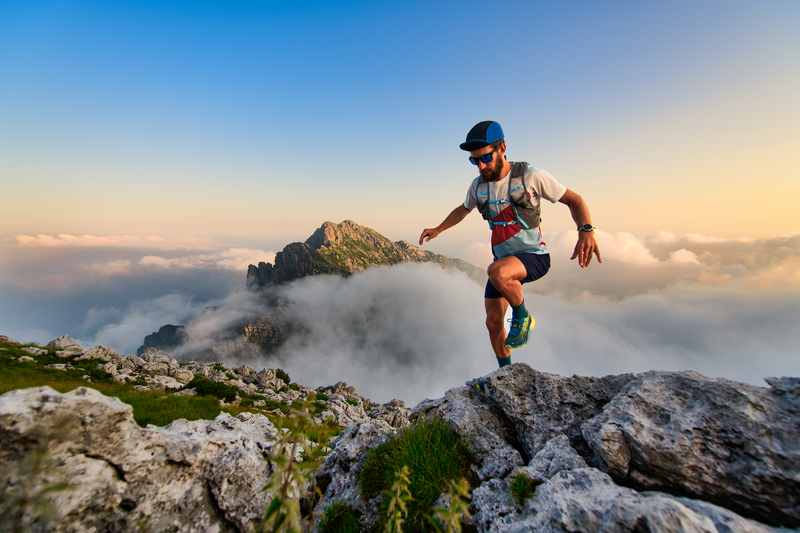
95% of researchers rate our articles as excellent or good
Learn more about the work of our research integrity team to safeguard the quality of each article we publish.
Find out more
MINI REVIEW article
Front. Ecol. Evol. , 15 June 2022
Sec. Population, Community, and Ecosystem Dynamics
Volume 10 - 2022 | https://doi.org/10.3389/fevo.2022.884159
This article is part of the Research Topic Sex Ratios in the Anthropocene View all 6 articles
Insects have evolved highly diverse genetic sex-determination mechanisms and a relatively balanced male to female sex ratio is generally expected. However, selection may shift the optimal sex ratio while meiotic drive and endosymbiont manipulation can result in sex ratio distortion (SRD). Recent advances in sex chromosome genomics and CRISPR/Cas9-mediated genome editing brought significant insights into the molecular regulators of sex determination in an increasing number of insects and provided new ways to engineer SRD. We review these advances and discuss both naturally occurring and engineered SRD in the context of the Anthropocene. We emphasize SRD-mediated biological control of insects to help improve One Health, sustain agriculture, and conserve endangered species.
Sex of an insect is determined by the chromosome complement it inherits from its parents. The chromosome systems that underly genetic sex-determination are quite diverse among insect species (Bachtrog et al., 2014; Beukeboom and Perrin, 2014; Biedler and Tu, 2016). Flies and mosquitoes are among the many insects that evolved the XX/XY sex chromosome system, where the heterogametic (XY) individuals are males and the homogametic (XX) individuals are females. Lepidopterans such as the silkworm, Bombyx mori, evolved the ZZ/ZW sex chromosome system where ZZ males are the homogametic sex while heterogametic ZW individuals are females. Hemizygous sex chromosome systems are also found including XX/XO in several insect orders, where the males are hemizygous as they only have one X chromosome (XO); and ZZ/ZO in some lepidopteran and tricopteran species, where the females are hemizygous (ZO). Although species within an insect order tend to share the same type of sex chromosome system, variations can occur within an order or even a family. In the aforementioned systems, the sex of an offspring is determined by the genotype of the gamete of the heterogametic or hemigametic parent. In Hymenopteran and Thysanopteran insects, however, sex is instead determined by the haplodiploidy of the individual, where fertilized diploid eggs (2n) develop to females while unfertilized haploid eggs (n) develop as males (reviewed in Beukeboom and Perrin, 2014; Biedler and Tu, 2016).
In contrast to the apparent plasticity and diversity of the sex-determining chromosome systems, two highly conserved transcription factors doublesex (dsx) and fruitless (fru) control the development of sexual dimorphism in all insects studied thus far (Herpin and Schartl, 2015; Biedler and Tu, 2016; Hopkins and Kopp, 2021). Sex-specific isoforms of the DSX and FRU proteins, which result from sex-specific splicing of their primary RNA transcripts, program sexual differentiation (Figure 1). Therefore, sex determination is a process in which the primary sex-specific signals, which reflect the sex-specific chromosome composition of the early embryo, are transduced in a signal cascade to modulate sex-specific splicing of the RNA transcripts of dsx and fru. Figure 1 presents two simplified models using dsx as an example. In the vinegar fly Drosophila melanogaster, the double dosage of the X chromosome in the females (XX) initiates the transcription of the sex lethal (sxl) gene in the early embryo, leading to the production of the primary signal which is the SXL protein (Figure 1A). The presence of SXL leads to the production of a functional protein isoform of transformer (TRA), which in turn enables female-specific splicing of the primary RNA transcripts of dsx and fru. In the male (XY) embryo, the single X chromosome fails to initiate the production of the primary signal SXL, leading to the default male-specific splicing of dsx and fru transcripts and hence the production of male-specific DSX and FRU protein isoforms. In the Mediterranean fruit fly Ceratitis capitata, however, the default sex is female (Figure 1B). The primary signal, a male-determining factor (M factor) MoY (Meccariello et al., 2019), resides on the Y chromosome. The presence of the M factor in the early male (XY) embryos results in male-specific splicing of the tra pre-mRNA, leading to the production of a non-functional TRA protein and subsequently, male-specific splicing of dsx and fru. The lack of the Y chromosome in females (XX) enables the TRA protein complex to catalyze the female-specific splicing of dsx and fru. The TRA intermediate evolved much faster than DSX and FRU and TRA is not found in all insects (Biedler and Tu, 2016). Recent advances have brought significant molecular insights into the diverse mechanisms of sex determination in an increasing number of insects (e.g., Kiuchi et al., 2014; Hall et al., 2015, 2016; Criscione et al., 2016; Krzywinska et al., 2016, 2021; Sharma et al., 2017; Meccariello et al., 2019; Qi et al., 2019; Wexler et al., 2019; Aryan et al., 2020; Liu et al., 2020; Zou et al., 2020; Lutrat et al., 2021; Zhuo et al., 2021).
Figure 1. Simplified models of the sex-determination pathways in the vinegar fly Drosophila melanogaster (A) and the Mediterranean fruit fly Ceratitis capitata (B), and meiotic sex ratio distortion (C). (A) In D. melanogaster, embryos that inherit two X chromosomes (depicted as red DNA molecules) express the primary signal Sex-lethal (SXL) which effects the female-specific splicing of the transformer (tra) transcript, leading to the production of a functional TRA protein (TRAF). The TRAF protein complex enables the female-specific splicing of the doublesex pre-mRNA, leading to the production of the female DSX protein isoform (DSXF) which programs female differentiation (left). In contrast, embryos with a single X chromosome do not express a functional SXL, resulting in a truncated non-functional TRA (TRAM) and subsequently the default male-specific splicing of dsx. This leads to the production of DSXM, which programs male differentiation (right). The Y chromosome (depicted as a blue DNA molecule) does not directly participate in sex-determination. (B) In C. capitata, a dominant male-determining factor, Maleness-on-the-Y (MoY) resides on the Y chromosome (in blue). Expression of MoY somehow induces male-specific splicing of the tra pre-mRNA, leading to the production of truncated and non-functional TRA proteins (TRAM). The lack of a functional TRA results in male DSXM isoform and male differentiation (left). Embryos that do not inherit the Y chromosome (or MoY) produce a functional TRA protein (TRAF) complex by default, which leads to the production of DSXF and female differentiation (right). See Meccariello et al. (2019) and Primo et al. (2020) for details and for the concept of an autoregulatory loop. (C) Normal spermatogenesis of a heterogametic male with XY chromosomes will produce X- or Y-bearing sperms in equal proportion (left). In a hypothetical Y-linked X-shredder system (right), either natural or engineered, double-stranded DNA breaks along the X chromosome could lead to non-functional X-bearing sperms without affecting the Y chromosome-bearing sperms. As a result, sex ratio distortion will occur in the progeny of an affected heterogametic male (XY).
A relatively balanced male to female sex ratio is expected for most insects. However, selection may shift the optimal sex ratios and meiotic drive and microbial manipulation can result in sex ratio distortion (SRD). In the following sections, we will review both naturally occurring and engineered SRD in the context of developing biological control of insects to help improve One Health, sustain agriculture, and conserve endangered species.
Gene drive refers to the phenomena in which one of the homologous chromosome pair, or an allele on one of the homologous chromosome pair, is transmitted to the next generation at a frequency greater than the expected 50% Mendelian segregation. If this bias results from a bias in the representation of gametes of a certain chromosome or genotype during meiosis (Figure 1C), then it is a meiotic drive. If this bias involves the sex chromosomes during meiosis in the heterogametic sex (e.g., during spermatogenesis in XY males), SRD will ensue. Meiotic and sex-linked drives have been discussed in a number of reviews including Jaenike (2001) and Courret et al. (2019). We will highlight a few examples emphasizing the concept and recent advances (Table 1). Meiotic drives typically involve two loci: a drive allele/locus and a drive-sensitive allele targeted by the drive locus (Lyttle, 1991). For example, the D. simulans Winters drive is comprised of the Distorter on the X (Dox) that targets Y-chromosome repeats and kills the Y-bearing sperm, leading to a female sex ratio bias (Tao et al., 2007a,b). Two autosomal suppressor alleles were found that counteract the drive (Tao et al., 2001, 2007a) and suppress Dox via RNA interference (Lin et al., 2018). The intragenomic arms race between an SRD drive and its suppressor and resistance alleles may have resulted in cryptic SRDs that are derived from multiple waves of SRD invasion followed by suppression and/or resistance (e.g., Muirhead and Presgraves, 2021). In Aedes aegypti mosquitoes a Y-linked distorter locus (D) is thought to disrupt the formation of the X-bearing sperm by causing X-chromosome breakage mainly at one of four sites during male meiosis I (Craig et al., 1960; Newton et al., 1976). Thus, D increases its own transmission and causes male-biased sex distortion (Figure 1C). This male bias is especially attractive in the context of controlling mosquito-borne infectious diseases as only female mosquitoes bite and transmit disease-causing pathogens. Suppressor alleles, resistant X chromosomes, and distortion enhancers associated with the D drive have been reported in various laboratory strains and wild populations (Wood, 1976; Suguna et al., 1977; Wood and Ouda, 1987; Owusu-Daaku et al., 1997). The Ae. aegypti Y chromosome is also called the M chromosome and it is similar to the X chromosome (or the m chromosome) except for its male-determining locus M (Matthews et al., 2018). Strictly speaking, these M- and m-bearing chromosomes are homomorphic sex-determining chromosomes.
Bacterial endosymbionts such as Wolbachia, Rickettsia, Cardinium and Spiroplasma can infect and manipulate the germline of insects that harbor them (reviewed in Werren et al., 2008; Ma et al., 2014; Hurst and Frost, 2015; Landmann, 2019). They are normally transmitted through the maternal germline. Wolbachia is thought to infect more than half of all arthropod species (Weinert et al., 2015) and is well known for its induction of cytoplasmic incompatibility (CI) and CI-related applications in pest control (e.g., Laven, 1967; Dobson et al., 2002; Zheng et al., 2019). Wolbachia can cause SRD by feminizing or killing males, inducing parthenogenesis, and regulating sex allocation. For example, Wolbachia can cause damage in the dosage compensated X chromosome that is only found in males to confer male-specific lethality in Drosophila (Harumoto et al., 2018). Wolbachia can also feminize and kill males by mis-regulating the factors in the sex determination pathway to shift dsx splicing (Sugimoto and Ishikawa, 2012). Wolbachia in female Eurema mandarina butterflies (genotype ZW) prevents the production of Z-bearing oocytes during oogenesis and initiates female development in ZO individuals, resulting in all-female offspring through a dual-acting meiotic drive (Kern et al., 2015; Kageyama et al., 2017). Wolbachia and other intracellular symbionts can also mediate higher fertilization rates leading to a female bias in haplodiploid species (Wang et al., 2020; Bagheri et al., 2022).
Altering or perturbing factors involved in sex-determination (Figures 1A,B) could either result in sex conversion, or sex-specific lethality, or infertile intersex, depending on the relative position of the factor in the sex-determination pathway (i.e., top master switch such as SXL or MoY versus bottom effector such as DSX) and whether or not sex chromosome dosage compensation is required (reviewed in Biedler and Tu, 2016; Scott, 2021). Table 1 lists a number of candidates that can be manipulated to cause SRD in diverse insect species. We will only highlight a few recent examples in which SRDs were demonstrated over many generations when the factors were stably inherited as transgenes. Stable expression of a transgenic copy of Nix, a master switch for male determination in Ae. aegypti and Ae. albopictus (Hall et al., 2015), converted genetic females into fertile males and resulted in a clear SRD (Adelman and Tu, 2016; Aryan et al., 2020; Lutrat et al., 2021). On the other hand, stable germline transformation of a Y-linked primary signal Guy1 resulted in female-specific lethality in An. stephensi (Criscione et al., 2016). Unlike Ae. aegypti, X chromosome dosage compensation is needed in An. stephensi (Jiang et al., 2015) and Guy1 regulates dosage compensation by increasing the transcription of genes on the X chromosome in XY males (Qi et al., 2019). Transgenic expression of Guy1 in XX females result in abnormally high transcription of X-linked genes and hence lethality (Qi et al., 2019). Targeting the sex-specific exon (or its splicing signal) of dsx, a gene at the bottom of the sex-determination pathway, could also result in SRD. A gene drive was developed in An. gambiae that disrupts the formation of female dsxF transcript while leaving the male dsxM unaffected (Kyrou et al., 2018, see below for details), resulting in sterile intersex XX individuals without affecting the development or fertility of XY males. In addition to genetic manipulations mentioned above, SRD can also be achieved by silencing genes in the sex-determination pathway using interfering RNA (e.g., Pane et al., 2002; Whyard et al., 2015; Meccariello et al., 2019; Taracena et al., 2019). Other sex-specific phenomena can also be used to engineer SRD (e.g., Fu et al., 2010; Kandul et al., 2020; Li et al., 2021).
An engineered X-shredder was developed in the malaria mosquito An. gambiae that uses an endonuclease I-PpoI to target ribosomal DNA repeats exclusive to the X chromosome to induce X chromosome breakage during spermatogenesis (Galizi et al., 2014). This results in the reduction of X-bearing sperm and > 95% male progeny. Releasing this X-shredder mosquito strain successfully suppressed a cage population, confirming its potential for genetic control. Unlike the D-locus in Ae. aegypti which is Y-linked and thus favors its own transmission at the expense of the X chromosome, the I-PpoI X-shredder is on an autosome and is transmitted at a 50% probability. Attempts to engineer a more powerful Y-linked sex ratio distorter have not been successful presumably due to inactivation of the Y-linked distorter transgene during meiosis (Turner, 2007; Taxiarchi et al., 2019; Alcalay et al., 2021). As an alternative, the I-PpoI X-shredder in An. gambiae was integrated into a CRISPR/Cas9-based gene drive (Simoni et al., 2020) that targets the female doublesex (dsx) isoform as previously described (Kyrou et al., 2018). This new strain produces male-only progeny and eventually crashed cage populations in only 10–14 generations with a starting gene drive frequency as low as 2.5%, demonstrating the potential for large-scale applications (Simoni et al., 2020). Large cage trials that incorporated mosquito ecology showed further promise as the dsx gene drive suppressed the mosquito population within a year without selecting for resistance to the drive (Hammond et al., 2021).
An X-shedder was also developed using an RNA-guided CRISPR/Cas9 nuclease to target the X chromosome in An. gambiae (Galizi et al., 2016). The use of a programmable CRISPR/Cas9 nuclease has facilitated the development of X-shredders in other insect species including D. melanogaster (Fasulo et al., 2020) and C. capitata (Meccariello et al., 2021). All that are required are guide RNAs that target X-specific sequences/repeats and a male germline promoter that directs the expression of the Cas9 nuclease at the appropriate stage during meiosis. A bioinformatic pipeline was developed to identify X-specific sequences for shredding (Papathanos and Windbichler, 2018). Single cell RNA sequencing could enable discovery of appropriate promoters [reviewed in Compton et al. (2020)].
We discuss insect SRDs from three main perspectives in the context of the Anthropocene. First, we consider whether or not climate change may impact the sex ratio of wild insect populations. It is not yet clear whether climate change will introduce instability to the otherwise relatively stable sex-determination pathways in some insects. It is also not clear how climate change may affect the naturally occurring SRDs including those mediated by endosymbiotic bacteria. However, sex-biased heat-tolerance has been shown in diverse insects which may lead to shifting sex ratios in response to climate change (Edmands, 2021). Temperature can also affect female fertilization and alter the sex ratio in Hymenoptera parasitic wasps (Moiroux et al., 2014). One study demonstrated that longer drought periods caused by a delay in the timing of summer rainfall in the Mediterranean region led to a female sex ratio bias in the acorn Weevil (Bonal et al., 2015). Endosymbiotic manipulation of sex ratio in Pezothrips kellyanus Thrips is influenced by abiotic factors such as temperature (Katlav et al., 2022). It has also been suggested that temperature may differentially impact male and female body size, mortality, protandry, and population-level sex ratios of wild bees (Slominski and Burkle, 2019).
Second, engineered SRDs have shown great promise in controlling insect pests of agricultural and medical importance as discussed in previous sections. These efforts are critical to help sustain agriculture and improve human health. However, a less discussed but perhaps equally important application of SRDs relates to conservation of wildlife or endangered species that are under the threat of insect-borne infectious diseases (National Academies of Sciences [NAS] et al., 2016). For example, only 20 of the 46 recorded forest bird species in Hawaii are still extant in the wild (Fortini et al., 2015). Many of these species are threatened by avian malaria, transmitted by the Culex quinquefasciatus mosquito. The presence of avian malaria constrains these birds to a narrow range of habitats that are unsuitable for the mosquito (Samuel et al., 2011). Climate change is expected to broaden the distribution of C. quinquefasciatus, thereby further shrink the habitat range for susceptible bird species (Benning et al., 2002; Ahumada et al., 2009; Samuel et al., 2011; Fortini et al., 2015). Integrating SRD-mediated mosquito population suppression strategies into the long-term conservation efforts would allow Hawaiian forest birds to reclaim lost habitat. New genomic resources1 and newly established genome-editing methods (Feng et al., 2021; Purusothaman et al., 2021) for C. quinquefasciatus provide the foundation for SRD development.
Finally, the potential environmental impacts of the engineered SRD control strategies should be critically evaluated in the context of current methods. Current insect control methods rely heavily on insecticides and increasing resistance has significantly reduced their effectiveness. Some widely used insecticides such as neonicotinoids, which were previously thought to be safe and highly target-specific (reviewed in Jeschke and Nauen, 2008), have been shown to have potential health risks (Thompson et al., 2020), can impact non-target invertebrates and insectivorous birds (Hallmann et al., 2014; Pisa et al., 2015), and potentially contribute to the decline of honeybees (Rundlöf et al., 2015). Genetic control strategies are species-specific as they all require mating and thus a direct impact on non-target species is not anticipated. However, some of the SRD-mediated methods, especially the ones involving gene drives, are very powerful and could potentially eliminate a pest species from a large region, which may have ecological consequences. There are ongoing efforts to fine-tune, confine or neutralize the power of some of the gene drive systems (Vella et al., 2017; Kandul et al., 2019; Noble et al., 2019; Li et al., 2020, 2021; Taxiarchi et al., 2021). It is unlikely that any one control measure will be the “silver bullet.” Integration or selective implementation of a set of control measures most appropriate to the specific goals and the social and environmental context will likely be most effective. Therefore, continued development of a diverse range of genetic control methods for insect pests are important for promoting sustainable agriculture, improving One Health, and preserving wildlife.
AC and ZT wrote and revised the manuscript. Both authors contributed to the article and approved the submitted version.
This work was supported by grants AI157491, AI154871, AI123338, and AI121284 from the National Institutes of Health and by the Virginia Experimental Station.
The authors declare that the research was conducted in the absence of any commercial or financial relationships that could be construed as a potential conflict of interest.
All claims expressed in this article are solely those of the authors and do not necessarily represent those of their affiliated organizations, or those of the publisher, the editors and the reviewers. Any product that may be evaluated in this article, or claim that may be made by its manufacturer, is not guaranteed or endorsed by the publisher.
We would like to thank James K. Biedler for helpful comments.
Adelman, Z. N., and Tu, Z. (2016). Control of Mosquito-Borne Infectious Diseases: Sex and Gene Drive. Trends Parasitol. 32, 219–229. doi: 10.1016/j.pt.2015.12.003
Ahumada, J. A., Samuel, M. D., Duffy, D. C., Dobson, A. P., and Hobbelen, P. H. F. (2009). “Modeling the epidemiology of avian malaria and pox in Hawaii,” in Conservation Biology of Hawaiian Forest Birds, eds T. K. Pratt, C. T. Atkinson, P. C. Banko, J. Jacobi, and B. L. Woodworth (New Haven: Yale University Press), 331–355.
Alcalay, Y., Fuchs, S., Galizi, R., Bernardini, F., Haghighat-Khah, R. E., Rusch, D. B., et al. (2021). The Potential for a Released Autosomal X-Shredder Becoming a Driving-Y Chromosome and Invasively Suppressing Wild Populations of Malaria Mosquitoes. Front. Bioeng. Biotechnol. 9:752253. doi: 10.3389/fbioe.2021.752253
Aryan, A., Anderson, M. A. E., Biedler, J. K., Qi, Y., Overcash, J. M., Naumenko, A. N., et al. (2020). Nix alone is sufficient to convert female Aedes aegypti into fertile males and myo-sex is needed for male flight. Proc. Natl. Acad. Sci. U.S.A. 117, 17702–17709. doi: 10.1073/pnas.2001132117
Bachtrog, D., Mank, J. E., Peichel, C. L., Kirkpatrick, M., Otto, S. P., Ashman, T.-L., et al. (2014). Tree of Sex C. Sex determination: why so many ways of doing it? PLoS Biol. 12:e1001899–e. doi: 10.1371/journal.pbio.1001899
Bagheri, Z., Talebi, A. A., Asgari, S., and Mehrabadi, M. (2022). Wolbachia promotes successful sex with siblings in the parasitoid Habrobracon hebetor. Pest Manage. Sci. 78, 362–368. doi: 10.1002/ps.6649
Benning, T. L., LaPointe, D., Atkinson, C. T., and Vitousek, P. M. (2002). Interactions of climate change with biological invasions and land use in the Hawaiian Islands: Modeling the fate of endemic birds using a geographic information system. Proc.Natl. Acad. Sci. 99:14246. doi: 10.1073/pnas.162372399
Beukeboom, L. W., and Perrin, N. (2014). The Evolution of Sex Determination. Oxford: Oxford University Press, 240.
Biedler, J. K., and Tu, Z. (2016). “Chapter Two - Sex Determination in Mosquitoes,” in Advances in Insect Physiology, ed. A. S. Raikhel (Cambridge:Academic Press), 37–66.
Bonal, R., Hernández, M., Espelta, J. M., Muñoz, A., and Aparicio, J. M. (2015). Unexpected consequences of a drier world: evidence that delay in late summer rains biases the population sex ratio of an insect. R. Soc. Open Sci. 2:150198. doi: 10.1098/rsos.150198
Carrami, E. M., Eckermann, K. N., Ahmed, H. M. M., Sánchez, C. H. M., Dippel, S., Marshall, J. M., et al. (2018). Consequences of resistance evolution in a Cas9-based sex conversion-suppression gene drive for insect pest management. Proc. Natl. Acad. Sci. 115:6189. doi: 10.1073/pnas.1713825115
Cheng, B., Kuppanda, N., Aldrich, J. C., Akbari, O. S., and Ferree, P. M. (2016). Male-Killing Spiroplasma Alters Behavior of the Dosage Compensation Complex during Drosophila melanogaster Embryogenesis. Curr. Biol. 26, 1339–1345. doi: 10.1016/j.cub.2016.03.050
Compton, A., Sharakhov, I. V., and Tu, Z. (2020). Recent advances and future perspectives in vector-omics. Curr. Opin. Insect. Sci. 40, 94–103. doi: 10.1016/j.cois.2020.05.006
Conte, C. A., Segura, D. F., Milla, F. H., Augustinos, A., Cladera, J. L., Bourtzis, K., et al. (2019). Wolbachia infection in Argentinean populations of Anastrepha fraterculus sp1: preliminary evidence of sex ratio distortion by one of two strains. BMC Microbiol. 19:289. doi: 10.1186/s12866-019-1652-y
Courret, C., Chang, C.-H., Wei, K. H. C., Montchamp-Moreau, C., and Larracuente, A. M. (2019). Meiotic drive mechanisms: lessons from Drosophila. Proc.R. Soc. B. 286:20191430. doi: 10.1098/rspb.2019.1430
Craig, G. B., Hickey, W. A., and VandeHey, R. C. (1960). An Inherited Male-Producing Factor in Aedes aegypti. Science 132, 1887–1889. doi: 10.1126/science.132.3443.1887
Criscione, F., Qi, Y., and Tu, Z. (2016). GUY1 confers complete female lethality and is a strong candidate for a male-determining factor in Anopheles stephensi. eLife 5:e19281. doi: 10.7554/eLife.19281
Dobson, S. L., Fox, C. W., and Jiggins, F. M. (2002). The effect of Wolbachia-induced cytoplasmic incompatibility on host population size in natural and manipulated systems. Proc Biol Sci 269, 437–445. doi: 10.1098/rspb.2001.1876
Edmands, S. (2021). Sex Ratios in a Warming World: Thermal Effects on Sex-Biased Survival. Sex Determination, and Sex Reversal. J. Hered. 112, 155–164. Epub 2021/02/16. PubMed doi: 10.1093/jhered/esab006
Fasulo, B., Meccariello, A., Morgan, M., Borufka, C., Papathanos, P. A., and Windbichler, N. A. (2020). fly model establishes distinct mechanisms for synthetic CRISPR/Cas9 sex distorters. PLoS Genet. 16:e1008647. doi: 10.1371/journal.pgen.1008647
Feng, X., López Del Amo, V., Mameli, E., Lee, M., Bishop, A. L., Perrimon, N., et al. (2021). Optimized CRISPR tools and site-directed transgenesis towards gene drive development in Culex quinquefasciatus mosquitoes. Nature Communications. 12:2960. doi: 10.1038/s41467-021-23239-0
Fortini, L. B., Vorsino, A. E., Amidon, F. A., Paxton, E. H., and Jacobi, J. D. (2015). Large-Scale Range Collapse of Hawaiian Forest Birds under Climate Change and the Need 21st Century Conservation Options. PLoS One. 10:e0140389. doi: 10.1371/journal.pone.0140389
Fu, G., Lees, R. S., Nimmo, D., Aw, D., Jin, L., Gray, P., et al. (2010). Female-specific flightless phenotype for mosquito control. Proc.Natl. Acad. Sci. U.S.A. 107:4550. doi: 10.1073/pnas.1000251107
Fukui, T., Kawamoto, M., Shoji, K., Kiuchi, T., Sugano, S., Shimada, T., et al. (2015). The Endosymbiotic Bacterium Wolbachia Selectively Kills Male Hosts by Targeting the Masculinizing Gene. PLoS Pathogens. 11:e1005048. doi: 10.1371/journal.ppat.1005048
Galizi, R., Doyle, L. A., Menichelli, M., Bernardini, F., Deredec, A., Burt, A., et al. (2014). synthetic sex ratio distortion system for the control of the human malaria mosquito. Nat. Commun. 5:3977. doi: 10.1038/ncomms4977
Galizi, R., Hammond, A., Kyrou, K., Taxiarchi, C., Bernardini, F., O’Loughlin, S. M., et al. (2016). Cas9 sex-ratio distortion system for genetic control. Sci. Rep. 6:31139. doi: 10.1038/srep31139
Hall, A. B., Basu, S., Jiang, X., Qi, Y., Timoshevskiy, V. A., Biedler, J. K., et al. (2015). male-determining factor in the mosquito Aedes aegypti. Science. 348:1268. doi: 10.1126/science.aaa2850
Hall, A. B., Papathanos, P.-A., Sharma, A., Cheng, C., Akbari, O. S., Assour, L., et al. (2016). Radical remodeling of the Y chromosome in a recent radiation of malaria mosquitoes. Proc. Natl. Acad. Sci. 113:E2114. doi: 10.1073/pnas.1525164113
Hallmann, C. A., Foppen, R. P., van Turnhout, C. A., de Kroon, H., and Jongejans, E. (2014). Declines in insectivorous birds are associated with high neonicotinoid concentrations. Nature. 511, 341–343. PubMed doi: 10.1038/nature13531
Hammond, A., Pollegioni, P., Persampieri, T., North, A., Minuz, R., Trusso, A., et al. (2021). Gene-drive suppression of mosquito populations in large cages as a bridge between lab and field. Nat. Commun. 12:4589. doi: 10.1038/s41467-021-24790-6
Harumoto, T., Anbutsu, H., Lemaitre, B., and Fukatsu, T. (2016). Male-killing symbiont damages host’s dosage-compensated sex chromosome to induce embryonic apoptosis. Nat. Commun. 7:12781. PubMed doi: 10.1038/ncomms12781
Harumoto, T., Fukatsu, T., and Lemaitre, B. (2018). Common and unique strategies of male killing evolved in two distinct Drosophila symbionts. Proc.R. So. B. 285:20172167. doi: 10.1098/rspb.2017.2167
Herpin, A., and Schartl, M. (2015). Plasticity of gene-regulatory networks controlling sex determination: of masters, slaves, usual suspects, newcomers, and usurpators. EMBO Rep. 16, 1260–1274. doi: 10.15252/embr.201540667
Hopkins, B. R., and Kopp, A. (2021). Evolution of sexual development and sexual dimorphism in insects. Curr. Opinion Gene. Dev. 69, 129–139. doi: 10.1016/j.gde.2021.02.011
Hurst, G. D., and Frost, C. L. (2015). Reproductive parasitism: maternally inherited symbionts in a biparental world. Cold Spring Harb Perspect. Biol. 7:a017699. doi: 10.1101/cshperspect.a017699
Hurst, G. D. D., and Jiggins, F. M. (2000). Male-Killing Bacteria in Insects: Mechanisms, Incidence, and Implications. Emerg. Infect. Dis. J. 6:329. doi: 10.3201/eid0604.000402
Jaenike, J. (2001). Sex Chromosome Meiotic Drive. Ann. Rev. Ecol. Syst. 32, 25–49. doi: 10.1146/annurev.ecolsys.32.081501.113958
Jeschke, P., and Nauen, R. (2008). Neonicotinoids—from zero to hero in insecticide chemistry. Pest Manag. Sci. 64, 1084–1098. doi: 10.1002/ps.1631
Jiang, X., Biedler, J. K., Qi, Y., Hall, A. B., and Tu, Z. (2015). Complete Dosage Compensation in Anopheles stephensi and the Evolution of Sex-Biased Genes in Mosquitoes. Genome Biol. Evol. 7, 1914–1924. doi: 10.1093/gbe/evv115
Kageyama, D., Narita, S., and Watanabe, M. (2012). Insect Sex Determination Manipulated by Their Endosymbionts: Incidences, Mechanisms and Implications. Insects 3161–99. doi: 10.3390/insects3010161
Kageyama, D., Ohno, M., Sasaki, T., Yoshido, A., Konagaya, T., Jouraku, A., et al. (2017). Feminizing Wolbachia endosymbiont disrupts maternal sex chromosome inheritance in a butterfly species. Evol. Lett. 1, 232–244. doi: 10.1002/evl3.28
Kandul, N. P., Liu, J., Hsu, A. D., Hay, B. A., and Akbari, O. S. A. (2020). drug-inducible sex-separation technique for insects. Nat. Commun. 11:2106. doi: 10.1038/s41467-020-16020-2
Kandul, N. P., Liu, J., Sanchez, C. H. M., Wu, S. L., Marshall, J. M., and Akbari, O. S. (2019). Transforming insect population control with precision guided sterile males with demonstration in flies. Nat. Commun. 10:84. doi: 10.1038/s41467-018-07964-7
Katlav, A., Nguyen, D. T., Morrow, J. L., Spooner-Hart, R. N., and Riegler, M. (2022). Endosymbionts moderate constrained sex allocation in a haplodiploid thrips species in a temperature-sensitive way. Heredity 128, 169–177. doi: 10.1038/s41437-022-00505-5
Kern, P., Cook, J. M., Kageyama, D., and Riegler, M. (2015). Double trouble: combined action of meiotic drive and Wolbachia feminization in Eurema butterflies. Biol. Lett. 11:20150095. doi: 10.1098/rsbl.2015.0095
Kiuchi, T., Koga, H., Kawamoto, M., Shoji, K., Sakai, H., Arai, Y., et al. (2014). single female-specific piRNA is the primary determiner of sex in the silkworm. Nature 509, 633–636. doi: 10.1038/nature13315
Krzywinska, E., Dennison Nathan, J., Lycett Gareth, J., and Krzywinski, J. A. (2016). maleness gene in the malaria mosquito Anopheles gambiae. Science 353, 67–69. doi: 10.1126/science.aaf5605
Krzywinska, E., Ferretti, L., Li, J., Li, J. C., Chen, C. H., and Krzywinski, J. (2021). femaleless Controls Sex Determination and Dosage Compensation Pathways in Females of Anopheles Mosquitoes. Curr. Biol. 31, 1084.e–1091.e. doi: 10.1016/j.cub.2020.12.014
Krzywinska, E., and Krzywinski, J. (2018). Effects of stable ectopic expression of the primary sex determination gene Yob in the mosquito Anopheles gambiae. Parasites Vect. 11:648. doi: 10.1186/s13071-018-3211-z
Kyrou, K., Hammond, A. M., Galizi, R., Kranjc, N., Burt, A., Beaghton, A. K., et al. (2018). Cas9 gene drive targeting doublesex causes complete population suppression in caged Anopheles gambiae mosquitoes. Nat. Biotechnol. 36, 1062–1066. PubMed doi: 10.1038/nbt.4245
Landmann, F. (2019). The Wolbachia Endosymbionts. Microbiol. Spectr. 7, 88–95. Epub 2019/04/07. doi: 10.1128/microbiolspec.BAI-0018-2019
Laven, H. (1967). Eradication of Culex pipiens fatigans through Cytoplasmic Incompatibility. Nature 216, 383–384. doi: 10.1038/216383a0
Li, M., Yang, T., Bui, M., Gamez, S., Wise, T., Kandul, N. P., et al. (2021). Suppressing mosquito populations with precision guided sterile males. Nat. Commun. 12:5374. doi: 10.1038/s41467-021-25421-w
Li, M., Yang, T., Kandul, N. P., Bui, M., Gamez, S., Raban, R., et al. (2020). Development of a confinable gene drive system in the human disease vector Aedes aegypti. Elife 9:e51701. Epub 2020/01/22. doi: 10.7554/eLife.51701
Lin, C.-J., Hu, F., Dubruille, R., Vedanayagam, J., Wen, J., Smibert, P., et al. (2018). The hpRNA/RNAi Pathway Is Essential to Resolve Intragenomic Conflict in the Drosophila Male Germline. Dev. Cell 46, 316.e–326.e. doi: 10.1016/j.devcel.2018.07.004
Liu, P., Jin, B., Li, X., Zhao, Y., Gu, J., Biedler, J. K., et al. (2020). Nix is a male-determining factor in the Asian tiger mosquito Aedes albopictus. Insect Biochem. Mol. Biol. 118:103311. doi: 10.1016/j.ibmb.2019.103311
Lutrat, C., Olmo, R. P., Baldet, T., Bouyer, J., and Marois, E. (2021). Transgenic expression of Nix; converts genetic females into males and allows automated sex sorting in Aedes albopictus. bioRxiv [Preprint]. doi: 10.1101/2021.07.28.454191
Lyttle, T. W. (1991). Segregation distorters. Annu Rev Genet. 25, 511–557. PubMed doi: 10.1146/annurev.ge.25.120191.002455
Ma, W. J., Vavre, F., and Beukeboom, L. W. (2014). Manipulation of arthropod sex determination by endosymbionts: diversity and molecular mechanisms. Sex Dev. 8, 59–73. PubMed doi: 10.1159/000357024
Matthews, B. J., Dudchenko, O., Kingan, S. B., Koren, S., Antoshechkin, I., and Crawford, J. E. (2018). Improved reference genome of Aedes aegypti informs arbovirus vector control. Nature 563, 501–507. doi: 10.1038/s41586-018-0692-z
Meccariello, A., Krsticevic, F., Colonna, R., Del Corsano, G., Fasulo, B., Papathanos, P. A., et al. (2021). Engineered sex ratio distortion by X-shredding in the global agricultural pest Ceratitis capitata. BMC Biol. 19:78. doi: 10.1186/s12915-021-01010-7
Meccariello, A., Salvemini, M., Primo, P., Hall, B., Koskinioti, P., and Dalíková, M. (2019). Maleness-on-the-Y (MoY) orchestrates male sex determination in major agricultural fruit fly pests. Science 365, 1457–1460. doi: 10.1126/science.aax1318
Moiroux, J., Brodeur, J., and Boivin, G. (2014). Sex ratio variations with temperature in an egg parasitoid: behavioural adjustment and physiological constraint. Anim. Behav. 91, 61–66. doi: 10.1016/j.anbehav.2014.02.021
Muirhead, C. A., and Presgraves, D. C. (2021). Satellite DNA-mediated diversification of a sex-ratio meiotic drive gene family in Drosophila. Nat. Ecol. Evol. 5, 1604–1612. doi: 10.1038/s41559-021-01543-8
National Academies of Sciences [NAS], Engineering, and Medicine, Division on Earth and Life Studies, Board on Life Sciences, and Committee on Gene Drive Research in Non-Human Organisms: Recommendations for Responsible Conduct. (2016). Gene Drives on the Horizon: Advancing Science, Navigating Uncertainty, and Aligning Research with Public Values. Washington (DC): National Academies Press.
Newton, M. E., Wood, R. J., and Southern, D. I. A. (1976). cytogenetic analysis of meiotic drive in the mosquito. Aedes aegypti (L.). Genetica. 46, 297–318. doi: 10.1007/BF00055473
Noble, C., Min, J., Olejarz, J., Buchthal, J., Chavez, A., Smidler, A. L., et al. (2019). Daisy-chain gene drives for the alteration of local populations. Proc.Natl. Acade. Sci. 116:8275. doi: 10.1073/pnas.1716358116
Owusu-Daaku, K., Wood, R., and Butler, R. (1997). Selected lines of Aedes aegypti with persistently distorted sex ratios. Heredity 79(Pt 4), 388–393. doi: 10.1038/hdy.1997.172
Pane, A., Salvemini, M., Delli Bovi, P., Polito, C., and Saccone, G. (2002). The transformer gene in Ceratitis capitata provides a genetic basis for selecting and remembering the sexual fate. Development 129, 3715–3725. Epub 2002/07/16. PubMed doi: 10.1242/dev.129.15.3715
Papathanos, P. A., and Windbichler, N. (2018). Redkmer: An Assembly-Free Pipeline for the Identification of Abundant and Specific X-Chromosome Target Sequences for X-Shredding by CRISPR Endonucleases. CRISPR J. 1, 88–98. doi: 10.1089/crispr.2017.0012
Pisa, L. W., Amaral-Rogers, V., Belzunces, L. P., Bonmatin, J. M., Downs, C. A., Goulson, D., et al. (2015). Effects of neonicotinoids and fipronil on non-target invertebrates. Environ. Sci. Pollution Res. 22, 68–102. doi: 10.1007/s11356-014-3471-x
Primo, P., Meccariello, A., Inghilterra, M. G., Gravina, A., Del Corsano, G., and Volpe, G. (2020). Targeting the autosomal Ceratitis capitata transformer gene using Cas9 or dCas9 to masculinize XX individuals without inducing mutations. BMC Genet 21:150. doi: 10.1186/s12863-020-00941-4
Purusothaman, D.-K., Shackleford, L., Anderson, M. A. E., Harvey-Samuel, T., and Alphey, L. C. R. I. S. P. R. (2021). /Cas-9 mediated knock-in by homology dependent repair in the West Nile Virus vector Culex quinquefasciatus Say. Sci. Rep. 11:14964. doi: 10.1038/s41598-021-94065-z
Qi, Y., Wu, Y., Saunders, R., Chen, X.-G., Mao, C., Biedler, J. K., et al. (2019). Guy1, a Y-linked embryonic signal, regulates dosage compensation in Anopheles stephensi by increasing X gene expression. eLife 8:e43570. doi: 10.7554/eLife.43570
Rundlöf, M., Andersson, G. K. S., Bommarco, R., Fries, I., Hederström, V., Herbertsson, L., et al. (2015). Seed coating with a neonicotinoid insecticide negatively affects wild bees. Nature 521, 77–80. doi: 10.1038/nature14420
Samuel, M. D., Hobbelen, P. H. F., Decastro, F., Ahumada, J. A., Lapointe, D., Atkinson, C. T., et al. (2011). The dynamics, transmission, and population impacts of avian malaria in native hawaiian birds: A modeling approach. Ecol. Appl. 21, 2960–2973. doi: 10.1890/10-1311.1
Scott, M. (2021). Sex determination and dosage compensation: femaleless is the Link in Anopheles mosquitoes. Curr. Biol. 31, R260–R263. doi: 10.1016/j.cub.2021.01.078
Shan, H.-W., Luan, J.-B., Liu, Y.-Q., Douglas, A. E., and Liu, S.-S. (2019). The inherited bacterial symbiont Hamiltonella influences the sex ratio of an insect host. Proc.R. Soc. B. 286:20191677. doi: 10.1098/rspb.2019.1677
Sharma, A., Heinze Svenia, D., Wu, Y., Kohlbrenner, T., Morilla, I., Brunner, C., et al. (2017). Male sex in houseflies is determined by Mdmd, a paralog of the generic splice factor gene CWC22. Science 356, 642–645. doi: 10.1126/science.aam5498
Simoni, A., Hammond, A. M., Beaghton, A. K., Galizi, R., Taxiarchi, C., Kyrou, K., et al. (2020). male-biased sex-distorter gene drive for the human malaria vector Anopheles gambiae. Nat. Biotechnol. 38, 1054–1060. doi: 10.1038/s41587-020-0508-1
Slominski, A. H., and Burkle, L. A. (2019). Solitary Bee Life History Traits and Sex Mediate Responses to Manipulated Seasonal Temperatures and Season Length. Front. Ecol. Evol. 7:314. doi: 10.3389/fevo.2019.00314
Sugimoto, T. N., and Ishikawa, Y. A. (2012). male-killing Wolbachia carries a feminizing factor and is associated with degradation of the sex-determining system of its host. Biol. Lett. 8, 412–415. doi: 10.1098/rsbl.2011.1114
Sugimoto, T. N., Kayukawa, T., Shinoda, T., Ishikawa, Y., and Tsuchida, T. (2015). Misdirection of dosage compensation underlies bidirectional sex-specific death in Wolbachia-infected Ostrinia scapulalis. Insect Biochem. Mol. Biol. 66, 72–76. PubMed doi: 10.1016/j.ibmb.2015.10.001
Suguna, S. G., Wood, R. J., Curtis, C. F., Whitelaw, A., and Kazmi, S. J. (1977). Resistance to meiotic drive at the MD locus in an Indian wild population of Aedes aegypti. Genet Res. 29, 123–132. PubMed doi: 10.1017/s0016672300017195
Tao, Y., Araripe, L., Kingan, S. B., Ke, Y., Xiao, H., and Hartl, D. L. A. (2007a). sex-ratio Meiotic Drive System in Drosophila simulans. II: An X-linked Distorter. PLoS Biol. 5, e293. doi: 10.1371/journal.pbio.0050293
Tao, Y., Hartl, D. L., and Laurie, C. C. (2001). Sex-ratio segregation distortion associated with reproductive isolation in Drosophila. Proc.Natl. Acad. Sci. 98:13183. doi: 10.1073/pnas.231478798
Tao, Y., Masly, J. P., Araripe, L., Ke, Y., and Hartl, D. L. A. (2007b). sex-ratio Meiotic Drive System in Drosophila simulans. I : An Autosomal Suppressor. PLoS Biol. 5:e292. doi: 10.1371/journal.pbio.0050292
Taracena, M. L., Hunt, C. M., Benedict, M. Q., Pennington, P. M., and Dotson, E. M. (2019). Downregulation of female doublesex expression by oral-mediated RNA interference reduces number and fitness of Anopheles gambiae adult females. Parasites Vectors. 12:170. doi: 10.1186/s13071-019-3437-4
Taxiarchi, C., Beaghton, A., Don, N. I., Kyrou, K., Gribble, M., Shittu, D., et al. (2021). genetically encoded anti-CRISPR protein constrains gene drive spread and prevents population suppression. Nat. Commun. 12:3977. doi: 10.1038/s41467-021-24214-5
Taxiarchi, C., Kranjc, N., Kriezis, A., Kyrou, K., Bernardini, F., Russell, S., et al. (2019). High-resolution transcriptional profiling of Anopheles gambiae spermatogenesis reveals mechanisms of sex chromosome regulation. Sci. Rep. 9:14841. doi: 10.1038/s41598-019-51181-1
Thompson, D. A., Lehmler, H.-J., Kolpin, D. W., Hladik, M. L., Vargo, J. D., Schilling, K. E., et al. (2020). critical review on the potential impacts of neonicotinoid insecticide use: current knowledge of environmental fate, toxicity, and implications for human health. Environ. Sci. 22, 1315–1346. doi: 10.1039/C9EM00586B
Turner, J. M. (2007). Meiotic sex chromosome inactivation. Development 134, 1823–1831. doi: 10.1242/dev.000018
Vella, M. R., Gunning, C. E., Lloyd, A. L., and Gould, F. (2017). Evaluating strategies for reversing CRISPR-Cas9 gene drives. Sci. Rep. 7:11038. doi: 10.1038/s41598-017-10633-2
Wang, Y.-B., Ren, F.-R., Yao, Y.-L., Sun, X., Walling, L. L., Li, N.-N., et al. (2020). Intracellular symbionts drive sex ratio in the whitefly by facilitating fertilization and provisioning of B vitamins. ISME J. 14, 2923–2935. doi: 10.1038/s41396-020-0717-0
Weinert, L. A., Araujo-Jnr, E. V., Ahmed, M. Z., and Welch, J. J. (2015). The incidence of bacterial endosymbionts in terrestrial arthropods. Proc. R. Soc. B. 282:20150249. doi: 10.1098/rspb.2015.0249
Werren, J. H., Baldo, L., and Clark, M. E. (2008). Wolbachia: master manipulators of invertebrate biology. Nat. Rev. Microbiol. 6, 741–751. doi: 10.1038/nrmicro1969
Wexler, J., Delaney, E. K., Belles, X., Schal, C., Wada-Katsumata, A., Amicucci, M. J., et al. (2019). Hemimetabolous insects elucidate the origin of sexual development via alternative splicing. Elife 8:e47490. Epub 2019/09/04. doi: 10.7554/eLife.47490
Whyard, S., Erdelyan, C. N. G., Partridge, A. L., Singh, A. D., Beebe, N. W., and Capina, R. (2015). Silencing the buzz: a new approach to population suppression of mosquitoes by feeding larvae double-stranded RNAs. Parasites Vectors. 8:96. doi: 10.1186/s13071-015-0716-6
Wood, R. J. (1976). Between-family variation in sex ratio in the Trinidad (T-30) strain of Aedes aegypti (L.) indicating differences in sensitivity to the meiotic drive gene MD. Genetica. 46, 345–361. doi: 10.1007/BF00055477
Wood, R. J., and Ouda, N. A. (1987). The genetic basis of resistance and sensitivity to the meiotic drive gene D in the mosquito Aedes aegypti L. Genetica 72, 69–79. Epub 1987/05/15. PubMed doi: 10.1007/bf00126980
Zhang, Z., Niu, B., Ji, D., Li, M., Li, K., James, A. A., et al. (2018). Silkworm genetic sexing through W chromosome-linked, targeted gene integration. Proc.Natl. Acad. Sci. 115:8752. doi: 10.1073/pnas.1810945115
Zheng, X., Zhang, D., Li, Y., Yang, C., Wu, Y., Liang, X., et al. (2019). Incompatible and sterile insect techniques combined eliminate mosquitoes. Nature 572, 56–61. doi: 10.1038/s41586-019-1407-9
Zhuo, J. C., Zhang, H. H., Hu, Q. L., Zhang, J. L., Lu, J. B., Li, H. J., et al. (2021). feminizing switch in a hemimetabolous insect. Sci Adv. 7:eabf9237. doi: 10.1126/sciadv.abf9237
Keywords: CRISPR/Cas9, sex chromosome, sex-determination, gene drive, endosymbiont bacteria, climate change, sex conversion, sex-specific lethality
Citation: Compton A and Tu Z (2022) Natural and Engineered Sex Ratio Distortion in Insects. Front. Ecol. Evol. 10:884159. doi: 10.3389/fevo.2022.884159
Received: 25 February 2022; Accepted: 17 May 2022;
Published: 15 June 2022.
Edited by:
Amanda Wilson Carter, The University of Tennessee, Knoxville, United StatesReviewed by:
Chuanxiao Xie, Institute of Crop Sciences (CAAS), ChinaCopyright © 2022 Compton and Tu. This is an open-access article distributed under the terms of the Creative Commons Attribution License (CC BY). The use, distribution or reproduction in other forums is permitted, provided the original author(s) and the copyright owner(s) are credited and that the original publication in this journal is cited, in accordance with accepted academic practice. No use, distribution or reproduction is permitted which does not comply with these terms.
*Correspondence: Austin Compton, YXVzdGMxNEB2dC5lZHU=; Zhijian Tu, amFrZXR1QHZ0LmVkdQ==
Disclaimer: All claims expressed in this article are solely those of the authors and do not necessarily represent those of their affiliated organizations, or those of the publisher, the editors and the reviewers. Any product that may be evaluated in this article or claim that may be made by its manufacturer is not guaranteed or endorsed by the publisher.
Research integrity at Frontiers
Learn more about the work of our research integrity team to safeguard the quality of each article we publish.