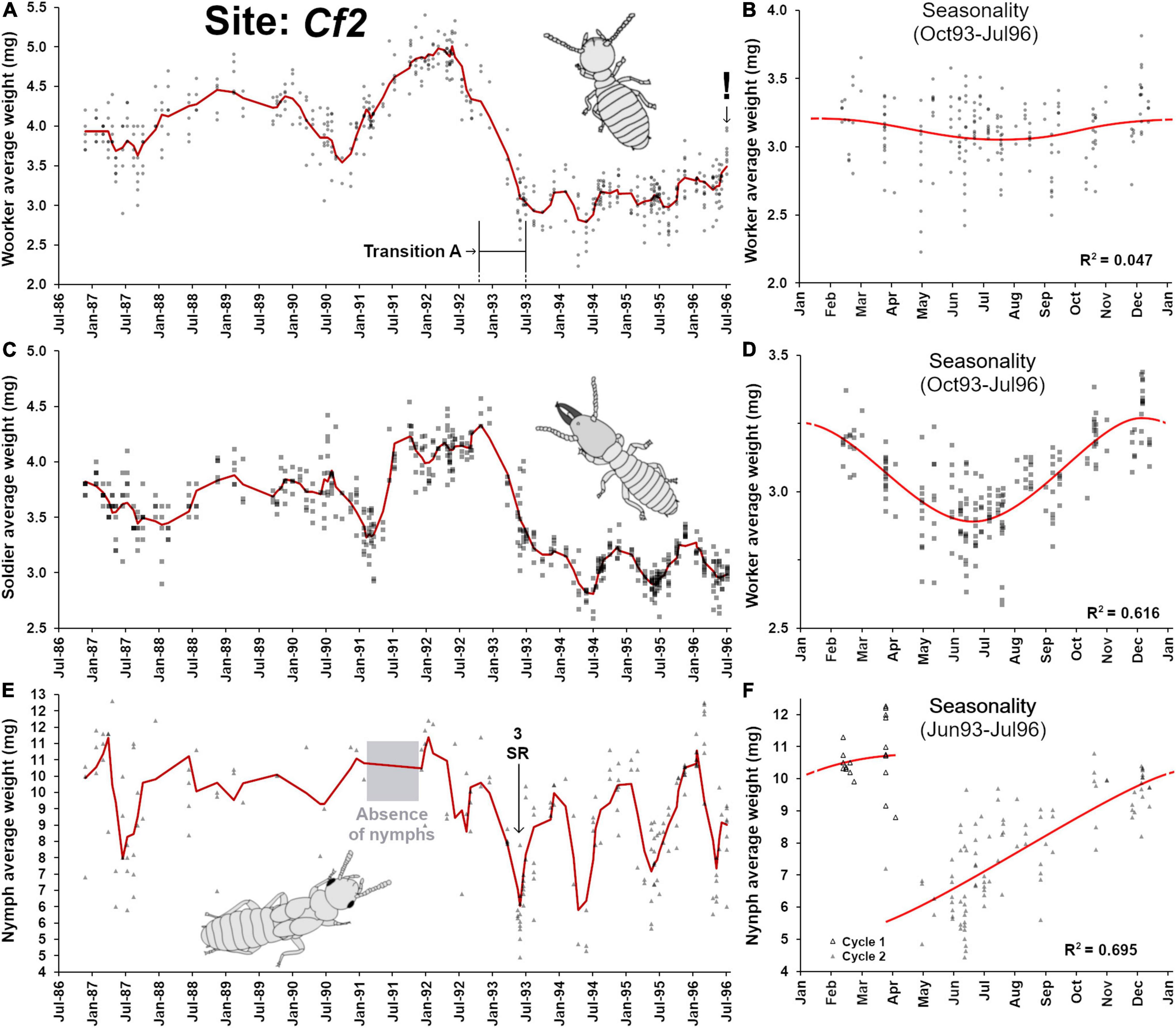
Figure 4. Colony demographic changes for Coptotermes formosanus at site Cf2. Each data point represents an average caste weight from a field trap. For the 1986–1996 demographic trends of each caste, the red line represents the rolling average function (period = 3). (A) Workers’ average weight between 1986 and 1996. (B) Workers’ average weight seasonality, following a weak annual sinusoidal function (F2,201 = 4.89, p = 0.008, R2 = 0.047). (C) Soldiers’ average weight between 1986 and 1996. (D) Soldiers’ average weight seasonality, following an annual sinusoidal function (F2,201 = 161.4, p < 0.001, R2 = 0.616). (E) Nymphs’ average weight between 1986 and 1996. (F) Nymphs’ average weight seasonality, with an annual growth that follows a polynomial function (poly3, F3,115 = 87.2, p < 0.001, R2 = 0.695). “Transition A” represents a major reproductive shift within the colony, with a hiatus in reproduction, followed by the re-establishment reproduction via secondary reproductives ( = SR).
Two additional notable observations were made: one during transition A and one during the final sampling event. First, the within-trap worker average weight heterogeneity during demographic transition A (January 1993 – September 1993) was higher (0.256 ± 0.131a, n = 36) than the heterogeneity prior (November 1988 – December 1992) to the transition (0.181 ± 0.100b, n = 187, p < 0.01) and the heterogeneity after (October 1993 – August 1996) the transition (0.145 ± 0.071b, n = 203, p < 0.01) (ANOVA, F2,423 = 24.82, p < 0.0001, Tukey HSD, different letters in text indicate α = 0.05 significant difference). Transition A followed a period where older workers and larger soldiers accumulated, with a temporary absence of nymphs in 1991 but a resumption of the presence of nymphs at the foraging sites in 1992 was observed. Combined, these observations imply that the termite colony may have gone through a temporary senescence, with a putative absence or reduction of oviposition for almost a year (starting in late 1990). During transition A, groups of foragers composed primarily of both old and young individuals but with few middle-aged individuals could be observed (high weight heterogeneity). Such situation may be explained by a relatively sudden resumption of oviposition within the colony through the presence of secondary reproductive (three of them were found in 1993, while none of them were collected prior to transition A). An alternative scenario remains possible, where the aging colony at Cf2 fused with a younger neighboring colony (Su and Scheffrahn, 1988b; Vargo and Husseneder, 2009; Husseneder et al., 2012; Lee et al., 2019; Vargo, 2019), resulting in a rapid drop in worker and soldier weights. However, the resumption of the presence of nymphs prior to transition A may make this alternative scenario unlikely, but not mutually exclusive.
The second notable observation relates to a written note found in the records by PMB on August 1st 1996 saying “Sentricon baits found on site, termite collection terminated.” This note was important, as it indicated that the owner of the property had commercial subterranean termite hexaflumuron bait stations (Su, 1994) installed, and that the site could no longer serve as a source for termite experiments in the laboratory. Unfortunately, the opportunity to observe the accelerated senescence of a CSI-baited colonies in the field with the rapid accumulation of old workers (Chouvenc, 2018; Gordon et al., 2022) was missed. However, the last sampling event still revealed the initial colony demographic shift that would be expected with the loss of the brood and young workers (Chouvenc and Lee, 2021), with a sudden rise of the soldier ratios, from an average of 4.26 ± 3.6% (n = 28) in the three months before the implementation of the CSI baits, to 32.44 ± 13.47% (n = 8) on August 1st 1996 (t-test, df = 34, t = 10.18, p < 0.001), and with the sudden increase in workers’ weight from an average of 3.25 ± 0.21 mg (n = 28) in the three months before the implementation of the CSI baits, to 3.68 ± 0.20 mg (n = 8) on August 1st 1996 (t-test, df = 34, t = 5.29, p < 0.001). Therefore, despite the lack of final observation of colony collapse when exposed to CSI baits (Su, 1994, 2019), all the early signs of accelerated colony senescence were confirmed.
Site Cf3, Successional Reproductive Shifts and Long-Term Inbreeding
Site Cf3 differed from the two previous sites on many aspects. First, the time frame of observation was over 22 years (1988–2009), giving a long-term perspective of termite colony activity at this site. Second, the demographic of the colony at this site went through a major shift during a 1995–1996 transition (here referred as “transition B”). Finally, no seasonality was found in worker and soldier weight throughout the observation (Figure 3C). Despite the visual compression of demographic changes from the long observation period (Figure 5A), a remarkable series of aging/rejuvenating cycles in the worker pool was visible. As the colony observation was initiated in 1988–1989, it was already going through a rapid decline in workers’ age demographic, as it was going through a phase of increased oviposition, phasing out an old cohort of workers. Such a pattern indicates that the colony had already reached maturity for several years, as confirmed by the already established presence of nymphs. Then, a slowdown of oviposition resulted in aging cohorts of workers in late 1990. Another rejuvenation period occurred in 1991, followed by another period of reduced oviposition with an accumulation of aging cohorts of workers through 1994. Then, this cycle came to an end with a fundamental shift observed in 1995 (“transition B”), with a prolonged period of high oviposition that lasted for more than 15 years, resulting in a semi-permanent state of a young worker demographic at foraging sites throughout Cf3. The aging/rejuvenation cycle resumed briefly in 2005 and in 2009, with a temporary accumulation of relatively old workers. The fate of the colony is unknown, as collection was terminated in 2009. Soldier colony demographics followed the patterns observed in workers over the years (Figure 5B). Contrary to Cf1 and Cf2, no seasonality fluctuations could be observed in Cf3 workers and soldiers (sinusoidal functions, p < 0.41) throughout the 22 years monitoring period, suggesting that various other factors (environmental and internal) may have influenced population demographics of this colony beyond seasonality and nymph production cycles, as observed in Cf1 and Cf2.
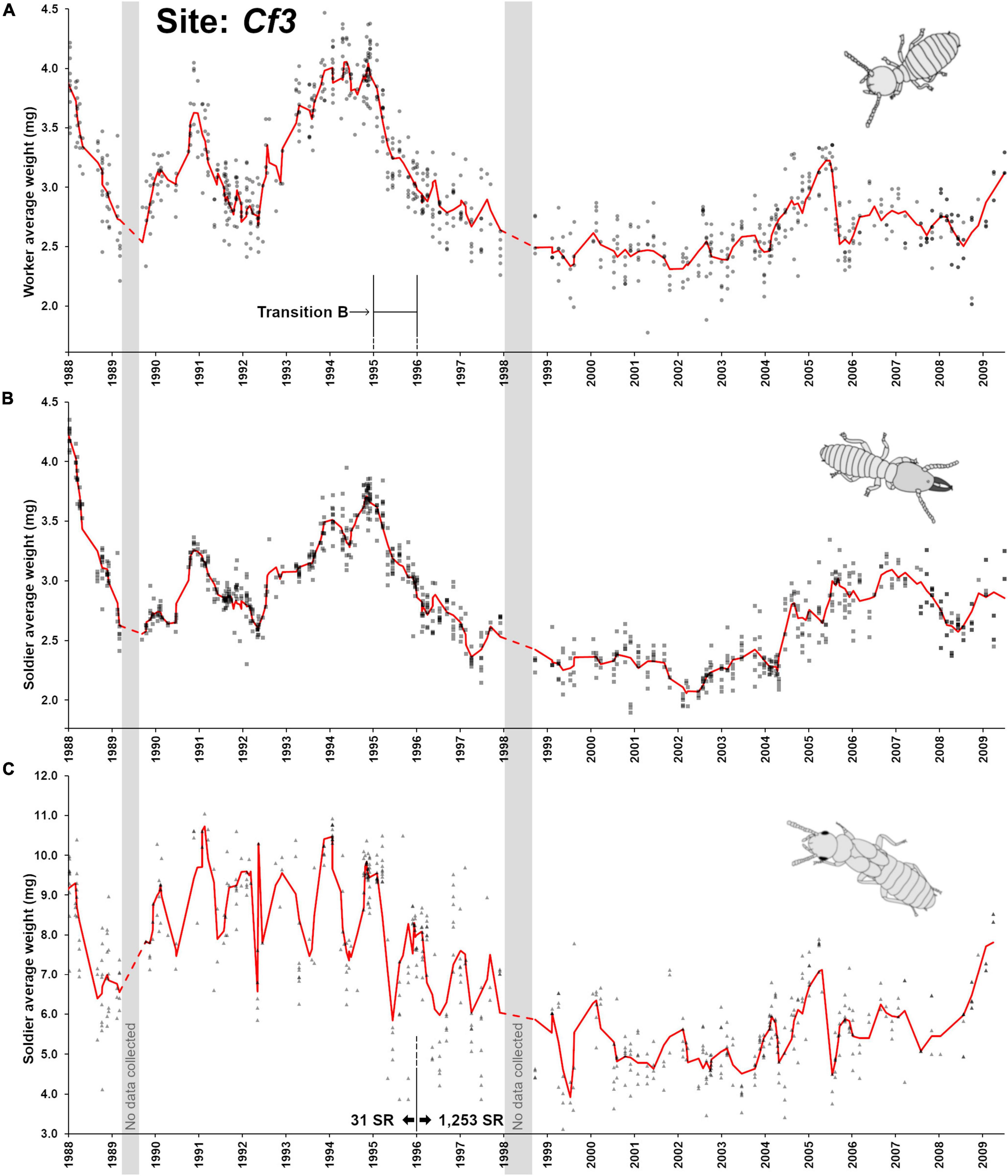
Figure 5. Colony demographic changes for Coptotermes formosanus at site Cf3. Each data point represents an average caste weight from a field trap. For the 1988–2009 demographic trends of each caste, the red line represents the rolling average function (period = 3). (A) Workers’ average weight between. (B) Soldiers’ average weight. (C) Nymphs’ average weight between. “Transition B” represents a major reproductive shift within the colony with the ongoing production of secondary reproductives ( = SR, 1,253 produced after 1995) which may have resulted in cycles of inbreeding. No clear annual demographic cycles could be found in workers and soldiers.
Nymphs were produced periodically each year (Figure 5C) with a marked seasonality in their maturation cycle (Figure 6A, F3,263 = 27.3, p < 0.001, R2 = 0.237). However, nymph demographics displayed two distinct weight patterns (t-test, t = 24.95, df = 611, p < 0.001) from before transition B (8.69 ± 1.4 mg, n = 231) and after transition B (5.87 ± 1.3 mg, n = 382), as nymphs produced after the transition were much smaller (Figure 5C). Alates produced by C. formosanus can vary in size depending on the timing of dispersal within the season and the colony of origin (Chouvenc et al., 2017), but the current observation also indicates that it may depend on the colony breeding structure, as reflected in the small nymph weight after transition B. The change in breeding structure was marked by the number of secondary reproductives found in the colony over time: while 31 supplementary reproductives were haphazardly collected in field traps (12 out of 412 traps) at the Cf3 site between 1988 and 1996, a total of 1,253 supplementary reproductives were collected between 1996 and 2009 (188 out of the 442 traps in this period). The large number of secondary reproductives was associated with relatively small workers, soldiers and nymphs. In addition, secondary reproductive were also much smaller after transition B, from averages of 5.43 ± 0.76 mg, n = 12 prior to the transition, to an average of 3.99 ± 0.44 mg, n = 188 after the transition, (t = 10.38, df = 198, p < 0.001). Reproductive functionality and sex of these secondary reproductives were not reported in the records, but Coptotermes mature colony may accumulate both functional and non-functional brachypterous neotenics (Myles, 1999; Costa-Leonardo et al., 2022). While the relatively small weight of workers and soldiers can partially be explained by the high reproductive rate of the colony from the large number of supplementary queens in the colony after 1996, the small weight of nymphs cannot. Instead, we suggest that transition B led to a long cycle of inbreeding within the colony (Vargo, 2019), resulting in developmental limitations for all castes, with smaller morphs produced as proposed by Husseneder et al. (2005). This observation in Cf3 also highlights that over time, colony demographic traits and breeding structure can be altered to extremes, as a colony may resort to various reproductive strategies throughout its lifetime, to ultimately optimize its fitness while being constrained by its ongoing demographic status.
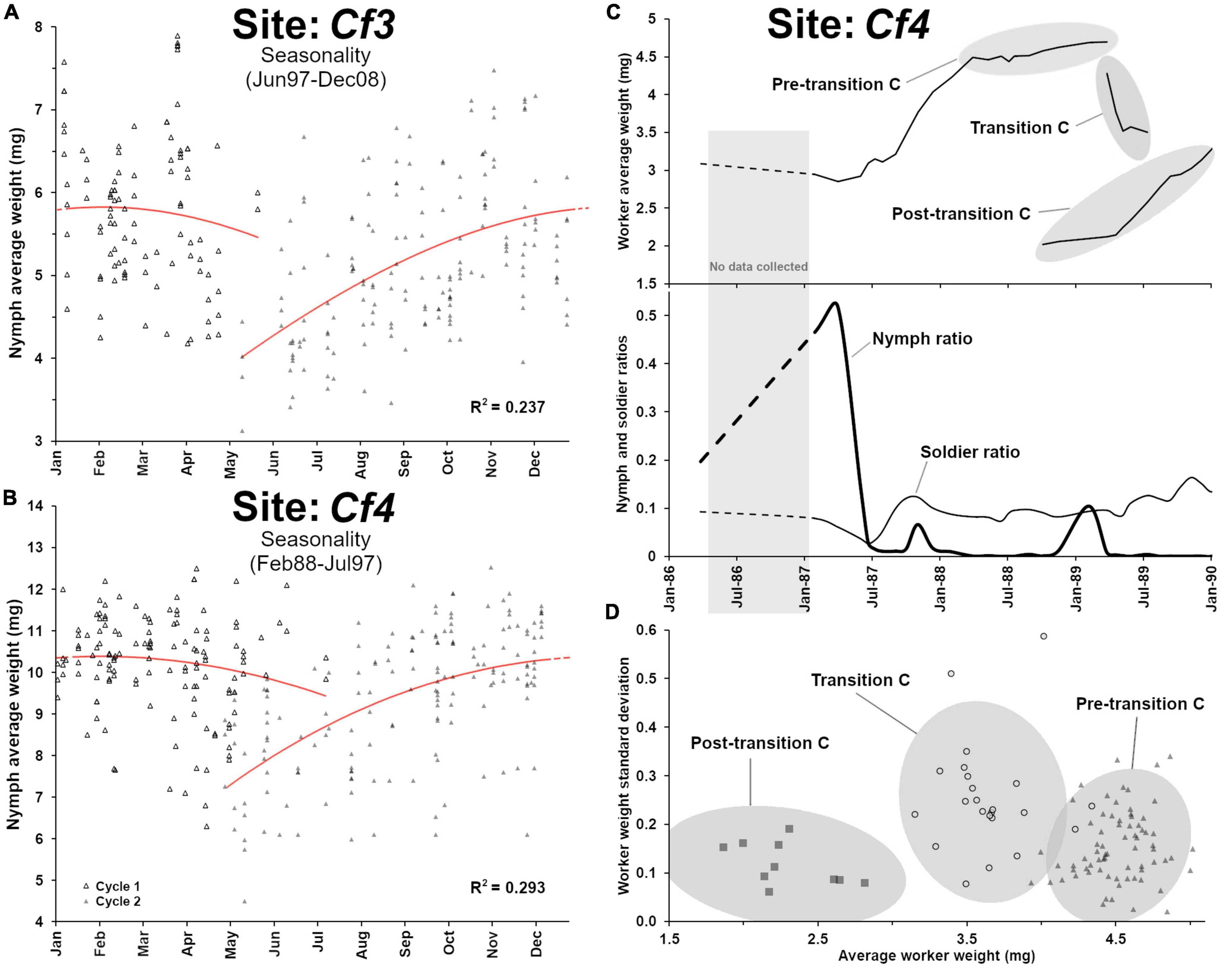
Figure 6. Additional demographic analyses from site Cf3 and site Cf4. (A) Nymphs’ average weight seasonality from Cf3, with an annual growth that follows a polynomial function (poly3, F3,263 = 27.3, p < 0.001, R2 = 0.237). (B) Nymphs’ average weight seasonality Cf4, with an annual growth that follows a polynomial function (poly3, F3,302 = 41.8, p < 0.001, R2 = 0.293). (C) Details of the demographic “transition C” from Cf4, showing a high nymph ratio event prior to the senescence of the colony (pre-transition C). (D) Weight heterogeneity among worker groups (with 95% ellipses) during transition C, (heterogeneity = 0.25 ± 0.11a) was higher than pre-transition C (0.15 ± 0.07b) and post-transition C (0.11 ± 0.05b) (ANOVA, Tukey post hoc, α = 0.05), suggesting a temporary colony fusion process with a young neighboring colony, during the colony senescence of the initial colony at site Cf4.
Site Cf4, a Succession of Complex Demographic Shifts
This last site was observed for the longest period of time (1986–2009) and displayed the most complex demographic changes when compared with the three previous sites, with a potential succession of established colonies over time. However, the information obtained from Cf1, Cf2, and Cf3 sites provided us with some key elements that guided our interpretation of the demographic changes over time at the Cf4 site (Figure 3D, Figure 7). The first obvious observation is that the C. formosanus population at this site went through two major demographic transitions, one around 1989 (referred as “transition C”) and another one around 2002 (referred as “transition D”). As explained below, site Cf4 may actually reflect the succession of two distinct colonies and one dramatic breeding structure shift over the decades. Worker age demography in 1986 appeared to be relatively young but a rapid accumulation of old workers occurred in 1988 (Figure 7A), with a similar trend for soldiers (Figure 7B). Remarkably, the colony produced a large number of small nymphs in 1987 (Figures 6C, 7C) with an average of more than 50% of nymphs in traps. This event happened just prior the rapid accumulation of old workers. Out of the 15 foraging traps collected between January and April 1987, 12 of them had > 40% nymphs, and 4 among these traps had > 90% of nymphs. This aberrant nymph ratio was never observed again at any point in time across all four sites. The large accumulation of nymphs and the rapid aging of the worker cohort both suggests that colony suddenly lost its reproductive ability and that most individuals were getting ready to “jump the ship” with most (if not all) larvae differentiating into nymphs instead of workers and soldiers. Such ‘last swarm’ strategy was not previously reported in Coptotermes (Osbrink et al., 2016; Chouvenc, 2020) but was observed in other lower termite species when resources are exhausted, or when reproduction is terminated, as most competent individuals turned into alates in a last fitness boost attempt (Gulmahamad, 2002; Korb and Lenz, 2004). However, out of season dispersal flight events of Coptotermes have been reported for colonies in the process of being baited by a CSI formulation (Su, 1994), as a possible analogous last-ditch effort to disperse one last time. In the case of Cf4, as most larvae engaged in nymph differentiation in early 1987, it directly competed with the renewal of the worker and soldier populations, contributing to the rapid to aging cohorts of the sterile castes. No secondary reproductives were found between 1987 and 1989, supporting a complete absence of reproduction at this point.
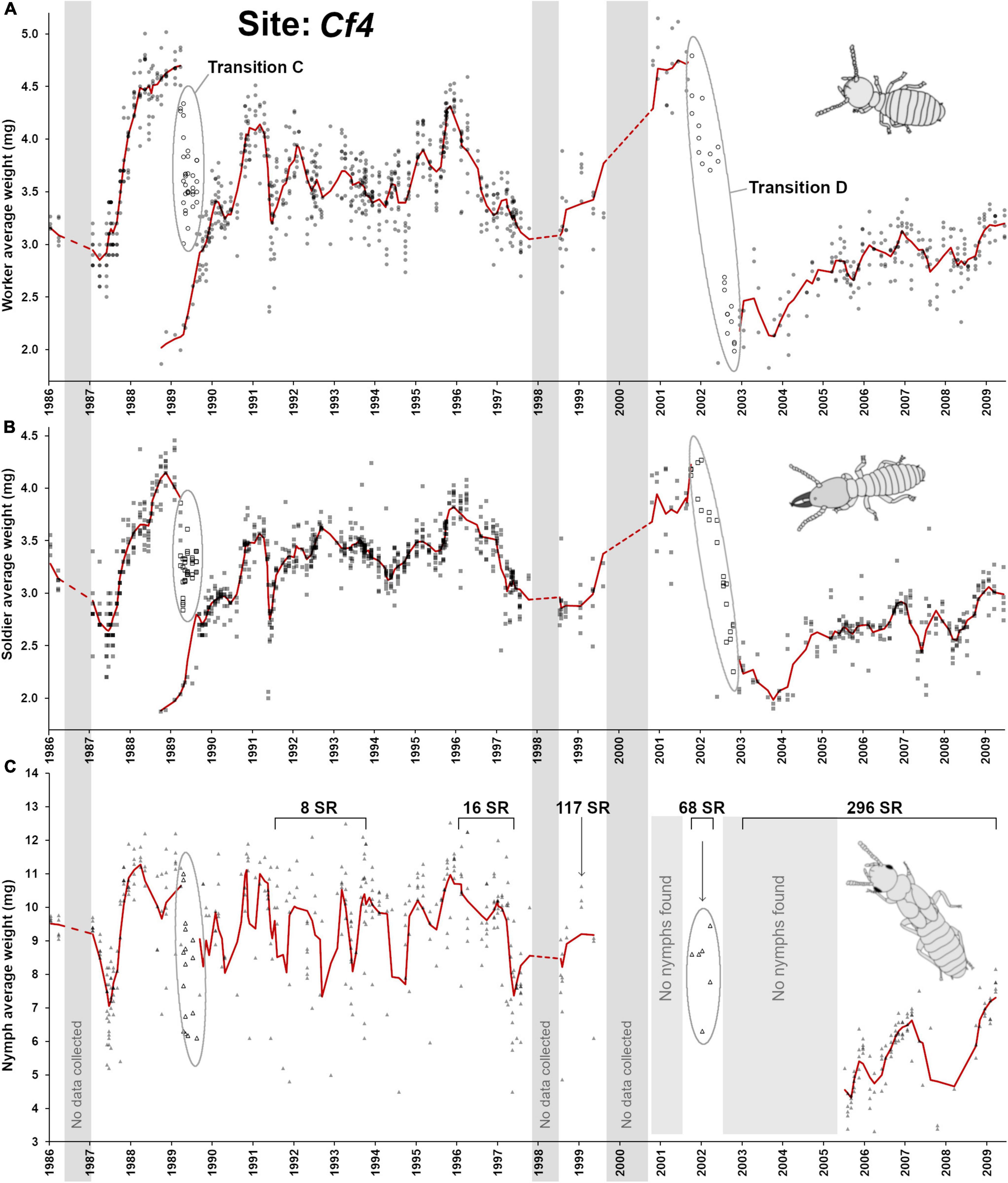
Figure 7. Colony demographic changes for Coptotermes formosanus at site Cf4. Each data point represents an average caste weight from a field trap. For the 1986–2009 demographic trends of each caste, the red line represents the rolling average function (period = 3). (A) Workers’ average weight. (B) Soldiers’ average weight. (C) Nymphs’ average weight. “Transition C” represents colony senescence and simultaneous colony fusion with a young neighboring colony. “Transition D” represents a generational demographic turnover followed by putative cycles of inbreeding associated with secondary reproductives = SR), and a temporary absence of nymph production prior to colony “re-maturation” (2003–2005).
While the 1987–1989 cascading demographic events should have led to the final senescence of the colony by 1990, as observed in Cf1, something else happened for Cf4 in 1988 that prevented such observation. In late 1988, a single trap at site Cf4 suddenly switched workers and soldiers demography, from ∼ 4.5 mg individuals to ∼ 2.0 mg individuals, between two consecutive sampling events (Figure 6C), confirming that a neighboring colony with a relatively young demography took over this foraging trap. Then, during the 1989 transition C event, the majority of foraging traps temporarily displayed highly heterogenous worker age groups (Figure 6D), implying that the two colonies were in the process of fusion (Su and Scheffrahn, 1988b), with a mix of old and young workers, leading to an intermediate average worker weight with a relatively high standard deviation within each trap. This mixed population was temporary, as all workers and soldiers from the initial colony eventually died of old age, leaving all traps with a uniformly “young” population during the post-transition C from the newly established colony (Figure 6D, ANOVA, post hoc α = 0.05). Therefore, the transition C event provides a remarkable example of a weakening senescent colony that was simultaneously being taken over by a relatively young neighboring colony (Husseneder et al., 2005; Messenger and Su, 2005; Messenger et al., 2005; Lee and Su, 2011; Bernard et al., 2017), which involved a temporary colony take-over process (Lee et al., 2019).
From 1990 to 1996, the newly established colony went through various cycles of aging/rejuvenating cohorts of workers and soldiers (Figures 7A,B), similar to what was observed at previous sites, with a cyclical production of nymphs (Figure 6B, poly3, F3,302 = 41.8, p < 0.001, R2 = 0.293). However, although some nymphs were found in 1989-1991 in the newly established colony, their numbers were very few when found (often < 10 nymphs per trap at best, with most traps displaying no nymphs). The number of nymphs found then increased substantially starting in 1992. The initial small weight of workers and the reduced number of nymphs therefore confirmed that the colony was relatively young and in the process of maturing (Chouvenc and Su, 2014) during and after transition C. Between 1990–1995 only 8 secondary reproductives were haphazardly collected, putatively as supplementary reproduction to the primary queen and king. However, a demographic shift occurred after a rise of the workers’ weight in 1995 suggesting the potential loss of the primary reproductives, or a long pause in oviposition. Supporting such interpretation is the accumulation of secondary reproductives in 1996 (16 collected that year), which resulted in a drop of the weight of soldiers and workers for two consecutive years (until 1998), as oviposition resumed.
The succession of events during 1998–2000 is unclear because of fragmentary sampling. However, key elements allow us to propose a solution to this puzzle. First, the weight of soldiers and workers increased continuously between 1998 to 2001, indicating a potential colony senescence. Second, there was a complete absence of nymphs between 1999 and 2004 also supporting ongoing senescence of the colony. Finally, 117 secondary reproductives were collected in a single trap in late 1998, and 68 secondary reproductives in early 2002, potentially indicating an attempt to resume oviposition. Interestingly, a few nymphs (<200 total) were produced and found in traps in early 2002 (transition D), at the same time as the 68 secondary reproductives, confirming that at least a small egg production occurred in the previous year and the oviposition resumption attempt was not a complete failure. Although a de- novo nymph production was proposed (Raina et al., 2004), i.e., workers differentiating into tertiary reproductives (Thorne, 1996), this scenario remains unlikely in Coptotermes (Lenz et al., 1988; Myles, 1999; Chouvenc and Su, 2014). With transition D, oviposition restarted, which resulted in the rapid rejuvenation of the worker pool, as old workers also died of senescence. However, the small worker/soldier weight and the lack of nymphs from 2003 to 2005 could instead imply that transition D reflected the taking over by a new juvenile neighboring colony, as observed in transition C, which would then finally mature with the first production of small nymphs toward alates for the 2006 season. Several elements however indicate that this alternative interpretation is unlikely. First, during 2002–2009, 296 additional secondary reproductives were found throughout this period, including in 2003–2005 when no nymph could be found. The development of functional secondary reproductives is often not possible in young Coptotermes colonies (Lenz and Runko, 1993; Costa-Leonardo et al., 2004; Chouvenc et al., 2015b). Second, just like we found in Cf3, the weight of secondary reproductives dropped dramatically after the transition D, from an of average 5.45 ± 1.46 mg, n = 24, to an average of 3.38 ± 0.80 mg, n = 77 (t = 8.91, df = 99, p < 0.001). Third, contrary to the newly established colony after transition C, which rapidly accumulated workers and soldiers between 3.5 to 4.5 mg (in < 2 years), sterile castes after transition D remained relatively small (2.0–3.0 mg) for > 6 years of observation. Fourth, nymphs were particularly small after transition D, with a shift from 9.29 ± 1.86 mg, n = 418, to an average of 5.65 ± 0.1.25 mg, n = 101 (t = 18.69, df = 517, p < 0.001). Finally, while wood consumption and the number of termites collected were not analyzed in this study because of the high variability over time and because it will be the focus of a future study, wood consumption rate at Cf4 changed notably after transition D, from 5.92 ± 3.12 g/d/trap, n = 579, to 2.78 ± 1.37 g/d/trap, n = 222 after the transition (t = 14.49, df = 799, p < 0.001), suggesting a potential reduced efficiency in wood foraging. All of these combined observations support that the colony was undergoing cycles of inbreeding (Husseneder et al., 2005) from secondary reproductives that accumulated since the early 2000s, as we previously concluded for site Cf3 after transition B. For Cf4, transition D resulted in a colony that most likely lost a large portion of its population, with a 2002 temporary senescence. Then, a delayed resumption of reproduction occurred through cycles of inbreeding. Without a critical mass of individuals, we here suggest that it temporarily lost its ability to produce nymphs (2003–2005) as the colony lost its “mature” status by temporarily displaying the profile of a juvenile colony (Chouvenc and Su, 2014), which it then regained in 2006 as the colony grew back to a critical mass of individuals and started producing small nymphs and alates again ( = colony “re-maturation”), until 2009 when the monitoring ended.
Conclusion
A subterranean termite colony is a complex biological entity that must thrive through various challenges once it has reached maturity (production of alates). Oster and Wilson (1978) provided a theoretical framework on how eusocial colonies may achieve degrees of perennity once they have completed their ergonomic growth and reached maturity, by investing differentially in different castes over time and adjusting colony functions depending on colony internal and external conditions over many years of activity. However, the current study indicates that colonies may go through dramatic demographic changes and breeding structure shifts, even many years after they have reached maturity, to maintain perennity and maximize fitness output. In a way, throughout its life, a colony displays its own demographic individuality, as the life of a colony is marked by internal and external events that drives its growth, its foraging ability, its competitiveness, its age demography composition, its senescence and ultimately, its death.
In this study, the single colony at site Cf1 reflected a simple perennial trajectory, as the colony sustained annual production of alates for at least a decade and went through cyclical seasonal variations of worker, soldier and nymph age structures. Eventually it lost reproductive abilities, and entered senescence with the accumulation of old individuals, lost colony essential functions, and ultimately died within 3 years. Site Cf2 introduced the concept of a temporary senescence within the life of a termite colony, as oviposition may be temporarily halted or highly reduced, which could have led to colony death. However, secondary reproductives were successfully produced which resulted in a rejuvenation of the population, phasing out old cohorts of sterile helpers, while resuming nymph production. Site Cf3 then showed that a subterranean colony can maintain its foraging activity and alate production for more than two decades. It also showed that the large accumulation of secondary reproductives, after the putative loss of primary reproduction and temporary senescence, can lead to rapid colony rejuvenation in conjunction with extensive cycles of colony inbreeding, resulting in the production of relatively small individuals across castes. Finally, site Cf4 displayed the most complex and dramatic colony demographic shifts, which involved a massive nymph production prior to a rapid colony senescence, in conjunction with a young neighboring colony take-over. The new colony then went through a decade-long perennial productivity before losing reproduction, which led to a critical attempt to resume oviposition with many secondary reproductives, almost fail to do so, but eventually managed to survive through cycles of inbreeding after losing a critical mass of old individuals. It temporarily lost its maturity status for a few years by demographically behaving like a juvenile colony, then eventually resumed nymph production toward a return to a perennial mature colony functioning. This study also provides new insights on the colony senescence process in subterranean termite field colonies. Colony senescence is not always straightforward from a demographic perspective and this study provides diverse scenarios where final colony collapse and death may sometimes be avoided by establishing secondary reproduction, which depending on the internal context, can be a chaotic transition and may not always be successful. Alternatively, colony senescence and collapse can be accelerated when neighboring colonies take over the foraging territory of the dying colony.
Regardless of the demographic changes and reproductive outcomes over the many years of the life of a mature colony, this study highlights that each colony may attempt different strategy to ultimately optimize its fitness. Cf1 went through a quiet death after many years of alate production. Cf2 was able to establish a stable colony productivity after a temporary loss of reproduction. Cf3 avoided colony senescence by massively producing secondary reproductives and imposing cycles of inbreeding, which still resulted in a large production of alates that would disperse and engage in sexual reproduction, potentially negating the temporary effects of inbreeding. Finally, on Cf4, the initial colony went through an alate production hail Mary, the second established colony took over and engaged in a perennial reproductivity, then narrowly avoided colony collapse by finally establishing secondary reproduction successfully.
To conclude, this study provides novel elements on how subterranean colonies function over time and revealed that each colony demographic trajectory is unique through their perennity. It also showed that a single sampling event of a colony only provides limited information, which lack the complex historical context of what was the demographic status before and after such single data snapshot. The dataset used in this study allowed us to reframe such demographic context over decades of observation. Yet, this analysis represents a fraction of the comprehensive dataset collected by PMB, which remains to be further curated and analyzed in future studies for a set of specific questions. Finally, while we acknowledge the inherent speculative nature of data interpretation in this study, owing from the uniqueness of the dataset, all interpretations were carefully considered and deemed as the most likely scenarios, when taking all of the available evidence. However, we encourage future researchers that may be skeptical of such interpretations, to subject them to further testing, and confirm or refute them. Regardless, we hope this study can be used, either way, as a building block toward a better understanding of social insect colony demographic complexity.
Data Availability Statement
The raw data supporting the conclusions of this article will be made available by the authors, without undue reservation.
Author Contributions
N-YS initiated the field plots and established the initial termite collection protocol. PMB coordinated the data collection. TC performed the data curation, data analysis, figure production, and first manuscript writing. All authors read and approved the final manuscript, except PMB, whose co-author status is a tribute to his essential contribution to the field of termite research.
Funding
This study was partially funded by USDA-NIFA Hatch Project No. FLA-FTL-005660 and FLA-FLT-005865, and by the Florida Department of Agriculture and Consumer Services under the project No. 026398. However, the data acquisition process was also directly and indirectly partially supported by virtually all funding acquired from the N-YS laboratory between 1986 and 2009.
Conflict of Interest
The authors declare that the research was conducted in the absence of any commercial or financial relationships that could be construed as a potential conflict of interest.
Publisher’s Note
All claims expressed in this article are solely those of the authors and do not necessarily represent those of their affiliated organizations, or those of the publisher, the editors and the reviewers. Any product that may be evaluated in this article, or claim that may be made by its manufacturer, is not guaranteed or endorsed by the publisher.
Acknowledgments
This manuscript is fully dedicated to the memory of PMB (1956–2009), who spearheaded and coordinated the termite collection process and the record keeping effort for more than two decades at the University of Florida termite laboratory. An unknown number of temporary technicians were involved in the data collection process, whose name were unfortunately not all found in the records. We thank them all nonetheless for their contribution. We thank Angelica Moncada, Stephanie Osorio, and Hou-Feng Li for their patience in contributing to the digitalization process of this massive dataset, Sang-Bin Lee and Aaron Mullins for their constructive feedback on early versions of this manuscript. Finally, we also thank the 8,842,143 termites reported in the current study, collected throughout this multidecade effort and contributed by dying in experiments which resulted in countless studies, many of which were essential for us to interpret this challenging dataset.
References
Albino, E., and Costa-Leonardo, A. M. (2011). Nymphs in foraging populations of Coptotermes gestroi (Isoptera: Rhinotermitidae). Anim. Biol. 61, 427–439. doi: 10.1163/157075511X596909
Arab, A., Costa-Leonardo, A. M., Casarin, F. E., de Camargo Guaraldo, A., and Chaves, R. C. (2005). Foraging activity and demographic patterns of two termite species (Isoptera: Rhinotermitidae) living in urban landscapes in southeastern Brazil. Eur. J. Entomol. 102, 691–697. doi: 10.14411/eje.2005.098
Bernadou, A., Kramer, B. H., and Korb, J. (2021). Major evolutionary transitions in social insects, the importance of worker sterility and life history trade-offs. Front. Ecol. Evol. 9:732907. doi: 10.3389/fevo.2021.732907
Bernard, S., Osbrink, W., and Su, N. Y. (2017). Response of the Formosan subterranean termite to neighboring con-specific populations after baiting with noviflumuron. J. Econ. Entomol. 110, 575–583. doi: 10.1093/jee/tow317
Bodot, P. (1969). Composition des colonies de termites: ses fluctuations au cours du temps. Insectes Soc. 16, 39–53. doi: 10.1007/BF02224461
Boomsma, J. J., and Gawne, R. (2018). Superorganismality and caste differentiation as points of no return: how the major evolutionary transitions were lost in translation. Biol. Rev. 93, 28–54. doi: 10.1111/brv.12330
Bourke, A. F., and Ratnieks, F. L. (1999). Kin conflict over caste determination in social Hymenoptera. Behav. Ecol. Sociobiol. 46, 287–297. doi: 10.1007/s002650050622
Chouvenc, T. (2018). Comparative impact of chitin synthesis inhibitor baits and non-repellent liquid termiticides on subterranean termite colonies over foraging distances: colony elimination versus localized termite exclusion. J. Econ. Entomol. 111, 2317–2328. doi: 10.1093/jee/toy210
Chouvenc, T. (2019). The relative importance of queen and king initial weights in termite colony foundation success. Insectes Soc. 66, 177–184. doi: 10.1007/s00040-019-00690-3
Chouvenc, T. (2020). Limited survival strategy in starving subterranean termite colonies. Insectes Soc. 67, 71–82. doi: 10.1007/s00040-019-00729-5
Chouvenc, T. (2022). Not enough bugs, too many bugs—Scaling up. Am. Entomol. 68, 32–33. doi: 10.1093/ae/tmac015
Chouvenc, T., and Lee, S. B. (2021). Queen egg laying and egg hatching abilities are hindered in subterranean termite colonies when exposed to a chitin synthesis inhibitor bait formulation. J. Econ. Entomol. 114, 2466–2472. doi: 10.1093/jee/toab200
Chouvenc, T., and Su, N. Y. (2014). Colony age-dependent pathway in caste development of Coptotermes formosanus Shiraki. Insectes Soc. 61, 171–182. doi: 10.1007/s00040-014-0343-9
Chouvenc, T., Mullins, A. J., Efstathion, C. A., and Su, N. Y. (2013a). Virus-like symptoms in a termite (isoptera: Kalotermitidae) field colony. Florida Entomol. 96, 1612–1614. doi: 10.1653/024.096.0450
Chouvenc, T., Bardunias, P., Efstathion, C. A., Chakrabarti, S., Elliott, M. L., Giblin-Davis, R., et al. (2013b). Resource opportunities from the nest of dying subterranean termite (Isoptera: Rhinotermitidae) colonies: a laboratory case of ecological succession. Ann. Entomol. Soc. Am. 106, 771–778. doi: 10.1603/AN13104
Chouvenc, T., Bardunias, P., Li, H. F., Elliott, M. L., and Su, N. Y. (2011). Planar arenas for use in laboratory bioassay studies of subterranean termites (Rhinotermitidae). Florida Entomol. 94, 817–826. doi: 10.1653/024.094.0413
Chouvenc, T., Basille, M., and Su, N. Y. (2015a). The production of soldiers and the maintenance of caste proportions delay the growth of termite incipient colonies. Insectes Soc. 62, 23–29. doi: 10.1007/s00040-014-0369-z
Chouvenc, T., Li, H. F., Austin, J., Bordereau, C., and Bourguignon, et al. (2016). Revisiting Coptotermes (Isoptera: Rhinotermitidae): a global taxonomic road map for species validity and distribution of an economically important subterranean termite genus. Syst. Entomol. 41, 299–306. doi: 10.1111/syen.12157
Chouvenc, T., Mullins, A. J., and Su, N. Y. (2015b). Rare production of nymphs in an Asian subterranean termite (Isoptera: Rhinotermitidae) incipient colony. Florida Entomol. 98, 972–973. doi: 10.1653/024.098.0327
Chouvenc, T., Scheffrahn, R. H., Mullins, A. J., and Su, N. Y. (2017). Flight phenology of two Coptotermes species (Isoptera: Rhinotermitidae) in southeastern Florida. J. Econ. Entomol. 110, 1693–1704. doi: 10.1093/jee/tox136
Chouvenc, T., Šobotník, J., Engel, M. S., and Bourguignon, T. (2021). Termite evolution: mutualistic associations, key innovations, and the rise of Termitidae. Cell. Mol. Life Sci. 78, 2749–2769. doi: 10.1007/s00018-020-03728-z
Collins, N. M. (1981). Populations, age structure and survivorship of colonies of Macrotermes bellicosus (Isoptera: Macrotermitinae). J. Anim. Ecol. 50, 293–311. doi: 10.2307/4046
Cook, S. C., Eubanks, M. D., Gold, R. E., and Behmer, S. T. (2011). Seasonality directs contrasting food collection behavior and nutrient regulation strategies in ants. PLoS One 6:e25407. doi: 10.1371/journal.pone.0025407
Costa-Leonardo, A. M., Arab, A., and Casarin, F. E. (2004). Neotenic formation in laboratory colonies of the termite Coptotermes gestroi after orphaning. J. Insect Sci. 4:10. doi: 10.1093/jis/4.1.10
Costa-Leonardo, A., Janei, V., and Da Silva, I. (2022). Comparative reproductive biology of pre-, imaginal, and neotenic castes of the Asian termite Coptotermes gestroi (Blattaria, Isoptera, Rhinotermitidae). Bull. Entomol. Res. 20, 1–10. doi: 10.1017/S0007485322000232
Cronin, A. L., Molet, M., Doums, C., Monnin, T., and Peeters, C. (2013). Recurrent evolution of dependent colony foundation across eusocial insects. Annu. Rev. Entomol. 58, 37–55. doi: 10.1146/annurev-ento-120811-153643
Crossland, M. W., and Su, N. Y. (2006). Work allocation among castes in a Rhinotermitid termite (Isoptera) – Are nymphs a working caste? Sociobiology 48, 585–598.
Dainat, B., Evans, J. D., Chen, Y. P., Gauthier, L., and Neumann, P. (2012). Predictive markers of honey bee colony collapse. PLoS One 7:e32151. doi: 10.1371/journal.pone.0032151
Darlington, J. P. E. C. (1984). A method for sampling the populations of large termite nests. Ann. Appl. Biol. 104, 427–436. doi: 10.1111/j.1744-7348.1984.tb03024.x
Darlington, J. P. E. C. (1991). Turnover in the populations within mature nests of the termite Macrotermes michaelseni in Kenya. Insectes Soc. 38, 251–262. doi: 10.1007/BF01314911
Darlington, J. P. E. C., and Dransfield, R. D. (1987). Size relationships in nest populations and mound parameters in the termite Macrotermes michaelseni in Kenya. Insectes Soc. 34, 165–180. doi: 10.1007/BF02224082
de Verge, J., and Nehring, V. (2016). A critical look at proximate causes of social insect senescence: damage accumulation or hyperfunction? Curr. Op. Insect Sci. 16, 69–75. doi: 10.1016/j.cois.2016.05.003
Dornhaus, A., Powell, S., and Bengston, S. (2012). Group size and its effects on collective organization. Ann. Rev. Entomol. 57, 123–141. doi: 10.1146/annurev-ento-120710-100604
Du, H., Chouvenc, T., Osbrink, W. L. A., and Su, N. Y. (2016). Social interactions in the central nest of Coptotermes formosanus juvenile colonies. Insectes Soc. 63, 279–290. doi: 10.1007/s00040-016-0464-4
Du, H., Chouvenc, T., Osbrink, W. L. A., and Su, N. Y. (2017). Heterogeneous distribution of castes/instars and behaviors in the nest of Coptotermes formosanus Shiraki. Insectes Soc. 64, 103–112. doi: 10.1007/s00040-016-0520-0
Eggleton, P. (2020). The state of the world’s insects. Ann. Rev. Environ. Resourc. 45, 61–82. doi: 10.1146/annurev-environ-012420-050035
Ellis, J. D., Evans, J. D., and Pettis, J. (2010). Colony losses, managed colony population decline, and Colony Collapse Disorder in the United States. J. Api. Res. 49, 134–136. doi: 10.3896/IBRA.1.49.1.30
Emerson, A. E. (1939). Social coordination and the superorganism. Am. Midland Nat. 21, 182–209. doi: 10.2307/2420380
Evans, J. D., and Chen, Y. (2021). “Colony collapse disorder and honey bee health,” in Honey Bee Medicine for the Veterinary Practitioner, eds T. R. Kane and C. M. Faux (Hoboken:John Wiley & Sons, Inc), 229–234. doi: 10.1002/9781119583417.ch19
Evans, T. A. (2021). Predicting ecological impacts of invasive termites. Curr. Op. Insect Sci. 46, 88–94. doi: 10.1016/j.cois.2021.03.003
Evans, T. A., Lenz, M., and Gleeson, P. V. (1998). Testing assumptions of mark–recapture protocols for estimating population size using Australian mound-building, subterranean termites. Ecol. Entomol. 23, 139–159. doi: 10.1046/j.1365-2311.1998.00114.x
Eyer, P. A., and Vargo, E. L. (2021). Breeding structure and invasiveness in social insects. Curr. Op. Insect Sci. 46, 24–30. doi: 10.1016/j.cois.2021.01.004
Fewell, J. H., and Harrison, J. F. (2016). Scaling of work and energy use in social insect colonies. Behav. Ecol. Sociobiol. 70, 1047–1061. doi: 10.1007/s00265-016-2097-z
Giraldo, Y. M., and Traniello, J. F. (2014). Worker senescence and the sociobiology of aging in ants. Behav. Ecol. Sociobiol. 68, 1901–1919. doi: 10.1007/s00265-014-1826-4
Giraldo, Y. M., Muscedere, M. L., and Traniello, J. F. (2021). Eusociality and senescence: neuroprotection and physiological resilience to aging in insect and mammalian systems. Front. Cell Dev. Biol. 9:673172. doi: 10.3389/fcell.2021.673172
Goldstein, J. R. (2009). “How populations age,” in International handbook of population aging, ed. P. Uhlenberg (Dordrecht: Springer), 7–18.
Gordon, J. M., Velenovsky, J. F., and Chouvenc, T. (2022). Subterranean termite colony elimination can be achieved even when only a small proportion of foragers feed upon a CSI bait. J. Pest Sci. 95, 1207–1216. doi: 10.1007/s10340-021-01446-4
Grace, J. K., Yamamoto, R. T., and Tamashiro, M. (1995). Relationship of individual worker mass and population decline in a Formosan subterranean termite colony (Isoptera: Rhinotermitidae). Environ. Entomol. 24, 1258–1262. doi: 10.1093/ee/24.5.1258
Gulmahamad, H. (2002). Survival strategy of a western drywood termite colony, Incisitermes minor, (Isoptera: Kalotermitidae) established in a limited food source. Sociobiology 40, 595–604.
Haagsma, K. A., and Rust, M. K. (1995). Colony size estimates, foraging trends, and physiological characteristics of the western subterranean termite (Isoptera: Rhinotermitidae). Environ. Entomol. 24, 1520–1528. doi: 10.1093/ee/24.6.1520
Harrison, M. C., Jongepier, E., Robertson, H. M., Arning, N., Bitard-Feildel, T., et al. (2018). Hemimetabolous genomes reveal molecular basis of termite eusociality. Nature Ecol. Evol. 2, 557–566. doi: 10.1038/s41559-017-0459-1
Heinze, J., and Keller, L. (2000). Alternative reproductive strategies: a queen perspective in ants. Trends Ecol. Evol. 15, 508–512. doi: 10.1016/S0169-5347(00)01995-9
Higa, S. Y. (1981). Flight, Colony Foundation, and Development of the Gonads of the Primary Reproductives of the Formosan Subterranean Termite, Coptotermes Formosanus Shiraki. [Ph.D. thesis] Honolulu: University of Hawaii.
Hölldobler, B., and Wilson, E. O. (2009). The superorganism: the beauty, elegance, and strangeness of insect societies. WW Norton & Company.
Hou, C., Kaspari, M., Vander Zanden, H. B., and Gillooly, J. F. (2010). Energetic basis of colonial living in social insects. Proc. Nat. Acad. Sci. 107, 3634–3638. doi: 10.1073/pnas.0908071107
Houston, A., Schmid-Hempel, P., and Kacelnik, A. (1988). Foraging strategy, worker mortality, and the growth of the colony in social insects. Am. Nat. 131, 107–114. doi: 10.1086/284776
Howard, K. J., and Thorne, B. L. (2010). “Eusocial evolution in termites and Hymenoptera,” in Biology of termites: a modern synthesis, eds D. E. Bignell, Y. Roisin, and N. Lo (Dordrecht: Springer), 97–132.
Husseneder, C., Messenger, M. T., Su, N. Y., Grace, J. K., and Vargo, E. L. (2005). Colony social organization and population genetic structure of an introduced population of Formosan subterranean termite from New Orleans. Louisiana. J. Econ. Entomol. 98, 1421–1434. doi: 10.1093/jee/98.5.1421
Husseneder, C., Simms, D. M., Delatte, J. R., Wang, C., Grace, J. K., and Vargo, E. L. (2012). Genetic diversity and colony breeding structure in native and introduced ranges of the Formosan subterranean termite. Coptotermes formosanus. Biol. Invas. 14, 419–437. doi: 10.1007/s10530-011-0087-7
Kakkar, G., Osbrink, W., and Su, N. Y. (2018). Molting site fidelity accounts for colony elimination of the Formosan subterranean termites (Isoptera: Rhinotermitidae) by chitin synthesis inhibitor baits. Sci. Rep. 8:1259. doi: 10.1038/s41598-018-19603-8
Kakkar, G., Osbrink, W., Mullins, A., and Su, N. Y. (2017). Molting site fidelity in workers of Formosan subterranean termites (Isoptera: Rhinotermitidae). J. Econ. Entomol. 110, 2512–2517. doi: 10.1093/jee/tox246
Kaspari, M., and Vargo, E. L. (1995). Colony size as a buffer against seasonality: Bergmann’s rule in social insects. Am. Nat. 145, 610–632. doi: 10.1086/285758
Keller, L., and Genoud, M. (1997). Extraordinary lifespans in ants: a test of evolutionary theories of ageing. Nature 389, 958–960. doi: 10.1038/40130
King, E. G. Jr., and Spink, W. T. (1969). Foraging galleries of the Formosan subterranean termite, Coptotermes formosanus, in Louisiana. Ann. Entomol. Soc. Am. 62, 536–542. doi: 10.1093/aesa/62.3.536
Koehler, P. G. (1980). The Formosan Subterranean Termite. Florida: Florida Cooperative Extension Service.
Korb, J., and Lenz, M. (2004). Reproductive decision-making in the termite, Cryptotermes secundus (Kalotermitidae), under variable food conditions. Behav. Ecol. 15, 390–395. doi: 10.1093/beheco/arh033
Kramer, B. H., and Schaible, R. (2013). Colony size explains the lifespan differences between queens and workers in eusocial Hymenoptera. Biol. J. Linn. Soc. 109, 710–724. doi: 10.1111/bij.12072
Kramer, B. H., Nehring, V., Buttstedt, A., Heinze, J., Korb, J., Libbrecht, R., et al. (2021). Oxidative stress and senescence in social insects: a significant but inconsistent link? Phil. Trans. Roy. Soc. B 376:20190732. doi: 10.1098/rstb.2019.0732
Lax, A. R., and Osbrink, W. L. A. (2003). United States Department of Agriculture—agriculture research service research on targeted management of the Formosan subterranean termite Coptotermes formosanus Shiraki (Isoptera: Rhinotermitidae). Pest Manag. Sci. 59, 788–800. doi: 10.1002/ps.721
Lee, S. B., Chouvenc, T., and Su, N. Y. (2021). Differential time allocation of foraging workers in the subterranean termite. Front. Zool. 18:61. doi: 10.1186/s12983-021-00446-5
Lee, S. B., Chouvenc, T., Mizumoto, N., Mullins, A., and Su, N. Y. (2022). Age-based spatial distribution of workers is resilient to worker loss in a subterranean termite. Sci. Rep. 12:7837. doi: 10.1038/s41598-022-11512-1
Lee, S. B., Mullins, A., Aguilera-Olivares, D., Chouvenc, T., and Su, N. Y. (2019). Fused colonies of the Formosan subterranean termite (Blattodea: Rhinotermitidae) for laboratory experiments. J. Econ. Entomol. 112, 2311–2315. doi: 10.1093/jee/toz154
Lee, S. H., and Su, N. Y. (2011). Territory size distribution of Formosan subterranean termites in urban landscape: Comparison between experimental and simulated results. J. Asia-Pacific Entomol. 14, 1–6. doi: 10.1016/j.aspen.2010.11.002
Lemanski, N. J., and Fefferman, N. H. (2018). How life history shapes optimal patterns of senescence: implications from individuals to societies. Am. Nat. 191, 756–766. doi: 10.1086/697225
Lenz, M., and Runko, S. (1993). Long-term impact of orphaning on field colonies of Coptotermes lacteus (Froggatt) (Isoptera: Rhinotermitidae). Insectes Soc. 40, 439–456. doi: 10.1007/BF01253906
Lenz, M., Barrett, R. A., and Miller, L. R. (1988). Mechanisms of colony re-establishment after orphaning in Coptotermes lacteus (Froggatt) (Isoptera: Rhinotermitidae). Sociobiology 14, 245–248.
Lepage, M., and Darlington, J. P. (2000). “Population dynamics of termites,” in Termites: evolution, Sociality, Symbioses, Ecology, eds T. Abe, D. E. Bignell, and M. Higashi (Dordrecht: Springer), 333–361.
Liu, Y., Henderson, G., Mao, L., and Laine, R. A. (2005). Seasonal variation of juvenile hormone titers of the Formosan subterranean termite. Coptotermes formosanus (Rhinotermitidae). Environ. Entomol. 34, 557–562. doi: 10.1603/0046-225X-34.3.557
Messenger, M. T., and Su, N. Y. (2005). Agonistic behavior between colonies of the Formosan subterranean termite (Isoptera: Rhinotermitidae) from Louis Armstrong Park. New Orleans, Louisiana. Sociobiology 45, 331–346.
Messenger, M. T., Su, N. Y., Husseneder, C., and Grace, J. K. (2005). Elimination and reinvasion studies with Coptotermes formosanus (Isoptera: Rhinotermitidae) in Louisiana. J. Econ. Entomol. 98, 916–929. doi: 10.1603/0022-0493-98.3.916
Michener, C. D. (1964). Reproductive efficiency in relation to colony size in hymenopterous societies. Insectes Soc. 11, 317–341. doi: 10.1007/BF02227433
Moret, Y., and Schmid-Hempel, P. (2009). Immune responses of bumblebee workers as a function of individual and colony age: senescence versus plastic adjustment of the immune function. Oikos 118, 371–378. doi: 10.1111/j.1600-0706.2008.17187.x
Moritz, R., and Southwick, E. E. (2012). Bees as superorganisms: an evolutionary reality.Berlin :Springer Science & Business Media.
Mueller, U. G., Gerardo, N. M., Aanen, D. K., Six, D. L., and Schultz, T. R. (2005). The evolution of agriculture in insects. Annu. Rev. Ecol. Evol. Syst. 36, 563–595. doi: 10.1146/annurev.ecolsys.36.102003.152626
Myles, T. G. (1999). Review of secondary reproduction in termites (Insecta: Isoptera) with comments on its role in termite ecology and social evolution. Sociobiology 33, 1–43.
Nakajima, S., Shimizu, K., and Nakajima, Y. (1963). Analytical studies on the vitality of colonies of the Formosan termite, Coptotermes formosanus Shiraki: II. Seasonal fluctuations on the external characters of the workers, the ratio of caste-number and carbon dioxide in the nest of a colony. Miyazaki Univ. Fac. Agric. Bull. 9, 222–227.
Nalepa, C. A. (2015). Origin of termite eusociality: trophallaxis integrates the social, nutritional, and microbial environments. Ecol. Entomol. 40, 323–335. doi: 10.1111/een.12197
Naug, D. (2009). Structure and resilience of the social network in an insect colony as a function of colony size. Behav. Ecol. Sociobiol. 63, 1023–1028. doi: 10.1007/s00265-009-0721-x
Nutting, W. L. (1969). “Flight and colony foundation,” in Biology of Termites, 1, eds K. Krishna and F. M. Weesner (New York, NY: Academic Press), 233–282.
Osbrink, W. L. A., Cornelius, M. L., and Showler, A. T. (2016). Bionomics and formation of “Bonsai” colonies with long-term rearing of Coptotermes formosanus (Isoptera: Rhinotermitidae). J. Econ. Entomol. 109, 770–778. doi: 10.1093/jee/tov346
Oster, G. F., and Wilson, E. O. (1978). Caste and ecology in the social insects.princeton, NJ: Princeton University Press.
Park, Y. I., and Raina, A. (2003). Factors regulating caste differentiation in the Formosan subterranean termite with emphasis on soldier formation. Sociobiology 41, 49–60.
Porter, S. D., and Tschinkel, W. R. (1985). Fire ant polymorphism: the ergonomics of brood production. Behav. Ecol. Sociobiol. 16, 323–336. doi: 10.1007/BF00295545
Porter, S. D., and Tschinkel, W. R. (1986). Adaptive value of nanitic workers in newly founded red imported fire ant colonies (Hymenoptera: Formicidae). Ann. Entomol. Soc. Am. 79, 723–726. doi: 10.1093/aesa/79.4.723
Raina, A. K. (2006). “Some of the unique features of development and caste differentiation in the Formosan subterranean termite (Isoptera: Rhinotermitidae),” in Proceedings of 2006 IUSSI Congress, Washington, DC (San Diego, CA: International Union for the Study of Social Insects).
Raina, A., Osbrink, W., and Park, Y. I. (2004). Nymphs of the Formosan subterranean termite (Isoptera: Rhinotermitidae): aspects of formation and transformation. Ann. Entomol. Soc. Am. 97, 757–764. doi: 10.1603/0013-87462004097[0757:NOTFST]2.0.CO;2
Ramsay, C., Lasko, P., and Abouheif, E. (2021). “Evo-Devo lessons from the reproductive division of labor in eusocial Hymenoptera,” in Evolutionary Developmental Biology: A Reference Guide, (eds) L. N. de la Rosa, G. B. Müller. (Berlin:Springer International Publishing), 791–804.
R Core Team (2021). A Language and Environment for Statistical Computing. Vienna: R Foundation for Statistical Computing.
Rueppell, O., Bachelier, C., Fondrk, M. K., and Page, R. E. Jr. (2007). Regulation of life history determines lifespan of worker honey bees (Apis mellifera L.). Exp. Gerontol. 42, 1020–1032. doi: 10.1016/j.exger.2007.06.002
Rust, M. K., and Su, N. Y. (2012). Managing social insects of urban importance. Annu. Rev. Entomol. 57, 355–375.
Shik, J. Z., Hou, C., Kay, A., Kaspari, M., and Gillooly, J. F. (2012). Towards a general life-history model of the superorganism: predicting the survival, growth and reproduction of ant societies. Biol. Lett. 8, 1059–1062. doi: 10.1098/rsbl.2012.0463
Shimizu, K. (1962). Analytical studies on the vitality of colonies of the Formosan subterranean termite, Coptotermes formosanus Shiraki. I. Analysis of the strength of vitality. Bull. Fac. Agric. Univ. Miyazaki 8, 106–110.
Šobotník, J., Bourguignon, T., Hanus, R., Demianová, Z., Pytelková, J., et al. (2012). Explosive backpacks in old termite workers. Science 337, 436–436. doi: 10.1126/science.1219129
Su, N. Y. (1994). Field evaluation of a hexaflumuron bait for population suppression of subterranean termites (Isoptera: Rhinotermitidae). J. Econ. Entomol. 87, 389–397. doi: 10.1603/ec10217
Su, N. Y. (2001). Studies on the foraging of subterranean termites (Isoptera). Sociobiology 37, 253–260.
Su, N. Y. (2013). Estimating population size of large laboratory colonies of the Formosan subterranean termite using the capture probability equilibrium. J. Econ. Entomol. 106, 2442–2447. doi: 10.1603/EC13258
Su, N. Y. (2019). Development of baits for population management of subterranean termites. Annu. Rev. Entomol. 64, 115–130. doi: 10.1146/annurev-ento-011118-112429
Su, N. Y., and Scheffrahn, R. H. (1986). A method to access, trap, and monitor field populations of the Formosan subterranean termite (Isoptera: Rhinotermitidae). Sociobiology 12, 299–304.
Su, N. Y., and Scheffrahn, R. H. (1988a). Foraging population and territory of the Formosan subterranean termite (Isoptera: Rhinotermitidae) in an urban environment. Sociobiology 14, 353–360.
Su, N. Y., and Scheffrahn, R. H. (1988b). Intra- and inter-specific of the Formosan and Eastern subterranean termite: Evidence from Field observations. Sociobiology 14, 157–164.
Su, N. Y., and Scheffrahn, R. H. (1998). A review of subterranean termite control practices and prospects for integrated pest management programmes. Integr. Pest Manage. Rev. 3, 1–13. doi: 10.1023/A:1009684821954
Su, N. Y., and Scherer, P. N. (2003). Feeding site selection by workers of the Formosan subterranean termite Coptotermes formosanus (Isoptera: Rhinotermitidae)–a re-analysis of field data from a mark–recapture study. Bull. Entomol. Res. 93, 467–473. doi: 10.1079/BER2003253
Su, N. Y., Ban, P. M., and Scheffrahn, R. H. (1991). Suppression of foraging populations of the Formosan subterranean termite (Isoptera: Rhinotermitidae) by field applications of a slow-acting toxicant bait. J. Econ. Entomol. 84, 1525–1531. doi: 10.1093/jee/84.5.1525
Su, N. Y., Osbrink, W., Kakkar, G., Mullins, A., and Chouvenc, T. (2017). Foraging distance and population size of juvenile colonies of the Formosan subterranean termite (Isoptera: Rhinotermitidae) in laboratory extended arenas. J. Econ. Entomol. 110, 1728–1735. doi: 10.1093/jee/tox153
Tamashiro, M., Fujii, J. K., and Lai, P. Y. (1973). A simple method to observe, trap, and prepare large numbers of subterranean termites for laboratory and field experiments. Environ. Entomol. 2, 721–722. doi: 10.1093/ee/2.4.721
Thorne, B. L., Russek-Cohen, E., Forschler, B. T., Breisch, N. L., and Traniello, J. F. (1996). Evaluation of mark–release–recapture methods for estimating forager population size of subterranean termite (Isoptera: Rhinotermitidae) colonies. Environ. Entomol. 25, 938–951. doi: 10.1093/ee/25.5.938
Tschinkel, W. R. (1991). Insect sociometry, a field in search of data. Insectes Soc. 38, 77–82. doi: 10.1007/BF01242715
Tschinkel, W. R. (1998). Sociometry and sociogenesis of colonies of the harvester ant. Pogonomyrmex badius: worker characteristics in relation to colony size and season. Insectes Soc. 45, 385–410. doi: 10.1007/s000400050097
Tschinkel, W. R., Adams, E. S., and Macom, T. (1995). Territory area and colony size in the fire ant Solenopsis invicta. J. Ani. Ecol. 473–480. doi: 10.2307/5650
Vargo, E. L. (2019). Diversity of termite breeding systems. Insects 10:52. doi: 10.3390/insects10020052
Vargo, E. L., and Husseneder, C. (2009). Biology of subterranean termites: insights from molecular studies of Reticulitermes and Coptotermes. Annu. Rev. Entomol. 54, 379–403. doi: 10.1146/annurev.ento.54.110807.090443
Vargo, E. L., Husseneder, C., and Grace, J. K. (2003). Colony and population genetic structure of the Formosan subterranean termite, Coptotermes formosanus, in Japan. Mol. Ecol. 12, 2599–2608. doi: 10.1046/j.1365-294X.2003.01938.x
Waller, D. A., and La Fage, J. P. (1987a). Seasonal patterns in foraging groups of Coptotermes formosanus (Rhinotermitidae). Sociobiology 13, 173–181.
Waller, D. A., and La Fage, J. P. (1987b). Food quality and foraging response by the subterranean termite Coptotermes formosanus Shiraki (Isoptera: Rhinotermitidae). Bull. Entomol. Res. 77, 417–424. doi: 10.1017/S0007485300011883
Wheeler, W. M. (1911). The ant-colony as an organism. J. Morph. 22, 307–325. doi: 10.1002/jmor.1050220206
Keywords: Coptotermes formosanus, colony perennity, reproduction, colony senescence, succession
Citation: Chouvenc T, Ban PM and Su N-Y (2022) Life and Death of Termite Colonies, a Decades-Long Age Demography Perspective. Front. Ecol. Evol. 10:911042. doi: 10.3389/fevo.2022.911042
Received: 01 April 2022; Accepted: 13 June 2022;
Published: 05 July 2022.
Edited by:
Alberto Arab, Federal University of ABC, BrazilReviewed by:
Paul Eggleton, Natural History Museum, United KingdomFabiana E. Casarin, Federal University of São Paulo, Brazil
Copyright © 2022 Chouvenc, Ban and Su. This is an open-access article distributed under the terms of the Creative Commons Attribution License (CC BY). The use, distribution or reproduction in other forums is permitted, provided the original author(s) and the copyright owner(s) are credited and that the original publication in this journal is cited, in accordance with accepted academic practice. No use, distribution or reproduction is permitted which does not comply with these terms.
*Correspondence: Thomas Chouvenc, dG9tY2hvdXZAdWZsLmVkdQ==
†Deceased