- 1Ph.D. Program in Earth and Environmental Sciences, Graduate Center of The City University of New York, New York, NY, United States
- 2Department of Earth and Environmental Sciences, Brooklyn College of The City University of New York, Brooklyn, NY, United States
- 3Department of Landscape Design and Sustainable Ecosystems, Peoples’ Friendship University of Russia (RUDN University), Moscow, Russia
- 4School of Geosciences, University of Louisiana at Lafayette, Lafayette, LA, United States
- 5Cornell Atkinson Center for Sustainability, Cornell University, Ithaca, NY, United States
- 6Microhumus, Université de Lorraine, Ecole Nationale Supérieure d’Agronomie et des Industries Alimentaires (ENSAIA)—Laboratoire Sols et Environnement, Nancy, France
- 7Environmental Sciences Initiative, Advanced Science Research Center at the Graduate Center of The City University of New York, New York, NY, United States
Lead (Pb) exposure has long been recognized as a hazard to human health. Urban garden soils often contain elevated levels of Pb, mainly from legacy sources, which is a main barrier for urban gardening. The capacity of gardeners to access, understand, and act on scientific data related to soil contamination is also variable. This synthesis paper briefly summarizes the current scientific knowledge on soil Pb in urban gardens. Our objective is to produce clear recommendations about assessing actual risks and limiting exposure. First, we synthesize the nature and extent of soil contamination with Pb, and then describe how the bioavailability and risk of this contamination to humans is assessed. We then go on to potential exposure pathway through plants and remediation methods to improve soil health and reduce human exposure. We have developed best management practices for practitioners that include: (1) urban soil testing should be prioritized because of the high probability of Pb contamination, and urban gardening should not begin until thorough testing or remediation has been done; (2) documentation of land-use history should be required in all property transactions so that the potential for soil (and other) contamination can be clearly identified; (3) amendments cannot be relied upon as a treatment for contaminated soils to reduce risk to gardeners because they do not always make contaminants less harmful; (4) certain crops (such as fruiting vegetables) are much less susceptible to contamination than others and thus should be prioritized in urban gardens; (5) wherever feasible, raised beds filled with upcycled local mineral and organic materials are the preferred substrate for urban gardening. Further monitoring of potentially contaminated and remediated soils as well as effective communication with the public are necessary to ensure human safety.
Highlights
- Urban soil testing should be prioritized.
- Documentation of land-use history should be required and made available.
- Amendments cannot be relied upon as treatment for contaminated soils.
- Certain crops are much less susceptible to contamination than others.
- Raised beds filled with upcycled local mineral and organics are preferred.
Introduction
One of the earliest metals discovered and smelted from ore by humans, lead (Pb) has been used for over 5,000 years (Lewis, 1985). Lead was used by the ancient Romans to make water pipes and drinking vessels and to line baths. During the Middle Ages, alchemists considered Pb as a key constituent used in techniques thought to generate gold from baser metals. Uses in paint and fuel increased the amount and the spread of Pb in the environment (Mielke, 2016). By the twentieth century, the U.S. had emerged as the world’s leading producer and consumer of refined Pb. According to the National Academy of Science’s report on Lead in the Human Environment, by 1980, the U.S. was consuming about 1.3 million tons of Pb per year (Lewis, 1985). Lead paint was banned in the US in 1978 and leaded gasoline was only banned worldwide in 2021 (Palmer, 2021).
An enduring concern about Pb is in urban soils, where it is often present at alarmingly high levels (Cheng et al., 2015; Gupta et al., 2019; Rai et al., 2019). According to the US EPA (USEPA, 1998), three primary sources contribute to elevated soil-lead levels: (1) lead-based paint, (2) point source emitters (e.g., smelters, refuse incinerators, dumpsites for lead-acid batteries), and (3) leaded gasoline emissions. It is often difficult to identify specific sources responsible for elevated soil-lead concentrations at any particular location (USEPA, 1998).
The presence of Pb in urban soils create risks for urban agriculture and gardening. Urban (and peri-urban) agriculture is estimated to produce 15–20% of the global food supply (Lal, 2020), with over 800 million people actively engaging in urban agriculture worldwide (Hamilton et al., 2014). Urban cropland covers over 67 million ha or more than 5% of the world’s cropland area. About 266 million households may be engaged in urban crop production across developing countries (Hamilton et al., 2014). Fruit and vegetable cultivation can potentially yield up to 50 kg m–2 year–1 of edible produce (Eigenbrod and Gruda, 2015).
From 2008 to 2013, the number of home gardens in the US increased by 4 –37 million households, while community gardens tripled from 1 to 3 million, a 200 percent increase (National Gardening Association, 2014). There are more than 18,000 community gardens throughout the United States and Canada (Lawson and Drake, 2013). In New York City, there are over 750 community-sponsored open space garden project sites (Lawson and Drake, 2013) covering more than 90 acres (Eizenberg, 2013). There are over 745 school gardens, over 100 gardens in land trusts, and over 700 gardens at public housing developments on city property (Eizenberg, 2013).
Miscommunication and Confusion About Soil Contamination and Risk
Unfortunately, trace metal contamination is common in urban soils and is a major constraint on urban agriculture (Gupta et al., 2019). In addition to Pb, contaminants found in urban soils include pesticides, petroleum products, asbestos, creosote, and radon (Alloway, 2004). Contaminated soils have the potential to produce contaminated plants, which can then be consumed by humans (Rai et al., 2019). There are many publications on the topic of trace element pollution (Hough et al., 2004; Pelfrêne et al., 2013, 2019; Izquierdo et al., 2015; Bidar et al., 2020) and on how to mitigate the health risk associated with gardening and consumption of garden produce. However, awareness and understanding by the general public of the actual risks related to urban gardening are often lacking (Burghardt et al., 2015; Hunter et al., 2019; Bidar et al., 2020).
Gardeners respond to and act on scientific data related to soil contamination in variable ways (Harms et al., 2013; Kim et al., 2014; Balotin et al., 2020). Communities are often unaware of soil contamination risks and remediation strategies (Whitzling et al., 2010). Around the world, many newspapers and websites encouraged urban gardening during the COVID-19 pandemic, but few highlighted potential contaminations in urban soils and the associated risk for human health. This is an unfortunate omission, and general interest publications such as newspapers are more accessible to community gardeners than scientific literature. In addition, property owners have great temptation to ignore contamination issues, as they may negatively impact property values (MacNair, 2004).
Even when gardeners are aware of contamination issues and mitigation strategies, they may not be able to apply these strategies properly or be aware of the extent of contamination and the routes of exposure (Harms et al., 2013; Kim et al., 2014; Balotin et al., 2020). There is also high variation among groups of gardeners, e.g., one study found that home gardeners were more aware of the potential health risks associated with soil trace elements than community gardeners (Balotin et al., 2020).
As is so frequently the case in complex societal issues, the flow of information from science to society regarding soil contamination is incomplete and inefficient, and scientific results are often misinterpreted. For example, in 2014, the New York Post published two articles on “toxic soils” and “toxic vegetables” grown in the Sterling Community Garden in Brooklyn (Buiso, 2014a,b). The stories were misinterpretations of the data published by McBride et al. (2014) and used sensational phrases such as “disturbing new state data,” “the numbers are startling,” and “this is insane.” Further research showed that only a small area in the garden was contaminated and that risks could be readily mitigated (Paltseva et al., 2020). Despite decades of research on soil contamination, there is still a clear need to synthesize information and produce clear, generally accessible, and understandable recommendations for safe urban gardening practices. Thus, the objectives for this synthesis paper are to briefly summarize the current scientific knowledge on soil Pb in urban gardens and to produce clear recommendations about assessing actual risk and limiting exposures. To write this paper we used online sources Web of Science, Google Scholar, Research Gate and purposefully searched or browsed for the articles related to our topic that we have been working on for several years. We also used reference lists in published articles to help us narrow down searches to the most recent and relevant materials. The following road map lays out the outline of the paper (Figure 1). In the sections below, we first review the nature and extent of soil contamination with Pb, and then describe risks to humans. We then discuss potential exposure pathways through plants, how Pb bioavailability is assessed, and remediation methods to improve soil health and reduce human exposure. We conclude with the best management practices for practitioners and future perspectives.
Urban and Suburban Soil Contamination
Levels and Distribution of Trace Elements in Urban Garden Soils
The first step to promote the many benefits of urban gardening is to assess the risk from trace element exposure by characterizing the nature and extent of Pb levels in gardens. For example, in NYC, high median Pb concentrations (n = 2,322) were found in a wide range of neighborhoods cutting across density and socioeconomic gradients (Paltseva and Cheng, 2019a). Similar results have been found in other cities (Table 1) and have been compiled in a number of review papers (Ajmone-Marsan and Biasioli, 2010; Wei and Yang, 2010; Alekseenko and Alekseenko, 2014; Cheng et al., 2014; Antoniadis et al., 2019; Hanfi and Yarmoshenko, 2020). We suggest that a clear and useful conclusion and recommendation would be to assume that urban soils of any industrial or postindustrial country have a high probability for Pb contamination and that urban gardening should not begin until thorough testing or prophylactic remediation (e.g., construction of raised beds with clean soil) has been done.
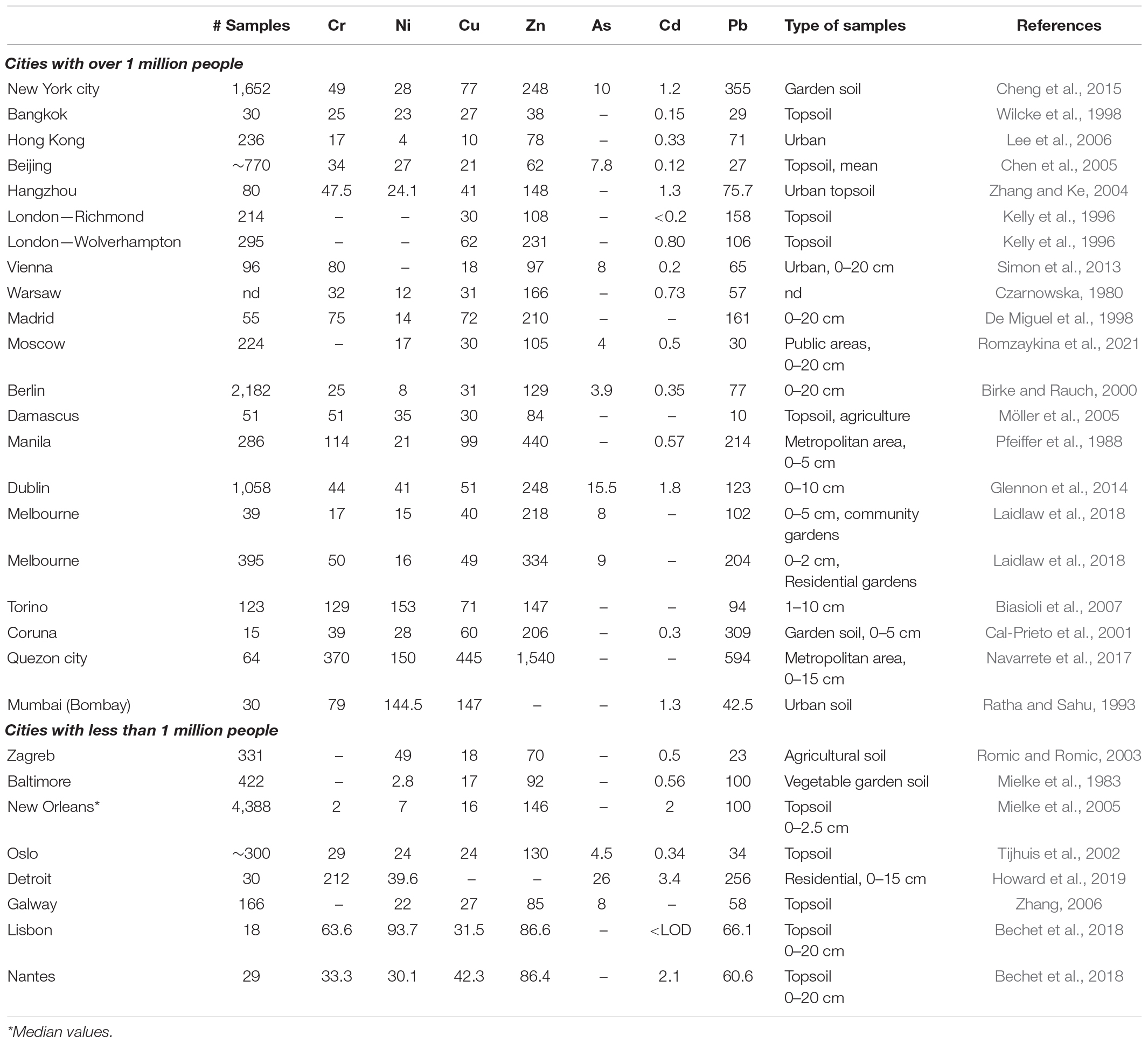
Table 1. Comparison of mean trace elements concentrations in urban soils in selected cities around the world (in mg kg–1).
Suburban Soil Contamination
A topic that has received less attention is that soil contamination by trace elements extends beyond city boundaries and is an issue for the much larger suburban areas around cities (Table 2). For example, trace metal concentrations in suburban Christchurch, New Zealand, garden soils were higher than background soil concentrations (Ashrafzadeh et al., 2018). Neighborhoods in Christchurch developed before the 1950s were affected by leaded paint and gasoline and had a mean Pb concentration of 282 mg kg–1 in their garden soils. Areas that experienced demolition and reconstruction work following earthquakes were vulnerable to redeposition of contaminants associated with this disturbance (Ashrafzadeh et al., 2018).
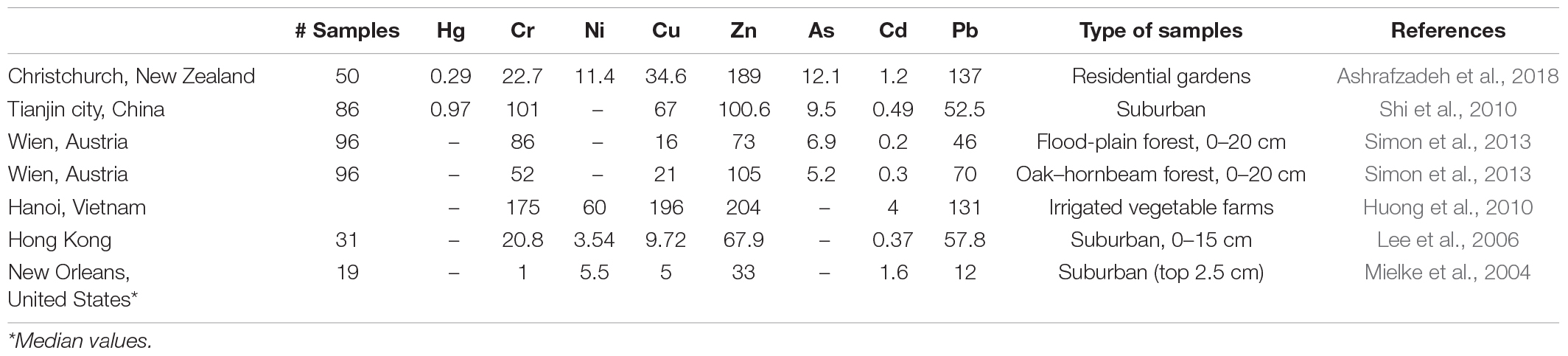
Table 2. Comparison of mean trace elements concentrations in suburban soils in selected cities around the world (in mg kg–1).
In suburbs of Tianjin City, China, Pb exceeded the “Soil Environmental Quality Risk Control Standard for Soil Contamination of Agricultural Land (GB 15618-2018)” with exceedance rates of 3.06% (Zhang et al., 2019). Wastewater and sludge irrigation and air deposition were the primary contaminant sources (Shi et al., 2010). In Vienna, Austria, Pb concentrations were significantly higher in urban than suburban and rural areas (Simon et al., 2013), but the differences were not statistically significant between suburban and rural areas. Vienna’s most important pollution sources are numerous roads and motorways with heavy traffic (Simon et al., 2013). Agricultural surface soils (0–20 cm) in Thanh Tri District of Tam Hiep Commune in Hanoi City, Vietnam, had concentrations of Pb (145 mg kg–1) exceeding the Vietnamese standard for agricultural soil (Huong et al., 2010). The primary source of contamination is the application of polluted irrigation water to the soil. Vegetable heavy metal concentrations also exceeded the Vietnamese standards for Pb (Huong et al., 2010).
At a location in the suburbs of New York City (Duke Farm, New Jersey) where lead-arsenate pesticides were used to control gypsy moths in the 1920s, vegetables had Pb levels above health and safety standards, especially root and leafy green vegetables (Paltseva et al., 2018). This contamination appears to have come from soil particles adhering to the vegetables; i.e., these crops did not appear to be taking up significant amounts of metals into their tissues via roots. Nevertheless, tomatoes were the only vegetables entirely safe for consumption. Phosphate-bearing amendments added to the soil reduced extractable Pb but increased extractable As in soils. Compost additions significantly improved soil quality, plant health, and yield and decreased soil and vegetable contamination levels. Lead-arsenate pesticides were widely used in orchards (McBride, 2013; Gamble et al., 2018) and have left a widespread legacy of contamination similar to that observed at Duke Farm.
There is a strong need to expand awareness of trace element problems beyond dense urban areas. While the contaminant levels found in suburban soils are commonly lower than densely populated urban areas, they are often elevated compared to background soils and exceed thresholds set forth for human health. We suggest that a clear and useful recommendation is to include land-use history in all property transactions so that the potential for soil (and other) contamination can be clearly identified.
Potential Health Risks of Soil Lead: The Effects of Environmental Variables and Exposure Pathways on Health Outcomes
While Pb is estimated to cause 412,000 premature deaths per year and has been deemed a “leading risk factor for premature death in the United States” (Lanphear et al., 2018), the relative importance of exposure to Pb in soil, a key concern in urban gardening, is not apparent (Gailey et al., 2020). Zahran et al. (2013b) reported that children’s blood lead levels (BLLs) were spatially correlated with neighborhood soil Pb levels in New Orleans. Research in Syracuse, NY, Minneapolis, and St. Paul, MN, Detroit, MI arrived at similar conclusions (Aschengrau et al., 1994; Johnson and Bretsch, 2002; Zahran et al., 2011; Mielke et al., 2016, 2019; Laidlaw et al., 2017). Despite these observations in numerous cities, along with data on soil Pb in New York City (NYC) (Nussbaumer-Streit et al., 2016), the NYC Health Department (DOHMH) does not officially recognize the impact of soil contamination on children’s Pb exposure and BLLs (Figure 2). Former NYC Health commissioner stated at a New York City Council Oversight Hearing, “Soil is not, I repeat not, a significant source of lead exposure for children in New York City” (Werth, 2019). Soil Pb concentrations in NYC gardens (mostly private home gardens) range up to 9,000 mg kg–1 with a median of 355 mg kg–1 (Cheng et al., 2015). Among all the garden samples tested (n = 1,646), 48% exceeded the US EPA standard of 400 mg kg–1 for children’s play areas (USEPA, 2014).
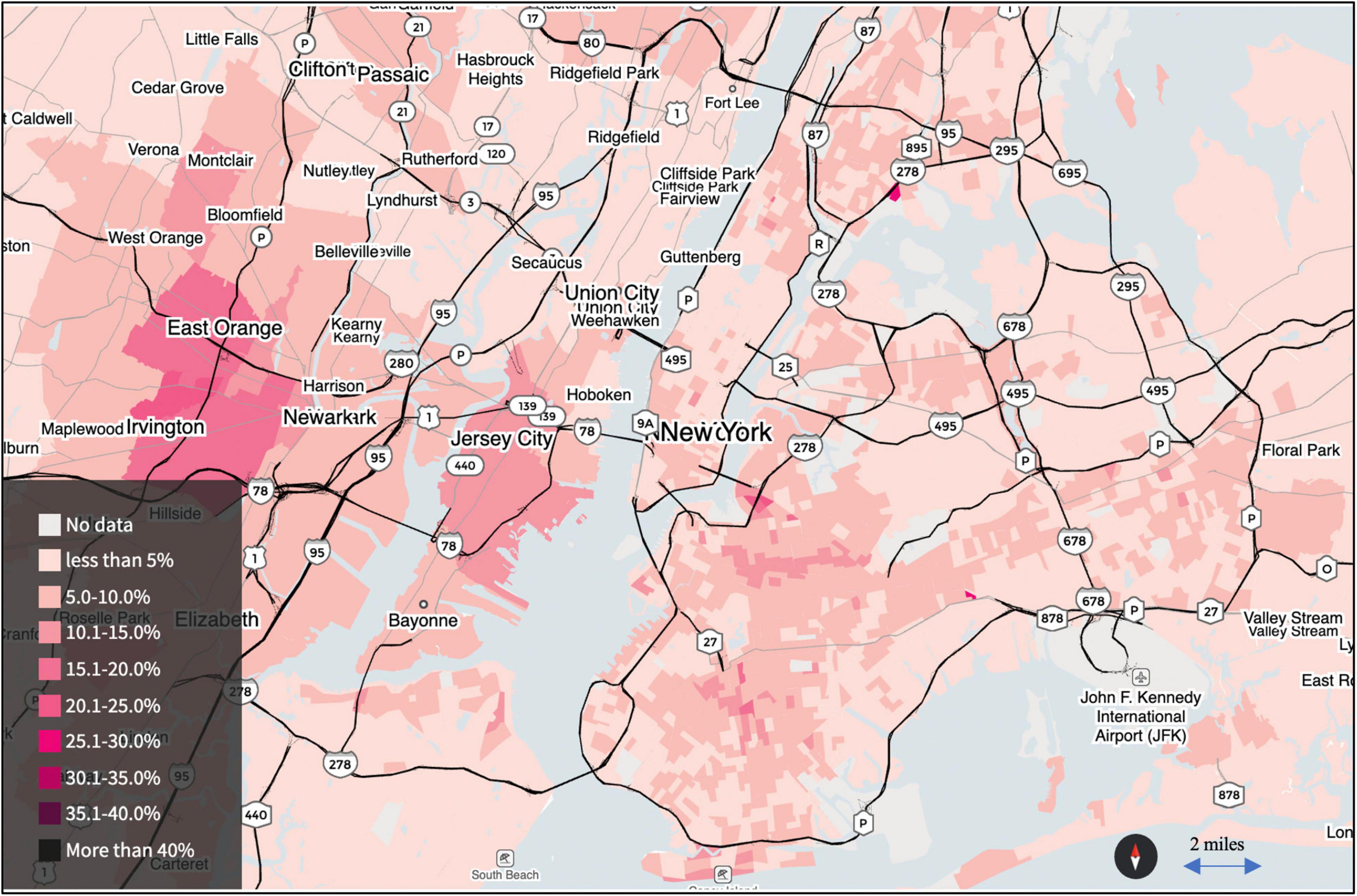
Figure 2. Map of children’s blood lead (BLL) data per census tract (2005–2014) in NYC created by Reuters. The CDC estimates that nationwide, around 2.5% of children ages 0–6 have an elevated lead level, defined as 5 mg/dL or higher (Scheneryer, 2017).
To achieve NYC’s goal to eliminate childhood Pb exposure, more research is needed on the degree to which soil Pb exposure affects children’s blood Pb levels. Exterior environmental sources of Pb dust can relocate more Pb to children’s hands (Viverette et al., 1996; Hunt et al., 2006; Laidlaw and Filippelli, 2008) than interior sources. Soil directly contributes to Pb found on children’s hands after outdoor play (Viverette et al., 1996), which occurs in private backyards and playgrounds that have been sinks for atmospheric Pb and tend to have higher Pb concentrations than public spaces (Cheng et al., 2015; Landes et al., 2019). Thus, it is crucial to investigate various sources of Pb exposure to assess their relative contribution as exposure pathways. Primary factors affecting the magnitude of sources include type and age of housing, home maintenance methods, proximity to major roads and industrial zones, socioeconomic factors, soil Pb, and water Pb. Studies relating to soil cover condition and garden Pb content in public areas, behavioral factors relating to children (children’s age, mouthing habits, time spent on playgrounds), and socioeconomic factors relating to the community (income, ethnicity, education, occupation) are also very important for the assessment of exposure pathways. Primary prevention is crucial for managing the associated risks of soil Pb in an urban environment; thus, public participation is necessary to lower childhood exposure (Mielke, 2015; LeadFreeNYC, 2019). Informed residents with basic remediation skills can directly reduce their children’s risk.
Identification of Pb exposure pathways affecting BLLs has to be done in collaboration with medical researchers and public health agencies to assess the relationship of environmental variables to actual health outcomes (i.e., lowered BLLs in children). Topics that need to be addressed include: the effectiveness of soil remediation in a neighborhood (e.g., importing clean topsoil) to lower Pb exposure in children, the impact of gardening activities on blood Pb of gardeners and their families, the impact of overall diet (e.g., calcium intake) of individuals on blood Pb, and the correlation between Pb concentrations in house dust and soil outside the house. Addressing these topics will be critical in reducing confusion around soil Pb contamination in urban gardening communities.
Exposure Through Garden Produce
There is great concern about the potential transfer of soil contaminants into vegetable crops when Pb and other toxicants contaminate garden soils. Contamination of vegetables grown in contaminated soil in a greenhouse has been shown to be dependent on soil total Pb concentrations, soil pH, and plant species (McBride, 2013). Exposure to contaminated produce can come via ingestion of shoots, roots, fruits, or adhered soil particles to any of these plant parts. This complexity and extent of produce contamination have been a major source of confusion for urban gardening stakeholder groups.
Plant Type
Crop contamination varies markedly with plant species and type. Zhou et al. (2016) found that crop contamination decreased as follows: leafy vegetables > stalk vegetables/root vegetables/solanaceous vegetables > legume vegetables/melon vegetables. Paltseva et al. (2020) found that onion > kale > eggplant > cabbage > tomato. Finster et al. (2004) found that most Pb was concentrated in the roots with some translocation into the shoots and that Pb was not concentrated in the edible parts of fruiting vegetable plants (beans, tomatoes, zucchini, peppers). Rather, edible leaf crops (Swiss chard, collard greens), herbs (mint, cilantro), and edible roots (carrot, onion, radish) had the highest levels of Pb (Finster et al., 2004). Studies by Paltseva et al. (2018, 2020) were consistent with previous work showing that crop type is more important than soil amendment type.
Contribution of Soil Particle Adherence/Entrapment to Vegetable Contamination
Plant tissues strongly retain soil solids, even after rigorous washing with soil-dispersing agents. This retention can significantly increase the Pb content of produce because soil usually has orders of magnitude higher Pb concentrations than plant tissues. McBride et al. (2014) found that vegetable Pb concentrations were correlated with Al concentrations and interpreted this observation as evidence that soil particle adherence was more important in determining vegetable contamination than root Pb uptake. This interpretation is based on the idea that because there is no biological need for Al, any Al in produce must be an indicator of soil particle adherence when soil is pH neutral or near-neutral. Sheppard and Evenden (1992) also determined that soil adhesion was a dominant pathway for plant accumulation of uranium, thorium, and Pb using plant/soil concentration ratios. Similar results were observed in case studies in New York and New Jersey (United States), where plant tissue Pb was correlated with plant tissue Al (Paltseva et al., 2018; Egendorf et al., 2021).
Certain crops, in particular, fruiting vegetables (e.g., tomato, eggplants, cucumbers), are much less susceptible to contamination than others. These crops should be prioritized in urban gardens. There should be a clear recommendation that crops more susceptible to contamination should be avoided unless thorough testing or prophylactic measures (e.g., construction of raised beds with clean soil) have been done.
Recontamination of Remediated Soils and Vegetable Surfaces
Although highly Pb-contaminated sites can be remediated by importing clean soil to replace or cover the existing surface soil, the environment is very dynamic, and recontamination can occur, particularly from aerial deposition (Engel-Di Mauro, 2021). Given that plant uptake of Pb from the soil is generally low, contamination by atmospheric sources has been detectable in various crops (Uzu et al., 2014; Amato-Lourenco et al., 2016; Shahid et al., 2017; Ma et al., 2019). Research has documented how contaminants can be lodged in tissues (Schreck et al., 2012) and absorbed by leaves (Uzu et al., 2010). However, more research is needed to effectively distinguish the role of metal speciation in foliar uptake, toxicity, compartmentation, and detoxification inside plants, especially in urban agriculture (Shahid et al., 2017).
Resuspension of contaminated soils have been shown to contribute to atmospheric Pb in Birmingham, Alabama, Chicago, Illinois, Detroit, Michigan, and Pittsburgh, Pennsylvania (Laidlaw et al., 2012), which has been linked to seasonal fluxes in elevated Blood Lead Levels (BLLs) of children (Havlena et al., 2009; Zahran et al., 2013a). Even though recontamination of remediated soils is expected to be a prolonged process, the possibility that clean soils imported into gardens could become Pb-contaminated again over decades warrants more study. As stated by Binns et al. (2004), “the long-term sustainability of intervention applications is unclear.” In addition, the effects of bioturbation and evapotranspiration of soil water on soil Pb translocation in capped and covered soils after remediation are still unknown. Thus, mitigating current contamination and preventing potential recontamination can be achieved by building raised beds, reducing dust, keeping soil moist, for example, with mulch. Adding compost also improves moisture retention, dilutes the overall contamination and can bind metals.
Bioaccessibility and Bioavailability
Variability of Lead Bioaccessibility in Urban Soils
A great source of complication in trace element contamination in soils is multiple forms of metals in soil. These forms vary significantly in their potential to cause harm in animals, including humans, as they are ingested or inhaled. “Knowledge of lead bioavailability is important because the amount of lead that actually enters the blood and body tissues from an ingested medium depends on the physical chemical properties of the lead and of the medium. For example, lead in soil may exist, at least in part, as poorly water-soluble minerals, and may also exist inside particles of inert matrices such as rock or slag of variable size, shape, and association. These chemical and physical properties may tend to influence (usually decrease) the absorption (bioavailability) of lead when ingested. Thus, equal ingested doses of different forms of lead in different media may not be of equal health concern” (USEPA, 2013).
Exposure may be assessed in vivo using Pb relative bioavailability (RBA; the fraction of soil-borne Pb absorbed into the systemic circulation following comparison to Pb absorbed from a Pb acetate reference dose) or by in vitro Pb bioaccessibility (IVBA) (the fraction of soil-borne Pb that is dissolved in simulated gastrointestinal [GI] solutions) (USEPA Oswer, 2007; USEPA, 2012; Kastury et al., 2019b). In vitro GI extraction tests have been developed to measure bioaccessible Pb concentrations as a predictor of bioavailable Pb concentrations. The Relative Bioaccessibility Leaching Procedure (RBALP) (Kelly et al., 2002; Drexler and Brattin, 2007), the Solubility/Bioavailability Research Consortium (SBRC) in vitro-gastric and in vitro-intestinal assays (Juhasz et al., 2009), EPA Method 1,340 are all based on a glycine solution extract using pH 1.5, or 2.5 in some cases. Other in vitro methods for measuring bioaccessibility have also been correlated with in vivo measurements, such as the PBET (Ruby et al., 1996), OSU IVG (Schroder et al., 2004), UBM, and BARGE (BARGE – INERIS, 2010; Wragg et al., 2011), RIVM (Oomen et al., 2003), USBLT (Chaney et al., 2011), and W-PBET (Furman et al., 2006) methods.
The mean values of Pb bioaccessibility in contaminated soils range widely from 3 to 59% (n = 140), according to the review conducted by Li et al. (2020). The authors found that SBRC produces the highest, followed by UBM, IVG, and DIN [German standard bioaccessibility methodology (DIN, 2000)] assays, with the PBET assay producing the lowest bioaccessibility values. The variability of results obtained by different methods leads to confusion when attempting to assess potential human exposure (especially children) by comparing results among tests.
It is important to note that due to the variation in bioaccessibility, bioaccessible Pb may not correlate with total Pb concentrations (i.e., some samples with low concentrations of total Pb may have a high fraction of bioaccessible Pb). Although Roussel et al. (2010) found significant positive correlations between Pb bioaccessibility (UBM model) and total Pb concentration in 27 urban contaminated soils, no correlations were found between total Pb concentration and its bioaccessibility in 20 soils from various sources based on the RBALP model (Morman et al., 2009), in 90 Dutch soils based on RIVM model (Hagens et al., 2009), in 28 soils polluted with various Pb sources (Walraven et al., 2015), or in 49 urban soils from NYC based on EPA Method 1340 at pH 2.5 (Paltseva et al., 2018). Regarding the effects of particle size of soil fractions on bioaccessibility, Li et al. (2021) established a trend of higher Pb bioaccessibility in the finer fractions based on the review of multiple studies. This trend is related to total metal concentration, its chemical form, and anthropogenic influence.
An obvious conclusion that emerges from the literature is that although many methods have been developed and used to determine the bioaccessibility of soil Pb, the results have been widely inconsistent. There is no consensus as to which method is more accurate than others. The US EPA Standard Method 1340 is also recognized as inappropriate for urban soils, which has highly variable Pb bioaccessibility (USEPA, 2017). This is a barrier to the clear transmission of information about risk to non-scientific audiences. To protect public health, a more conservative approach should be adopted, i.e., to assume a high bioaccessibility value before a reliable method can be found. Indeed, the failure of the ability of science to produce a well-defined and transparent assessment methodology underlies our recommendation to assume that any urban soil has a high potential for Pb contamination and associated risks.
Effects of Phosphate: In vitro and in vivo Studies on Urban Soils Amended With Phosphates
A complication in developing methods for assessing soil Pb bioavailability is complex interactions between phosphate and Pb (Miretzky and Fernandez-Cirelli, 2008). It has been generally understood that phosphate interacts with Pb to form insoluble pyromorphite minerals (Scheckel et al., 2013). These minerals stay largely insoluble after ingestion, and therefore, there is great interest in the ability of phosphate additions to decrease Pb bioavailability and bioaccessibility. However, the formation of Pb phosphates in typical urban soils with near-neutral pH and high organic matter content is likely very limited or slow even when soluble phosphate is added to the soil (Chrysochoou et al., 2007). In addition, lead is very water-insoluble in most urban soils and reacts extremely slowly with the added phosphate (Cai et al., 2017).
Phosphate treatment efficacy to reduce Pb bioavailability has been shown to depend on phosphate source, soil pH, Pb concentration, and competing ions based on the wide range of treatment effect ratio (TER) values (0.03–1.16) found in the literature (Scheckel et al., 2013; Juhasz et al., 2014). The application of liquified bone meal soil amendment to residential soils across Detroit, Michigan resulted in a 9.8% decrease in IVBA (Good, 2020). Greater immobilization efficacy (lower human bioaccessibility) of Pb (up to 19%) was observed for granular (2–4 mm diameter) compared to ground (< 0.5 mm) struvite (phosphate mineral) applied to urban agriculture sites in Chicago, Illinois (Gu et al., 2020).
Juhasz et al. (2014) demonstrated the possibility of pyromorphite formation in vivo (i.e., in the small intestines of mice) following solubilization of Pb and P in the stomach when phosphate amendments and Pb-contaminated soil were gavaged sequentially. They concluded that an initially soluble source of phosphate is more effective in immobilizing Pb than the much less soluble rock phosphate, which is not reflected by the in vitro assay. Kastury et al. (2019a) called for more extensive studies with different animal models and endpoints (e.g., Pb concentration in liver, kidney, and bones) to better predict Pb RBA in phosphate amended soils.
While phosphate has been shown to immobilize Pb, it can also mobilize As by increasing the solubility of soil As through competitive anion exchange (Peryea, 1991). Such complex interactions between Pb, phosphates, and As can bring unintended adverse consequences of phosphate-bearing amendments. Overall, from existing research, it can be concluded that it is often difficult to predict the effectiveness of many amendments in reducing Pb bioaccessibility, especially when information about other soil properties are not available. Urban soils are very heterogeneous, which makes the prediction of Pb bioaccessibility especially challenging. This is one of the major impediments to transmitting clear and useful information about urban gardening risks to stakeholders. A clear and useful recommendation is that in general, phosphate amendments cannot be relied upon to reduce risk to gardeners, and they should not be recommended blindly as a treatment for contaminated urban soils.
Rapid Assessment of Lead Bioaccessibility and Leachability With Field Portable X-Ray Fluorescence and Soil Lead Kits
One solution to the challenge of complex and confusing bioaccessibility testing protocols is the development of simple tools and methods to perform diagnostic screening to detect hotspots of contamination and associated risks. As discussed above, current methods for analyzing soil bioaccessibility in the lab are time-consuming and costly (Spliethoff et al., 2016). Urban soils, which are notable for their spatial heterogeneity, differ greatly in total and bioaccessible Pb concentrations over even small lateral or vertical distances, creating a need for large numbers of analyses.
One approach for low cost and rapid assessment of soil Pb bioaccessibility is based on portable X-Ray Fluorescence (pXRF) analyzers (Landes et al., 2019; Paltseva and Cheng, 2019b). These devices are relatively expensive (> $20,000) but are easy to use and can be made available to community groups with brief training. The procedure includes the following steps: (1) air-drying of soil samples, (2) extraction (0.4 M glycine) with a soil:extract ratio of 1:10 for 1 h at room temperature, (3) filtration of the extract, and (4) direct measurement of Pb in the liquid extract in cups by pXRF. The pXRF and ICP-MS measurements of the same extractants were highly correlated (y = 1.16x-2, R2 = 0.994). This procedure can also be used to estimate IVBA as described in Drexler and Brattin (2007) and EPA Method 1340 (USEPA, 2013). This estimation would involve measuring Pb in the field-procedure extract solution by XRF or ICP-MS and then estimating the Pb extracted by the Drexler and Brattin (DB) method as PbDB = (PbField_procedure – 89)/0.34 (Landes et al., 2019).
An additional approach to rapid assessments is the use of inexpensive test kits. Landes et al. (2019) developed a soil bioaccessibility kit (∼$5/test) to measure hazardous levels of Pb in soil. The procedure for this kit was derived from the IVBA method of Drexler and Brattin (2007) and EPA Method 1340 but uses a higher soil-to-solution ratio. Currently, there are two commercially available tests for Pb that detect Pb in different media, including soil. For example, Lead Soil Check test strips (∼$4.6–$6.3/test) estimate 100, 200, 300, and 400 ppm (mg/L) of bioaccessible Pb in less than 10 min. However, these tests may not be reliable due to interferences with zinc and other trace elements in urban soils and should be used with caution.
Management of Soil Contamination
Given that contaminants can pose health risks through direct ingestion of soil or dust or the consumption of contaminant-bearing produce, it is prudent to reduce children’s exposure to urban soils and gardens. However, playing outside, touching soil, observing soil and plant biodiversity, and growing food are practices that have been shown to have a significant positive effect on the intellectual and psychological development of a child (Koller et al., 2004; Moser and Martinsen, 2010; Chawla, 2015), especially for those in urban areas. Thus, there is great interest in the management and remediation of soil contamination.
Effective Methods of Soil Remediation
Ex situ Approaches
Remediation of metal-contaminated soils has been achieved by ex situ (i.e., removal of contaminated soil) or in situ (i.e., reduction of contaminant mobility by altering physical or chemical characteristics of the contaminated soil) processes. Ex situ treatments are often based on excavation and disposal in a landfill (Table 3) and replacing clean soil from another site (Deeb et al., 2020). An alternative approach is to “cap” contaminated soil to provide a physical barrier restricting access to contaminated soil and/or inhibit surface water infiltration from preventing release of contaminants to the surrounding areas or groundwater (Evanko and Dzombak, 1997). Removing contaminated soils is expensive and importing established topsoil reduces soil resources in one location to improve resources at another, which is ultimately unsustainable (Deeb et al., 2020).

Table 3. Remedial options for metal-contaminated soil (adapted from Karna et al., 2017).
Recent research has focused on mitigating exposure by building raised beds filled with constructed soils, or Technosols, which are defined as mixtures of organic and inorganic wastes and byproducts constructed to meet specific requirements (Egendorf et al., 2018; Deeb et al., 2020). In New York City, the Clean Soil Bank (CSB) program uses thoroughly tested and excavated sediments (deep subsoils) from construction projects to provide clean soils for municipal and community uses throughout the city. The program also produces constructed “topsoil” by amending sediments with compost particularly for urban gardens and farms (Walsh et al., 2018). Ensuring compost is tested for contaminants prior to use is an important consideration, as is assessing compost viability for supplying plant nutrients and monitoring for potential deposition or recontamination over time (Egendorf et al., 2018).
In New Orleans, Louisiana, fresh low-Pb alluvial deposits from the Mississippi River provide a great source of native soil materials. Projects using a geotextile soil cover (capping) showed the efficiency of remediation efforts at childcare centers in areas of the city where Pb concentrations exceed 400 mg kg–1 (Mielke et al., 2011). These projects demonstrated a relatively low cost and rapid method (only a few hours to complete) for reducing soil Pb on children’s play areas (Mielke, 2016).
While the use of Technosols in raised beds is quite promising, large-scale implementation and long-term studies of this method have not been done (Erdem and Nassauer, 2006), and some key issues need to be resolved. Raised beds do not remove existing underlying contamination, so barriers separating contaminated materials below must be maintained over time. Continued monitoring for a breakdown of barriers between the raised bed and contaminated subsoil is recommended. Soil caps may require periodic maintenance when breaches appear, which means continued costs over time. Planting crops with deep roots can also lead to root penetration into contaminated soils, which may present limited risks of uptake but may further degrade physical barriers. Warning signs indicating subsoil contamination should be posted on sites utilizing this method to inform future gardeners, urban planners, and landscape designers (Cooper et al., 2020).
An additional potential challenge with constructed soils is that the organic amendments such as composts used in their construction may have elevated concentrations of metals. Metals can accumulate and reach a higher level over time in soil after repeated use of these amendments. Hornick et al. (1980) noted high Pb levels (300 mg kg–1) in leaf compost, while Egendorf et al. (2018) found up to 226 mg Pb kg–1 in food waste and wood chip compost. Manure is usually a low-Pb organic amendment. However, near cities with smelters, manures may be heavily contaminated and should not be used (Chaney et al., 1984). Some modern swine and poultry feeding programs incorporate high Cu and/or Zn levels to increase feed use efficiency, often generating manure with as much Cu and Zn as sewage sludge (Mullins et al., 1982).
In situ Approaches
Remediation of metal-contaminated soil through in situ amendment applications can be uncertain because of various factors that influence geochemical properties and the specific metal immobilization mechanisms. For example, pH, conductivity, oxidation-reduction potential, and mineralogy can all influence the effectiveness of the amendments. Immobilization of metals mainly occurs through the addition of inorganic (e.g., fly ash, slag, zeolites), organic (e.g., biosolids, manures, paper pulp), or a combination of both inorganic and organic byproduct amendment types, often via the modification of soil pH and the precipitation of metal hydroxides (USEPA Office of Superfund Remediation and Technology Information, 2013).
Currently, organic byproducts (e.g., biosolids or food waste) are available in large volumes that can be used for remediation with such benefits as low cost, the proximity of the source to the remediation site, and/or a reduction in the use of virgin materials. The major environmental advantages of using “waste” byproducts as amendments are that wastes are diverted from landfills or surface impoundments, and the need to mine or synthetically produce a similar material is reduced.
Using biosolids (i.e., nutrient-rich organic materials derived from sewage sludge in the wastewater treatment process) (Palansooriya et al., 2020) and biosolids-based products, especially those high in Fe, have been recommended to reduce the bioavailability of Pb and other trace elements, thus, lessening human and ecological exposure (McIvor et al., 2012). However, because biosolids are a municipal byproduct, they contain many different synthetic organic and metal contaminants. Only a few of the contaminants are regulated, specifically nine metals and no synthetic organic pollutants, according to USEPA Office of Water (1995). A recent discovery of the fact that a substantial proportion of biosolids in the United States are contaminated by the non-degradable perfluoroalkyl substances (PFAS) (Stoiber et al., 2020) raises further concern about the use of these materials. However, the relative benefits and risks of biosolids use in urban agriculture should be assessed comprehensively and objectively in the context of climate change, waste management, food security, and public health.
Biochar produced at high temperatures has been shown to reduce metal mobility in soil (O’Connor et al., 2018). The effectiveness of biochar immobilization of Pb varies with feedstock type and application rate, biochar mixing depth, crop type, soil properties, and meteorological factors. However, the aging effect of biochar may reduce the efficiency of metal immobilization over time (O’Connor et al., 2018). The conversion of biosolids to biochar would be a promising way to increase biosolids’ use efficiency and safety. During the pyrolysis process, microbial and organic contaminants in biosolids are subjected to thermal breakdown, and metals such as and Hg tend to volatilize during the thermal conversion process, but other metals such as Pb may become more concentrated (Palansooriya et al., 2020).
Best Management Practices
To achieve the best results, it may be necessary to combine several soil remediation strategies and sustainable conservation practices to decrease contamination risk and prevent recontamination (Figure 3):
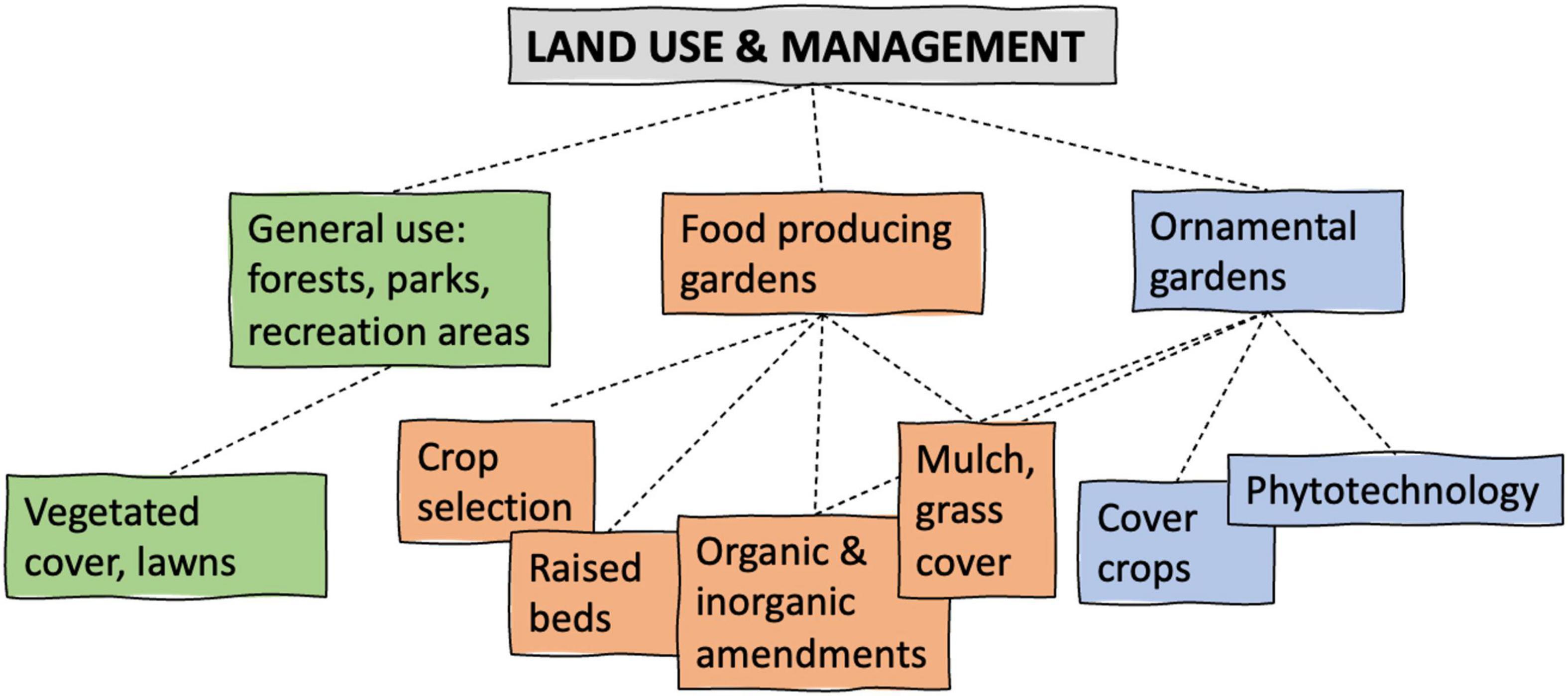
Figure 3. Managing strategies required for different land uses (modified from Vasenev et al., 2017).
• Dust control measures, e.g., mulch on top of contaminated soil and drip irrigation (moist soil), result in minimum resuspension of contaminated soil particles into the air as well as reduced splash or flooding (compared to other watering techniques), decreasing potential recontamination of the soil surface and above-ground plant tissues.
• The addition of organic matter (e.g., compost, biosolids, biochar) improves soil structure with more stable aggregates that adsorb contaminants and reduce erosion (Deeb et al., 2017), reducing the possibility of fine particle resuspension into the air.
• Conservation tillage practices that leave residue on the soil surface increase soil organic matter content that immobilizes metals by forming stable organo-metal complexes in the soil. The vegetation protects soil from raindrop impact and impedes overland flow, while root systems help bind the soil together to reduce runoff (Weil, 2015). These practices reduce topsoil erosion and decomposition rate of organic matter (Weil, 2015), enhance aggregate formation, diminish the possibility of particle resuspension into air or water, and prolong the effect of soil remediation by organic matter amendments.
• Liming increases soil pH, which reduces trace metal bioavailability and improves soil aggregation and structure (Lwin et al., 2018).
• Installing a garden at least 50 m away from heavy traffic avoids the accumulation of contaminants in the soil over time (Werkenthin et al., 2014).
• Climate change effects, especially temperature and precipitation extremes on soil contamination and metal mobility in soils, requires special attention. In the case of dry seasons, the addition of phosphate fertilizers, composts, and soil amendments may not form stable (and thus less bioavailable) forms of metals due to the lack of water content required for chemical reactions to occur (Paltseva and Neaman, 2020).
Conclusion
Gardening brings many benefits to urban residents such as nutritious food, grocery bill reduction, physical (diet, exercise) and mental (time spent in nature, pleasure) health benefits, a deeper connection to agriculture and urban nature, aesthetics, and promotion of biodiversity (Draper and Freedman, 2010; Clavin, 2011; Cameron et al., 2012; Chalmin-Pui et al., 2021). The importance of gardening to urban residents was made very apparent during the COVID-19 pandemic. It significantly contributed to human wellbeing by reducing anxiety, maintaining physical activities, and other effects (Sofo and Sofo, 2020; Chalmin-Pui et al., 2021). Raymond et al. (2019) identified emerging co-benefits of home gardening such as “the desire to leave a legacy for future generations, multiple sense of place benefits related to identity, family and friend bonding in place and the sheer enjoyment associated with incidental nature experiences like seeing a rare bird feed in their yard.” In the future, gardening may decrease the need to import food, encourage the use of indigenous and traditional seeds, and motivate local exchange of fruits, vegetables, flowers, and seeds (Židak and Osmanagić Bedenik, 2019).
Our review suggests that while encouraging individual or community urban gardening is important, information about this activity must be communicated carefully with comprehensive safety guidelines to raise awareness about soil contamination. In-person classes, hands-on workshops, and physical and digital references for gardeners would be beneficial and an option for gardeners to ask experts questions on soil heavy metals contamination (Balotin et al., 2020).
Lead is often found at high levels in soils near current and old industrial sites, old orchards, high-traffic streets and highways, and older houses. Rapid screening methods estimating total soil Pb can locate the hotspots for target investigation. However, better, more reliable testing kits that can be accessible to a layperson are still needed along with biodegradable sensors for monitoring Pb over time. Despite the abundance of scientific literature discussing soil contamination, cases of elevated children BLLs are still widely found worldwide.
Despite decades of research on soil Pb contamination in urban area clear, consistent, generally accessible, and understandable recommendations for safe urban gardening practices need to be effectively shared with the public. The failure of science to produce a well-defined and transparent assessment methodology underlies our recommendation to assume that soil of any urban area has a high potential for Pb contamination. This paper suggests that urban gardening should not begin until thorough testing, mitigation, or remediation measures have been taken. Another recommendation would be to include land-use history in all property transactions so that the potential for soil contamination can be clearly identified in urban and suburban areas.
Certain crops such as fruiting vegetables, in particular, are much less susceptible to contamination than others. These crops should be prioritized in urban gardens. There should be a clear recommendation that crops that are more susceptible to contamination should be avoided unless thorough testing or ex situ remediation (e.g., construction of Technosols) has been done. There is significant uncertainty when soil amendments are used to reduce risk to gardeners. Compost has many beneficial effects on soil structure, metal solubility, and plant growth, reducing toxic metal concentrations in crops. However, ongoing monitoring of composts and soils is necessary to prevent accumulation of undesired trace elements in remediated soils over time.
Author Contributions
AP, ZC, MD, and PG contributed to conception and design of the study. AP wrote the first draft of the manuscript. ZC, PG, MD, MM, and SPE contributed to manuscript revision, read, and approved the submitted version. All authors contributed to the article and approved the submitted version.
Funding
The present study was partially supported by the Strategic Leadership Program. AP was partially supported by the Russian Science Foundation project 19−77−30012. This research was supported in part by the Cornell Atkinson Center for Sustainability.
Conflict of Interest
The authors declare that the research was conducted in the absence of any commercial or financial relationships that could be construed as a potential conflict of interest.
Publisher’s Note
All claims expressed in this article are solely those of the authors and do not necessarily represent those of their affiliated organizations, or those of the publisher, the editors and the reviewers. Any product that may be evaluated in this article, or claim that may be made by its manufacturer, is not guaranteed or endorsed by the publisher.
References
Ajmone-Marsan, F., and Biasioli, M. (2010). Trace elements in soils of urban areas. Water Air Soil Pollut. 213, 121–143. doi: 10.1007/s11270-010-0372-6
Alekseenko, V., and Alekseenko, A. (2014). The abundances of chemical elements in urban soils. J. Geochem. Explor. 147, 245–249. doi: 10.1016/j.gexplo.2014.08.003
Alloway, B. J. (2004). Contamination of domestic gardens and allotments. Land Contam. Reclam. 12, 179–187. doi: 10.2462/09670513.658
Amato-Lourenco, L. F., Moreira, T. C. L., de Oliveira Souza, V. C., Barbosa, F., Saiki, M., Saldiva, P. H. N., et al. (2016). The influence of atmospheric particles on the elemental content of vegetables in urban gardens of Sao Paulo, Brazil. Environ. Pollut. 216, 125–134. doi: 10.1016/j.envpol.2016.05.036
Antoniadis, V., Shaheen, S. M., Levizou, E., Shahid, M., Niazi, N. K., Vithanage, M., et al. (2019). A critical prospective analysis of the potential toxicity of trace element regulation limits in soils worldwide: are they protective concerning health risk assessment? – a review. Environ. Int. 127, 819–847. doi: 10.1016/j.envint.2019.03.039
Aschengrau, A., Beiser, A., Bellinger, D., Copenhafer, D., and Weitzman, M. (1994). The impact of soil lead abatement on urban children’s blood lead levels: phase II results from the boston lead-in-soil demonstration project. Environ. Res. 67, 125–148. doi: 10.1006/ENRS.1994.1069
Ashrafzadeh, S., Lehto, N. J., Oddy, G., McLaren, R. G., Kang, L., Dickinson, N. M., et al. (2018). Heavy metals in suburban gardens and the implications of land-use change following a major earthquake. Appl. Geochem. 88, 10–16. doi: 10.1016/j.apgeochem.2017.04.009
Balotin, L., Distler, S., Williams, A., Peters, S. J. W., Hunter, C. M., Theal, C., et al. (2020). Atlanta residents’ knowledge regarding heavy metal exposures and remediation in urban agriculture. Int. J. Environ. Res. Public Health 17, 7–10. doi: 10.3390/ijerph17062069
BARGE – INERIS (2010). UBM Procedure for the Measurement of Inorganic Contaminant Bioaccessibility from Solid Matrices. Available online at: https://www.bgs.ac.uk/barge/docs/BARGE_UBM_DEC_2010.pdf
Bechet, B., Joimel, S., Jean-Soro, L., Hursthouse, A., Agboola, A., Leitão, T. E., et al. (2018). Spatial variability of trace elements in allotment gardens of four European cities: assessments at city, garden, and plot scale. J. Soils Sediments 18, 391–406. doi: 10.1007/s11368-016-1515-1
Biasioli, M., Grcman, H., Kralj, T., Madrid, F., Dıaz-Barrientos, E., and Ajmone-Marsan, F. (2007). Potentially toxic elements contamination in urban soils: a comparison of three European cities. J. Environ. Qual. 36, 70–79.
Bidar, G., Pelfrêne, A., Schwartz, C., Waterlot, C., Sahmer, K., Marot, F., et al. (2020). Urban kitchen gardens: effect of the soil contamination and parameters on the trace element accumulation in vegetables – a review. Sci. Total Environ. 738:139569. doi: 10.1016/j.scitotenv.2020.139569
Binns, H. J., Gray, K. A., Chen, T., Finster, M. E., Peneff, N., Schaefer, P., et al. (2004). Evaluation of landscape coverings to reduce soil lead hazards in urban residential yards: the safer yards project. Environ. Res. 96, 127–138. doi: 10.1016/j.envres.2004.02.010
Birke, M., and Rauch, U. (2000). Urban geochemistry: investigations in the Berlin metropolitan area. Environ. Geochem. Health 22, 233–248. doi: 10.1023/A:1026554308673
Buiso, G. (2014a). Root of all Evil: Vegetables in NYC Gardens are “Toxic” [WWW Document]. New York Post. Available online at: https://nypost.com/2014/11/16/toxic-veggies-found-in-nycs-community-gardens/ (accessed October 1, 19).
Buiso, G. (2014b). Why NYC’s Toxic Community Gardens May Give You Cancer. New York, NY: New York Post.
Burghardt, W., Morel, J. L., and Zhang, G.-L. (2015). Development of the soil research about urban, industrial, traffic, mining and military areas (SUITMA). Soil Sci. Plant Nutr. 61, 3–21. doi: 10.1080/00380768.2015.1046136
Cai, M., McBride, M. B., Li, K., and Li, Z. (2017). Bioaccessibility of As and Pb in orchard and urban soils amended with phosphate, Fe oxide and organic matter. Chemosphere 173, 153–159. doi: 10.1016/j.chemosphere.2017.01.049
Cal-Prieto, M. J., Carlosena, A., Andrade, J. M., Martínez, M. L., Muniategui, S., López-Mahía, P., et al. (2001). Antimony as a tracer of the anthropogenic influence on soils and estuarine sediments. Water Air Soil Pollut. 129, 333–348. doi: 10.1023/A:1010360518054
Cameron, R. W. F., Blanuša, T., Taylor, J. E., Salisbury, A., Halstead, A. J., Henricot, B., et al. (2012). The domestic garden – its contribution to urban green infrastructure. Urban Forest. Urban Green. 11, 129–137. doi: 10.1016/j.ufug.2012.01.002
Chalmin-Pui, L. S., Griffiths, A., Roe, J., Heaton, T., and Cameron, R. (2021). Why garden? – attitudes and the perceived health benefits of home gardening. Cities 112:103118. doi: 10.1016/j.cities.2021.103118
Chaney, R. L., Sterrett, S. B., and Mielke, H. W. (1984). “The potential for heavy metal exposure from urban gardens and soils,” in Heavy Metals in Urban Gardens, ed. J. R. Preer (Washington, DC: University District Columbia Extension Service), 37–84.
Chaney, R. L., Zia, M. H., Scheckel, K. G., and Codling, E. (2011). “Simplified urban soil bioaccessible Pb test correlated with bioavailability of soil-Pb to humans in untreated and phosphate-treated Joplin soils,” in Proceedings of the International Symposium on Biogeochemistry of Trace Elements, Vol. 4, Florence.
Chen, T., Zheng, Y. M., Lei, M., Huang, Z. C., Wu, H. T., Chen, H., et al. (2005). Assessment of heavy metal pollution in surface soils of urban parks in Beijing, China. Chemosphere 60, 542–551. doi: 10.1016/j.chemosphere.2004.12.072
Cheng, H., Li, M., Zhao, C., Li, K., Peng, M., Qin, A., et al. (2014). Overview of trace metals in the urban soil of 31 metropolises in China. J. Geochem. Explor. 139, 31–52. doi: 10.1016/j.gexplo.2013.08.012
Cheng, Z., Paltseva, A., Li, I., Morin, T., Huot, H., Egendorf, S., et al. (2015). Trace metal contamination in New York city garden soils. Soil Sci. 180, 167–174. doi: 10.1097/SS.0000000000000126
Chrysochoou, M., Dermatas, D., and Grubb, D. G. (2007). Phosphate application to firing range soils for Pb immobilization: the unclear role of phosphate. J. Hazard. Mater. 144, 1–14. doi: 10.1016/j.jhazmat.2007.02.008
Clavin, A. A. (2011). Realising ecological sustainability in community gardens: a capability approach. Local Environ. 16, 945–962. doi: 10.1080/13549839.2011.627320
Cooper, A. M., Felix, D., Alcantara, F., Zaslavsky, I., Work, A., Watson, P. L., et al. (2020). Monitoring and mitigation of toxic heavy metals and arsenic accumulation in food crops: a case study of an urban community garden. Plant Direct 4:e00198. doi: 10.1002/pld3.198
Czarnowska, K. (1980). Akumulacja metali ciezick w glebach, oslinach i niektoych zwieretach na erenie. Warzawy Rocz Glebozn 31, 77–115.
De Miguel, E., Jiménez de Grado, M., Llamas, J. F., Martín-Dorado, A., and Mazadiego, L. F. (1998). The overlooked contribution of compost application to the trace element load in the urban soil of Madrid (Spain). Sci. Total Environ. 215, 113–122. doi: 10.1016/S0048-9697(98)00112-0
Deeb, M., Desjardins, T., Podwojewski, P., Pando, A., Blouin, M., and Lerch, T. Z. (2017). Interactive effects of compost, plants and earthworms on the aggregations of constructed technosols. Geoderma 305, 305–313. doi: 10.1016/j.geoderma.2017.06.014
Deeb, M., Groffman, P. M., Blouin, M., Perl Egendorf, S., Vergnes, A., Vasenev, V., et al. (2020). Using constructed soils for green infrastructure – challenges and limitations. Soil 6, 413–434. doi: 10.5194/soil-6-413-2020
DIN (2000). Soil Quality-Absorption Availability of Organic and Inorganic Pollutants from Contaminated Soil Material. Deutsches Institut fur Normung e.V. Available online at: https://www.techstreet.com/standards/din-19738?product_id=1982137#document
Draper, C., and Freedman, D. (2010). Review and analysis of the benefits, purposes, and motivations associated with community gardening in the United States. J. Commun. Pract. 18, 458–492. doi: 10.1080/10705422.2010.519682
Drexler, J. W., and Brattin, W. J. (2007). An in vitro procedure for estimation of lead relative bioavailability: with validation. Hum. Ecol. Risk Assess. 13, 383–401.
Egendorf, S. P., Cheng, Z., Deeb, M., Flores, V., Paltseva, A., Walsh, D., et al. (2018). Constructed soils for mitigating lead (Pb) exposure and promoting urban community gardening: the New York city clean soil bank pilot study. Landsc. Urban Plann. 175, 184–194. doi: 10.1016/j.landurbplan.2018.03.012
Egendorf, S. P., Spliethoff, H. M., Shayler, H. A., Russell-Anelli, J., Cheng, Z., Minsky, A. H., et al. (2021). Soil lead (Pb) and urban grown lettuce: sources, processes, and implications for gardener best management practices. J. Environ. Manag. 286:112211. doi: 10.1016/j.jenvman.2021.112211
Eigenbrod, C., and Gruda, N. (2015). Urban vegetable for food security in cities. A review. Agron. Sustain. Dev. 35, 483–498. doi: 10.1007/s13593-014-0273-y
Eizenberg, E. (2013). From the Ground Up: Community Gardens in New York City and the Politics of Spatial Transformation. Farnham: Ashgate publishing Limited.
Engel-Di Mauro, S. (2021). Atmospheric sources of trace element contamination in cultivated urban areas: a review. J. Environ. Qual. 50, 38–48.
Erdem, M., and Nassauer, J. I. (2006). Design of brownfield landscapes under different contaminant remediation policies in Europe and the United States. Landsc. J. 32, 277–292.
Evanko, C. R., and Dzombak, D. A. (1997). Remediation of Metals-Contaminated Soils and Groundwater. Pittsburgh, PA: Ground-water remediation technologies analysis center, 5–13.
Finster, M. E., Gray, K. A., and Binns, H. J. (2004). Lead levels of edibles grown in contaminated residential soils: a field survey. Sci. Total Environ. 320, 245–257. doi: 10.1016/j.scitotenv.2003.08.009
Furman, O., Strawn, D. G., Heinz, G. H., and Williams, B. (2006). Risk assessment test for lead bioaccessibility to waterfowl in mine-impacted soils. J. Environ. Qual. 35, 450–458. doi: 10.2134/jeq2005.0316
Gailey, A. D., Schachter, A. E., Egendorf, S. P., and Mielke, H. W. (2020). Quantifying soil contamination and identifying interventions to limit health risks. Curr. Probl. Pediatr. Adolesc. Health Care 50:100740. doi: 10.1016/j.cppeds.2019.100740
Gamble, A. V., Givens, A. K., and Sparks, D. L. (2018). Arsenic speciation and availability in orchard soils historically contaminated with lead arsenate. J. Environ. Qual. 128, 121–128. doi: 10.2134/jeq2017.07.0264
Glennon, M. M., Harris, P., Ottesen, R. T., Scanlon, R. P., and O’Connor, P. J. (2014). The Dublin SURGE project: geochemical baseline for heavy metals in topsoils and spatial correlation with historical industry in Dublin, Ireland. Environ. Geochem. Health 36, 235–254. doi: 10.1007/s10653-013-9561-8
Good, S. R. (2020). A Field Study Of Bioaccessible Lead In Detroit Soils: Insight Into The Effectiveness Of Phosphate-Based Lead Sequestration. Ph.D. thesis. Detroit, MI: Wayne State University.
Gu, C., Gates, B. A., and Margenot, A. J. (2020). Phosphate recycled as struvite immobilizes bioaccessible soil lead while minimizing environmental risk. J. Clean. Prod. 276:122635. doi: 10.1016/j.jclepro.2020.122635
Gupta, N., Yadav, K. K., Kumar, V., Kumar, S., Chadd, R. P., and Kumar, A. (2019). Trace elements in soil-vegetables interface: translocation, bioaccumulation, toxicity and amelioration – a review. Sci. Total Environ. 651, 2927–2942. doi: 10.1016/j.scitotenv.2018.10.047
Hagens, W. I., Walraven, N., Minekus, M., Havenaar, R., Lijzen, J. P. A., and Oomen, A. G. (2009). Relative Oral Bioavailability of Lead from Dutch Made Grounds. RIVM Report 711701086A. Utrecht: National Institute for Public Health and the Environment.
Hamilton, A. J., Burry, K., Mok, H.-F., Barker, S. F., Grove, J. R., and Williamson, V. G. (2014). Give peas a chance? Urban agriculture in developing countries. A review. Agron. Sustain. Dev. 34, 45–73. doi: 10.1007/s13593-013-0155-8
Hanfi, M. Y., and Yarmoshenko, I. V. (2020). Health risk assessment quantification from heavy metals contamination in the urban soil and urban surface deposited sediment. J. Taibah Univ. Sci. 14, 285–293. doi: 10.1080/16583655.2020.1735735
Harms, A. M. R., Presley, D. R., Hettiarachchi, G. M., and Thien, S. J. (2013). Assessing the educational needs of urban gardeners and farmers on the subject of soil contamination. J. Extens. 51:1FEA10.
Havlena, J., Kanarek, M. S., and Coons, M. (2009). Factors associated with the seasonality of blood lead levels among preschool Wisconsin children. WMJ 108, 151–155.
Hornick, S. B., Patterson, J. C., and Chaney, R. L. (1980). “An evaluation of urban garden soil, vegetation, and soil amendments,” in Proceedings of the 2nd Conference on Scientific Research in National Parks, Vol. 3, San Francisco, CA, 158–167.
Hough, R. L., Breward, N., Young, S. D., Crout, N. M. J., Tye, A. M., Moir, A. M., et al. (2004). Assessing potential risk of heavy metal exposure from consumption of home-produced vegetables by urban populations. Environ. Health Perspect. 112, 215–221. doi: 10.1289/ehp.5589
Howard, J., Weyhrauch, J., Loriaux, G., Schultz, B., and Baskaran, M. (2019). Contributions of artifactual materials to the toxicity of anthropogenic soils and street dusts in a highly urbanized terrain. Environ. Pollut. 255:113350. doi: 10.1016/j.envpol.2019.113350
Hunt, A., Johnson, D. L., and Griffith, D. A. (2006). Mass transfer of soil indoors by track-in on footwear. Sci. Total Environ. 370, 360–371. doi: 10.1016/j.scitotenv.2006.07.013
Hunter, C. M., Williamson, D. H. Z., Gribble, M. O., Bradshaw, H., Pearson, M., Saikawa, E., et al. (2019). Perspectives on heavy metal soil testing among community gardeners in the united states: a mixed methods approach. Int. J. Environ. Res. Public Health 16:2350. doi: 10.3390/ijerph16132350
Huong, N. T. L., Ohtsubo, M., Li, L., Higashi, T., and Kanayama, M. (2010). Heavy-metal contamination of soil and vegetables in wastewater-irrigated agricultural aoil in a suburban area of Hanoi, Vietnam. Commun. Soil Sci. Plant Anal. 41, 390–407. doi: 10.1080/00103620903494350
Izquierdo, M., De Miguel, E., Ortega, M. F., and Mingot, J. (2015). Bioaccessibility of metals and human health risk assessment in community urban gardens. Chemosphere 135, 312–318. doi: 10.1016/j.chemosphere.2015.04.079
Johnson, D. L., and Bretsch, J. K. (2002). Soil lead and children’s blood lead levels in Syracuse, NY, USA. Environ. Geochem. Health 24, 375–385. doi: 10.1023/A:1020500504167
Juhasz, A. L., Gancarz, D., Herde, C., McClure, S., Scheckel, K. G., and Smith, E. (2014). In situ formation of pyromorphite is not required for the reduction of in vivo Pb relative bioavailability in contaminated soils. Environ. Sci. Technol. 48, 7002–7009. doi: 10.1021/es500994u
Juhasz, A. L., Weber, J., Smith, E., Naidu, R., Marschner, B., Rees, M., et al. (2009). Evaluation of SBRC-gastric and SBRC-intestinal methods for the prediction of in vivo relative lead bioavailability in contaminated soils. Environ. Sci. Technol. 43, 4503–4509. doi: 10.1021/es803238u
Karna, R. R., Luxton, T., Bronstein, K. E., Hoponick Redmon, J., and Scheckel, K. G. (2017). State of the science review: potential for beneficial use of waste by-products for in situ remediation of metal-contaminated soil and sediment. Crit. Rev. Environ. Sci. Technol. 47, 65–129. doi: 10.1080/10643389.2016.1275417
Kastury, F., Placitu, S., Boland, J., Karna, R. R., Scheckel, K. G., Smith, E., et al. (2019a). Relationship between Pb relative bioavailability and bioaccessibility in phosphate amended soil: uncertainty associated with predicting Pb immobilization efficacy using in vitro assays. Environ. Int. 131:104967. doi: 10.1016/j.envint.2019.104967
Kastury, F., Smith, E., Lombi, E., Donnelley, M. W., Cmielewski, P. L., Parsons, D. W., et al. (2019b). Dynamics of lead bioavailability and speciation in indoor dust and X-ray spectroscopic investigation of the link between ingestion and inhalation pathways. Environ. Sci. Technol. 53, 11486–11495. doi: 10.1021/acs.est.9b03249
Kelly, J., Thornton, I., and Simpson, P. R. (1996). Urban geochemistry: a study of the influence of anthropogenic activity on the heavy metal content of soils in traditionally industrial and non-industrial areas of Britain. Appl. Geochem. 11, 363–370.
Kelly, M. E., Brauning, S. E., Schoof, R. A., and Ruby, M. V. (2002). Assessing Oral Bioavailability of Metals in Soil. Columbus, OH: Battelle Press.
Kim, B. F., Poulsen, M. N., Margulies, J. D., Dix, K. L., Palmer, A. M., and Nachman, K. E. (2014). Urban community gardeners’ knowledge and perceptions of soil contaminant risks. PLoS One 9:e87913. doi: 10.1371/journal.pone.0087913
Koller, K., Brown, T., Spurgeon, A., and Levy, L. (2004). Recent developments in low-level lead exposure and intellectual impairment in children. Environ. Health Perspect. 112, 987–994.
Laidlaw, M. A. S., Alankarage, D. H., Reichman, S. M., Taylor, M. P., and Ball, A. S. (2018). Assessment of soil metal concentrations in residential and community vegetable gardens in Melbourne, Australia. Chemosphere 199, 303–311. doi: 10.1016/j.chemosphere.2018.02.044
Laidlaw, M. A. S., and Filippelli, G. M. (2008). Resuspension of urban soils as a persistent source of lead poisoning in children: a review and new directions. Appl. Geochem. 23, 2021–2039. doi: 10.1016/j.apgeochem.2008.05.009
Laidlaw, M. A. S., Filippelli, G. M., Brown, S., Paz-Ferreiro, J., Reichman, S. M., Netherway, P., et al. (2017). Case studies and evidence-based approaches to addressing urban soil lead contamination. Appl. Geochem. 83, 14–30. doi: 10.1016/j.apgeochem.2017.02.015
Laidlaw, M. A. S., Zahran, S., Mielke, H. W., Taylor, M. P., and Filippelli, G. M. (2012). Re-suspension of lead contaminated urban soil as a dominant source of atmospheric lead in Birmingham, Chicago, Detroit and Pittsburgh, USA. Atmos. Environ. 49, 302–310. doi: 10.1016/j.atmosenv.2011.11.030
Lal, R. (2020). Home gardening and urban agriculture for advancing food and nutritional security in response to the COVID-19 pandemic. Food Secur. 12, 871–876. doi: 10.1007/s12571-020-01058-3
Landes, F. C., Paltseva, A., Sobolewski, J. M., Cheng, Z., Ellis, T. K., Mailloux, B. J., et al. (2019). A field procedure to screen soil for hazardous lead. Anal. Chem. 91, 8192–8198. doi: 10.1021/acs.analchem.9b00681
Lanphear, B. P., Rauch, S., Auinger, P., Allen, R. W., and Hornung, R. W. (2018). Low-level lead exposure and mortality in US adults: a population-based cohort study. Lancet Public Health 3, e177–e184. doi: 10.1016/S2468-2667(18)30025-2
Lawson, L., and Drake, L. (2013). Community gardening organization survey 2011-2012. Community Green. Rev. 18, 20–47.
Lee, C. S. L., Li, X., Shi, W., Cheung, S. C. N., and Thornton, I. (2006). Metal contamination in urban, suburban, and country park soils of Hong Kong: a study based on GIS and multivariate statistics. Sci. Total Environ. 356, 45–61. doi: 10.1016/j.scitotenv.2005.03.024
Li, H.-B., Li, M.-Y., Zhao, D., Li, J., Li, S.-W., Xiang, P., et al. (2020). Arsenic, lead, and cadmium bioaccessibility in contaminated soils: measurements and validations. Crit. Rev. Environ. Sci. Technol. 50, 1303–1338. doi: 10.1080/10643389.2019.1656512
Li, Y., Padoan, E., and Ajmone-Marsan, F. (2021). Soil particle size fraction and potentially toxic elements bioaccessibility: a review. Ecotoxicol. Environ. Saf. 209:111806. doi: 10.1016/j.ecoenv.2020.111806
Lwin, C. S., Seo, B.-H., Kim, H.-U., Owens, G., and Kim, K.-R. (2018). Application of soil amendments to contaminated soils for heavy metal immobilization and improved soil quality—a critical review. Soil Sci. Plant Nutr. 64, 156–167. doi: 10.1080/00380768.2018.1440938
Ma, C., Liu, F.-Y., Hu, B., Wei, M.-B., Zhao, J.-H., Zhang, K., et al. (2019). Direct evidence of lead contamination in wheat tissues from atmospheric deposition based on atmospheric deposition exposure contrast tests. Ecotoxicol. Environ. Saf. 185:109688. doi: 10.1016/j.ecoenv.2019.109688
MacNair, G. (2004). Valuation of Contaminated Properties: A Canadian Perspective, Canadian Appraiser.
McBride, M. B. (2013). Arsenic and lead uptake by vegetable crops grown on historically contaminated orchard soils. Appl. Environ. Soil Sci. 2013:283472. doi: 10.1155/2013/283472
McBride, M. B., Shayler, H. A., Spliethoff, H. M., Mitchell, R. G., Marquez-Bravo, L. G., Ferenz, G. S., et al. (2014). Concentrations of lead, cadmium and barium in urban garden-grown vegetables: the impact of soil variables. Environ. Pollut. 194, 254–261. doi: 10.1016/j.envpol.2014.07.036
McIvor, K., Cogger, C., and Brown, S. (2012). Effects of biosolids based soil products on soil physical and chemical properties in urban gardens. Compost Sci. Util. 20, 199–206. doi: 10.1080/1065657X.2012.10737049
Mielke, H., Gonzales, C., Powell, E., and Mielke, P. (2005). Changes of multiple metal accumulation (MMA) in New Orleans soil: preliminary evaluation of differences between survey I (1992) and survey II (2000). Int. J. Environ. Res. Public Health 2, 308–313. doi: 10.3390/ijerph2005020016
Mielke, H. W. (2015). Soils and health: closing the soil knowledge gap. Soil Horizons 56, 1–3. doi: 10.2136/sh2015-56-4-gc
Mielke, H. W. (2016). Nature and extent of metal-contaminated soils in urban environments (keynote talk). Environ. Geochem. Health 38, 987–999. doi: 10.1007/s10653-016-9792-6
Mielke, H. W., Anderson, J. C., Berry, K. J., Mielke, P. W., Chaney, R. L., and Leech, M. (1983). Lead concentrations in inner-city soils as a factor in the child lead problem. Am. J. Public Health 73, 1366–1369. doi: 10.2105/AJPH.73.12.1366
Mielke, H. W., Gonzales, C. R., and Mielke, P. W. (2011). The continuing impact of lead dust on children’s blood lead: comparison of public and private properties in New Orleans. Environ. Res. 111, 1164–1172. doi: 10.1016/j.envres.2011.06.010
Mielke, H. W., Gonzales, C. R., Powell, E. T., Laidlaw, M. A. S., Berry, K. J., Mielke, P. W., et al. (2019). The concurrent decline of soil lead and children’s blood lead in New Orleans. Proc. Natl. Acad. Sci. U.S.A. 116, 22058–22064. doi: 10.1073/pnas.1906092116
Mielke, H. W., Gonzales, C. R., Powell, E. T., and Mielke, P. W. (2016). Spatiotemporal dynamic transformations of soil lead and children’s blood lead ten years after Hurricane Katrina: new grounds for primary prevention. Environ. Int. 94, 567–575. doi: 10.1016/j.envint.2016.06.017
Mielke, H. W., Wang, G., Gonzales, C. R., Powell, E. T., Le, B., and Quach, V. N. (2004). PAHs and metals in the soils of inner-city and suburban New Orleans, Louisiana, USA. Environ. Toxicol. Pharmacol. 18, 243–247. doi: 10.1016/j.etap.2003.11.011
Miretzky, P., and Fernandez-Cirelli, A. (2008). Phosphates for Pb immobilization in soils: a review. Environ. Chem. Lett. 6, 121–133. doi: 10.1007/s10311-007-0133-y
Möller, A., Müller, H. W., Abdullah, A., Abdelgawad, G., and Utermann, J. (2005). Urban soil pollution in Damascus, Syria: concentrations and patterns of heavy metals in the soils of the Damascus Ghouta. Geoderma 124, 63–71. doi: 10.1016/j.geoderma.2004.04.003
Morman, S. A., Plumlee, G. S., and Smith, D. B. (2009). Application of in vitro extraction studies to evaluate element bioaccessibility in soils from a transect across the United States and Canada. Appl. Geochem. 24, 1454–1463. doi: 10.1016/j.apgeochem.2009.04.015
Moser, T., and Martinsen, M. T. (2010). The outdoor environment in Norwegian kindergartens as pedagogical space for toddlers’ play, learning and development. Eur. Early Childh. Educ. Res. J. 18, 457–471. doi: 10.1080/1350293X.2010.525931
Mullins, G. L., Martens, D. C., Miller, W. P., Kornegay, E. T., and Hallock, D. L. (1982). Copper availability, form, and mobility in soils from three annual copper-enriched hog manure applications. J. Environ. Qual. 11, 316–320. doi: 10.2134/jeq1982.00472425001100020033x
National Gardening Association (2014). National Gardening Association Special Report. Garden to Table. A 5-Year Look at Food Gardening in America. South Burlington, VT: National Gardening Association.
Navarrete, I. A., Gabiana, C. C., Dumo, J. R. E., Salmo, S. G., Guzman, M. A. L. G., Valera, N. S., et al. (2017). Heavy metal concentrations in soils and vegetation in urban areas of Quezon City, Philippines. Environ. Monit. Assess. 189:145. doi: 10.1007/s10661-017-5849-y
Nussbaumer-Streit, B., Yeoh, B., Griebler, U., Pfadenhauer, L. M., Busert, L. K., Lhachimi, S. K., et al. (2016). Household interventions for preventing domestic lead exposure in children. Cochrane Database Syst Rev. 10:CD006047. doi: 10.1002/14651858.CD006047.pub5
O’Connor, D., Peng, T., Zhang, J., Tsang, D. C. W., Alessi, D. S., Shen, Z., et al. (2018). Biochar application for the remediation of heavy metal polluted land: a review of in situ field trials. Sci. Total Environ. 619–620, 815–826. doi: 10.1016/J.SCITOTENV.2017.11.132
Oomen, A. G., Rompelberg, C. J. M., Bruil, M. A., Dobbe, C. J. G., Pereboom, D. P. K. H., and Sips, A. J. A. M. (2003). Development of an in vitro digestion model for estimating the bioaccessibility of soil contaminants. Arch. Environ. Contam. Toxicol. 44, 281–287. doi: 10.1007/s00244-002-1278-0
Palansooriya, K. N., Shaheen, S. M., Chen, S. S., Tsang, D. C. W., Hashimoto, Y., Hou, D., et al. (2020). Soil amendments for immobilization of potentially toxic elements in contaminated soils: a critical review. Environ. Int. 134:105046. doi: 10.1016/j.envint.2019.105046
Palmer, S. (2021). Euronews.Green [WWW Document]. The End of a “Toxic Era”: The UN has Completely Banned Leaded Petrol. Available online at: https://www.euronews.com/green/2021/08/31/the-un-has-just-completely-phased-out-leaded-petrol (accessed May 10, 2022).
Paltseva, A., and Cheng, Z. (2019b). “Rapid screening of bioaccessible Pb in Urban soils using pXRF,” in Urbanization: Challenge and Opportunity for Soil Functions and Ecosystem Services. SUITMA 2017. Springer Geography, eds V. Vasenev, E. Dovletyarova, Z. Cheng, T. Prokof’eva, J. Morel, and N. Ananyeva (Berlin: Springer). doi: 10.1007/978-3-319-89602-1_29
Paltseva, A., Cheng, Z., Deeb, M., Groffman, P. M., Shaw, R. K., and Maddaloni, M. (2018). Accumulation of arsenic and lead in garden-grown vegetables: factors and mitigation strategies. Sci. Total Environ. 640–641, 273–283. doi: 10.1016/j.scitotenv.2018.05.296
Paltseva, A. A., and Cheng, Z. (2019a). Geospatial analysis and assessment of garden soil contamination in New York City. RUDN J. Agron. Anim. Indust. 14, 239–254. doi: 10.22363/2312-797x-2019-14-3-239-254
Paltseva, A. A., Cheng, Z., Egendorf, S. P., and Groffman, P. M. (2020). Remediation of an urban garden with elevated levels of soil contamination. Sci. Total Environ. 722:137965. doi: 10.1016/j.scitotenv.2020.137965
Paltseva, A. A., and Neaman, A. (2020). An emerging frontier: metal(loid) soil pollution threat under global climate change. Environ. Toxicol. Chem. 39, 1653–1654. doi: 10.1002/etc.4790
Pelfrêne, A., Douay, F., Richard, A., Roussel, H., and Girondelot, B. (2013). Assessment of potential health risk for inhabitants living near a former lead smelter. Part 2: site-specific human health risk assessment of Cd and Pb contamination in kitchen gardens. Environ. Monit. Assess. 185, 2999–3012. doi: 10.1007/s10661-012-2767-x
Pelfrêne, A., Sahmer, K., Waterlot, C., and Douay, F. (2019). From environmental data acquisition to assessment of gardeners’ exposure: feedback in an urban context highly contaminated with metals. Environ. Sci. Pollut. Res. 26, 20107–20120. doi: 10.1007/s11356-018-3468-y
Peryea, F. J. (1991). Bioremediation of Lead Arsenate-Contaminated Soils. Proj. A-168-WASH Rep. Pullman, WA: State of Washington Water Research Center.
Pfeiffer, E. M. (1988). Trace Elements and Heavy Metals in Soils and Plants of the Southeast Asian Metropolis Metro Manila and of Some Rice Cultivation Provinces in Luzon, Philippines. Hamburg: Verein zur Förderung d. Bodenkunde
Rai, P. K., Lee, S. S., Zhang, M., Tsang, Y. F., and Kim, K.-H. (2019). Heavy metals in food crops: health risks, fate, mechanisms, and management. Environ. Int. 125, 365–385. doi: 10.1016/j.envint.2019.01.067
Ratha, D. S., and Sahu, B. K. (1993). Source and distribution of metals in urban soil of Bombay, India, using multivariate statistical techniques. Environ. Geol. 22, 276–285. doi: 10.1007/BF00767413
Raymond, C. M., Diduck, A. P., Buijs, A., Boerchers, M., and Moquin, R. (2019). Exploring the co-benefits (and costs) of home gardening for biodiversity conservation. Local Environ. 24, 258–273. doi: 10.1080/13549839.2018.1561657
Romic, M., and Romic, D. (2003). Heavy metals distribution in agricultural topsoils in urban area. Environ. Geol. 43, 795–805. doi: 10.1007/s00254-002-0694-9
Romzaykina, O. N., Vasenev, V. I., Paltseva, A., Kuzyakov, Y. V., Neaman, A., and Dovletyarova, E. A. (2021). Assessing and mapping urban soils as geochemical barriers for contamination by heavy metal (loid) sin Moscow megapolis. J. Environ. Qual. 50, 22–37.
Ruby, M. V., Davis, A., Schoof, R., Eberle, S., and Sellstone, C. M. (1996). Estimation of lead and arsenic bioavailability using a physiologically based extraction test. Environ. Sci. Technol. 30, 422–430. doi: 10.1021/es950057
Roussel, H., Waterlot, C., Pelfrne, A., Pruvot, C., Mazzuca, M., and Douay, F. (2010). Cd, Pb and Zn oral bioaccessibility of urban soils contaminated in the past by atmospheric emissions from two lead and zinc smelters. Arch. Environ. Contam. Toxicol. 58, 945–954. doi: 10.1007/s00244-009-9425-5
Scheckel, K. G., Diamond, G. L., Burgess, M. F., Klotzbach, J. M., Maddaloni, M., Miller, B. W., et al. (2013). Amending soils with phosphate as means to mitigate soil lead hazard: a critical review of the state of the science. J. Toxicol. Environ. Health Part B 16, 337–380. doi: 10.1080/10937404.2013.825216
Scheneryer, J. (2017). Looking for Lead [WWW Document]. Reuters Inversigates Legacy of Lead. Available online at: https://www.reuters.com/investigates/graphics/lead-water/en/ (accessed June 20, 20).
Schreck, E., Bonnard, R., Laplanche, C., Leveque, T., Foucault, Y., and Dumat, C. (2012). DECA: a new model for assessing the foliar uptake of atmospheric lead by vegetation, using Lactuca sativa as an example. J. Environ. Manag. 112, 233–239. doi: 10.1016/j.jenvman.2012.07.006
Schroder, J. L., Basta, N. T., Casteel, S. W., Evans, T. J., Payton, M. E., and Si, J. (2004). Validation of the in vitro gastrointestinal (IVG) method to estimate relative bioavailable lead in contaminated soils. J. Environ. Qual. 33, 513–521. doi: 10.2134/jeq2004.5130
Shahid, M., Dumat, C., Khalid, S., Schreck, E., and Xiong, T. (2017). Foliar heavy metal uptake, toxicity and detoxification in plants: a comparison of foliar and root metal uptake. J. Hazard. Mater. 325, 36–58. doi: 10.1016/j.jhazmat.2016.11.063
Sheppard, S. C., and Evenden, W. G. (1992). Concentration enrichment of sparingly soluble contaminants (U, Th and Pb) by erosion and by soil adhesion to plants and skin. Environ. Geochem. Health. 14, 121–131. doi: 10.1007/BF01783487
Shi, R., Lv, J., Cai, Y., Liu, Y., Wang, Z., Feng, J., et al. (2010). Levels, spatial distribution and possible sources of heavy metals contamination of suburban soils in Tianjin, China. Bull. Environ. Contam. Toxicol. 85, 287–290. doi: 10.1007/s00128-010-0070-5
Simon, E., Vidic, A., Braun, M., Fábián, I., and Tóthmérész, B. (2013). Trace element concentrations in soils along urbanization gradients in the city of Wien, Austria. Environ. Sci. Pollut. Res. 20, 917–924. doi: 10.1007/s11356-012-1091-x
Sofo, A., and Sofo, A. (2020). Converting home spaces into food gardens at the time of Covid-19 quarantine: all the benefits of plants in this difficult and unprecedented period. Hum. Ecol. 48, 131–139.
Spliethoff, H. M., Mitchell, R. G., Shayler, H., Marquez-Bravo, L. G., Russell-Anelli, J., Ferenz, G., et al. (2016). Estimated lead (Pb) exposures for a population of urban community gardeners. Environ. Geochem. Health 38, 955–971. doi: 10.1007/s10653-016-9790-8
Stoiber, T., Evans, S., and Naidenko, O. V. (2020). Disposal of products and materials containing per- and polyfluoroalkyl substances (PFAS): a cyclical problem. Chemosphere 260:127659. doi: 10.1016/j.chemosphere.2020.127659
Tijhuis, L., Brattli, B., and Sæther, O. M. (2002). A geochemical survey of topsoil in the City of Oslo, Norway. Environ. Geochem. Health 24, 67–94. doi: 10.1023/A:1013979700212
USEPA (1998). Sources of Lead in Soil: a Literature Review Final Report 5–28. Washington, DC: USEPA.
USEPA (2012). Standard Operation Procedure for an in Vitro Bioaccessibility Assay for Lead in Soil (No. 9200.2–86). Washington, DC: USEPA.
USEPA (2013). Method 1340 in Vitro Bioaccessibility Assay for Lead in Soil. SW-846 Hazardous Waste Test Methods. Washington, DC: USEPA.
USEPA (2014). Technical Review Workgroup Recommendations Regarding Gardening and Reducing Exposure to Lead-Contaminated soils. Washington, DC: USEPA.
USEPA (2017). Method 1340: in Vitro Bioaccessibility Assay for Lead in Soil. SW-846 Update VI. Washington, DC: USEPA.
USEPA Office of Superfund Remediation and Technology Information (2013). Use of Amendments for In Situ Remediation at Superfund Sediment Sites. OSWER Directive 9200.2-128FS. Washington, DC: USEPA.
USEPA Office of Water (1995). Part 503 Implementation Guidance. EPA 833-R-95-001. Washington, DC: USEPA.
USEPA Oswer (2007). Estimation of Relative Bioavailability of Lead in Soil and Soil-Like Materials Using in Vivo and in Vitro Methods. (No. OSWER 9285.7-77). Washington, DC: USEPA.
Uzu, G., Schreck, E., Xiong, T., Macouin, M., Lévêque, T., Fayomi, B., et al. (2014). Urban market gardening in Africa: foliar uptake of metal(loid)s and their bioaccessibility in vegetables; implications in terms of health risks. Water Air Soil Pollut. 225:2185. doi: 10.1007/s11270-014-2185-5
Uzu, G., Sobanska, S., Sarret, G., Muñoz, M., and Dumat, C. (2010). Foliar lead uptake by lettuce exposed to atmospheric fallouts. Environ. Sci. Technol. 44, 1036–1042. doi: 10.1021/es902190u
Vasenev, V. I., Smagin, A. V., Ananyeva, N. D., Ivashchenko, K. V., Gavrilenko, E. G., Prokofeva, T. V., et al. (2017). “Urban soil’s functions: monitoring, assessment, and management,” in Adaptive Soil Management: From Theory to Practices, eds A. Rakshit, P. Abhilash, H. Singh, and S. Ghosh (Singapore: Springer Nature), 359–409. doi: 10.1007/978-981-10-3638-5
Viverette, L., Mielke, H. W., Brisco, M., Dixon, A., Schaefer, J., and Pierre, K. (1996). Environmental health in minority and other underserved populations: Benign methods for identifying lead hazards at day care centres of New Orleans. Environ. Geochem. Health 18, 41–45. doi: 10.1007/BF01757218
Walraven, N., Bakker, M., Van Os, B. J. H., Klaver, G. T., Middelburg, J. J., and Davies, G. R. (2015). Factors controlling the oral bioaccessibility of anthropogenic Pb in polluted soils. Sci. Total Environ. 506, 149–163.
Walsh, D., Glass, K., Morris, S., Zhang, H., McRae, I., Anderson, N., et al. (2018). Sediment exchange to mitigate pollutant exposure in urban soil. J. Environ. Manag. 214, 354–361. doi: 10.1016/j.jenvman.2018.03.013
Wei, B., and Yang, L. (2010). A review of heavy metal contaminations in urban soils, urban road dusts and agricultural soils from China. Microchem. J. 94, 99–107. doi: 10.1016/j.microc.2009.09.014
Weil, R. R. (2015). “Soil management for sustainable intensification: some guidelines,” in Sustainability of Agricultural Systems in Transition, Vol. 64, eds W. A. Payne, D. R. Keeney, and S. C. Rao (Madison, WI: ASA Special Publication), 143–154. doi: 10.2134/asaspecpub64.ch13
Werkenthin, M., Kluge, B., and Wessolek, G. (2014). Metals in European roadside soils and soil solution – a review. Environ. Pollut. 189, 98–110. doi: 10.1016/j.envpol.2014.02.025
Werth, C. (2019). Lead in the Land [WWW Document]. WNYC. Available online at: https://www.wnyc.org/story/lead-in-the-land/ (accessed May 5, 2020).
Whitzling, L., Wander, M., and Phillips, E. (2010). Testing and educating on urban soil lead: a case of Chicago community gardens. J. Agric. Food Syst. Commun. Dev. 1, 167–185. doi: 10.5304/jafscd.2010.012.015
Wilcke, W., Müller, S., Kanchanakool, N., and Zech, W. (1998). Urban soil contamination in Bangkok: heavy metal and aluminium partitioning in topsoils. Geoderma 86, 211–228. doi: 10.1016/S0016-7061(98)00045-7
Wragg, J., Cave, M., Basta, N., Brandon, E., Casteel, S., Denys, S., et al. (2011). An inter-laboratory trial of the unified BARGE bioaccessibility method for arsenic, cadmium and lead in soil. Sci. Total Environ. 409, 4016–4030. doi: 10.1016/j.scitotenv.2011.05.019
Zahran, S., Mielke, H. W., McElmurry, S. P., Filippelli, G. M., Laidlaw, M. A. S., and Taylor, M. P. (2013b). Determining the relative importance of soil sample locations to predict risk of child lead exposure. Environ. Int. 60, 7–14. doi: 10.1016/j.envint.2013.07.004
Zahran, S., Laidlaw, M. A. S., McElmurry, S. P., Filippelli, G. M., and Taylor, M. (2013a). Linking source and effect: resuspended soil lead, air lead, and children’s blood lead levels in Detroit, Michigan. Environ. Sci. Technol. 47, 2839–2845. doi: 10.1021/es303854c
Zahran, S., Mielke, H. W., Weiler, S., and Gonzales, C. R. (2011). Nonlinear associations between blood lead in children, age of child, and quantity of soil lead in metropolitan New Orleans. Sci. Total Environ. 409, 1211–1218. doi: 10.1016/j.scitotenv.2010.11.036
Zhang, C. (2006). Using multivariate analyses and GIS to identify pollutants and their spatial patterns in urban soils in Galway, Ireland. Environ. Pollut. 142, 501–511. doi: 10.1016/j.envpol.2005.10.028
Zhang, M. K., and Ke, Z. X. (2004). Heavy metals, phosphorus and some other elements in urban soils of Hangzhou City, China. Pedosphere 14, 177–185.
Zhang, Y. W., Han, J. H., and Tu, Q. (2019). Accumulation characteristics and evaluation of heavy metals in suburban farmland soils of Tianjin. J. Ecol. Rural Environ. 35, 1445–1452.
Zhou, H., Yang, W. T., Zhou, X., Liu, L., Gu, J. F., Wang, W. L., et al. (2016). Accumulation of heavy metals in vegetable species planted in contaminated soils and the health risk assessment. Int. J. Environ. Res. Public Health 13:289. doi: 10.3390/ijerph13030289
Keywords: urban garden, risk, best management practices, lead, soil contamination
Citation: Paltseva AA, Cheng Z, McBride M, Deeb M, Egendorf SP and Groffman PM (2022) Legacy Lead in Urban Garden Soils: Communicating Risk and Limiting Exposure. Front. Ecol. Evol. 10:873542. doi: 10.3389/fevo.2022.873542
Received: 10 February 2022; Accepted: 10 May 2022;
Published: 03 June 2022.
Edited by:
Stephanie Ann Yarwood, University of Maryland, College Park, United StatesReviewed by:
Gabriele Buttafuoco, National Research Council (CNR), ItalyStefan Siebert, North-West University, South Africa
Copyright © 2022 Paltseva, Cheng, McBride, Deeb, Egendorf and Groffman. This is an open-access article distributed under the terms of the Creative Commons Attribution License (CC BY). The use, distribution or reproduction in other forums is permitted, provided the original author(s) and the copyright owner(s) are credited and that the original publication in this journal is cited, in accordance with accepted academic practice. No use, distribution or reproduction is permitted which does not comply with these terms.
*Correspondence: Anna A. Paltseva, QW5uYS5QYWx0c2V2YUBMb3Vpc2lhbmEuZWR1