- 1Department of Cellular and Molecular Medicine, Koç University Research Center for Translational Medicine (KUTTAM), Istanbul, Turkey
- 2Department of Evolutionary Genetics, Max Planck Institute for Evolutionary Biology, Plön, Germany
In urban habitats, animals are faced with different and often challenging environmental conditions compared to their native habitats. Behavior is the fastest response to environmental change and therefore a very important component to adjust to human-altered environments. Behaviors such as novelty responses and innovativeness which allow animals to cope with novel stimuli are often altered in urban populations. The mechanisms producing such adaptations are currently not well understood. In this study, we investigate whether urban living has an impact on the microevolution of mouse behavioral and life-history traits including boldness, stress-coping, growth, longevity, and emphasis on reproduction. We hypothesized that animals living together with humans for longer show increased novelty-seeking and boldness characteristics at the species and subspecies level. We, therefore, compared behavior and life history characteristics among Mus musculus, a commensal rodent, Mus spicilegus as a synanthropic but not commensal, and Apodemus uralensis as a strictly rural species. In addition, we compared three subspecies of M. musculus (in total six populations) that differ in the time living together with humans. Behavioral and life history differences are stronger between populations even of the same subspecies rather than showing a structural trend with the time animals have spent with humans. In addition, species differ in behavior and life history, albeit not in a pattern that suggests an evolutionary adaptation to living in human-altered habitats. We, therefore, suggest that behavioral adaptations of wild mice are geared toward environmental differences such as geographic origin or habitat specifics but not necessarily directly evolve by living together with humans.
Introduction
Urbanization and the effects of humans on the planet’s flora and fauna have exponentially accelerated since the onset of the industrial revolution, causing native species to decline in numbers or be lost completely at an unprecedented rate. On the other hand, the change in the environment created novel niches for species that are able to adapt, for example, by decreasing the chance of predation, offering new food resources, and often ameliorate climatic conditions (McKinney, 2002; Gross, 2018; Szulkin et al., 2020). Currently, our understanding of why some species are able to adapt to and thrive in urban environments (so-called “urban dwellers”) while others merely cope, is limited. Urban dwellers are hypothesized to possess specific traits which enable them to cope with challenges such as frequent anthropogenic disturbances, altered social environments, light and air pollution or noise and habitat fragmentation that are presented by urban habitats (McKinney, 2002; Sih, 2013).
Behavior is generally the fastest response to cope with environmental challenges and changes (Sih et al., 2010; Lowry et al., 2013). Therefore, behavior has been proposed to be an important component in adapting to human-altered habitats. Indeed, several behaviors have already been shown to differ between urban and rural populations of the same animal species. For example, enhanced problem-solving abilities, reflecting high rates of flexibility and innovation, are being recognized as significant contributors for animals to thrive in human-altered environments (Reader, 2003; Sih, 2013; Vrbanec et al., 2021). In addition, behaviors that allow animals to cope with novel stimuli, such as high levels of neophilia and boldness or low levels of disturbance tendencies, are often altered in urban populations (Tryjanowski et al., 2016; Sol et al., 2018; von Merten et al., 2022). In addition to such behavioral adaptations, several studies indicate that life-history characteristics such as developmental time, longevity, and emphasis on reproduction, are altered in animals living in urban environments. For instance, Calliphora vicina Robineau-Desvoidy, a forensically important blowfly, is common in southern England and can occur in both urban and rural areas as well as in transition areas. C. vicina has altered life histories in urban areas that often have higher temperatures than the surrounding rural areas. Between 16 and 20°C, females grow slower but to a larger final mass than males, while at 28°C, both sexes grow similarly but males reach a larger maximum body mass (Hwang and Turner, 2009). A recent meta-analysis showed that urban birds generally have higher adult survival rates and thus tend to have a longer life (Sepp et al., 2018). In addition, urban birds seem to shift to fewer offspring for which they care more. In general, however, and for taxa other than birds, knowledge on which traits differ between urban and rural populations, is still largely missing.
Traits facilitating urban-dwelling might adapt through different mechanisms. In general, it is assumed that different forms of phenotypic plasticity, such as within-generation (e.g., learning and behavioral flexibility) and intergenerational plasticity (e.g., grandparental and parental effects or epigenetic processes) play an important role in the initial steps of shifting to an urban-dwelling life-style and may prevent individuals from being eliminated by changing environmental conditions (Lowry et al., 2013; Miranda et al., 2013). Therefore, phenotypic plasticity might often precede micro-evolutionary changes in populations adapting to urban habitats (Miranda, 2017). Micro-evolution is a process involving changes in gene frequencies across several generations of a population and thus occurs over prolonged time periods, eventually leading to speciation processes (Boutin and Lane, 2014; Nicoglou, 2015). Given the paucity of scientific studies investigating adaptations to urban environments, actual evidence for micro-evolutionary changes during the urban adaptation process is currently very scarce.
In this study, we aim to investigate whether living closely together with humans has an impact on the microevolution of mouse behavioral and life-history traits such as boldness, stress-coping, growth, longevity, and emphasis on reproduction. Therefore, we conducted a common-garden experiment with descendants of three species of wild mice; Apodemus uralensis, Mus spicilegus, and Mus musculus (house mice). Descendants of all mice have been kept and bred under standard laboratory conditions for several generations (8–16), thus, minimizing the potential of phenotypic plasticity to influence differences in traits. While A. uralensis and M. spicilegus have (on an evolutionary time-scale) recently begun to use human resources such as the abundant food supply on agricultural fields, M. musculus already has a long evolutionary history of close association with humans, representing one of the best studied examples of human-commensalism. Commensals are species that have already adapted to a life with and often off humans and have often already diverged from their ancestral form (Banks and Smith, 2015). Early human settlements led to the evolution of such human commensals and produced urban specialists (Johnson and Munshi-South, 2017). Studying adjustments of such undoubtedly successful exploiters of human habitats provides a natural evolutionary experiment and may also help to understand trait adjustments of non-commensal species to an urban life style.
Apodemus uralensis is a strictly non-commensal species occurring in central and eastern Europe through Russia and the Caucasus into China. Its preferred habitats are forest edges and habitats adjacent to woodlands, foraging both, on the ground and in trees, where it also often builds its nests (Denys et al., 2017). While a few newer studies report the occurrence of this species in agricultural areas, there are no reports that they have been encountered within human settlements (Cichocki et al., 2011). Mus spicilegus also does not use human settlements as a habitat but they have a much closer connection to humans, often occurring on harvested cereal fields and other agricultural areas (Denys et al., 2017). Thus, they are recognized as synanthropomorpic but not commensal (as for example, house mice) species. No official records about the social system of A. uralensis exist but the closest known relative, Apodemus sylvaticus, has a promiscuous mating system (Bartmann and Gerlach, 2001). Mus spicilegus on the other hand is known as the only Mus species practicing social monogamy (Denys et al., 2017).
The house mouse, M. musculus, diverged into several different subspecies. At least three of those subspecies have independently evolved a commensal lifestyle during the last several thousand years (Cucchi et al., 2012). The three subspecies Mus musculus musculus (MMM), Mus musculus domesticus (MMD), and Mus musculus casteneus (MMC) diverged about 350–500 thousand years ago. Records show that MMD already occurred in human settlements as early as 11000–13000 years ago (Bonhomme and Searle, 2012; Cucchi et al., 2012). MMM first occurred in human settlements around 8000 years ago in today’s Balkan area. MMC has spread into India, East Asia, and Southeast Asia and started to live commensally with humans around 7600–3800 years ago (Suzuki et al., 2013). To minimize potential effects of geographical origin rather than a subspecies effect, we used two populations of each subspecies originating from different geographical locations within the subspecies range. The social system of commensally living house mice is flexible, with polygynous males holding territories in which female groups with close kin associations live and raise their offspring communally (Denys et al., 2017).
House mice have long been a classic species to investigate changes due to commensal living. Morphological adaptations such as longer tails as well as changes in behavior and life history have been described previously (Berry, 1981; Pockock et al., 2005). Behaviors associated with flexibility (i.e., innovation propensity and inhibitory control) are heightened in house mouse subspecies that have lived together with humans for longer (Vrbanec et al., 2021). In addition, Frynta et al. (2018) found commensal populations to show increased exploration behavior of elevated places over non-commensal house mouse populations. Based on these previous results, we hypothesize that mice living together with humans more closely (species level) or for longer (subspecies level) show more novelty seeking behavior in general and a more active stress-coping strategy. In addition, we hypothesize these animals have evolved life history traits resembling a slower pace of life in accordance with what has been observed in birds (Sepp et al., 2018). In particular, house mice should have smaller litters and should be weaned at a higher proportional weaning weight than M. spicilegus and A. uralensis. A similar pattern should be observed on the subspecies level within house mice.
Materials and Methods
Animals and Housing Conditions
All mice (N = 232 + additional N = 305 for life history traits) used in this experiment are descendants of wild-caught animals of three different species of mice (M. musculus, M. spicilegus, and A. uralensis) that were kept and bred under standard laboratory conditions for several generations. Since capture, mice were bred in an outbreeding regime to maintain genetic diversity. Animals were bred in monogamous pairs and litters were removed after 4 weeks to avoid inbreeding. After weaning, same sex siblings were kept together in groups up to five animals per cage. All animals were kept in standard laboratory cages (Type III, Bioscape) that included an egg carton as shelter, wood, wool or toilet paper as nesting material and various enrichment such as running wheels or climbing frames. Woodchip bedding (Rettenmaier) was used to line cages. Water and food (Altromin Diet 1324) was given ad libitum. Housing rooms were maintained at 20–24°C and 50–65% humidity. All animal rooms received artificial light from 7 a.m. to 7 p.m. During experiments, mice experienced a natural dark-light cycle with additional artificial light from 8 a.m. to 4 p.m. Prior to the start of experiments, mice lived together in same-sex sibling groups of 2–5 animals. Three weeks before experiments, animals were separated into same-sex sibling pairs to have a similar group size per cage and allow for social contact between animals. At separation, all animals received an individual pit-Tag for permanent individual recognition. Therefore, an RFID chip (ISO Transponder, Planet ID, 1.4 × 9 mm) was inserted subcutaneously in the neck area. We separated animals later on in cases when severe fights occurred. This only happened in males of MMD (4 male-male pairs) and MMM (2 male-male pairs) subspecies.
Each 24 mice (12 males, 12 females) of M. spicilegus, A. uralensis, and each of six populations of M. musculus were used for behavioral testing. In addition, we measured maximum adult body mass and longevity (only in house mice) in these animals. Therefore, animals were maintained after the end of experiments until they died a natural death. However, to oblige animal welfare standards, animals were euthanized if signs of pain or suffering occurred. Additionally, we set up 8–29 monogamous breeding pairs of each species/population to obtain data on developmental life history traits such as litter sizes and weaning mass. The number of breeding pairs varies depending on the available number of unrelated animals.
Apodemus uralensis were originally caught in Kazakhstan where it occurred sympatrically with the M. musculus population and M. spicilegus were caught at different sites in Slovakia. Of the six house mice populations, each two represent a different subspecies (M. m. domesticus, M. m. musculus, and M. m. castaneus). M. m. domesticus populations (MMD) were originally caught in the central massif region in France or in the Khuzestan Province in Iran. M. m. musculus (MMM) populations originated from Austria and from Kazakhstan, where the population occurred sympatrically with A. uralensis. M. m. castaneus (MMC) populations originated in India and in Taiwan. While all M. musculus were caught in or close to human buildings, M. spicilegus and A. uralensis were never caught in human buildings but on agricultural fields. Detailed information including GPS locations of trapping sites can be found in Harr et al. (2016) and Vrbanec et al. (2021).
Behaviors
Three behavioral tests were conducted twice with an interval of 3–4 weeks between tests for each 24 mice per species/population. At the start of behavioral testing, mice were adult (∼6 months) and fully grown. Since all mice are nocturnal and/or crepuscular, all behavioral tests were conducted either between 7 and 10 a.m. or 4 and 8 p.m., when mice are still active or starting to get active again. To prevent any carry-over effects between animals sharing a cage and between experiments, only one animal per cage was tested per day and an animal only participated in one test per day.
Open Field Test
A mouse was placed into the center of the open field arena consisting of a 60 x 60 cm square with white, opaque walls. We recorded the distance the mouse moved as well as the percentage of time that was spent in the central area (more than 12 cm off the wall) within 5 min.
Elevated Plus Maze Test
A mouse was placed in the center of two crossed arms for 5 min. Two of the arms had a surrounding with dark gray opaque walls (=closed arms) while the other two arms were surrounded by transparent Plexiglas walls (=open arms). This modification was necessary because in pilot trials, wild mice frequently jumped from the open arms and escaped the test. We recorded the distance moved in the whole test setup and the percentage of time spent in the open arms.
To introduce mice into the Open Field or Elevated Plus Maze, we used tube handling to prevent any additional stress due to handling. Both tests were conducted in an experimental room under standard light conditions of 220 lux. Behaviors were video-recorded and analyzed using VideoMot2 (TSE). Apparatuses were cleaned with 70% ethanol between tests.
Novel Object Test
Two to ten days prior to the Novel Object Test, mice were changed into two standard cages that were connected by a tube so that animals could move freely between both sides. Directly before the test, animals living in a pair were gently guided so that each animal would be in one cage and the tube was closed. For animals that lived singly, we checked in which of the two connected cages it was and closed the tube. A novel object was introduced into each cage and the animals were left for 20 min to explore the objects. The objects were made from Lego bricks of different colors and were about as high as a mouse to catch its attention easily. Lego bricks were put into a different shape for the second trial. The experimental cages were covered with a Plexiglas lid and we recorded the latency to touch the object, the number of touches and the time spent interacting with the object.
Life History
From the animals used for the behavioral tests, we collected adult life history data such as adult body mass as well as longevity. Adult body mass was measured twice, before the start of behavioral tests and at the end of behavioral tests. Longevity is calculated as the number of days alive from birth to the natural death or the date of euthanization due to suffering (N = 43).
Litter size and body mass at weaning were measured in an independent set of animals from our in house population maintenance facility. Litter size, sex, and body mass of offspring were measured on the date of weaning at 27–37 days after birth. Weaning age was controlled for in the statistical analysis.
Statistics
Statistical analyses were carried out using R (R 4.0.1). We used the R package rptR package (Stoffel et al., 2017) to calculate repeatability of behavioral variables. We calculated a univariate model for every behavioral variable using trial (1st or 2nd) as fixed and animal ID as random effect. We calculated repeatability separately for M. musculus and the two non-commensal species. Initially, we assumed a Gaussian distribution. After checking model assumptions using qq-plots and plotting residual versus fitted values, we assumed a Poisson distribution for the number of exploration trips into the Novel environment while a Gaussian error distribution was assumed for all other variables.
To test if behavioral and life history variables differ between species, subspecies and/or populations, we used a sequential analysis for each variable. Wherever we had data for all three species (i.e., except longevity and weaning mass), we first calculated models including species, subspecies nested within species and sex as fixed effects. For data for which we only had one estimate per individual (e.g., litter size), we used linear models. For variables, for which we had multiple measurements per individual (e.g., behaviors), we calculated mixed effects models also including individual identity as a random effect (package lmerTest, Kuznetsova et al., 2017; RRID:SCR_015656). After this initial species analysis, we then calculated a second model separately for M. musculus, fitting population nested within subspecies and sex as fixed effects. For the other two species, we did not have multiple populations, so this step was not necessary there. As done already for repeatability analysis, we initially assumed a Gaussian distribution but changed to a Poisson distribution for the number of touches with a novel object for M. musculus.
Whenever a full model indicated a significant effect, we performed a post hoc comparison to test for pairwise differences using the package emmeans (Lenth et al., 2019, RRID:SCR_018734). The full statistical output of all models can be found in the Supplementary Material.
Ethics
Animal facilities are approved and regularly controlled for breeding and keeping mice by national authorities. All experimental procedures are approved by local authorities under license V244-19227/2019 (43-4/19). Experiments were conducted in accordance with all national and international guidelines for the use of animals in Research (ABS/ASAB guidelines).
Results
Does Living in Human-Altered Habitats Affect Behavior?
All behavioral variables except the latency to touch a novel object and the number of touches toward the novel were significantly repeatable, i.e., personality traits (see Table 1). The highest repeatability for all species is observed in the time spent in the center of the Open field. Variance components of between-individual and within-individual variance differed considerably between species and test situations but do not show any specific pattern of change depending on how long mice lived closely together with humans (for details see Supplementary Material 11).
The distance covered in the open field but not the time spent in the center showed a significant subspecies effect (F = 35.1, df = 2, p < 0.0001, see Supplementary Figures 1.4, 2.4). Populations nested within subspecies differed significantly from each other for both variables, indicating a larger difference between populations rather than subspecies (see Supplementary Figures 1.4, 2.4). MMD covered the most distance while MMM was intermediate and MMC covered the least distance. The two non-commensal species A. uralensis and M. spicilegus were intermediate for the distance covered and did not differ statistically from M. musculus. While Apodemus avoided the central area of the open field the most, M. spicilegus was again intermediate and did not differ from M. musculus significantly.
For the elevated plus-maze, we found a significant subspecies effect for the distance covered (F = 28.08, df = 2, p < 0.0001; see Supplementary Figure 3.4) as well as the time spent in the open arms (F = 7.36, df = 2, p = 0.0009, Figure 1). Again, however, populations nested within subspecies showed larger differences from each other than subspecies (see Supplementary Figure 3.4 and Supplementary Material 3). Both non-commensal species covered more distance in the elevated plus maze and spent more time in the open arms (Figure 1).
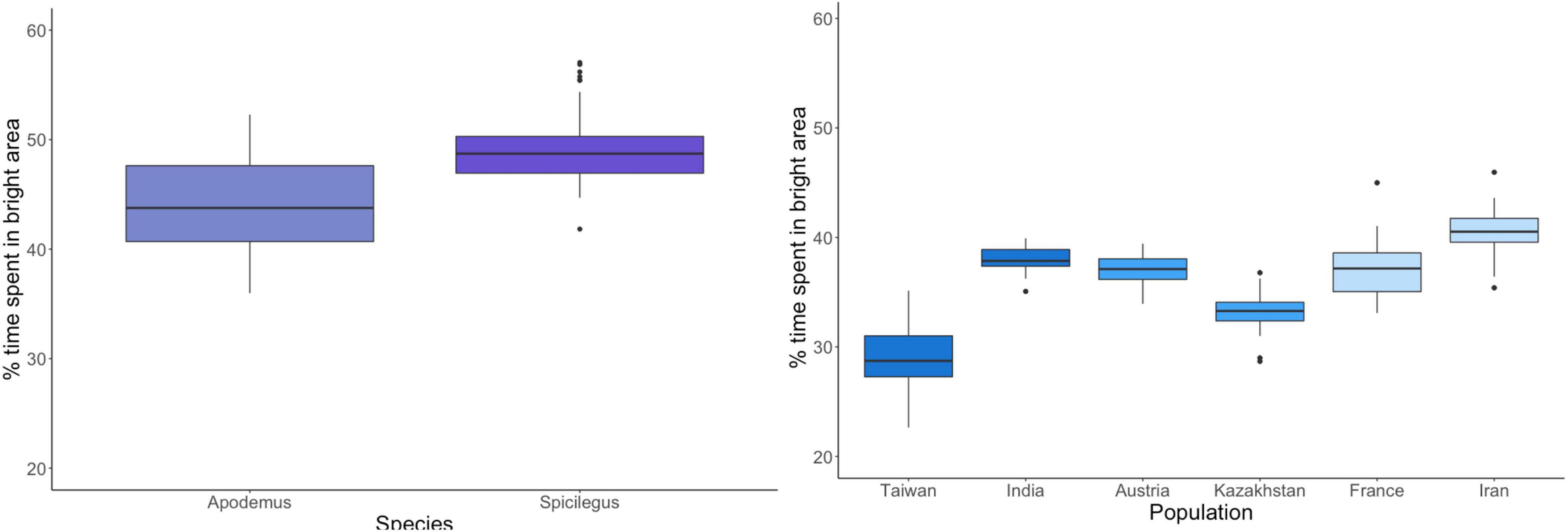
Figure 1. Percent of time spent in the bright area in elevated plus-maze. Species are depicted in different colors with non-commensal species in shades of violet and commensal house mouse subspecies are shown in blue. The two populations per subspecies are shown in the same color.
Regarding the latency to touch a novel object, we found a significant subspecies effect with most significant differences between the MMC and MMM (p = 0.0006), and MMD and MMM (p = 0.0007) subspecies (see Supplementary Figure 5.4). MMD were the slowest to approach the novel object while there was no difference between MMM and MMC. Both non-commensal species were faster to touch a novel object than M. musculus. When looking at the number of touches with the object, both non-commensal species did not differ from M. musculus (see Supplementary Figure 6). Within M. musculus, MMM populations touched the object most often and significantly more than MMC (MMM-MMC: p = 0.0001) and MMD (MMM-MMD: p < 0.0001), while no difference was found between MMC and MMD. The duration of interaction with the novel object differed strongly between populations (Figure 2) with no consistent pattern observed for subspecies. In addition, both non-commensal species were intermediate but showed very little variation in comparison with all M. musculus populations.
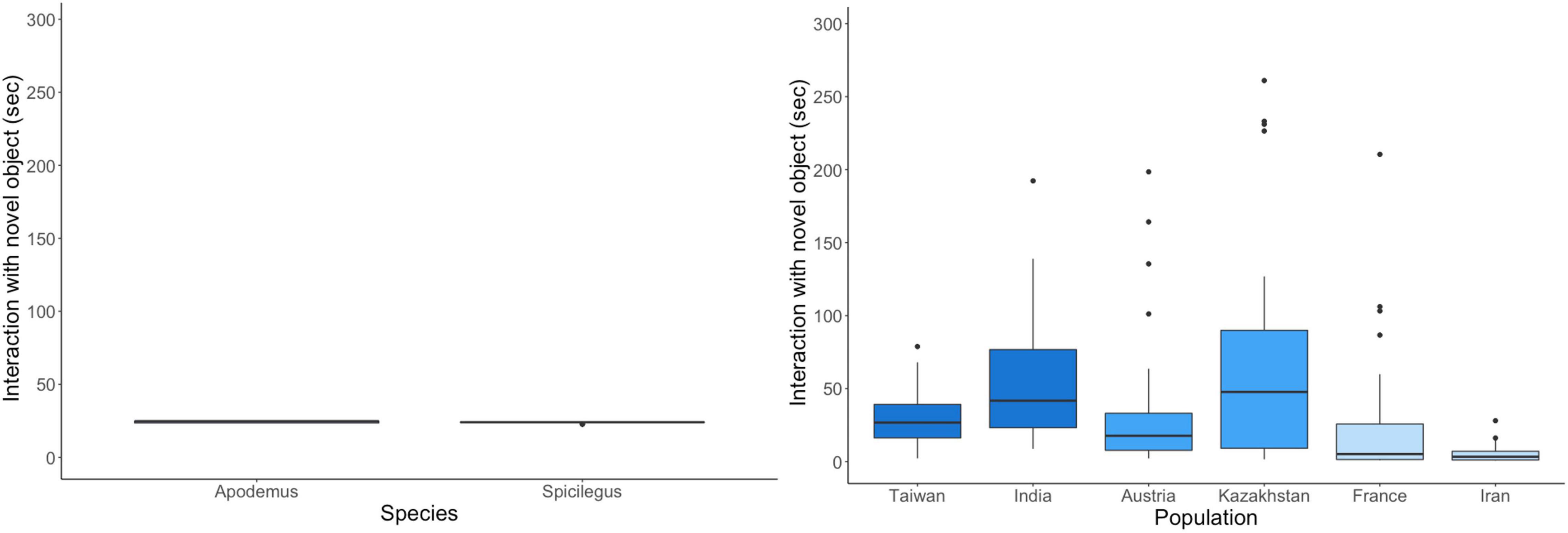
Figure 2. Duration of interaction with the novel object. Species are depicted in different colors with non-commensal species in shades of violet and commensal house mouse subspecies are shown in blue. The two populations per subspecies are shown in the same color.
A significant effect of sex was only observed for the distance covered in the open field (p = 0.0167) and the number of touches toward the novel objects (p = 0.0147).
Does Living in Human-Altered Habitats Affect Life History Traits?
Both MMM populations had on average larger litters than both MMD populations (p < 0.0001, Figure 3). MMC populations, however, did not differ statistically from MMM or MMD. The non-commensal A. uralensis showed an intermediate litter size while M. spicilegus had the overall highest litter sizes.
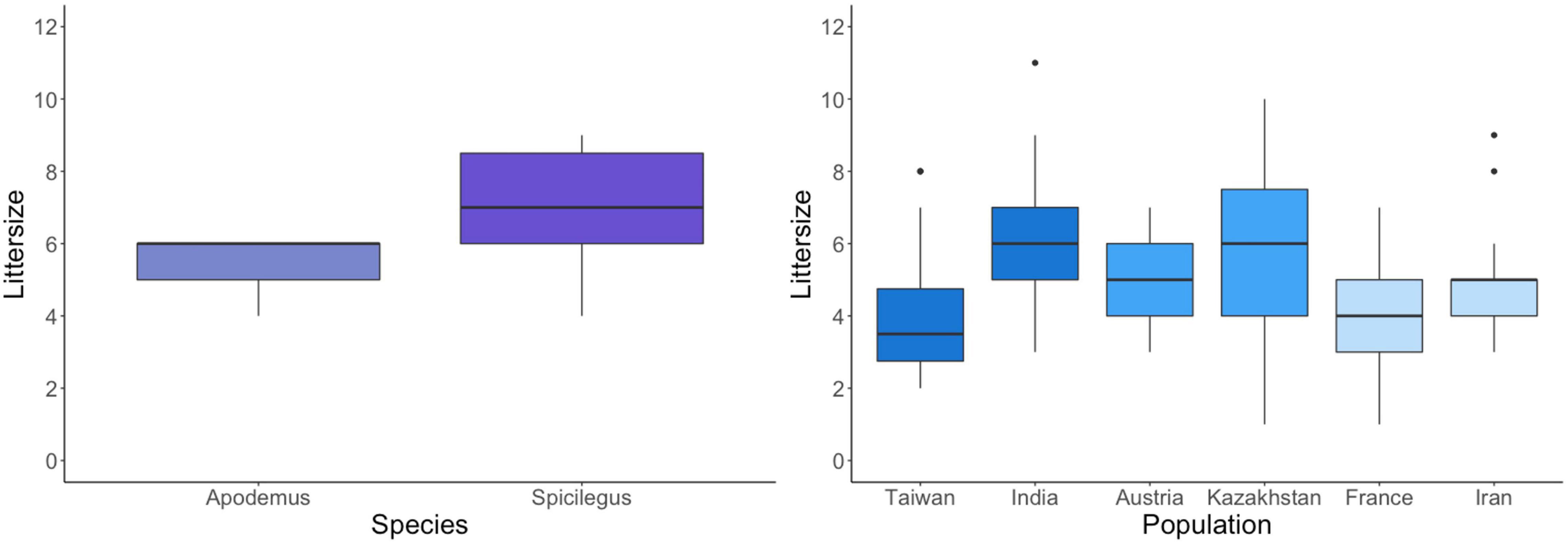
Figure 3. Littersize at weaning of the Mus musculus populations. The two populations per subspecies are shown in the same shade of blue.
Body mass at weaning differed between subspecies and populations. Subspecies that lived with humans for longer, weaned larger pups (p < 0.0001, Figure 4, Supplementary Material 8). Populations within subspecies differed for MMC (p < 0.0001) and MMM (p = 0.0001) and MMD (p < 0.0001).
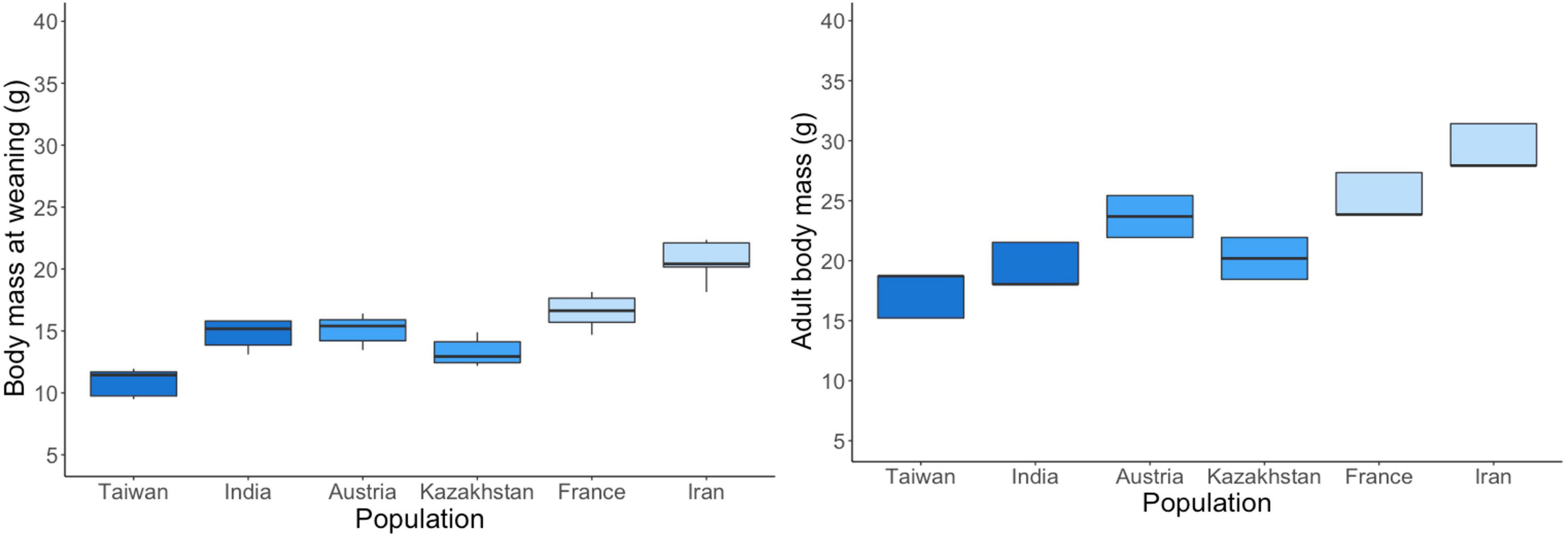
Figure 4. Body mass at weaning (28–30 days) and adulthood (6–12 months). The two populations per subspecies are shown in the same shade of blue.
Adult body mass did not differ statistically between subspecies (p = 0.1639, Supplementary Material 9) while populations within subspecies differed from each other for MMC (p = 0.0221) but not for MMM and MMD. With an average of 16.3 ± 0.36 g, M. spicilegus resembled the MMC population from Taiwan (the smallest animals) most closely (average body mass 16.3 ± 0.30 g) while A. uralensis (22.0 ± 0.84 g) showed an intermediate body mass.
Longevity did not differ between subspecies (F = 1.8, df = 2, p = 0.1653, Figure 5), however, populations within subspecies differed significantly from each other (F = 4.5, p = 0.0044). For the subspecies MMC, the population from India had a shorter life span compared to the population from Taiwan (t = –3.2, p = 0.0207). For MMM and MMD subspecies, the two populations did not differ from each other. Males and females had on average similar longevity (F = 2.0, df = 1, p = 0.1644). Longevity for M. spicilegus and A. uralensis is not available from our data but exceeds the average longevity of all M. musculus populations. Anecdotally, M. spicilegus is observed to frequently live longer than 4 years in our captive population.
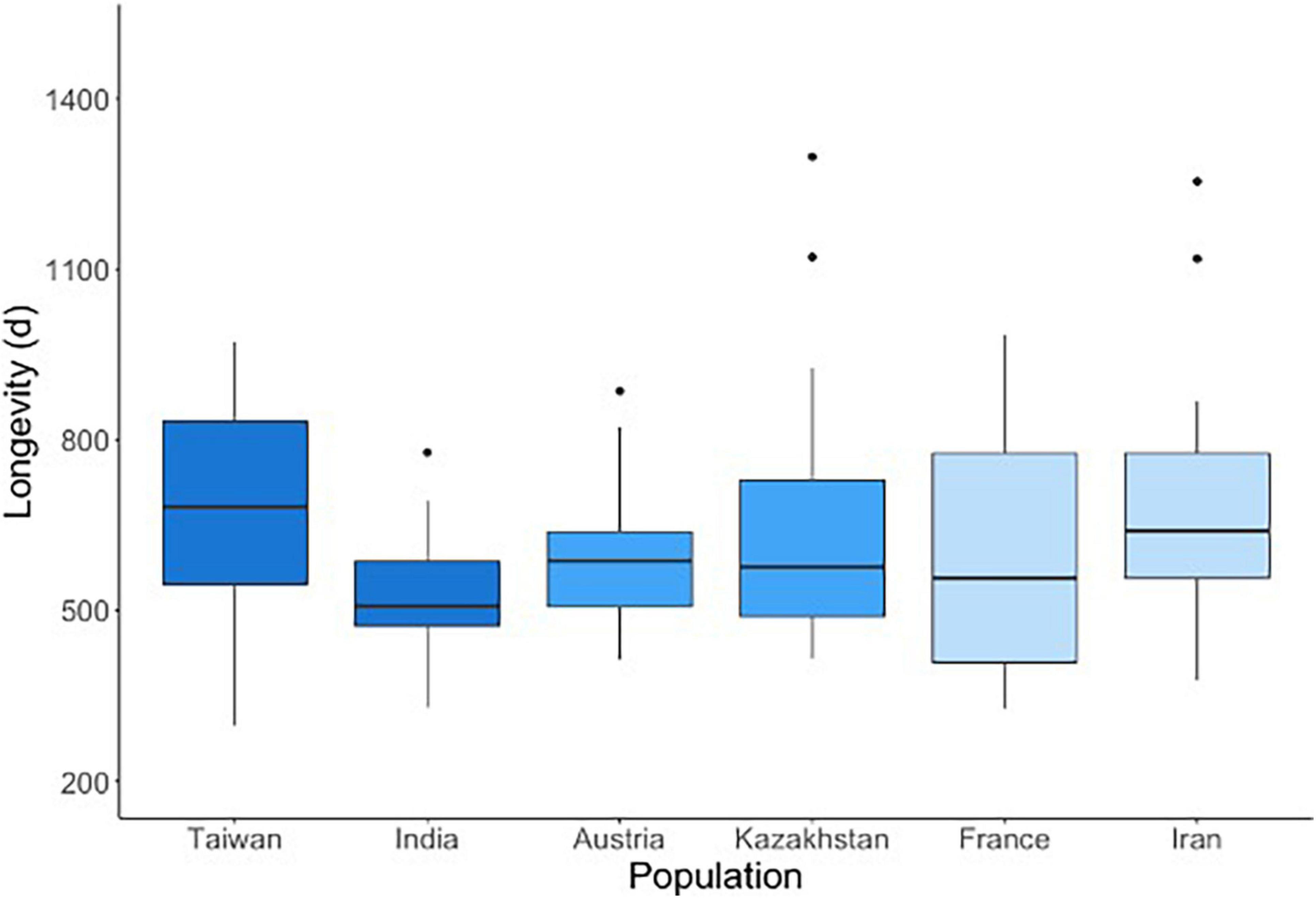
Figure 5. Longevity of Mus musculus populations. The two populations per subspecies are shown in the same shade of blue.
Discussion
In this study, we explored patterns in risk-taking, stress-coping and life history variation of three small rodent species for concordance with micro-evolutionary changes depending on how close their relationship with human habitats are. We found several differences on the species, subspecies, and population level, albeit no pattern that suggests an evolutionary adaptation to living in close proximity to humans.
On the species level, we did not find any conclusive evidence that small, muroid mammals evolved specific behavioral responses in stress-coping or risk-taking behaviors to adjust to a life close to humans. For the distance covered in an Open Field, a situation that is considered mildly stressful for rodents (Archer, 1973), both, A. uralensis and M. spicilegus covered intermediate distances compared to three different subspecies of M. musculus. Regarding the time spent in the central area, which reflects the avoidance of being in the open without cover and possibility for retreat to safety, A. uralensis showed the most avoidance and spent significantly less time in the central zone compared to M. spicilegus and M. musculus while there was no difference between M. spicilegus and M. musculus. This suggests an adaptation to the natural habitat of A. uralensis, which consists of shrublands and forest-edges and agricultural areas close to such vegetation, while M. spicilegus is more often found on open, agricultural areas and M. musculus is usually found within or in close proximity to human settlements. Initially, this may suggest that species that live closer to human settlements may show an altered behavioral pattern, but we would have expected a similar finding then for the Elevated Plus Maze, another mildly stressful situation for rodents. Here, however, both, A. uralensis and M. spicilegus tend to cover more distance compared to M. musculus and do not differ from each other. Also for the time spent in the bright arms of the Elevated Plus Maze, we found both non-commensal species to be intermediate with M. musculus and to not differ from each other. Likewise, A. uralensis and M. spicilegus did not differ in their responses to novelty and always overlapped with at least two of the M. musculus subspecies. Finding the three species to overlap strongly in many of the behavioral measures suggests only (if any) little phylogenetic influence. This would be in agreement with a recently published meta-analysis on behavioral responses toward human-induced rapid environmental change, which only found a phylogenetic signal for birds and for studies conducted in the wild (Gunn et al., 2021). However, some caution should be taken here since the number of studies on mammals was very small and in the present study, we also only used three species.
At the within-species level, we compared two geographically distant populations of the three most common subspecies of M. musculus each. While MMD already started to live with humans more than 10.000 years ago, MMC only started living with humans relatively recently (3.800–7.500 years ago) and MMM is intermediate between the two. While the subspecies significantly differed from each other for several behaviors such as the distance covered in the Open Field and the distance covered in the Elevated Plus Maze, we never found a directional change depending on the time that the subspecies lived with humans. In addition, for many behaviors, the two sampled populations within each subspecies also differed from each other considerably. These findings suggest no directed evolution of behavioral patterns to adjust to an urban-dwelling life-style, which is in contrast to an earlier study on problem-solving and cognitive flexibility in house mice (Vrbanec et al., 2021). In this study, the authors found a general pattern of a better evolved problem-solving ability reflecting cognitive flexibility in house mice populations that lived with humans longer.
Traits in general need to have a genetically determined and thus heritable basis to be able to influence evolutionary processes over long time scales. The behaviors we chose to study here are usually heritable across species (Stirling et al., 2002; Dochtermann et al., 2015) and especially for mice (DeFries et al., 1970; Rinaldi et al., 2001, although information is only available for inbred mouse strains). Repeatability, i.e., the proportion of among-individual variation divided by the total phenotypic variation, is often used to assess the upper boundary of heritability for any given trait (Falconer and Mackay, 1996). At the same time, repeatability reflects the potential for within-individual flexibility in reacting to environmental changes (Royauté and Dochtermann, 2017). Here, we found behaviors shown in the Open Field and Elevated Plus Maze as well as the duration of interaction with an unknown object to be repeatable in all three species with similar estimates and strongly overlapping confidence intervals. This indicates that the measured behaviors represent important and consistent traits in all tested species.
While we did not observe any directed changes in behavioral means in response to a longer association with humans, we interestingly observed a general pattern that the two non-commensal species show less variation in many traits compared to populations of M. musculus. This effect was consistent despite similar age at testing, similar number of animals and identical experimental conditions. This pattern was observed for the Open Field, Elevated Plus Maze, and Novel Object but not for life history traits, thus, indicating a general pattern for behavioral traits. Previous studies reported more variation in urban compared to rural populations measured under field conditions with unknown population history (e.g., Bókony et al., 2012; Dammhahn et al., 2020; von Merten et al., 2022). Variance of flight initiation distance was found to be initially reduced when birds colonize urban habitats (Carrete and Tella, 2011) because only a specific subset of phenotypes would lead the colonization process. During the following population establishment and increase, however, among-individual variation increased considerably and eventually became larger in urban populations (Møller, 2010). The original interpretation of this pattern was that urban populations gained variation in behavioral flexibility through adapting to urban habitats (Møller, 2010). A recent review by Thompson et al. (2022) found increased variance in morphological traits across 13 pairs of rural and urban tit pairs, suggesting that increased variance in urban populations is a consistent phenomenon. Whether such changes in variance are due to within-individual (i.e., flexibility) or between-individual (i.e., a wider spread of phenotypes) variation has not been investigated in many studies. A study conducted on common voles (Microtus arvalis) supports an enormous role of behavioral flexibility in adapting to human-altered habitats (Mazza et al., 2020). The authors found higher levels of risk-taking and exploration behavior in urban compared to rural populations when animals were directly tested after capture. After relocating animals to the laboratory and giving them 9–12 weeks habituation time, initial differences were lost due to a highly flexible adjustment in behavior of the urban animals (Mazza et al., 2020). Another study comparing rural with urban populations in two species of shrews found urban populations to harbor consistently more among-individual variance, i.e., found individuals to differ more from each other in urban habitats (von Merten et al., 2022). Here, we found different patterns for different traits. While, for example, behaviors measured in the Open Field mainly differed for between-individual variance when compared between species, behaviors measured in the Elevated Plus maze showed huge species differences for both variance components. Since our sample size is rather low to thoroughly compare variance components with each other, however, we suggest to interpret them cautiously. To disentangle effects of urbanization on behavioral variation and elucidate their potential ecological and evolutionary potential, future studies should focus on changes in population variance rather than just focusing on the population mean.
Life history traits related to survival and reproductive investment are also sometimes found to differ between rural and urban populations and a recent meta-analysis formally suggested that an urban-dwelling life-style should lead to a generally slower pace-of-life (Sepp et al., 2018). Traits indicative of a slow pace-of-life should include a slower growth, later onset of reproduction and less investment into reproduction at a given point in time. This combination of traits should then increase survival and potentially lead to a higher longevity (Stearns, 1992). In birds, meta-analytic records indeed support higher adult survival rates and smaller clutch sizes while no such information is yet available for other taxa (Sepp et al., 2018). Under natural conditions though, smaller clutches could also be the result of poorer nestling development and survival due to a constraint, non-optimal diet rather than reflecting an adaptation toward an urban-dwelling life-style (Peach et al., 2008; Seress et al., 2012). In the present study, we did not find evidence for a decrease in litter size within M. musculus subspecies, however, body mass at weaning increased in populations of subspecies which have lived with humans for longer. This might suggest that populations that have lived with humans for longer invest more into each single offspring even in the absence of the direct environmental influences and after having been bred under standardized laboratory conditions for several generations. Such a pattern suggests that the increased investment into single offspring might be an adaptive pattern rather than being a constraint due to poorer developmental conditions. On the other hand, we could not find the predicted increased longevity, thus, observed adult survival rates under natural conditions might more likely reflect benefits from better immune responses, less likelihood to fall prey or similar rather than reflecting a physiological adaptation for a longer life span. In fact, both non-commensal species we tested in our study, easily outlive the commensal house mice. One potentially important aspect we could not assess in our study is, however, the effect of the original social and breeding system of the mice. While M. spicilegus is a monogamous species and hence our breeding design reflected the natural situation of the species quite closely, no information on the natural breeding system of A. uralensis is available. It might thus be that the smaller observed litter size in A. uralensis is due to an enforced deviation from their optimal social and breeding system. Likewise, no information on the details of social and breeding systems of M. musculus subspecies is known. The social system of house mice is described as very flexible and adjusted to ecological and habitat characteristics specific populations face. We cannot fully exclude the possibility that aspects of our keeping and breeding design mask differences between species or subspecies because they might deviate from natural conditions more strongly for some species/populations than for others.
Taken together, our findings suggest no or only little micro-evolutionary change in specific behavioral or life history characteristics that is maintained under common-garden conditions across several generations in small rodents. Rather, the absence of an evolutionary adaptation to a life in human-altered habitats argues for an important role of fast and flexible adjustments of behavior and life history induced by different mechanisms of phenotypic plasticity as suggested by the “plasticity first” hypothesis (Levis and Pfennig, 2016; Perry et al., 2018).
Data Availability Statement
The original contributions presented in the study are included in the article/Supplementary Material, further inquiries can be directed to the corresponding author.
Ethics Statement
The animal study was reviewed and approved by Ministerium für Energiewende, Landwirtschaftliche Räume und Umwelt, Kiel.
Author Contributions
AG designed the study. Data curation and analysis were done by FK. Both authors wrote and edited the manuscript, contributed to the article, and approved the submitted version.
Funding
This project was funded by institutional resources of the Max Planck Society (AG). The funder had no influence on the experimental design or interpretation.
Conflict of Interest
The authors declare that the research was conducted in the absence of any commercial or financial relationships that could be construed as a potential conflict of interest.
Publisher’s Note
All claims expressed in this article are solely those of the authors and do not necessarily represent those of their affiliated organizations, or those of the publisher, the editors and the reviewers. Any product that may be evaluated in this article, or claim that may be made by its manufacturer, is not guaranteed or endorsed by the publisher.
Acknowledgments
We thank Vanja Matijević and Kübra Bingül as well as Milan Jovicić and Janine Dose for help in collecting the behavioral data. Furthermore, we thank the whole Mausteam of the MPI Plön for helping to collect the life history data of our mice and for mice breeding.
Supplementary Material
The Supplementary Material for this article can be found online at: https://www.frontiersin.org/articles/10.3389/fevo.2022.892752/full#supplementary-material
References
Archer, J. (1973). Tests for emotionality in rats and mice: a review. Anim. Behav. 21, 205–235. doi: 10.1016/s0003-3472(73)80065-x
Banks, P. B., and Smith, H. M. (2015). The ecological impacts of commensal species: black rats, Rattus rattus, at the urban–bushland interface. Wildlife Res. 42, 86–97.
Bartmann, S., and Gerlach, G. (2001). Multiple paternity and similar variance in reproductive success of male and female wood mice (Apodemus sylvaticus) housed in an enclosure. Ethology 107, 889–899.
Bókony, V., Kulcsár, A., Tóth, Z., and Liker, A. (2012). Personality traits and behavioral syndromes in differently urbanized populations of house sparrows (Passer domesticus). PLoS One 7:e36639. doi: 10.1371/journal.pone.0036639
Bonhomme, F., and Searle, J. B. (2012). “House mouse phylogeography,” in Evolution of the House Mouse, eds M. Macholán, S. J. E. Baird, P. Munclinger, and J. Piálek (Cambridge: Cambridge University Press), 278–296.
Boutin, S., and Lane, J. E. (2014). Climate change and mammals: evolutionary versus plastic responses. Evol. Appl. 7, 29–41. doi: 10.1111/eva.12121
Carrete, M., and Tella, J. L. (2011). Inter-individual variability in fear of humans and relative brain size of the species are related to contemporary urban invasion in birds. PLoS One 6:e18859. doi: 10.1371/journal.pone.0018859
Cichocki, J., Ruprecht, A. L., and Ważna, A. (2011). Distribution of pygmy field mouse Apodemus uralensis (Pallas, 1811) population in Poland: review of the studies and new data. Fragmenta Faunistica 54, 77–85. doi: 10.3161/00159301ff2011.54.1.077
Cucchi, T., Auffray, J., and Vigne, J. (2012). “History of house mouse synanthropy and dispersal in the near east and europe: zooarcheological review and perspectives,” in Evolution of the House Mouse, eds M. Macholán, S. J. Baird, P. Munclinger, and J. Piálek (Cambridge: Cambridge University Press), 65–93.
Dammhahn, M., Mazza, V., Schirmer, A., Göttsche, C., and Eccard, J. A. (2020). Of city and village mice: behavioural adjustments of striped field mice to urban environments. Sci. Rep. 10, 1–12. doi: 10.1038/s41598-020-69998-6
DeFries, J. C., Wilson, J. R., and McClearn, G. E. (1970). Open-field behavior in mice: selection response and situational generality. Behav. Genet. 1, 195–211. doi: 10.1007/BF01074652
Denys, C., Taylor, P. J., and Aplin, K. P. (2017). “Family muridae (true mice and rats, gerbils and relatives),” in Handbook of the Mammals of the World, eds D. Wilson, T. Lacher, and R. Mittermeier (Barcelona: Lynx Edicions), 536–886. doi: 10.1111/j.1749-6632.1999.tb07798.x
Dochtermann, N. A., Schwab, T., and Sih, A. (2015). The contribution of additive genetic variation to personality variation: heritability of personality. Proc. R. Soc. B Biol. Sci. 282:20142201. doi: 10.1098/rspb.2014.2201
Frynta, D., Kaftanová-Eliášová, B., Žampachová, B., Voráčková, P., Sádlová, J., and Landová, E. (2018). Behavioural strategies of three wild-derived populations of the house mouse (Mus m. musculus and M. m. domesticus) in five standard tests of exploration and boldness: searching for differences attributable to subspecies and commensalism. Behav. Process. 157, 133–141. doi: 10.1016/j.beproc.2018.09.008
Gross, M. (2018). Adapting to life in the city. Curr. Biol. 28, R635–R638. doi: 10.1016/j.cub.2018.05.043
Gunn, R. L., Hartley, I. R., Algar, A. C., Niemelä, P. T., and Keith, S. A. (2021). Understanding behavioural responses to human-induced rapid environmental change: a meta-analysis. Oikos, 2022:e08366.
Harr, B., Karakoc, E., Neme, R., Teschke, M., Pfeifle, C., Pezer, Z., et al. (2016). Genomic resources for wild populations of the house mouse, Mus musculus and its close relative Mus spretus. Sci. Data 3, 1–14. doi: 10.1038/sdata.2016.75
Hwang, C. C., and Turner, B. D. (2009). Small-scaled geographical variation in life-history traits of the blowfly Calliphora vicina between rural and urban populations. Entomol. Exp. Appl. 132, 218–224. doi: 10.1111/j.1570-7458.2009.00891.x
Johnson, M. T., and Munshi-South, J. (2017). Evolution of life in urban environments. Science 358, eaam8327. doi: 10.1126/science.aam8327
Kuznetsova, A., Brockhoff, P. B., and Christensen, R. H. B. (2017). lmerTest package: tests in linear mixed effects models. J. Stat. Softw. 82, 1–26. doi: 10.18637/JSS.V082.I13
Levis, N. A., and Pfennig, D. W. (2016). Evaluating “plasticity-first” evolution in nature: key criteria and empirical approaches. Trends Ecol. Evol. 31, 563–574. doi: 10.1016/j.tree.2016.03.012
Lowry, H., Lill, A., and Wong, B. B. M. (2013). Behavioural responses of wildlife to urban environments. Biol. Rev. 88, 537–549. doi: 10.1111/brv.12012
Mazza, V., Dammhahn, M., Lösche, E., and Eccard, J. A. (2020). Small mammals in the big city: Behavioural adjustments of non-commensal rodents to urban environments. Glob. Change Biol. 26, 6326–6337. doi: 10.1111/gcb.15304
McKinney, M. L. (2002). Urbanization, biodiversity, and conservation: the impacts of urbanization on native species are poorly studied, but educating a highly urbanized human population about these impacts can greatly improve species conservation in all ecosystems. BioScience 52, 883–890.
Miranda, A. C. (2017). “Mechanisms of behavioural change in urban animals: the role of microevolution and phenotypic plasticity,” in Ecology And Conservation Of Birds In Urban Environments, eds E. Murgui and M. Hedblom (Berlin: Springer), 113–132.
Miranda, A. C., Schielzeth, H., Sonntag, T., and Partecke, J. (2013). Urbanization and its effects on personality traits: a result of microevolution or phenotypic plasticity? Glob. Change Biol. 19, 2634–2644. doi: 10.1111/gcb.12258
Møller, A. P. (2010). Interspecific variation in fear responses predicts urbanization in birds. Behav. Ecol. 21, 365–371. doi: 10.1093/beheco/arp199
Nicoglou, A. (2015). “Phenotypic plasticity: from microevolution to macroevolution,” in Handbook of Evolutionary Thinking in the Sciences, eds G. Lecointre, M. Silberstein, P. Huneman, and T. Heams (Dordrecht: Springer Netherlands), 285–318. doi: 10.1007/978-94-017-9014-7_14
Peach, W. J., Vincent, K. E., Fowler, J. A., and Grice, P. V. (2008). Reproductive success of house sparrows along an urban gradient. Anim. Conserv. 11, 493–503. doi: 10.1111/j.1469-1795.2008.00209.x
Perry, B. W., Schield, D. R., and Castoe, T. A. (2018). Evolution: plasticity versus Selection, or Plasticity and Selection? Curr. Biol. 28, R1104–R1106. doi: 10.1016/j.cub.2018.07.050
Pockock, M. J. O., Hauffe, H. C., and Searle, J. B. (2005). Dispersal in house mice. Biol. J. Linnean Soc. 84, 565–583.
Reader, S. (2003). Innovation and social learning: individual variation and brain evolution. Anim. Biol. 53, 147–158. doi: 10.1163/157075603769700340
Rinaldi, D., Larrigaldie, V., Chapouthier, G., and Martin, B. (2001). Unexpected absence of correlation between the genetic mechanisms regulating β-carboline-induced seizures and anxiety manifested in an elevated plus-maze test. Behav. Brain Res. 125, 159–165. doi: 10.1016/s0166-4328(01)00293-5
Royauté, R., and Dochtermann, N. A. (2017). When the mean no longer matters: developmental diet affects behavioral variation but not population averages in the house cricket (Acheta domesticus). Behav. Ecol. 28, 337–345. doi: 10.1093/beheco/arw164
Sepp, T., McGraw, K. J., Kaasik, A., and Giraudeau, M. (2018). A review of urban impacts on avian life-history evolution: does city living lead to slower pace of life? Glob. Change Biol. 24, 1452–1469. doi: 10.1111/gcb.13969
Seress, G., Bókony, V., Pipoly, I., Szép, T., Nagy, K., and Liker, A. (2012). Urbanization, nestling growth and reproductive success in a moderately declining house sparrow population. J. Avian Biol. 43, 403–414. doi: 10.1111/j.1600-048X.2012.05527.x
Sih, A. (2013). Understanding variation in behavioural responses to human-induced rapid environmental change: a conceptual overview. Anim. Behav. 85, 1077–1088. doi: 10.1016/j.anbehav.2013.02.017
Sih, A., Stamps, J., Yang, L. H., McElreath, R., and Ramenofsky, M. (2010). Behavior as a key component of integrative biology in a human-altered world. Integr. Comp. Biol. 50, 934–944. doi: 10.1093/icb/icq148
Sol, D., Maspons, J., Gonzalez-Voyer, A., Morales-Castilla, I., Garamszegi, L. Z., and Moller, A. P. (2018). Risk-taking behavior, urbanization and the pace of life in birds. Behav. Ecol. Sociobiol. 72, 1–9.
Stirling, D. G., Réale, D., and Roff, D. A. (2002). Selection, structure and the heritability of behaviour. J. Evol. Biol. 15, 277–289. doi: 10.1046/j.1420-9101.2002.00389.x
Stoffel, M. A., Nakagawa, S., and Schielzeth, H. (2017). rptR: repeatability estimation and variance decomposition by generalized linear mixed-effects models. Methods Ecol. Evol. 8, 1639–1644. doi: 10.1111/2041-210X.12797
Suzuki, H., Nunome, M., Kinoshita, G., Aplin, K. P., Vogel, P., Kryukov, A. P., et al. (2013). Evolutionary and dispersal history of Eurasian house mice Mus musculus clarified by more extensive geographic sampling of mitochondrial DNA. Heredity 111, 375–390. doi: 10.1038/hdy.2013.60
Szulkin, M., Munshi-South, J., and Charmantier, A. (2020). “Introduction,” in Urban Evolutionary Biology, eds A. Charmantier, J. Munshi-South, and M. Szulkin (Oxford: Oxford University Press), 1–12. doi: 10.1093/oso/9780198836841.003.0001
Thompson, M. J., Capilla-Lasheras, P., Dominoni, D. M., Réale, D., and Charmantier, A. (2022). Phenotypic variation in urban environments: mechanisms and implications. Trends Ecol. Evol. 37, 171–182. doi: 10.1016/j.tree.2021.09.009
Tryjanowski, P., Møller, A. P., Morelli, F., Biaduń, W., Brauze, T., Ciach, M., et al. (2016). Urbanization affects neophilia and risk-taking at bird-feeders. Sci. Rep. 6, 1–7. doi: 10.1038/srep28575
von Merten, S., Oliveira, F. G., Tapisso, J. T., Pustelnik, A., da Luz Mathias, M., and Rychlik, L. (2022). Urban populations of shrews show larger behavioural differences among individuals than rural populations. Anim. Behav. 187, 35–46.
Keywords: animal personality, rodents, commensalism, behavioral adaptation, human-induced environmental change, HIREC
Citation: Küçüktaş FM and Guenther A (2022) Does Living in Human-Altered Environments Affect Life-History and Personality of Wild Mice? Front. Ecol. Evol. 10:892752. doi: 10.3389/fevo.2022.892752
Received: 09 March 2022; Accepted: 11 May 2022;
Published: 07 June 2022.
Edited by:
Tasmin Rymer, James Cook University, AustraliaReviewed by:
Melanie Dammhahn, University of Veterinary Medicine Vienna, AustriaBarbara Koenig, University of Zurich, Switzerland
Copyright © 2022 Küçüktaş and Guenther. This is an open-access article distributed under the terms of the Creative Commons Attribution License (CC BY). The use, distribution or reproduction in other forums is permitted, provided the original author(s) and the copyright owner(s) are credited and that the original publication in this journal is cited, in accordance with accepted academic practice. No use, distribution or reproduction is permitted which does not comply with these terms.
*Correspondence: Anja Guenther, guenther@evolbio.mpg.de