- 1Biology Centre of the Czech Academy of Sciences (CAS), Institute of Entomology, České Budějovice, Czechia
- 2Department of Zoology, Faculty of Science, University of South Bohemia, České Budějovice, Czechia
- 3Institut de Systématique, Evolution, Biodiversité, Muséum National d’Histoire Naturelle, CNRS, Sorbonne Université, EPHE, Université des Antilles, Paris, France
- 4Organismal and Evolutionary Biology Research Program, Faculty of Biological and Environmental Sciences, University of Helsinki, Helsinki, Finland
Advertising escape ability could reduce predatory attacks. However, the effectiveness of certain phenotypic cues (e.g., color, shape, and size) in signaling evasiveness is still unknown. Understanding the role of such signals in driving predator learning is important to infer the evolutionary mechanisms leading to convergent evasiveness signals among prey species (i.e., evasive mimicry). We aim to understand the role of the color pattern (white patches on dark background) and morphology (extended butterfly hindwings) in driving learning and avoidance of escaping prey by surrogate avian predators, the European blue tit. These cues are common in butterflies and have been suspected to advertise escape ability in nature. We use dummy butterflies harboring shape and color patterns commonly found in skippers (family Hesperiidae). The prey models displayed the studied phenotypical cues (hindwing tails and white bands) in factorial combinations, and we tested whether those cues were learned as evasive signals and were generalised to different phenotypes. Our results suggest that hindwing tails and white bands can be associated with prey evasiveness. In addition, wild blue tits might learn and avoid attacking prey models bearing the studied phenotypic cues. Although blue tits seem to have an initial preference for the phenotype consisting of white patches and hindwing tails, the probability of attacking it was substantially reduced once the cues were associated with escaping ability. This suggests that the same morphological cues might be interchangeable as preference/avoidance signals. Further investigation of the salience of hindwing tails vs. white bands as cues for escaping ability, revealed that predators can associate both color pattern and shape to the difficulty of capture, and possibly generalize their aversion to other prey harboring those cues. More studies with larger sample sizes are needed to confirm this trend. Altogether, our results highlight the hitherto overlooked role of shape (butterfly hindwing tails) for signaling prey unprofitability.
Introduction
Anti-predatory interactions can lead to astonishing phenotypic diversity shaped by selection and adaptation. A classic example of natural selection is aposematism, when predators learn to associate a prey phenotype (e.g., a vivid color pattern) with an unpleasant experience (Poulton, 1890; Cott, 1940). This results in the selection of signals advertising unprofitability, which predators can recognize and generalize to avoid defended prey (Ruxton et al., 2004). Aposematic cues are warning signals that are present in prey species and indicate the existence of defense mechanisms, such as secondary chemical compounds (Pawlik et al., 1988; Santos et al., 2016) or primary defenses, such as warning coloration (Johansen et al., 2011; Pinheiro et al., 2016) or elusiveness (FitzGibbon and Fanshawe, 1988; van Belleghem et al., 2020).
In advertising unprofitability associated with a primary defense, there is still little empirical evidence on how predators learn and respond to different phenotypes, and whether signaling evasiveness is beneficial for both prey and predators in nature (Gibson, 1974, 1980; Hancox and Allen, 1991; Pinheiro and Freitas, 2014; Llaurens et al., 2021; Páez et al., 2021). Warning colorations associated with secondary defense mechanisms have been studied extensively and we have already a good understanding that bright, contrasting colors are easy to learn and memorize by predators (Dell’aglio et al., 2016; Casas-Cardona et al., 2018). However, other phenotypic cues, like body shape or combination of cues (e.g., coloration and shape) have been studied much less (but see Valkonen et al., 2011; McLean and Herberstein, 2021) and have not been associated with advertising evasiveness. While the effect of shape has been tested for secondary defense mechanisms (Valkonen et al., 2011; unpalatability – Hegedus et al., 2019), the salience of shape in advertising prey evasiveness is yet to be tested conclusively.
Cues that advertise difficulty of pursuit have been studied in a few living organisms [e.g., weevils (Guerra, 2019) and butterfly/grasshoppers (Balgooyen, 1997)] and largely lacked an experimental design to test for stimulus salience among prey colors and body shapes (Young, 1971; Srygley, 1994; Pinheiro, 1996; Golding et al., 2005). In palatable butterflies, certain color patterns, such as white bands on forewings, have been associated with advertising escape ability, but such conclusions were based only on observations in the field of tropical insectivorous birds aiming to attack flying butterflies (Pinheiro and Freitas, 2014; Pinheiro and Cintra, 2017). Further, butterfly wing shapes are also strongly correlated with flight speed and maneuverability (Ortega et al., 2017; Le Roy et al., 2019), which might as well advertise escape ability in the form of flight behavior and be under strong selection pressure (Srygley, 1994). The evidence for cues solely advertising difficulty of pursuit is also equivocal as certain wing shapes, such as hindwing tails, and certain color patterns, such as eye spots, have been related to deflecting attacks (Weeks, 1903; Blest, 1957; Lyytinen et al., 2004), disruptive cryptic coloration (Tan et al., 2020), and camouflage (Cuthill et al., 2005; Fraser et al., 2007). For example, butterfly hindwing tails are fragile and are attacked more often compared to other body parts, suggesting an evolutionary pressure driven by predators for a deflection effect and the salience of tails (Chotard et al., 2022). Nonetheless, in the few behavioral experiments to date, it has been shown that signaling evasiveness using colored cues might be as effective as signaling distastefulness in educating naïve predators (Gibson, 1974, 1980; Hancox and Allen, 1991; Páez et al., 2021).
Recent molecular phylogenies have suggested large-scale convergent patterns in wing phenotypes of skipper butterflies (family Hesperiidae), i.e., unrelated species having evolved similar shapes with extended hindwings (tails) and white bands on forewings (Li et al., 2019; Zhang et al., 2019). Skippers possess strong evasive power among butterflies and have one of the fastest startle reflexes in the animal kingdom (Sourakov, 2009). Although the evolutionary mechanisms driving such convergences are not yet known, cues such as white bands might be associated with evasive mimicry in Neotropical butterflies (Pinheiro and Freitas, 2014).
Here, we ask whether prey color patterns and/or wing shapes are effective in signaling evasiveness and in triggering predator learning. Moreover, we investigate whether the generalization of such cues to different prey phenotypes is possible. To test our research question, we use an experimental setup that has recently been deployed to test whether European blue tits (Cyanistes caeruleus) learn to avoid colored evasive prey (Páez et al., 2021).
Materials and Methods
Wild blue tits have been used in behavioral experiments as surrogate predators because of both their cognitive abilities to learn complex feeding tasks and ease of rearing in the laboratory (Church et al., 1998; Grieco, 1999; Hämäläinen et al., 2020). From December 2020 to March 2021 (Year 1) and November 2021 to February 2022 (Year 2), we caught wild blue tits in the campus vicinity of the University of South Bohemia in České Budějovice, Czechia (48.980N, 14.417E, and 48.992N, 14.435E). The habitat of the birds consisted of moist to xerothermic scrubland with poplar, pine, blackthorn, and oak. During collection, we recorded the birds’ age, sex, and weight. We kept the birds individually in breeding cages of 45 cm × 45 cm × 90 cm with the walls covered from the outside by bed linen to reduce stress. The roof of the cage was left open for illumination. The breeding cages were in a room with a 12/12-h light cycle. We fed the birds ad libitum with water supplemented with vitamins, sunflower seeds, insect pate, mealworms, and mixed grains. We kept the birds in captivity for a maximum of 10 days, and we released them back to their habitat as soon as they completed the behavioral experiments.
All the necessary permits were obtained for this research: license no. 1004 issued by the National Museum in Prague to capture wild birds, license no. 43873/2019-MZE-18134 issued by the Czech Ministry of Agriculture to keep wild birds in captivity, permit no. 22395/2014-MZE-17214 issued by the Ministry of Agriculture of the Czech Republic, and license no. MZP/2020/630/1544 was granted by the Czech Ministry of Environment to conduct behavioral experiments with wild birds. The study was approved by the Ethics Committee of the Faculty of Science, University of South Bohemia, and the experiments were approved by a licensed person (Petr Veselý – license no. CZ02766 issued by the Ministry of the Agriculture of the Czech Republic). This research adhered to the Association for the Study of Animal Behaviour/Animal Behaviour Society (ASAB/ABS) guidelines for the use of animals in research and complied with the laws of the Czech Republic and the European Union.
Prey Models
We used paper models (distance between both tips of the forewings: 3 cm; distance between the top of the head and end of the hindwing tail: 3.2 and 1.8 cm for models with and without tails, respectively) of skipper butterflies classified in the tribe Eudamina (Hesperiidae, Eudaminae) as prey. We constructed four phenotypes based on a photograph of the species Autochton neis (Geyer, 1832) that was further edited in the software Inkscape v1.0. As we were interested in wing color patterns and shapes triggering learning of evasive prey, we constructed the following models: the presence of hindwing tails (phenotype T), white bands (phenotype W), white bands and hindwing tails (phenotype WT), and neither white bands nor tails (phenotype O) (Figure 1). Such models resemble actual skipper butterfly phenotypes found in the Neotropics (Figures 1A–D). We also created two butterfly dummies having different wing color patterns and shapes, a brown dummy used as a control during the learning phase in Year 1 (Figure 1) and a black dummy used during the Pre-training phase to acclimatize the birds to the experimental setup. The models were printed using a Color LaserJet Pro MFP M277dw printer and REY international paper 80 g/sqm.
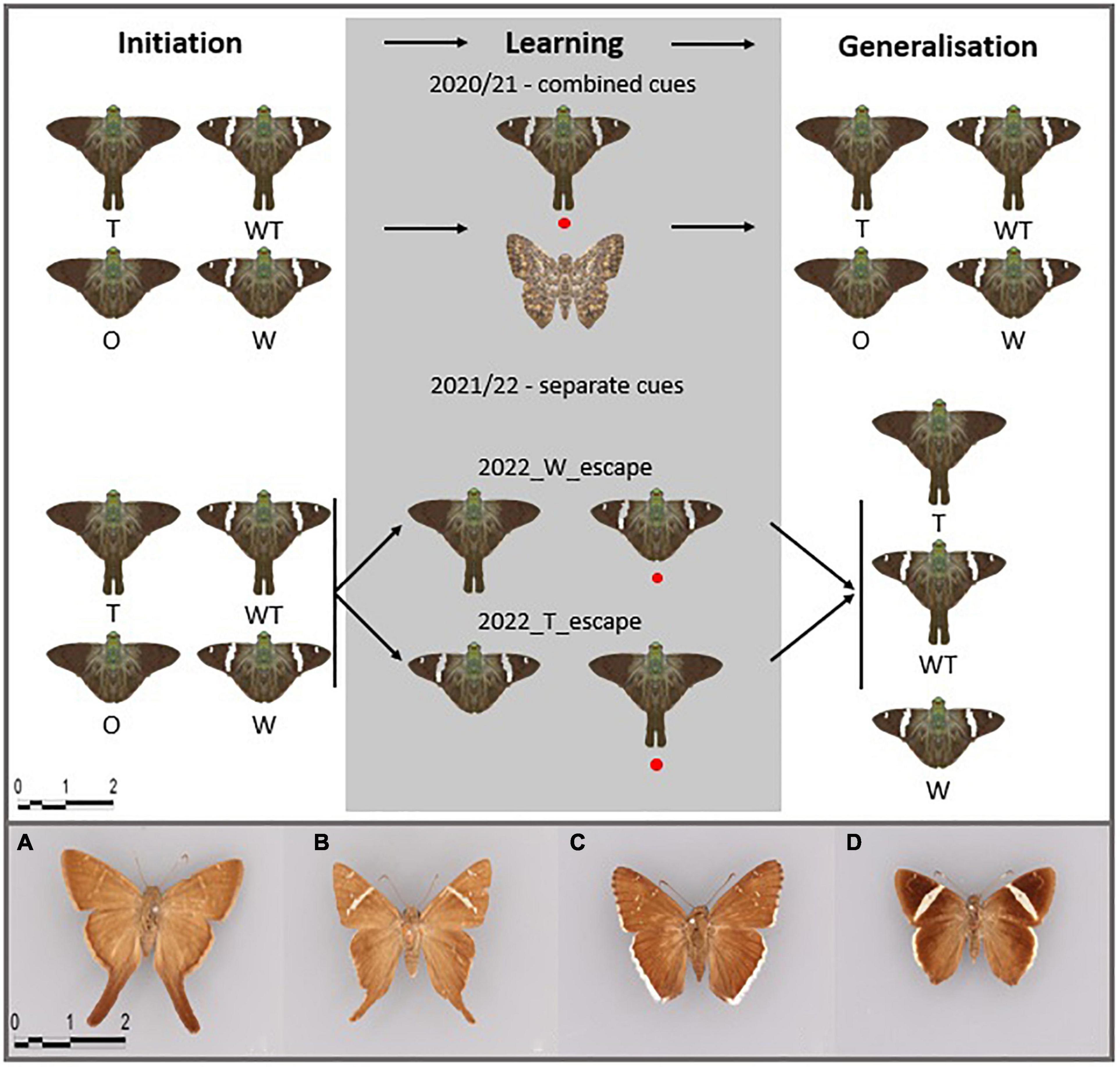
Figure 1. Experimental setup for the three behavioral tests of learning and generalization abilities of pre-trained blue tits and depiction of used butterfly phenotypes: T, hindwing tails; W, white bands; WT, white bands and hindwing tails; O, neither hindwing tails nor white bands. The red circle marks the evasive model during the Learning phase. Initiation was introduced during the winter of Year 1 resulting in a lower sample size. The phenotypes resemble Neotropical butterflies: (A) Spicauda simplicius (Stoll, 1790), (B) Cecropterus carmelita (Herrich-Schäffer, 1868), (C) C. casica (Herrich-Schäffer, 1868), and (D) C. zarex (Hübner, 1818) (captured in the Natural History Museum Berlin).
Behavioral Experiment
We pre-trained the birds to find almond pieces (rewards) on the underside of the dummy forewings. The Pre-training phase started 2 days after catching the birds, so they were already acclimatized to the breeding cages. The birds were pre-trained in a stepwise manner, by displaying the reward in the breeding cages: (1) two almond flakes with no dummy butterfly, (2) almond flakes fully visible on top of two dummies, (3) almond flakes glued to the underside of two dummies, and partially visible from above, and (4) almond flakes glued to the underside of two dummies, and not visible from above. The pre-training was considered successful and the bird was ready for the experiment once it ate all the almond pieces in each consecutive step.
The behavioural experiments consisting of initial preference, learning, and generalization (Figure 1) was carried out in a dark room and using 50 cm × 50 cm × 80 cm experimental boxes made of plywood as described in Páez et al. (2021) (except that we illuminated the cages using LED strips SQ3-300, 4800K, 74 CRI, no UV emission, 30 cm per box). The box was equipped with a wooden perch (25 cm height), a bowl of fresh water, two iron rails on either side for pulling the butterfly’s dummies, and the floor was covered with a light brown wrapping paper. The front side of the experimental box was outfitted with polarized Plexiglas to allow observations of birds’ behavior with minimal stress for the birds. To evaluate the motivation of the birds before the experiments, we offered two black pre-training dummies with hidden almonds on the underside of the model, and we waited for their consumption.
The initial preference (Initiation phase) of blue tits for any of the study phenotypes was tested using a subset of 17 birds during the winter of Year 1 (December–March) and 49 birds during the winter of Year 2 (November–February). The Initiation phase was conducted by simultaneously offering all four butterfly dummies (WT, T, W, and O), all arranged randomly in every trial and recording the attack order and attack time. We waited until all four dummies were attacked or a maximum of 10 min.
During the Learning phase, we displayed two different butterfly dummies with hidden almonds: the control and the escape models. Controls were allowed to be consumed. Escape models were not allowed to be consumed. To resemble an evasive behavior by the escaping model, we rapidly pulled the butterfly dummy out of the cage (<1 s) as soon as the bird showed signs of attacking the prey and was closer than 5 cm to it. The learning experiment consisted of a maximum of 80 trials per bird. During the first five trials, we allowed the bird to approach both models to kickstart the learning process (see the video in Supplementary Material 1). Afterward, we allowed the bird to make only one choice per trial, leaving the control dummy to be consumed or pulling the escape phenotype out of the cage. After every trial, we swapped the sides where we placed the escaping and control dummies, either to the left or to the right of the experimental cage. We considered the Learning phase successful once the control dummy was consumed in 10 consecutive trials, allowing a maximum of 2 mistakes in between. Two different learning setups were used, hereafter called combined cues (investigation of whether white bands and hindwing tails combined trigger learning of evasive prey) and separate cues (investigation of whether white bands or hindwing tails trigger more effective and memorable learning of evasive prey).
During the Generalization phase, we evaluated the salience of the learned traits: shape (hindwing tails) or color pattern (white bands). We gave a minimum of a 30-min break to every bird after completing the Learning phase. If the bird showed signs of fatigue (i.e., falling asleep on the perch or being uninterested in the butterfly models and offered food) after the Learning phase, we continued with the Generalization phase the following day.
Combined–Cues – Learning and Generalization Phases
We studied the salience of the combination of the white band (color) and hindwing tail (shape) when associated with escaping behavior. During the experimental season of Year 1, the escaping model in the Learning phase was the white bands and hindwing-tailed phenotype (WT), and the control was represented by a brown butterfly model with different wing shapes. The Generalization phase was conducted in the same way as the Initiation phase, that is, all four phenotypes (WT, T, W, and O) were simultaneously offered with the position of phenotypes arranged randomly for every bird. We recorded the amount of time it took the bird to attack each phenotype model for a maximum of 10 min. If cues are memorized and generalized, we expected that the birds avoid the WT phenotype after learning its evasive abilities, followed by a model bearing the most salient cue – color (W phenotype) or shape (T phenotype).
During Year 1 additional birds were processed using an altered generalization setup, while the initiation and learning phases were kept the same. However, due to an insufficient sample size for this subset, the generalization results were not used, but the Learning and Initiation results were merged with the remaining experimental data of Year 1 discussed here (full bird list, see Supplementary Material 2).
Separate–Cues – Learning and Generalization Phases
We tested the relative strength of either color (white bands) or shape (tails), in triggering the learning and generalization of evasive prey. Individual birds were used for one of the two Learning phase setups, each differing in the escaping model: with T as the escape model and W as the control (Year 2_T_evasive), and with W as the escape model and T as the control (Year 2_W_evasive) (Figure 1). We used either W or T phenotypes as control models during Year 2 because cue efficiency might differ between both stimuli (color and shape) and might depend on which cue is associated with positive (control) or negative experiences (evasive phenotype). The generalization setup was the same for both experiments and consisted of displaying W, T, and WT models (Figure 1). We expected that white bands and tails will be similarly important signals for the bird predators, thus, the birds during generalization would avoid the learned evasive phenotype followed by the model-harboring phenotype WT, since it harbors the salient cue (either tail or white band).
Statistical Analyses
We tested differences in attack rates between the escaping and control models, in relation to the number of learning trials. To evaluate the learning curves, we used generalized linear mixed model (GLMMs) with binomial distribution using the lme4 package (Bates et al., 2015) in R x64 4.0.2 (R Foundation for Statistical Computing, 2020). For both GLMMs we considered data from all tested birds (including birds that failed the learning criterion or were non-cooperative). The GLMMs, thus, gave a better understanding of the actual learning behavior compared to the average number of trials needed to learn the evasive phenotype. Using model selection, we accounted for all additive, direct interactions between four additional factors (age, sex, year, experiment – i.e., either WT, W, or T as the evasive phenotype), as well as all possible second-order interactions between these factors. Automatic model selection was facilitated using the MUMIn package (Burnham and Anderson, 2010). Additionally, the learning difference between both experiments in Year 2, i.e., evasive phenotypes W and T, was compared using a reduced GLMM by omitting data from Year 1 using the same model selection tools as explained previously, and all parameters (experiment, age, and sex) including their second-order interactions.
To compare success rates (in the Pre-training and Learning phases), as well as sex and age ratios, we employed Chi-square tests; we compared the observed rates during Year 2 to the expected rates based on the results from Year 1. We used standard Chi-square tests in the Initiation and Generalization phases to test if birds had any biases to prefer or avoid any of the four prey types. In addition, for the comparison between attack rates during the Initiation and Generalization phases, a Chi-square test was used, where the initial preference’s probabilities were considered as the null model. This was possible only for the experiments in Year 1, where Initiation and Generalization designs were similar, and not for experiments in Year 2, where O was deliberately excluded from the Generalization phase to investigate exclusively the effect of T and W. Instead, generalization results for birds that were exposed to the two different types of evasive prey (i.e., T and W) were compared with each other. In all comparisons, only the first attack was used for statistical analysis. As a complementary analysis to our Chi-square tests, we also conducted log-likelihood tests following Páez et al. (2021); see also (Mérot et al., 2015; Willmott et al., 2017). We compared scenarios with different configurations of attack rates (e.g., identical attack rates on all prey, attack rates all different, and attack rate different for WT) and compared them using AICc values (all scenarios are presented in Supplementary Material 3). For Year 1 we were also able to implement a model matching the attack rates observed during the Initiation phase.
Graphics were generated using R x62 4.0.2 (R Foundation for Statistical Computing, 2020) using the ggplot2 package (Wickham, 2016).
Results
During the experiments in Year 1 (combined cues), the Pre-training phase was completed by 43 out of 46 caught birds (93%), and 36 pre-trained birds (84%) learned to avoid the escaping butterfly (phenotype WT). Of the 36 birds that passed the Learning phase, 15 (42%) were males and 21 (58%) were females. Three birds (8%) were juvenile hatchlings of 2020 (i.e., younger than 6 months), and 33 birds (92%) were at least 6-month-old. These numbers differ significantly from those of the birds caught during Year 2 (separate cues) (Table 1), when 63 blue tits were caught, and only 49 of these completed the Pre-training phase (78%). Further, only 34 pre-trained birds completed the Learning phase successfully (69%). Of the birds that completed the Learning phase, 15 (44%) were females and 19 (56%) were males, while 16 (47%) were juveniles (caught mostly in November and December) and 18 (53%) were adults (see Table 1). All 34 birds completed the Generalization phase successfully, but 1 of them was excluded because a wrong combination of phenotypes was offered during Generalization. During Year 2, significantly more birds failed the Pre-training phase (N = 63; df = 1, χ2 = 25.4, p < 0.001) and the Learning phase (N = 49; df = 1, χ2 = 7.4, p = 0.007) compared to the experiments in the winter of Year 1. Additionally, during Year 2 the proportion of juveniles was significantly higher compared to Year 1 (N = 34; df = 1, χ2 = 66.7, p < 0.001). No differences in the sex ratio between years were detected (N = 34; df = 1, χ2 = 0.08, p = 0.77) (Table 1). Further, no direct influence of age, sex, or experiment was detected in the model selection using GLMMs. Summarizing table for all birds is found in Supplementary Material 2.

Table 1. Comparison between Year 1 and Year 2 regarding number of caught birds, successful birds (Pre-training and Learning phase), sex ratio, and proportion of juveniles.
Initiation
Although not statistically significant, results of the Initiation phases suggest some form of prey preference for the phenotype WT across years (Figure 2). These results were more obvious during the experimental season in Year 1 when almost half (47%) of the birds attacked the WT model (white bands and hindwing tails), followed by the O model (neither tails nor bands, 29%). While those differences were not statistically significant with the Chi-squared test (N = 17; df = 3, χ2 = 5.82, p = 0.12), the log-likelihood analysis highlighted three equally likely scenarios (i.e., ΔAICc < 2; Supplementary Material 4): (1) all models are attacked at the same rate (AICc = 35.48), (2) WT is attacked more frequently than the other prey (AICc = 35.71), and (3) WT and O are attacked more frequently than W and T (AICc = 35.20).
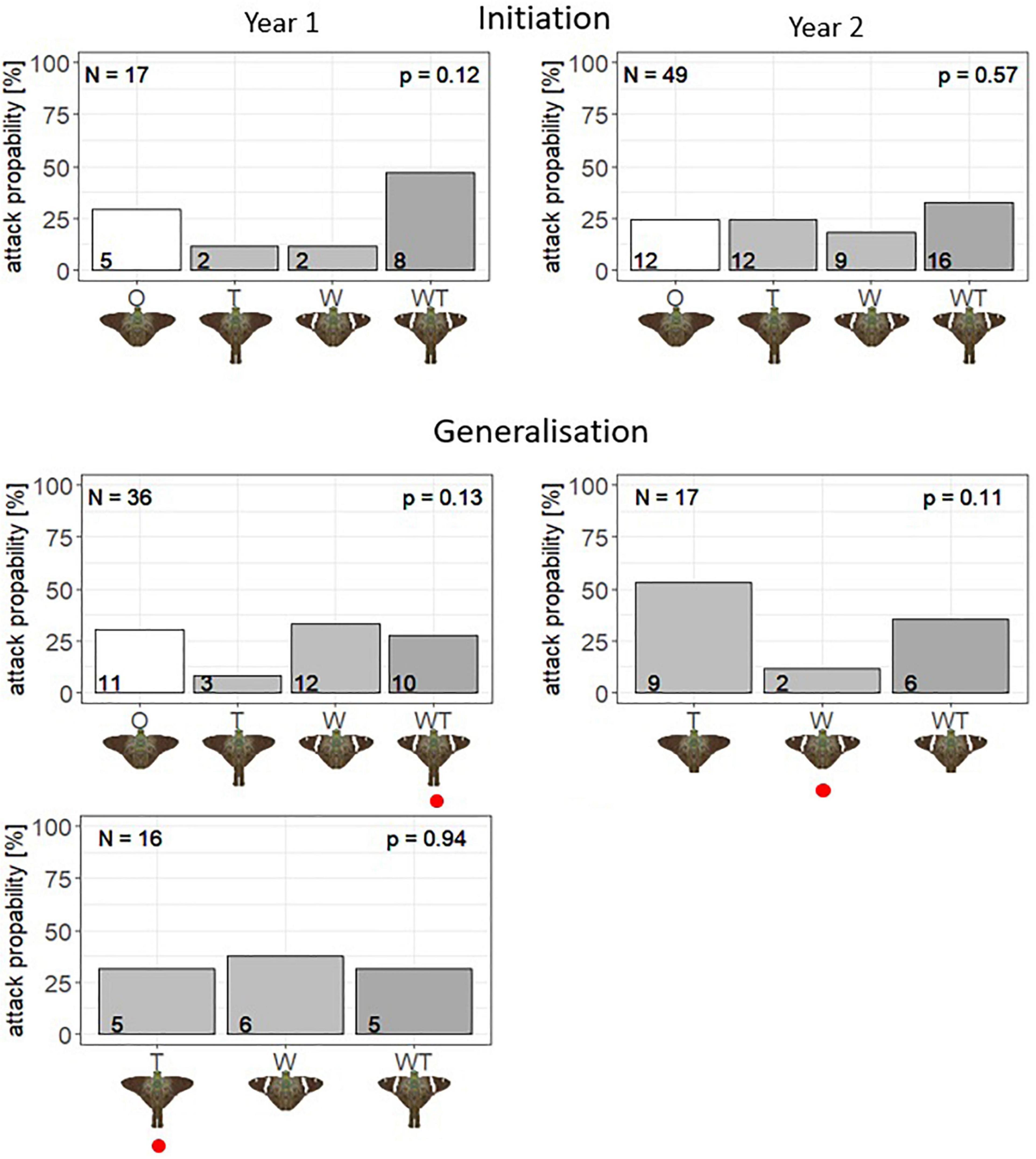
Figure 2. Proportion of blue tits to first attack the different offered butterfly dummies (O, T, W, and WT) during Year 1 (left column), and Year 2 (right column). The first row represents Initiation results, while the second and third rows are representing Generalization results. The number in each bar indicates the absolute number of first attacks on this phenotype and the p-value is the result of the Chi-square test comparing any difference in attack rates among prey models within each experiment. The red circle marks the evasive model during the Learning phase.
During Year 2, a similar initial preference pattern was detected, although the differences were less pronounced as 33% of all birds attacked WT first, followed by the O and T phenotypes with 25% each. Again, no significant difference among the attacked prey was detected with the Chi-square test (N = 36; df = 3, χ2 = 2.02, p = 0.57) (Figure 2), but under the log-likelihood framework three models were equally likely: (1) all models are attacked at the same rate (AICc = 97.82), (2) WT is attacked more frequently than the other prey (AICc = 99.14), and (3) W is attacked less frequently than the other prey (AICc = 99.30, Supplementary Material 4). Although not fully conclusive, the observations of a possible attack preference for phenotype WT are consistent across years and the only difference is that in Year 2 there appears to be less preference towards W, whereas in Year 1 the models W and T were less preferred.
All Initiation diagrams depicting all four attacks can be found in Supplementary Material 5.
Learning
Although the wild blue tits seem to have an initial preference for the phenotype WT during the winter of Year 1, they successfully learned to avoid such a phenotype when associated with escaping prowess (36 of 43 birds were successful, while 6 adult females and 1 adult male did not successfully pass the learning criteria). Among birds that succeeded in learning, the females took on average 34.5 ± 21.8 (N = 26) trials, while the males took 36.5 ± 22.6 (N = 15) trials to fulfill our learning criteria (Figure 3).
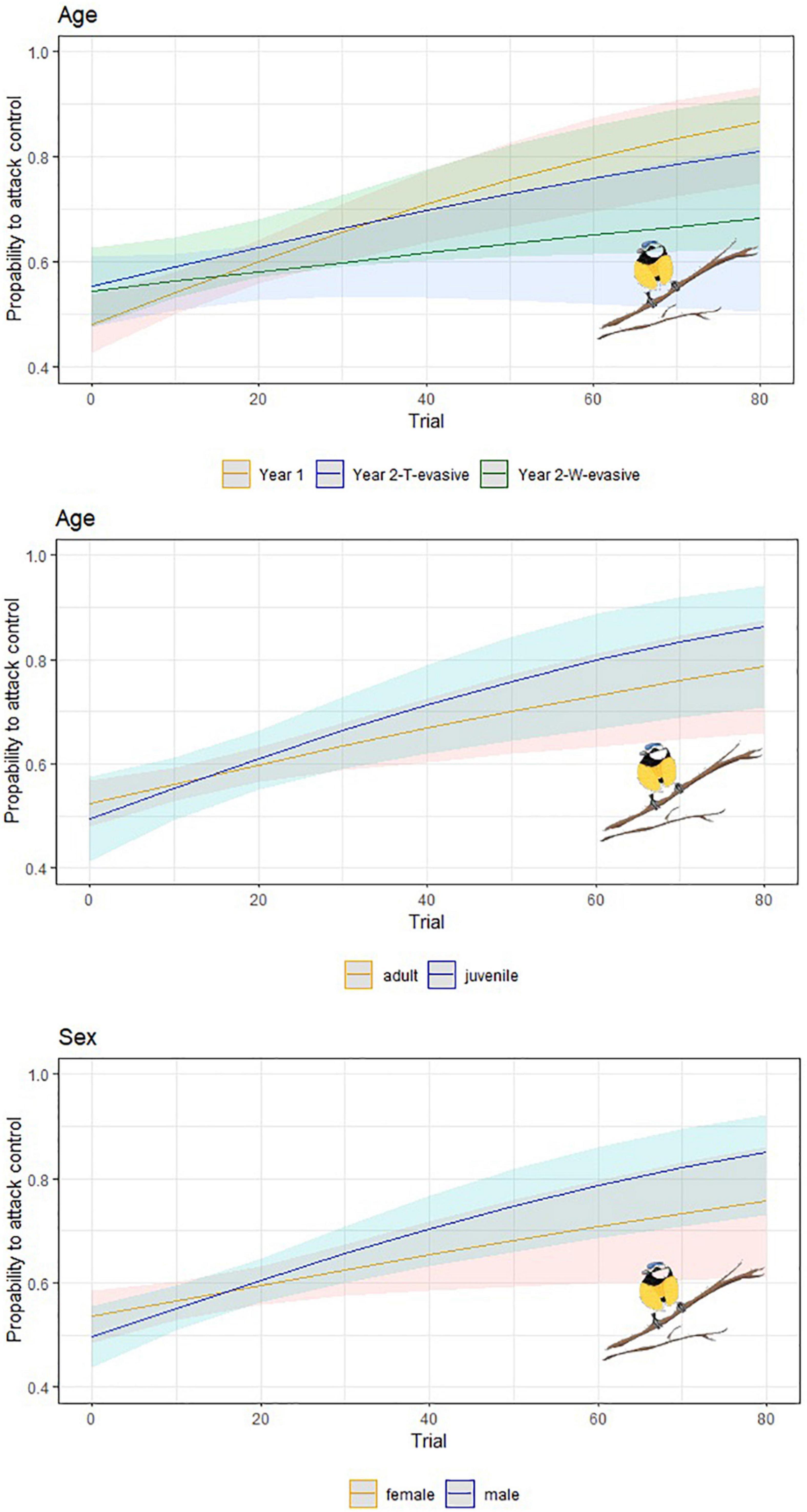
Figure 3. Predicted probability of attacks targeting the escaping model by blue tits in dependence on the number of trials. Upper-left: learning behavior separated between different experiments; upper-right: difference in learning behavior between adult and juvenile birds; lower-left: difference between female and male ones. Including 95% confidence intervals.
During Year 2, the blue tits (18 of 23 birds were successful, 2 females and 2 males were unsuccessful, 1 male bird was a juvenile) that learned that phenotype T is evasive (i.e., successful birds) required on average 35.5 ± 22 trials [N = 18; males (N = 11) = 35 ± 25, females (N = 7) = 36.3 ± 24.5]. Birds (16 of 26 were successful, 9 adult females, and 1 juvenile male failed learning), learning that phenotype W is evasive, took on average 40.5 ± 26.1 trials [N = 16, males (N = 8) = 43 ± 25.8, and females (N = 8) = 38 ± 25.1].
In the GLMM analysis accounting for the effect of trial, experiment type (i.e., WT, T, or W, as evasive models), year, sex, age, and their interactions, 15 models were within 2 units of AICc from the model with the lowest AICc (Table 2). The effect of trial was present in all best-fit models, while the effect of year was present in all best-fit models but one. Age, sex, and experiment were present in about half (eight models for age and six for sex and Experiment) of the models. The model with the lowest number of parameters (excluding the null model, which was not retained among the best model) had an only trial as a factor. Therefore, the birds’ avoidance of learning of the escaping prey was best explained by the number of experiences with the escape model represented by the learning trials, since this factor appeared in all retained models, including the simplest one. In addition to trial, the influence of different combinations of factors cannot be ruled out, as they appear in some of the retained models.
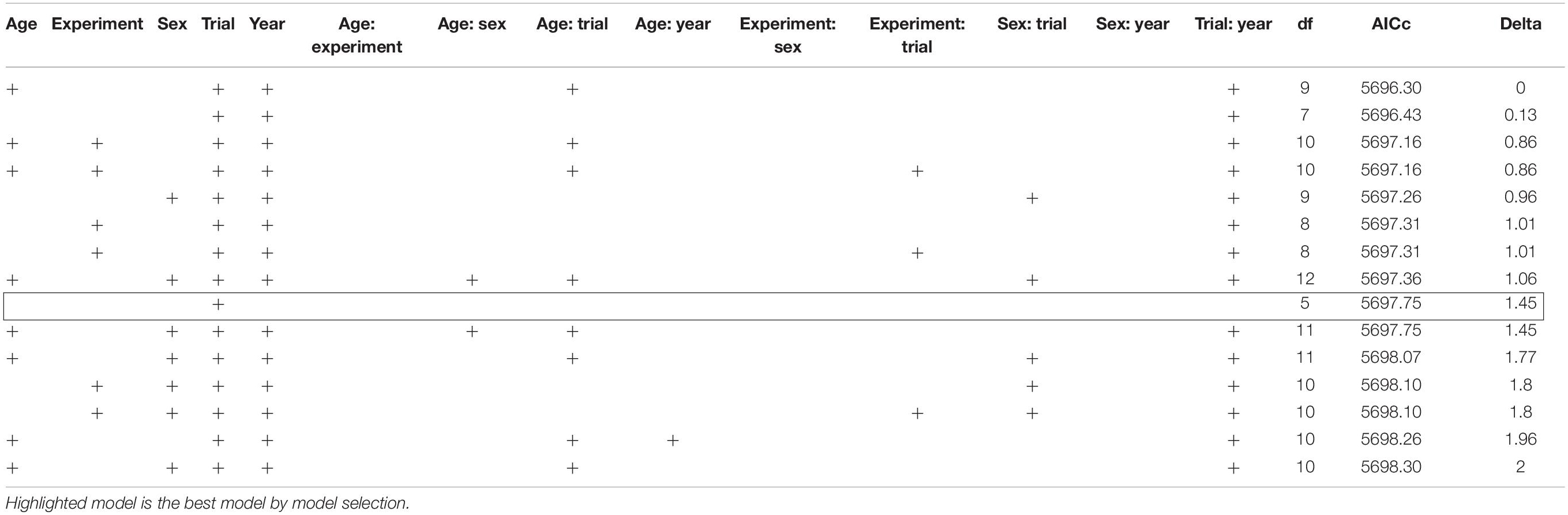
Table 2. Generalized linear mixed models (GLMMs) to assess the learning capabilities of evasive phenotypes (evasive phenotype in comparison to control model) by European blue tits in dependence sex, experiment, year, and age including all second-order interactions and a delta Akaike information criterion corrected for small sample sizes (dAICc) value of below 2.
Based on the learning curves (Figure 3), the escaping phenotype WT (shape and color) appeared to trigger the fastest learning behavior, followed by the escaping phenotype T (shape) and the escaping phenotype W (color). Thus, the prey shape seemed to trigger a faster learning behavior compared to the color cue alone (phenotype W). Additionally, males seem to learn faster than females and juveniles faster than adults (Figure 3), although sex and age factors were present in only half of the models (Table 2), meaning their effect cannot be ruled out. The GLMM, encompassing only the data from Year 2 to test for an effect of the experiment (either W or T escaping) without the confounding factor of year or experiment, revealed 17 models within 2 units of the AICc of the best model (Supplementary Material 6). The effect of the experiment was included in 12 of these models, supporting the observation of faster learning of the shape cue (phenotype T) than the color cue (phenotype W).
Generalization
In the experiment during Year 1, the proportion of first attacks of ∼50% on WT in the initial preference phase was reduced to ∼30% after learning that WT is an evasive prey (Figure 2). By contrast, the attack probability to the phenotype W (white bands) rose roughly threefold, from 12 to 34%. All tailed butterfly models (phenotypes WT and T) were more avoided than the non-tailed models (phenotypes W and O) during the Generalization phase, as about 58% of first attacks toward tailed models during the Initiation phase were reduced to 36% in the Generalization phase. Yet, the attack probabilities among the four model types during Generalization were not significantly different from random expectations based on the Chi-square test (N = 36, df = 3, χ2 = 5.56, p = 0.14). Nevertheless, a statistical comparison between the first attack probabilities in the Initiation and Generalization phases revealed a significant shift in the observed attack pattern driven by our Learning experiment (Chi-square test using Initiation attack rates as the null model: N = 36, df = 3, χ2 = 17.46, p < 0.005).
Further exploration using log-likelihood indicated that three scenarios were equally likely (Supplementary Material 3): (1) all phenotypes are attacked at the same rate (AICc = 72.45), (2) lower attack rate on T and WT (i.e., aversion toward tails, AICc = 73.07), and (3) lower attack rate on phenotype T (AICc = 71.07). Importantly, the scenario wherein the Generalization attack patterns matched the initiation was not supported (AICc = 74.24, Supplementary Material 4), again suggesting a shift in attack decisions driven by the Learning phase (specifically, a decrease in attack rate toward WT). Overall, these results suggest that birds might have retained the aversion for WT that was built during the Learning phase in a setting different from that of the Learning phase, despite the initial preference for this phenotype. However, there is no evidence for generalization toward models bearing some of the learned cues: T models suffer a lower attack rate in one of the scenarios, but this was already the case in the Initiation phase, as testified by one of the retained scenarios. Regarding the white band, while W had a lower attack rate during Initiation (testified by one of the best scenarios), during generalization it is the phenotype that suffers the largest number of attacks.
In the experiments during Year 2, when W was the escaping model, 53% of all birds attacked the T model first, followed by WT (35%) and W (12%) (Figure 2). The Chi-square test revealed no significant difference between these figures, possibly because of a low sample size (N = 17, df = 2; χ2 = 4.35, p = 0.11). In the log-likelihood analysis, two scenarios are equally likely (Supplementary Material 3): (1) all models are attacked at a similar rate (AICc = 30.46), and (2) W is attacked at a lower rate (AICc = 30.47, Supplementary Material 4). Both these scenarios were already represented during the Initiation but we were unable to compare the two phases directly because the setting was not the same (no O phenotype during Generalization). Nevertheless, there seemed to be a shift in attack rates between Initiation and Generalization, with attacks on T accounting for more than half of all attacks (i.e., on T + W + WT), whereas this proportion was only one third during Initiation. This suggests that the learned avoidance of W might have been retained, triggering the generalization of white bands with evasiveness to the phenotype WT.
When T was the escaping model, there was a slightly higher attack rate on W (T = 31%, WT = 31%, W = 38%), but this was not significant in either the Chi-square test or the log-likelihood analysis (Chi-square test: N = 16, df = 2; χ2 = 0.13, p = 0.93; log-likelihood: single best model has equal attack rates, AICc = 28.82, Supplementary Material 4). However, the fact that one of the best scenarios in Initiation, with higher attack rates for T and WT, is not found any more in Generalization suggests that birds might have retained aversion against T and generalized it toward WT.
Finally, a Chi-square test comparing the generalization results of both experiments showed a marginally significant difference driven by attacks on T and W (N = 17, df = 2; χ2 = 5.65, p = 0.06), suggesting again that the impact of the Learning phase may extend to the Generalization phase.
All Generalization diagrams depicting all four attacks can be found in Supplementary Material 7.
Overall, for Year 2, our results pointed toward learning of evasive prey driven by butterfly hindwing tails and white bands. However, our results were inconclusive regarding cue salience, possibly due to the high variability in behavioral studies stipulating large sample sizes to achieve significance, and a potential initial preference bias toward WT (and possibly against W). Nevertheless, our results hint at memorization of the escaping phenotype (i.e., the perfect mimic) in a context different from that of the Learning phase, and, possibly, to the generalization of the learned avoidance of prey that bears cues of the escape model, i.e., imperfect evasive mimics.
Discussion
In this study, we show that coloration (white bands) and butterfly wing shapes (hindwing tails) might be important cues in advertising prey escape prowess. Although blue tits showed some signs of an initial attack preference toward the white-banded and hindwing-tailed model (phenotype WT), the birds associated such cues with escaping prey and avoided attacking them. This suggests that initial preference for color and shape cues can be readily shifted toward aversion. This is in line with the expectations of signal theory, wherein effective signals (both sexual and warning) are those that the receiver pays more attention to and can be rapidly learned (Smallegange et al., 2006; Rodrigues et al., 2010; Finkbeiner et al., 2014). The patterns observed by our study represent the first step toward a deeper understanding of the importance of color and shape in signaling prey evasiveness. Both factors can act as important cues and can be memorized by cognitive predators in different attack contexts, and potentially foster generalization of learned avoidance to other prey bearing similar cues.
Indeed, our results suggest that differences in shape and color, such as the presence of tails or of white bands, can be learned and associated with escape abilities. Although white coloration is not considered a strong aposematic signal (Cibulková et al., 2014), its combination with other cues such as shape might result in stronger predator learning to avoid an unprofitable prey. Our results suggest that learning to avoid evasive prey with expected weaker warning colorations, in this case, white bands instead of yellow, orange, or red, is possible (Figure 2) and relatively fast compared to earlier escape experiments investigating conspicuous color patterns (Páez et al., 2021). In the case of the studied skipper butterfly phenotypes, it is possible that distantly related and co-occurring species in the wild converge to similar signals (white bands, hindwing tails) driven by predatory selection. Our results also suggest that a surrogate avian predator can learn and associate shape and color cues with evasive behavior, either in combination (phenotype WT) or separately (phenotypes W and T). As similar phenotypic patterns occur in sympatric species, the possibility of sexual selection in driving such convergences is limited, making the escape mimicry scenario a more plausible explanation (Pinheiro and Freitas, 2014; Penz et al., 2017).
Memorability and generalization in blue tits seem to depend on the cue combination associated with unprofitability during the Learning phase. When WT was the escaping model (Year 1), there was evidence for memorability, but not for generalization to either W (increased attack rate) or T (low attack rate, but this was already the case during Initiation). By contrast, when the escaping model displayed only one of these cues (W or T, Year 2), our results hint toward the possible generalization of avoidance to WT, although this remains to be confirmed with increased sample size. No conclusive explanation can be given for this pattern. However, it is possible that when several salient cues are combined, the effectiveness in advertising unprofitability might be increased.
We cannot rule out other external drivers of preference and memorability abilities of birds to certain phenotypic cues acting in different years. Birds caught during Year 2 required slightly more learning trials to succeed in our learning criteria compared to birds caught the previous year. In addition, proportionally fewer birds were able to successfully pass our Generalization phase in Year 2 (53.2%) compared to Year 1 (78.5%). One explanation is that the warmer conditions in Year 2, with almost no snow cover nor longer freezing temperatures in the study locality, might have influenced the learning and memorability abilities of the birds. Similar observations were made in Finland (J. Mappes, pers. obs.) and it has been shown that ambient temperature influences foraging behavior in birds (Chatelain et al., 2013). Alternatively, the higher amount of caught juveniles during Year 2 (47%) compared to Year 1 (8%) might have also influenced the observed differences in learning and generalization. Likewise, the apparent initial preference for the phenotype WT detected in Year 1 might be explained by the higher ratio of caught adults with likely more experience with handling prey in the wild, compared to the experiments in Year 2, when the preference for the phenotype WT was less pronounced.
Blue tits seem to prefer attacking prey with specific coloration and shape combinations, such as those found in red firebugs (Propokova et al., 2010). In the case of wild European blue tits, the marginal preference shown for white bands and hindwing tails (phenotype WT) might come from previous experience with butterfly phenotypes, having similar white markings, including the palatable nymphalid butterfly Araschnia levana (Linnaeus, 1758) (summer form) and the day flying geometrid Chiasmia clathrata (Linnaeus, 1758). However, no European butterfly species exactly resembles the phenotype WT found in the Neotropics. Another explanation for our findings is an apparent preference for large dummies with tails (body size), but the phenotype T with the same sizes were attacked at a lower rate in our initiation tests in Year 1 when a higher ratio of adult birds was caught. It is still puzzling that the birds did neither prefer hindwing-tailed (shape, phenotype T) nor white-banded butterflies (color, phenotype W), but the combination of both is only present in the escaping model WT (color and shape). However, it is clear that cues associated with escaping ability, either shape or color, are effective signals as the three escaping models W, T, and WT were learned by the blue tits.
Escape mimicry involves a primary prey defense, escape ability, which a predator might learn following unsuccessful attacks, which is before the capture or subjugation of prey (Marden and Chai, 1991; Speed et al., 2010). This is in contrast with mimicry involving secondary defenses in prey, such as unpalatability and toxicity, which require the capture, handling, and potential death of prey to educate naïve predators (McLain, 1984; Mallet and Gilbert, 1995). For both predator and prey, mimicry has benefits: prey species reduce attacks of cognitive predators by advertising unprofitability, while predators generalize signals from aversive experiences to make a cost-effective decision before pursuing a prey (MacDougall and Dawkins, 1998; Ihalainen et al., 2012). In Müllerian mimicry (Müller, 1878), aposematic signals are shared among two unprofitable species, thereby, sharing the cost to educate and deter predators, whereas, in Batesian mimicry (Bates, 1862), undefended species mimic an unprofitable model species. While theoretical (Ruxton et al., 2004), experimental, and observational evidence (Edmunds, 1974; Gibson, 1974, 1980; Llaurens et al., 2021; Páez et al., 2021) supports the existence of escape mimicry, it is yet unknown how prevalent it is in nature nor what cues in hard-to-catch prey (color, shape) are more memorable for predators. Here, we show that both color and shape are strong cues in advertising evasiveness and that they can be learned effectively by naïve predators. Because of our relatively low sample size and the high variability in individual bird responses, we still lack statistical power to assess the relative effectiveness of each cue (shape and color). Thus, our study only partially supports the hypothesis that the convergence of white bands observed in evasive Neotropical butterflies might be related to escape mimicry driven by avian predators (Pinheiro and Freitas, 2014).
Hindwing tails in Lepidoptera (butterflies and moths) are hypothesized to divert the attacks of predators to non-essential parts of the wing (Weeks, 1903; Blest, 1957; Chotard et al., 2022). Our results suggest that hindwing tails might as well be involved in signaling evasive behavior for visual predators, thereby, further reducing the risk of fatal attacks. When wild evasive butterflies lose the hindwing tails, the coloration of the forewings might still serve as cues signaling unprofitability, hence acting as a two-tiered system in diverting attack and advertising evasiveness. Nevertheless, further behavioral experiments are needed to determine whether the salience of shape remains when combined with stronger aposematic colorations such as yellow, orange, or red. Our results are hinting at a more complex predator-prey selection dynamic where both shape and coloration together might be of importance during learning of prey evasive capabilities.
Data Availability Statement
The raw data supporting the conclusions of this article are available at Doi: 10.5281/zenodo.6790077 and any further enquiries should be directed to the corresponding author.
Ethics Statement
The animal study was reviewed and approved by license no. MZP/2020/630/1544 granted by the Czech Ministry of Environment to conduct behavioral experiments with wild birds.
Author Contributions
DL: investigation, methodology, data curation and analysis, writing – original draft, review, and editing. ME: conceptualization, methodology, data analysis, review, and editing. IK: investigation, methodology, review, and editing. JM: conceptualization, methodology, review, and editing. PM-M: funding acquisition, investigation, methodology, conceptualization, review, and editing. All authors contributed to the article and approved the submitted version.
Funding
This study was provided by the Junior GAČR grant (GJ20-18566Y) and the PPLZ program of the Czech Academy of Sciences (fellowship grant L20096195), as well as GAJU n. 04-048/2019/P and n. 014/2022/P.
Conflict of Interest
The authors declare that the research was conducted in the absence of any commercial or financial relationships that could be construed as a potential conflict of interest.
Publisher’s Note
All claims expressed in this article are solely those of the authors and do not necessarily represent those of their affiliated organizations, or those of the publisher, the editors and the reviewers. Any product that may be evaluated in this article, or claim that may be made by its manufacturer, is not guaranteed or endorsed by the publisher.
Acknowledgments
We would like to extent our gratitude toward Petr Veselý and Michaela Syrová who helped us to catch and transport wild blue tits to the laboratory, as well as determined their age and sex. Furthermore, we would like to thank Klára Aurová, Stanislav Vrba, Legi Sam, Kateřina Sam, and student assistants Ladislava Krausová, Alena Fischerová, Marika Davídkova, Petra Krejcova, Tereza Hyblova, Barbora Belovska, and Jan Pixa during the experiments. We would also like to thank Leonardo Ré Jorge for his advice in statistical tests, and Théo Léger for the possibility to photograph butterflies in the MFN Berlin.
Supplementary Material
The Supplementary Material for this article can be found online at: https://www.frontiersin.org/articles/10.3389/fevo.2022.910695/full#supplementary-material
References
Balgooyen, T. G. (1997). Evasive mimicry involving a butterfly model and grasshopper mimic. Am. Midland Natur. 137:183. doi: 10.2307/2426768
Bates, D., Mächler, M., Bolker, B., and Walker, S. (2015). Fitting linear mixed-effects models using lme4. J. Statist. Softw. 67:i01. doi: 10.18637/jss.v067.i01
Bates, H. W. (1862). Contributions to an insect fauna of the amazon valley (lepidoptera: heliconidae). Biol. J. Lin. Soc. 16, 41–54. doi: 10.1111/j.1095-8312.1981.tb01842.x
Burnham, K. P., and Anderson, D. R. (2010). Model Selection And Multimodel Inference. A Practical Information-Theoretic Approach. New York, NY: Springer.
Casas-Cardona, S., Márquez, R., and Vargas-Salinas, F. (2018). Different color morphs of the poison frog Andinobates bombetes (dendrobatidae) are similarly effective visual predator deterrents. Ethology 124, 245–255. doi: 10.1111/eth.12729
Chatelain, M., Halpin, C. G., and Rowe, C. (2013). Ambient temperature influences birds’ decisions to eat toxic prey. Animal Behav. 86, 733–740. doi: 10.1016/j.anbehav.2013.07.007
Chotard, A., Ledamoisel, J., Decamps, T., Herrel, A., Chaine, A., Llaurens, V., et al. (2022). Evidence of attack defelection suggests adaptive evolution of wing tails in butterflies. Proc. R. Soc. B. 2022:562. doi: 10.1098/rspb.2022.0562
Church, S. C., Bennett, A. T. D., Cuthill, I. C., and Partridge, J. C. (1998). Ultraviolet cues affect the foraging behavior of blue tits. Proc. Biol. Sci. 265, 1509–1514. doi: 10.1098/rspb.1998.0465
Cibulková, A., Vesely, P., and Fuchs, R. (2014). Importance of conspicuous colors in warning signals: the great tit’s (parus major) point of view. Evolu. Ecol. 28, 427–439. doi: 10.1007/s10682-014-9690-2
Cuthill, I. C., Stevens, M., Sheppard, J., Maddocks, T., Párraga, C. A., and Troscianko, T. S. (2005). Disruptive coloration and background pattern matching. Nature 434, 72–74. doi: 10.1038/nature03312
Dell’aglio, D. D., Stevens, M., and Jiggins, C. D. (2016). Avoidance of an aposematically colored butterfly by wild birds in a tropical forest. Ecol. Entomol. 41, 627–632. doi: 10.1111/een.12335
Edmunds, M. (1974). Defence in Animals. A Survey Of Anti-Predator Defences/M. Edmunds. Harlow: Longman.
Finkbeiner, S. D., Briscoe, A. D., and Reed, R. D. (2014). Warning signals are seductive: relative contributions of color and pattern to predator avoidance and mate attraction in Heliconius butterflies. Evolution 68, 3410–3420. doi: 10.1111/evo.12524
FitzGibbon, C. D., and Fanshawe, J. H. (1988). Stotting in thomson’s gazelles: an honest signal of condition. Behav. Ecol. Sociobiol. 23, 69–74. doi: 10.1007/BF00299889
Fraser, S., Callahan, A., Klassen, D., and Sherratt, T. N. (2007). Empirical tests of the role of disruptive coloration in reducing detectability. Proc. Biol. Sci. 274, 1325–1331. doi: 10.1098/rspb.2007.0153
Geyer, C. (1832). Zuträge zur Sammlung Exotischer Schmettlinge, Zuträge Sammlung Exotischer Schmetterlinge. Augsburg: Im Verlag der Hübnerschen Werke bei C. Geyer.
Gibson, D. O. (1974). Batesian mimicry without distastefulness? Nature 250, 77–79. doi: 10.1038/250077a0
Gibson, D. O. (1980). The role of escape in mimicry and polymorphism: I. the response of captive birds to artificial prey. Biol. J. Lin. Soc. 14, 201–214. doi: 10.1111/j.1095-8312.1980.tb00105.x
Golding, Y. C., Edmunds, M., and Ennos, A. R. (2005). Flight behavior during foraging of the social wasp vespula vulgaris (hymenoptera: vespidae) and four mimetic hoverflies (diptera: syrphidae) Sericomyia silentis, Myathropa florea, helophilus sp. and syrphus sp. J. Exp. Biol. 208(Pt. 23) 4523–4527. doi: 10.1242/jeb.01932
Grieco, F. (1999). Prey selection in blue tits Parus caeruleus as a response to food levels. Acta Ornithol. 34, 199–203.
Hämäläinen, L., Mappes, J., Rowland, H. M., Teichmann, M., and Thorogood, R. (2020). Social learning within and across predator species reduces attacks on novel aposematic prey. J. Animal Ecol. 89, 1153–1164. doi: 10.1111/1365-2656.13180
Hancox, A. P., and Allen, J. A. (1991). A simulation of evasive mimicry in the wild. J. Zool. 223, 9–13. doi: 10.1111/j.1469-7998.1991.tb04745.x
Hegedus, M., Devries, P., and Penz, C. M. (2019). The influence of mimicry on wing shape evolution in the butterfly papilio dardanus (lepidoptera: papilionidae). Ann. Entomol. Soc. Am. 112, 33–43. doi: 10.1093/aesa/say045
Hübner (1818). Zuträge zur Sammlung exotischer Schmettlinge. Z. Samml. Exot. Schmett. 1, 4–6, 8–32, l.[1–2], f.1–12; l.[3–25], f.13–146; l.[26–35], f.147–200.
Ihalainen, E., Rowland, H. M., Speed, M. P., Ruxton, G. D., and Mappes, J. (2012). Prey community structure affects how predators select for Mullerian mimicry. Proc. Biol. Sci. 279, 2099–2105. doi: 10.1098/rspb.2011.2360
Johansen, A. I., Tullberg, B. S., and Gambrale-Stille, G. (2011). Motion level in Graphosoma lineatum coincides with ontogenetic change in defensive coloration. Entomol. Exp. Appl. 141, 163–167. doi: 10.1111/j.1570-7458.2011.01182.x
Le Roy, C., Debat, V., and Llaurens, V. (2019). Adaptive evolution of butterfly wing shape: from morphology to behavior. Biol. Rev. 94, 1261–1281. doi: 10.1111/brv.12500
Li, W., Cong, Q., Shen, J., Zhang, J., Hallwachs, W., Janzen, D. H., et al. (2019). Genomes of skipper butterflies reveal extensive convergence of wing patterns. Proc. Natl. Acad. Sci. 116, 6232–6237. doi: 10.1073/pnas.1821304116
Linnaeus, C. (1758). Systema naturae per regna tria naturae, secundum clases, ordines, genera, species, cum characteribus, differentiis, symonymis, Locis. Tomis I. 10th Edition. Syst. Nat. 1, 1–338, 339–824.
Llaurens, V., Le Poul, Y., Puissant, A., Blandin, P., and Debat, V. (2021). Convergence in sympatry: evolution of blue-banded wing pattern in Morpho butterflies. J. Evolu. Biol. 34, 284–295. doi: 10.1111/jeb.13726
Lyytinen, A., Brakefield, P. M., Lindström, L., and Mappes, J. (2004). Does predation maintain eyespot plasticity in Bicyclus anynana? Proc. R. Soc. B 271, 279–283. doi: 10.1098/rspb.2003.2571
MacDougall, A., and Dawkins, M. S. (1998). Predator discrimination error and the benefits of Müllerian mimicry. Animal Behav. 55, 1281–1288. doi: 10.1006/anbe.1997.0702
Mallet, J., and Gilbert, L. E. (1995). Why are there so many mimicry rings? Correlations between habitat, behavior and mimicry in Heliconius butterflies. Biol. J. Linn. Soc. 55, 159–180. doi: 10.1111/j.1095-8312.1995.tb01057.x
Marden, J. H., and Chai, P. (1991). Aerial predation and butterfly design: how palatability, mimicry, and the need for evasive flight constrain mass allocation. Am. Natur. 138, 15–36. doi: 10.1086/285202
McLain, D. K. (1984). Coevolution: müllerian mimicry between a plant bug (miridae) and a seed bug (lygaeidae) and the relationship between host plant choice and unpalatability. Oikos 43:143. doi: 10.2307/3544761
McLean, D. J., and Herberstein, M. E. (2021). Mimicry in motion and morphology: do information limitation, trade-offs or compensation relax selection for mimetic accuracy? Proc. Biol. Sci. 288:20210815. doi: 10.1098/rspb.2021.0815
Mérot, C., Frérot, B., Leppik, E., and Joron, M. (2015). Beyond magic traits: multimodal mating cues in Heliconius butterflies. Evolution 69, 2891–2904. doi: 10.1111/evo.12789
Ortega, A., Eastwood, R., Vogt, D., Ithier, C., Smith, M., Wood, R., et al. (2017). Aerodynamic evaluation of wing shape and wing orientation in four butterfly species using numerical simulations and a low-speed wind tunnel, and its implications for the design of flying micro-robots. Int. Focus 7:20160087. doi: 10.1098/rsfs.2016.0087
Páez, E., Valkonen, J. K., Willmott, K. R., Matos-Maraví, P., Elias, M., and Mappes, J. (2021). Hard to catch: experimental evidence supports evasive mimicry. Proc. Biol. Sci. 288:20203052. doi: 10.1098/rspb.2020.3052
Pawlik, J. R., Kernan, M. R., Molinski, T. F., Harper, M. K., and Faulkner, D. J. (1988). Defensive chemicals of the spanisch dancer nudibranch hexabranchus sanguineus and its egg ribbons: macrolides derived from a sponge diet. J. Exp. Mari. Biol. Ecol. 119, 99–109. doi: 10.1016/0022-0981(88)90225-0
Penz, C. M., Casagrande, M. M., Devries, P., and Simonsen, T. J. (2017). Documenting diversity in the amazonian butterfly genus (lepidoptera, nymphalidae). Zootaxa 4258, 201–237. doi: 10.11646/zootaxa.4258.3.1
Pinheiro, C. E. G. (1996). Palatablility and escaping ability in Neotropical butterflies: tests with wild kingbirds (Tyrannus melancholicus, tyrannidae). Biol. J. Lin. Soc. 59, 351–365. doi: 10.1111/j.1095-8312.1996.tb01471.x
Pinheiro, C. E. G., and Cintra, R. (2017). Butterfly predators in the neotropics: which birds are involved? J. Lepidopt. Soc. 71, 109–114. doi: 10.18473/lepi.71i2.a5
Pinheiro, C. E. G., and Freitas, A. V. L. (2014). Some possible cases of escape mimicry in neotropical butterflies. Neotr. Entomol. 43, 393–398. doi: 10.1007/s13744-014-0240-y
Pinheiro, C. E. G., Freitas, A. V. L., Campos, V. C., DeVries, P. J., and Penz, C. M. (2016). Both palatable and unpalatable butterflies use bright colors to signal difficulty of capture to predators. Neotr. Entomol. 45, 107–113. doi: 10.1007/s13744-015-0359-5
Poulton, E. B. (1890). The Colors of Animals, Their Meaning and Use, Especially Considered in the Case of Insects. New York, NY: D. Appleton and Company.
Propokova, M., Vesely, P., Fuchs, R., and Zrzavy, J. A. N. (2010). The role of size and color pattern in protection of developmental stages of the red firebug (Pyrrhocoris apterus) against avian predators. Biol. J. Lin. Soc. 100, 890–898. doi: 10.1111/j.1095-8312.2010.01463.x
R Foundation for Statistical Computing (2020). R: A language and environment for statistical computing. Vienna, Austria: R Core Team.
Rodrigues, D., Goodner, B. W., and Weiss, M. R. (2010). Reversal learning and risk-averse foraging behavior in the monarch butterfly, danaus plexippus (lepidoptera: nymphalidae). Ethology 116, 270–280. doi: 10.1111/j.1439-0310.2009.01737.x
Ruxton, G. D., Speed, M., and Sherratt, T. N. (2004). Evasive mimicry: when (if ever) could mimicry based on difficulty of capture evolve? Proc. Biol. Sci. 271, 2135–2142. doi: 10.1098/rspb.2004.2816
Santos, J. C., Tarvin, R. D., and O’Connell, L. A. (2016). “A review of chemical defense in poison frogs (dendrobatidae): ecology, pharmacokinetics, and autoresistance,” in Chemical Signals in Vertebrates 13. Cham, 2016, eds B. A. Schulte, T. E. Goodwin, and M. H. Ferkin (Cham: Springer), 305–337.
Smallegange, R., Everaarts, T., and van Loon, J. (2006). Associative learning of visual and gustatory cues in the large cabbage white butterfly, Pieris brassicae. Animal Biol. 56, 157–172. doi: 10.1163/157075606777304159
Sourakov, A. (2009). Extraordinarily quick visual startle reflexes of skipper butterflies (lepidoptera: hesperiidae) are among the fastest recorded in the animal kingdom. Florida Entomol. 92:653. doi: 10.1653/024.092.0420
Speed, M. P., Brockhurst, M. A., and Ruxton, G. D. (2010). The dual benefits of aposematism: predator avoidance and enhanced resource collection. Evolution 64, 1622–1633. doi: 10.1111/j.1558-5646.2009.00931.x
Srygley, R. B. (1994). Locomotor mimicry in butterflies? The associations of positions of centres of mass among groups of mimetic, unprofitable prey. Philos. Trans. R. Soc. London Seri. B Biol. Sci. 343, 145–155. doi: 10.1098/rstb.1994.0017
Stoll, C. (1790). Aanhangsel van het Werk, de Uitlandsche Kapellen Aanhangsel Werk. Uitl. Kapellen 1–42, 1–8.
Tan, E. J., Wilts, B. D., Tan, B. T. K., and Monteiro, A. (2020). What’s in a band? The function of the color and banding pattern of the banded swallowtail. Ecol. Evolu. 10, 2021–2029. doi: 10.1002/ece3.6034
Valkonen, J. K., Nokelainen, O., and Mappes, J. (2011). Antipredatory function of head shape for vipers and their mimics. PLoS One 6, e22272. doi: 10.1371/journal.pone.0022272
van Belleghem, S. M., Alicea Roman, P. A., Carbia Gutierrez, H., Counterman, B. A., and Papa, R. (2020). Perfect mimicry between Heliconius butterflies is constrained by genetics and development. Proc. Biol. Sci. 287:20201267. doi: 10.1098/rspb.2020.1267
Weeks, A. C. (1903). Theory as to evolution of secondaries of moths of the genus catocala. J. N.Y. Entomol. Soc. 11, 221–226.
Wickham, H. (2016). Ggplot2. Elegrant graphics for data analysis/Hadley Wickham; with contributions by Carson Sievert. Switzerland: Springer.
Willmott, K. R., Robinson Willmott, J. C., Elias, M., and Jiggins, C. D. (2017). Maintaining mimicry diversity: optimal warning color patterns differ among microhabitats in Amazonian clearwing butterflies. Proc. Biol. Sci. 284:1855. doi: 10.1098/rspb.2017.0744
Young, A. M. (1971). Wing coloration and reflectance in Morpho butterflies as related to reproductive behavior and escape from avian predators. Oecologia 7, 209–222. doi: 10.1007/BF00345212
Keywords: aposematism, attack avoidance, convergence, Hesperiidae, prey selection, phenotypic cues
Citation: Linke D, Elias M, Klečková I, Mappes J and Matos-Maraví P (2022) Shape of Evasive Prey Can Be an Important Cue That Triggers Learning in Avian Predators. Front. Ecol. Evol. 10:910695. doi: 10.3389/fevo.2022.910695
Received: 01 April 2022; Accepted: 10 June 2022;
Published: 12 July 2022.
Edited by:
Eunice Jingmei Tan, Yale-NUS College, SingaporeReviewed by:
Brett Michael Seymoure, Colorado State University, United StatesCarlos Cordero, National Autonomous University of Mexico, Mexico
Copyright © 2022 Linke, Elias, Klečková, Mappes and Matos-Maraví. This is an open-access article distributed under the terms of the Creative Commons Attribution License (CC BY). The use, distribution or reproduction in other forums is permitted, provided the original author(s) and the copyright owner(s) are credited and that the original publication in this journal is cited, in accordance with accepted academic practice. No use, distribution or reproduction is permitted which does not comply with these terms.
*Correspondence: Daniel Linke, ZGFuaWVsLmxpbmtlQGVudHUuY2FzLmN6