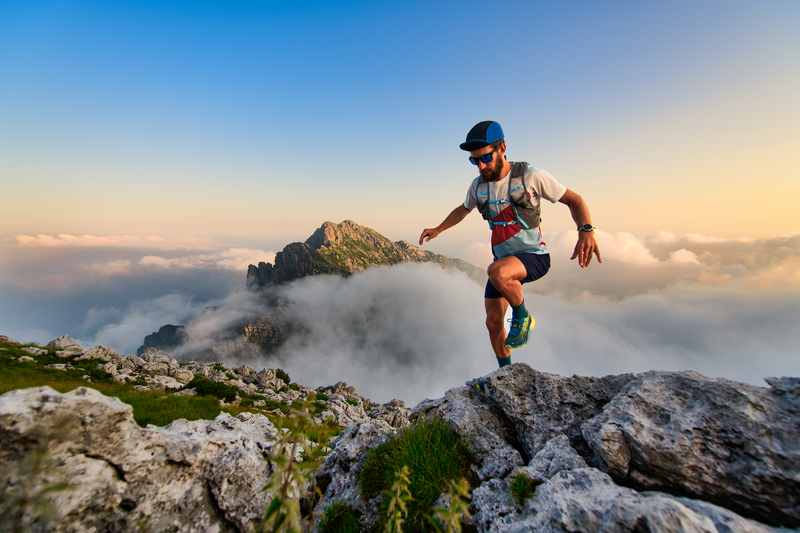
95% of researchers rate our articles as excellent or good
Learn more about the work of our research integrity team to safeguard the quality of each article we publish.
Find out more
ORIGINAL RESEARCH article
Front. Ecol. Evol. , 13 June 2022
Sec. Models in Ecology and Evolution
Volume 10 - 2022 | https://doi.org/10.3389/fevo.2022.866950
This article is part of the Research Topic Stability Across Spatial and Temporal Scales View all 6 articles
Ecological stability depends on interactions between different levels of biological organization. The insurance effects occur when increasing species diversity leads to more temporally invariable (i.e., more stable) community-level properties, due in part to asynchronous population-level fluctuations. While the study of insurance effects has received considerable attention, the role of dominant species that contribute with particular functional traits across different level of organizations is less understood. Using a field-based manipulative experiment, we investigated how species richness and different types of parameters at the population level, such as the invariability of dominants, population invariability, and population asynchrony, influence the community invariability. The experiment involved the repetitive removal of the canopy forming alga Mazzaella laminarioides (hereafter “Mazzaella”) during 32 months in two rocky intertidal sites of northern-central Chile. We predicted that the invariability of dominants enhances community invariability, that the effect of multispecies population-level parameters on community invariability are dependent on species richness, and that subdominant algae are unable to fully compensate the loss of canopies of the dominant species. Biomass of algae and mobile invertebrates was quantified over time. We observed independent effects of Mazzaella removal and community-wide asynchrony on community invariability. While canopy removal reduced community invariability, population asynchrony boosted community invariability regardless of the presence of canopies. In addition, filamentous and foliose algae were unable to compensate the loss of biomass triggered by the experimental removal of Mazzaella. Canopy removal led to a severe decrement in the biomass of macrograzers, while, at the same time, increased the biomass of mesograzers. Asynchrony stemmed from compensatory trophic responses of mesograzers to increased abundances of opportunistic algae. Thus, further work on consumer-resource interactions will improve our understanding of the links between population- and community-level aspects of stability.
Biodiversity loss represents a major disturbance impacting the functioning of ecosystems worldwide (Hooper et al., 2012). Stability encompasses multiple attributes or dimensions that allow us to understand the ability of ecosystems to remain relatively invariable over time in the face of environmental disturbances (Grimm and Wissel, 1997; Tilman, 1999; Lehman and Tilman, 2000; Donohue et al., 2016; Van Meerbeek et al., 2021). These attributes can be assessed for aggregate properties subsumed across populations in the community, such as community biomass, productivity, metabolism, and carbon storage among others (Yachi and Loreau, 1999; Tilman et al., 2006; Loreau and De Mazancourt, 2008; Kéfi et al., 2019). Since these properties support several ecosystems’ “good and services” provided to society, understanding the stability of ecosystems across ecological scales is relevant to predict negative consequences of biodiversity loss for human welfare (Cardinale et al., 2012; Loreau et al., 2021).
Among the multiple attributes of stability, invariability is a key aspect because it reflects the magnitude of a community’s response to unpredictable environmental changes (Pimm, 1984; Grimm and Wissel, 1997; Tilman, 1999; Donohue et al., 2016; Van Meerbeek et al., 2021). This metric can be estimated, for example, as the inverse of temporal variation in an aggregate community property like biomass or carbon storage (e.g., Tilman et al., 2006), and integrates the whole recovery process of a dynamic system exposed to disturbances (Arnoldi et al., 2016). Invariability (and other stability dimensions) has been suggested to be strongly related to the number of species in the community, partly depending on the amplitude and asynchrony of population-level fluctuations (Tilman, 1999; Stachowicz et al., 2007; de Mazancourt et al., 2013; Gross et al., 2014; Kéfi et al., 2019; Kigel et al., 2021). Particularly, species richness has an insurance effect that allows dampening the effects of disturbances on populations, maintaining relatively invariable community-level functions (Yachi and Loreau, 1999; Langenheder et al., 2012; Haughey et al., 2018). This buffering effect of richness involves the asynchrony in the temporal fluctuations of populations abundances as the result of competition and different population responses to environmental fluctuations (i.e., “population asynchrony”): the increase (decrease) in abundance of a certain populations can be compensated by the decrease (increase) in the abundance of others (Ives et al., 2000; González and Loreau, 2009; Leary and Petchey, 2009; de Mazancourt et al., 2013). In this line, species-rich communities usually include species with extreme functional trait values, such as large body sizes (Loreau and Hector, 2001; Polley et al., 2003). Consequently, these species can account for a large proportion of the magnitude of aggregated properties across the community, for example community biomass (Loreau, 2000). In addition, as species richness increases, the variance of an aggregated community property becomes smaller than the summed variance of individual populations (Doak et al., 1998; Cottingham et al., 2001; van Ruijven and Berendse, 2005). Thus, the responses of populations to environmental changes have profound consequences for the invariability of the community as a whole (Loreau, 2000).
Dominant species are those with the largest abundances in the community and usually have a large direct contribution to community biomass (Grime, 1998). Dominant species, such as canopy-forming plants and algae, often possess complex morphological structures and large body sizes, which can reduce the magnitude and variability of abiotic environmental factors underneath them. Canopy sheltering provides an ameliorated physical environment for the recruitment and persistence of a potentially diverse set of understory species (Bruno and Bertness, 2001; Stachowicz, 2001; Miller et al., 2018). Therefore, the population dynamics of dominant species can be expected to impact the whole community dynamics (Paine, 1974; Menge, 1976; Grime, 1998; Hillebrand et al., 2008). While the large contribution of dominant’s demographic stochasticity to community-level dynamics can reduce community invariability (de Mazancourt et al., 2013), empirical evidence indicates that larger dominant’s invariability leads to more invariable community biomass (e.g., Grman et al., 2010; Sasaki and Lauenroth, 2011). Therefore, dominant species are expected to contribute to community invariability by enhancing species richness, population invariability, population asynchrony, or the interaction among these attributes (Lamy et al., 2020).
Marine intertidal rocky ecosystems provide an opportunity to investigate the interdependent effects of dominant species and population-level dynamics on community invariability. For example, the experimental removal of dominant canopy forming algae usually results in an increase in light and substrate availability that allows opportunistic or rare species to spread over primary substrata (Benedetti-Cecchi and Cinelli, 1993; Stachowicz et al., 2008; Bertolini, 2018). Subordinate species, such as branched or foliose algae, can become “alternative dominants” that support the invariability of disturbed communities (Bertolini, 2018). However, the expansion of subordinates may be often a short-term and season-dependent response to the local extinction of canopy forming algae (Stachowicz et al., 2008). In these ecosystems, the constant movement of tides generates a sharp increase of environmental stress from low to high intertidal elevations—this stress is related to wide ranges of temperature, irradiance, and desiccation, among other factors (Harley and Helmuth, 2003; Harley et al., 2006; Harley et al., 2012; Zwerschke et al., 2013; Kordas et al., 2014; Kordas and Harley, 2016; Petraitis and Dudgeon, 2020). Population responses to disturbances in intertidal habitats (e.g., heat waves, swells) can be modulated by, for example, ecological interactions (e.g., competition for settlement space), the order of colonization in disturbed patches (Benedetti-Cecchi et al., 1999; Berlow, 1999), colonization-competition trade-offs (Sousa, 1979; Sousa, 1980; Underwood, 2000; Maggi et al., 2011), density-dependent recruitment, growth, and survival (Underwood et al., 1983; Navarrete et al., 2005), and strong consumer–resource interactions (e.g., Tejada-Martínez et al., 2016; Aguilera et al., 2020). Anthropogenic impacts also have profound effects on rocky ecosystems. For instance, overexploitation of large herbivores (i.e., macrograzers like fish, sea urchins, and large mollusks) has led to an increase in the abundance of smaller consumers (i.e., small snails, amphipods, and juvenile limpets collectively called as mesograzers), likely due to competitive release (Moreno, 2001; Aguilera and Navarrete, 2012). These unbalances can have consequences on community-wide invariability after the loss of habitat-forming canopies. Populations of mesograzers can exert a strong consumptive pressure on sessile species, particularly subordinate species like filamentous and foliose opportunistic seaweeds (Poore et al., 2012). The magnitude of such effects on seaweeds populations can be similar or even larger than those of larger consumers (Díaz and McQuaid, 2011). Therefore, consumer–resource interactions can mediate the ability of subordinate species to compensate the loss of larger canopies. Indeed, subordinate species has been shown to be unable to achieve the level of functionality of dominants (e.g., gross primary productivity or biomass; Crowe et al., 2013). Therefore, subordinate species might not compensate the community attribute provided by the function of the otherwise dominant algae species (Crowe et al., 2013; Filbee-dexter and Wernberg, 2018). Across marine and terrestrial ecosystems, most studies on stability target one level of organization, usually the community (Kéfi et al., 2019). Thus, understanding the interplay of invariability across scales of ecological organization can enhance our ability to predict the functional consequences of biodiversity loss (Kéfi et al., 2019).
Here, we use a field-based manipulative study to analyze invariability at two scales of biological organization: populations and communities. We assess the associations among the invariability of dominants, species richness, multispecies population invariability and asynchrony, and community invariability. We analyzed the dynamics of a temperate rocky shore community disturbed by the experimental removal of canopy-forming alga that dominates the mid intertidal shore. We removed the corticated red alga Mazzaella in a species-rich community of algae and mobile invertebrates (mostly grazers) of northern-central Chile, where direct harvesting of algae constitutes a common press disturbance. Canopy-forming algae allow the persistence of populations of environmentally sensitive species such as red and brown understory algae—which are negatively affected by excessive solar radiation (Santelices et al., 1981; Jara and Moreno, 1984; Steneck and Dethier, 1994)—and grazer gastropods that find shelter under the fronds (Aguilera et al., 2013). Therefore, we analyzed population- and community-level dynamics of the biomass of both algae and grazers. We used a model selection approach to test the following hypotheses:
(H1) Higher invariability of dominants enhances community invariability,
(H2) greater population invariability and population asynchrony enhance community invariability, but these effects depend on mean species richness, and
(H3) subordinate species, as alternative dominants in a disturbed community, are unable to compensate the “stabilizing” role of the dominant canopy forming species (see Table 1 for literature support).
Table 1. Empirical support of hypotheses proposed to explain invariability in our experimental community.
The experimental field study was carried out in two sites separated by ca. 22 km on the wave-exposed rocky shores of northern-central Chile (“Limarí” and “Punta de Talca”, ∼30°43′ S and 30°55′ S, respectively). In both sites, we assessed the effect of the experimental extinction of the dominant canopy-forming alga Mazzaella on community invariability. This red corticated alga inhabits the mid and low intertidal rocky shore between Coquimbo and Punta Arenas (Hoffmann and Santelices, 1997; Montecinos et al., 2012). Mazzaella can be highly dominant, with canopies covering up to ∼65% of secondary space (Santelices, 1990; Hoffmann and Santelices, 1997; Valdivia et al., 2021). The thallus can reach near to 30 cm in maximum length and the thick fronds can ameliorate environmental conditions beneath them (Hoffmann and Santelices, 1997). Particularly in our study sites, common sizes range from 5 to 15 cm in length (Supplementary Figure 1).
Direct harvesting of Mazzella constitutes a strong anthropogenic disturbance that drives abundance change in intertidal communities of the southeast Pacific coast (Buschmann et al., 2001). This activity occurs for the extraction of carrageenans used in food and pharmaceutical industry (Buschmann et al., 2001). Landings data from 2002 to 2017 shows that near to 3 tons of dry alga are extracted annually in Chile (Lopez, 2019).
Underneath Mazzaella canopies, a diverse assemblage of algae is observed, including the red algae Nothogenia fastigiata, Corallina officinalis var. chilensis, Gelidium sp. and Pyropia sp., and the green Chaetomorpha firma and Ulva spp. Also, the canopy proves habitat for macro and mesograzers such as the chitons Chiton granosus and C. barnesii, keyhole limpets (Fissurella spp.), the gastropod Siphonaria lessoni, the littorinid Austrolittorina araucana, and at least six species of the genus Scurria (Moreno and Jaramillo, 1983; Jara and Moreno, 1984; Moreno et al., 1984; Rivadeneira et al., 2002).
The experiment was established and monitored from January 2015 to August 2017, covering a sampling period of 32 months and at least three full seasonal cycles. The observations were conducted on 20 experimental plots equally distributed across both sites. Both sites were open access areas, where local fishermen and visitors are allowed to harvest marine resources directly (Buschmann et al., 2001). Also, both sites are exposed to similar environmental and oceanographic conditions (Aguilera et al., 2013; Valdivia et al., 2013, 2015).
In each site, we deployed at the mid intertidal zone ten 30 cm × 30 cm experimental plots, which were permanently marked with stainless-steel bolts. The size of the plots was chosen to minimize the impact on the local intertidal community. Experimental plots were placed within areas of high secondary cover (ca. 20–65% percent cover) of the locally dominant alga Mazzaella, and on flat and horizontal rocky surfaces, avoiding crevices and tide pools to reduce variability associated to substrate heterogeneity. Half of the experimental plots were haphazardly selected for the experimental treatment in which Mazzaella was completely removed with scrappers and chisels, leaving all the understory species intact (i.e., disturbed plots). The remaining half of the plots were kept unmanipulated (i.e., controls plots). Therefore, considering both sites together, our experimental design included a total of 10 control plots and 10 dominant species-removed plots. All settlers of Mazzaella were removed every three months in the disturbed plots. Three months between manipulations has been shown earlier to be an appropriate timespan to functionally exclude dominant species from intertidal communities in these sites and elsewhere (Bulleri et al., 2012; Aguilera et al., 2013; Valdivia et al., 2015).
All plots were sampled immediately before the removal of dominant canopies of Mazzaella, then two months after and then every three months until the end of the experiment in August of 2017. During each monitoring we estimated species abundance using a 30 cm × 30 cm frame divided in 25 fields with a monofilament line, following protocols described elsewhere (e.g., Broitman et al., 2011; Valdivia et al., 2020). All algae and invertebrates (c.a. >5 mm) occurring on each plot were identified in situ and the organisms were classified at the species level whenever possible. Abundance of algal species was estimated as substrate percentage cover (1% resolution), while grazers were registered as density (number of individuals per m2). All manipulations and observations were conducted during diurnal low-tide hours (ca. 1.5 m tidal range).
Abundance data of algae and grazers (percent cover and density, respectively) were transformed to biomass (dry weight g m–2). This allowed us to analyze together mobile and sessile species. For algae, biomass was estimated through calibration curves generated from destructive samples obtained in the field (10–20 replicates for each of the 19 species identified in experimental plots). For algae whose abundances in the field were too low to generate a calibration curve, we used published linear and nonlinear models of percentage cover-dry weight for morphologically similar taxa (Ko et al., 2008). In addition, the biomass of grazer species was estimated as the product between each species’ density (ind. m–2) and the average individual dry weight (g) obtained from Camus et al. (2013) and in situ samples (5–7 replicates for each of the main group of grazers: Littorinid snails, Scurria spp., Chiton spp., and Fissurella spp.). For biomass estimation, we excluded crustose algae due to logistic constraints, sessile invertebrates because their cover was always less than 1%, and predators because their frequency of occurrence and densities were very low (e.g., starfish and crabs). Community biomass was calculated for each plot as the sum of all individual species biomasses.
Community invariability was estimated for each plot as the inverse coefficient of variation (μ/σ) in community biomass over the 32 months of monitoring. This included together the biomass of the dominant canopy, understory algae, and grazers. This measure of invariability standardizes the temporal mean of community biomass to its temporal standard deviation; therefore, higher values indicate that community biomass is more constant over time (Tilman, 1999).
Population invariability was estimated following Thibaut and Connolly (2013) as the inverse of the weighted mean coefficient of variation in the biomass of all populations (species) within a sampling plot:
where μi and σi denote the temporal mean and standard deviation of biomass of each population i, N is the number of populations, and μc is the temporal mean of community biomass. Larger values of population invariability indicate that, on average, population abundances are more constant over time.
Following Sasaki et al. (2019), population asynchrony (φP) was estimated as:
where φP is the variance of community biomass () divided by the squared sum of the standard deviations of all N individual populations (Sxi). Values around 0 indicate that biomass fluctuations among populations are perfectly synchronized, while values close to 1 will indicate that are perfectly asynchronized.
Invariability of dominants was estimated for control plots as the inverse coefficient of variation (μ/σ) of Mazzaella’s biomass over time. For disturbed plots we estimated the invariability of the “alternative dominants” as the invariability of the summed biomass of the foliose algae Ulva spp. and Pyropia sp., which were the most abundant algae when Mazzaella was absent. The separate estimation of dominant’s invariability in control and disturbed communities was carried out to assess, in separate statistical models, the contribution of dominant and alternative dominants to the invariability of the whole community, as well in the association among drivers that support invariability. Finally, mean species richness was estimated as the temporal mean of the number of taxonomic identities for each plot.
Additionally, local environmental variability during the experiment was assessed by analyzing sea surface temperature (publicly available at http://www.ceazamet.cl/) using OnSet HOBO® water temperature dataloggers, which were deployed in both study sites, Limarí and Punta de Talca, at ca. 1m below low tide sea level following housed into concrete blocked anchored to the rocks with chain links. Deployment methods are extensively described elsewhere (Valdivia et al., 2013, 2015). Daily minimum, maximum, and average temperature data were derived from the temperature time series recorded during the experiment. Seawater temperature is a useful proxy for local community-wide environmental conditions as it correlates with other environmental variables such as nutrient and Chlorophyll-a concentration (Nielsen and Navarrete, 2004; Witman et al., 2008), and also through its influence on metabolic rates for ectotherms, animal and plant phenology, and population growth rates of coastal marine organisms (Strathmann et al., 2002; Baldanzi et al., 2018; Suárez et al., 2020).
Before testing our working hypotheses, we verified whether it was possible to pool the data from both sites. This was done to increase replication and thus statistical power for hypothesis testing. We used one-way analysis of variance (ANOVA) to compare mean percentage cover of Mazzaella, mean species richness, population asynchrony, and community invariability between both sites. For cover of Mazzaella, we analyzed only the control plots at each site (10 plots in total). For the other variables, we used the entire dataset (20 plots in total). Since we did not detect statistical differences between both sites in any variable (see Supplementary Table 1), we decided to analyze and report the result of both sites together.
We used information theory to assess the strength of empirical evidence supporting each of our hypotheses (Burnham and Anderson, 2002). We developed a set of general linear models (LM) linking community invariability to different combinations of predictor variables, in accordance with each hypothesis (see models in Table 2). These models were constructed on the basis of empirical and theoretical evidence supporting the premises described in the introduction section (see hypotheses H1, H2, and H3 in the introduction and Table 1). Model parameters were estimated through maximum likelihood. All numerical variables were centered and standardized before fitting.
Models were ranked according to bias-corrected Akaike Criterion of Information (AICc; Burnham et al., 2011). Model selection was based on Δ AICc, expressed a AICci − AICcmin, the Akaike weight, which represents the probability of each model as , where gi is model i and li = L(gi|data = e−0.5Δi, and the evidence ratio of the top model, calculated as wtop/wj (Burnham et al., 2011). Among the ranked models, we selected those with a Δ AICc < 6, as our “top model set” (Richards, 2005, 2008; Harrison et al., 2018).
Since AICc tends to favor too complex models (i.e., models including many variables; Burnham and Anderson, 2002), we used the “nesting rule” to reduce the number of models used for inference from the top model set (Harrison et al., 2018). The nesting rule consists of selecting the model with the strongest empirical support (lowest AICc) and that is nested within more complex models with delta AICc < 6 (Richards, 2005, 2008; Harrison et al., 2018).
In addition, we used LMs to assess the effect of seasons and treatments on community biomass and that of each functional group. Model diagnostics were checked by visual inspection of quantile-quantile plots and fitted vs. adjusted residual plots. All the graphs and analyses were developed and fitted in R programming environment 4.0.2, using the sjPlot, ggplot2 packages (R Core Team, 2020). Community stability and synchrony were computed with “codyn” R package (Hallett et al., 2016). Linear models were computed by the “stats” package (R Core Team, 2020) and the “MuMIn” package was used to estimate AICc and Akaike weight scores (Barton, 2020).
Within the top model set (delta AICc < 6; Table 3), we selected model 12 because it had the strongest empirical support and it was a more parsimonious version of the others (i.e., nesting rule). This model included the separate effects of Mazzaella removal and population asynchrony on community invariability. The empirical support (evidence ratio) for model 12 was 4.6 times that of model 13, and 17.6 times that of model 15 (both in the top model set). Model 12 accounted for 75 % of community invariability (R2 = 0.75, Table 4). The removal of Mazzaella decreased community invariability (Figure 1; difference between means = 1.16 [SE = 0.24], t-value = −4.9). Independent of the experimental removal, community invariability increased lineally with increasing population asynchrony (Figure 1; slope = 0.67 [0.12], t-value = 5.6).
Table 3. AICc table results of top model set selected in the evaluation of the role of invariability of dominants, mean species richness, population invariability and population asynchrony on overall community invariability in an intertidal community exposed to a press disturbance.
Table 4. Summary results of the best performing model (Delta AICc < 6.0), including population asynchrony (ϕP) and dominant species removal (RD).
Figure 1. Relationship between population asynchrony and community invariability in control (circles) and disturbed (triangles) experimental intertidal communities from northern-central Chile. Blue and red lines, and gray areas represent predicted values and standard errors, respectively, of community invariability (see model parameters in Table 4). Based on 20 experimental plots (10 control and 10 disturbed).
In control plots, mean biomass of dominant canopies ranged between 30 and 300 g m–2 during the experiment (see purple symbols in Figure 2A). Despite the large range of variation, canopies dominated in biomass most of the time. An initial decrease in canopy biomass in controls was followed by a peak increase in biomass of foliose algae; however, foliose algae biomass remained near to zero afterwards (cyan symbols in Figure 2A). The biomass of filamentous algae ranged from 0 to ∼70 g m–2 and remained as subdominants during all the experiment, except when canopies decreased almost to zero in the early autumn of 2016 (blue symbols in Figure 2A). Branched algae ranged from 0 to ∼100 g m–2 and fluctuated almost synchronically with dominant canopies during 2017 (yellow symbols in Figure 2A; see species composition of each group in Supplementary Table 2).
Figure 2. Temporal variation in mean biomass (±SE) of algae (A,B) and grazers (C,D) in control (left panels) and disturbed (right panels) conditions. Algae were categorized according to morphological functional groups (canopy of Mazzaella, branched, filamentous, and foliose) and grazers according to average adult body size (Chiton granosus, C. barnesi, Fissurella crassa, F. limbata and Fissurella sp. as macrograzers; Littorina araucana, L. peruviana, Scurria araucana, S. ceciliana, S. plana, S. variabilis, S. viridula, S. zebrina and Siphonaria lessoni as mesograzers). Each year label is positioned in May of each year (early autumn in southern hemisphere). Based on 20 experimental plots (10 control and 10 disturbed).
When canopies of Mazzaella were experimentally removed, the community became dominated by foliose, branched (corticated), and filamentous algae. We observed a large initial increase in foliose algae, which fluctuated from 20 to 250 g m–2 in biomass and were the group with the highest contribution to community biomass until mid 2016 for this treatment (Figure 2B). Filamentous and branched algae were subdominant in these plots and fluctuated from 10 to 70 g m–2 in biomass, showing a low contribution to overall community biomass. After a decrease in mid 2016, filamentous and branched algae remained in the same range of biomass and did not compensate the decrease in foliose algae (Figure 2B).
In controls, the biomass of macrograzers ranged from 5 to 55 g m–2, peaking in early autumn of 2015 and early winter of 2016 (light-blue symbols in Figure 2C). Mesograzers, on the other hand, were less variable and ranged from 15 to 25 g m–2 (pink symbols in Figure 2C). When Mazzaella was experimentally removed, macrograzers almost disappeared (0–5 g m–2). An opposite response was observed for mesograzers, such as Siphonaria lessoni and Scurria spp., which increased in density and biomass, ranging from 10 to 40 g m–2 (Figure 2D) and sometimes even overshooting the controls (from mid 2015 to late 2016).
Mean sea surface temperature ranged from 12.5 to 16.5° C during the experiment (Figure 3). The lowest temperature was reached during austral Autumn months, while the maximum temperature was reached in Summer months, particularly in January 2016. Community biomass showed no differences between treatments in the cold seasons (Figure 4). Between-treatment differences were evident in summer, where control plots reached almost twice the biomass of disturbed plots (model R2 = 0.203; Figure 4 and Supplementary Table 3). The difference in community biomass between treatments observed in Summer was highly influenced by the increase in canopy biomass in controls (R2 = 0.490; Supplementary Figure 2 and Supplementary Table 4). Seasonal effects were also observed for filamentous algae, in which biomass increased in Winter regardless of the experimental treatment (R2 = 0.104; Supplementary Figure 2 and Supplementary Table 4). On the other hand, foliose algae showed the highest biomass in Autumn. Also, differences between treatments were observed in the biomass of foliose algae, with disturbed plots reaching almost two to three times the biomass of foliose algae in controls (R2 = 0.206; Supplementary Figure 2 and Supplementary Table 4). The biomass of macrograzers was not affected by seasons but only by the experimental treatment (R2 = 0.155), while the biomass of mesograzers tended to increase in Spring in the removal treatment (Supplementary Figure 2 and Supplementary Table 5).
Figure 3. Temporal variation in mean (±SE) sea surface temperature in the experimental sites used in our study. For temperature, we considered monthly averaged temperature of both sites, Limarí and Punta de Talca. Each year label is positioned in January of each year (early summer in southern hemisphere).
Figure 4. Seasonal variation in overall community biomass (±SE) on control (red circles, n = 10) and disturbed (blue circles, n = 10) plots. Each season account with three replications (three seasonal cycles).
After 32 months of monitoring covering almost three full seasonal cycles, our empirical study shows independent effects of dominant species removal (Mazzaella) and population asynchrony on community invariability. The greater the asynchrony, the greater the invariability of the community. At the same time, invariability was greater when canopies were present, probably due to an increase in community biomass in summer months. Filamentous and foliose subdominant algae were unable to compensate the loss of biomass following by the experimental removal of Mazzaella canopy. Causes and consequences of the separate effects of dominant species-removal and population-level asynchrony on community-wide invariability are discussed.
Following the calculation of invariability (mean divided by the standard deviation; Tilman, 1999), the removal of canopies reduced community invariability respect to controls by decreasing the mean and/or increasing the standard deviation of community biomass (Bulleri et al., 2012). The removal of canopies led to a community dominated by low-biomass opportunistic foliose algae and mesograzers (Supplementary Table 2). Indeed, the removal of canopies decreased almost to zero the biomass of macrograzers, which are active consumers of algae such as the encrusting Hildenbrandia sp., calcareous branched Corallinaceae, and foliose Ulva spp. (Camus et al., 2013). Thus, the absence of these macrograzers in the removal treatment could explain the increase of foliose, branched, and filamentous species.
The species that became dominant under the canopy-removal condition were algae characterized by high colonization and mortality rates, low resistance to grazers, and physiological adaptations to environmental stress (Littler and Littler, 1984; Santelices, 1990; Duffy and Hay, 1994). In agreement with their opportunistic lifestyle, these algae also display large surface areas (i.e., foliose algae), which allows high productivity and growth rates (Littler and Littler, 1984; Steneck and Dethier, 1994). Consequently, the demographic stochasticity of these species may increase biomass variability at the community level (i.e., reduce community invariability; Stachowicz et al., 2008), as predicted by theoretical models of invariability (Loreau and de Mazancourt, 2013). Particularly, species such as Ulva spp. and Pyropia orbicularis can show high growth rates under broad environmental fluctuations and abiotic stress, quickly colonizing available niches as opportunistic species (Rosenberg and Ramus, 1982; Pérez-Mayorga et al., 2011). Also, elevated temperatures can trigger reproduction and settlement of some Ulva spp. (Gao et al., 2017). These foliose algae usually characterize early successional stages of intertidal communities, before the establishment of larger competitors like canopies or invertebrates (Foster et al., 2003; Edwards and Connell, 2012). Probably the absence of Mazzaella allowed constant available space for recruitment of these foliose algae, but seasonally variable disturbances—such as Winter extreme waves or enhanced mesograzer activity in Spring—might have limited their biomass. In this way, the demographic dynamics of the newly dominant species may have increased the temporal variance in total community biomass, decreasing thus community invariability.
The positive asynchrony-invariability relationship has been previously predicted by theory and has been found in a wide range of ecosystem across marine and terrestrial habitats (de Mazancourt et al., 2013; Valencia et al., 2020; Kigel et al., 2021). The positive effect of population asynchrony on community invariability has been associated to competitive interactions, differential species’ environmental tolerances, and trophic dynamics (Doak et al., 1998; Klug et al., 2000; Lehman and Tilman, 2000; Loreau and de Mazancourt, 2013). For example, the feeding activity of grazers can limit the expansion of subdominant algae after experimental disturbances such as canopy removal, increased nutrient load, and ocean acidification (Connell and Russell, 2010; Ghedini et al., 2015). Similarly, we observed an increase in the abundance of mesograzers in the canopy-removal plots (Figure 2C), as a result of milder interspecific competition with macrograzers and the increased habitat complexity provided by branched algae (e.g., Nothogenia fastigiata, Gelidium sp., Ahnfeltiopsis sp. and Trematocarpus dichotomus; O’Brien and Scheibling, 2018; Bertolini, 2018). Mesograzers, in turn, could have “dampened” the overshoot of foliose algae, which could explain the pattern of temporal variation observed for these algae (Figure 2B). Indeed, intertidal mesograzers can exert an important control on the abundance and diversity of algae and even sessile invertebrates (Aguilera et al., 2015; Tejada-Martínez et al., 2016). Specifically, mesograzers like Siphonaria lessoni and Scurria spp. are generalist consumers that consume algae such as Ulva spp., Ulvella sp., Hildenbrandia sp., and Codium dimorphum (Camus et al., 2013). In general, the feeding activity of (macro and meso) grazers on propagules and macroscopic stages can alter the dynamics of algal populations (Iken, 2012). These effects can be particularly strong during early successional stages, and thus, on the composition of algal communities (Arrontes, 1999; Aguilera and Navarrete, 2007; Aguilera, 2011; Iken, 2012). Additionally, moderate levels of herbivory by mesograzers could be beneficial during succession of intertidal communities dominated by Mazzaella (Aguilera and Navarrete, 2007). Therefore, a proportional increase in grazing, a trophic compensation, could well have counteracted the expansion of opportunistic algae as a response to canopy removal.
Theoretical, laboratory and field-based studies demonstrate that species richness generates invariability (Romanuk et al., 2006; Tilman et al., 2006; Loreau and de Mazancourt, 2013; Hallett et al., 2014). In our study, however, the empirical evidence supporting the independent effects of canopy removal and asynchrony on community invariability were ca. 18 times stronger than that supporting the effects of species richness. In the same line, a recent study of plant communities at the global scale has indicated that synchrony could be more relevant than species richness per se (Valencia et al., 2020). Asynchrony is indeed one of the three main mechanisms underpinning the stabilizing effects of species richness (de Mazancourt et al., 2013). Our results suggest therefore that the other two mechanisms driving the diversity-stability relationship—overyielding and reduced observation error—may have been less relevant than asynchrony in the analyzed community.
In addition to dominants, rare species can have strong effects on community invariability. For example, recent theoretical work suggests that perturbations that target rare species can have disproportionate effects on community dynamics, due to indirect effects cascading through the assemblage (Säterberg et al., 2019). In addition, the abundances of rare species are usually highly variable over time, which can have a strong influence on invariability averaged across all populations and even lead to negative diversity-stability relationships at the community level (Arnoldi et al., 2019). In our study, crustose algae and low-biomass filamentous and branched algae represented rare species. Future research could focus on the potential effect of these species on population and community stability. Our results support the idea that multiple factors, in addition to species richness, should be investigated to understand community invariability and other aspects of stability (Ives and Carpenter, 2007).
Despite canopy-removal plots exhibited lower invariability than control plots, this community-level property was independent from the invariability generated by the canopy of Mazzaella. Mazzaella exerts indirect effects on community invariability via trophic links and competitive interactions with the understory species. Alternatively, it can be the case the contribution of Mazzaella to mean community biomass was much larger than the “dampening” effect of this species on biomass standard deviation. Dominant species have been shown to influence both, the mean and standard deviation of total community biomass (Grman et al., 2010; Valdivia et al., 2013).
Our experimental study allowed us to empirically demonstrate that population-level dynamics, such as the experimental removal of a dominant species and patterns of population asynchrony, have strong effects on community invariability. The results suggest that both processes independently and simultaneously underpin the overall community response to a major disturbance. Consumer-resource interactions seemingly led population asynchrony. This may represent a compensatory “internal” response of the community to the disturbance, providing a key link between population and community levels of biological organization in this ecosystem. Further experimental work of compensatory consumer-resource interactions will allow us to improve our mechanistic understanding of the role of species asynchrony in maintaining community stability under the global biodiversity loss scenario that is unraveling.
The datasets presented in this study can be found in online repositories. The names of the repository/repositories and accession number(s) can be found below: https://www.frontiersin.org/articles/10.3389/fmars.2020.569650/full#supplementary-material.
BB and NV conceived to the study and contributed with field work and analytical material. EF-R and AC analyzed the data. EF-R and NV wrote the manuscript. AC, BB, and AP-M contributed significantly to the writing of the manuscript. All authors contributed to the article and approved the submitted version.
This study was financially supported by the FONDECYT research grants #1190529 (NV), #1181300 (BB) and by the ANID research grant for doctoral studies #21181568 (EF-R). While writing NV was financially supported by FONDAP grant #1515003 “IDEAL”. BB also acknowledges support from Instituto Milenio de Socio-Ecologiìa Costera (SECOS, ICN2019_015) and Núcleo Milenio UPWELL (NCN19_153).
The authors declare that the research was conducted in the absence of any commercial or financial relationships that could be construed as a potential conflict of interest.
All claims expressed in this article are solely those of the authors and do not necessarily represent those of their affiliated organizations, or those of the publisher, the editors and the reviewers. Any product that may be evaluated in this article, or claim that may be made by its manufacturer, is not guaranteed or endorsed by the publisher.
We acknowledge members from Changolab, particularly Manuel Nuñez Cruzat for his continued commitment during the experimental monitoring.
The Supplementary Material for this article can be found online at: https://www.frontiersin.org/articles/10.3389/fevo.2022.866950/full#supplementary-material
Aguilera, M. A. (2011). The functional roles of herbivores in the rocky intertidal systems in Chile: a review of food preferences and consumptive effects. Rev. Chil. de Hist. Nat. 84, 241–261. doi: 10.4067/S0716-078X2011000200009
Aguilera, M. A., and Navarrete, S. A. (2007). Effects of Chiton granosus (Frembly, 1827) and other molluscan grazers on algal succession in wave exposed mid-intertidal rocky shores of central Chile. J. Exp. Mar. Biol. Ecol. 349, 84–98. doi: 10.1016/j.jembe.2007.05.002
Aguilera, M. A., and Navarrete, S. A. (2012). Functional identity and functional structure change through succession in a rocky intertidal marine herbivore assemblage. Ecology 93, 75–89. doi: 10.1890/11-0434.1
Aguilera, M. A., Navarrete, S. A., and Broitman, B. R. (2013). Differential effects of grazer species on periphyton of a temperate rocky shore. Mar. Ecol. Prog. Ser. 484, 63–78. doi: 10.3354/meps10297
Aguilera, M. A., Valdivia, N., and Broitman, B. R. (2015). Facilitative effect of a generalist herbivore on the recovery of a perennial alga: consequences for persistence at the edge of their geographic range. PLoS One 10, 1–23. doi: 10.1371/journal.pone.0146069
Aguilera, M. A., Valdivia, N., Broitman, B. R., Jenkins, S. R., and Navarrete, S. A. (2020). Novel co-occurrence of functionally redundant consumers induced by range expansion alters community structure. Ecology. 0, 1–17. doi: 10.1002/ecy.3150
Arnoldi, J. F., Loreau, M., and Haegeman, B. (2016). Resilience, reactivity and variability: a mathematical comparison of ecological stability measures. J. Theor. Biol. 389, 47–59. doi: 10.1016/j.jtbi.2015.10.012
Arnoldi, J. F., Loreau, M., and Haegeman, B. (2019). The inherent multidimensionality of temporal variability: how common and rare species shape stability patterns. Ecol. Lett. 22, 1557–1567. doi: 10.1111/ele.13345
Arrontes, J. (1999). On the evolution of interactions between marine mesoherbivores and Algae. Bot. Mar. 42, 137–155.
Baldanzi, S., Storch, D., Navarrete, S. A., Graeve, M., and Fernández, M. (2018). Latitudinal variation in maternal investment traits of the kelp crab Taliepus dentatus along the coast of Chile. Mar. Biol. 165, 1–12. doi: 10.1007/s00227-018-3294-2
Benedetti-Cecchi, L., and Cinelli, F. (1993). Early patterns of algal succession in a midlittoral community of the Mediterranean Sea: a multifactorial experiment. J. Exp. Mar. Biol. Ecol. 169, 15–31. doi: 10.1016/0022-0981(93)90040-U
Benedetti-Cecchi, L., Menconi, M., and Cinelli, F. (1999). Pre-emption of the substratum and the maintenance of spatial pattern on a rocky shore in the northwest Mediterranean. Mar. Ecol. Prog. Ser. 181, 13–23.
Bennett, S., and Wernberg, T. (2014). Canopy facilitates seaweed recruitment on subtidal temperate reefs. J. Ecol. 102, 1462–1470.
Berlow, E. L. (1999). Strong effects of weak interactions in ecological communities. Nature 398, 330–334.
Bertolini, C. (2018). Can secondary species maintain a primary role? Consistent inter-regional effects of understory algae on diversity. Mar. Biodivers. 49, 841–849. doi: 10.1007/s12526-018-0862-0
Broitman, B. R., Véliz, F., Manzur, T., Wieters, E. A., Finke, G. R., Fornes, P. A., et al. (2011). Geographic variation in diversity of wave exposed rocky intertidal communities along central Chile. Rev. Chil. de Hist. Nat. 84, 143–154. doi: 10.4067/S0716-078X2011000100011
Bruno, J. F., and Bertness, M. D. (2001). “Habitat modification and facilitation in benthic marine communities,” in Marine community ecology, eds M. D. Bertness, M. E. Hay, and S. D. Gaines (Sunderland: Sinauer), 201–218.
Bulleri, F., Benedetti-Cecchi, L., Cusson, M., Maggi, E., Arenas, F., Aspden, R., et al. (2012). Temporal stability of European rocky shore assemblages: variation across a latitudinal gradient and the role of habitat-formers. Oikos 121, 1801–1809. doi: 10.1111/j.1600-0706.2011.19967.x
Burnham, K. P., and Anderson, D. R. (2002). Model Selection and Multimodel Inference, 2nd Edn. New York, NY: Springer-Verlag.
Burnham, K. P., Anderson, D. R., and Huyvaert, K. P. (2011). AIC model selection and multimodel inference in behavioral ecology: some background, observations, and comparisons. Behav. Ecol. Sociobiol. 65, 23–35. doi: 10.1007/s00265-010-1029-6
Buschmann, A. H., Correa, J. A., Westermeier, R., Hernández-González, M., del, C., and Norambuena, R. (2001). Red algal farming in Chile: a review. Aquac. 194, 203–220. doi: 10.1016/S0044-8486(00)00518-4
Camus, P. A., Arancibia, P. A., and Ávila-Thieme, I. (2013). A trophic characterization of intertidal consumers on Chilean rocky shores. Rev. Biol. Mar. Oceanogr. 48, 431–450. doi: 10.4067/s0718-19572013000300003
Cardinale, B. J., Duffy, J. E., Gonzalez, A., Hooper, D. U., Perrings, C., Venail, P., et al. (2012). Biodiversity loss and its impact on humanity. Nature. 486, 59–67. doi: 10.1038/nature11148
Cottingham, K. L., Brown, B. L., and Lennon, J. T. (2001). Biodiversity may regulate the temporal variability of ecological systems. Ecol. Lett. 4, 72–85. doi: 10.1046/j.1461-0248.2001.00189.x
Chesson, P. (2018). Updates on mechanisms of maintenance of species diversity. J. Ecol. 106, 1773–1794. doi: 10.1111/1365-2745.13035
Connell, S. D., and Russell, B. D. (2010). The direct effects of increasing CO2 and temperature on non-calcifying organisms: increasing the potential for phase shifts in kelp forests. Proc. Royal Soc. B. 277, 1409–1415. doi: 10.1098/rspb.2009.2069
Crowe, T. P., Cusson, M., Bulleri, F., Davoult, D., Arenas, F., Aspden, R., et al. (2013). Large-Scale variation in combined impacts of canopy loss and disturbance on community structure and ecosystem functioning. PLoS One 8:6. doi: 10.1371/journal.pone.0066238
de Mazancourt, C., Isbell, F., Larocque, A., Berendse, F., De Luca, E., Grace, J. B., et al. (2013). Predicting ecosystem stability from community composition and biodiversity. Ecol. Lett. 16, 617–625. doi: 10.1111/ele.12088
Díaz, E. R., and McQuaid, C. D. (2011). A spatially explicit approach to trophic interactions and landscape formation: patchiness in small-scale variability of grazing effects along an intertidal stress gradient. J. Ecol. 99, 416–430.
Doak, D. F., Bigger, D., Harding, E. K., Marvier, M. A., Malley, R. E. O., and Thomson, D. (1998). The Statistical inevitability of stability-diversity in community ecology. Am. Nat. 151, 264–276. doi: 10.1086/286117
Donohue, I., Hillebrand, H., Montoya, J. M., Petchey, O. L., Pimm, S. L., Fowler, M. S., et al. (2016). Navigating the complexity of ecological stability. Ecol. Lett. 19, 1172–1185. doi: 10.1111/ele.12648
Duffy, J. E., and Hay, M. E. (1994). Herbivore resistance to seaweed chemical defense: the roles of mobility and predation risk. Ecology 75, 1304–1319.
Edwards, M., and Connell, S. D. (2012). “Competition, a major factor structuring seaweed communities,” in Seaweed biology, eds C. Wiencke and K. Bischof (Berlin: Springer), 135–156. doi: 10.1007/978-3-642-28451-9
Filbee-dexter, K., and Wernberg, T. (2018). Rise of turfs: a new battlefront for globally declining kelp forests. BioScience 68, 64–76. doi: 10.1093/biosci/bix147
Foster, M. S., Nigg, E. W., Kiguchi, L. M., Hardin, D. D., and Pearse, J. S. (2003). Temporal variation and succession in an algal – dominated high intertidal assemblage. J. Exp. Mar. Biol. Ecol. 289, 15–39. doi: 10.1016/S0022-0981(03)00035-2
Gao, G., Clare, A. S., Rose, C., and Caldwell, G. S. (2017). Eutrophication and warming-driven green tides (Ulva rigida) are predicted to increase under future climate change scenarios. Mar. Pollut. Bull. 114, 439–447. doi: 10.1016/j.marpolbul.2016.10.003
Ghedini, G., Russell, B. D., and Connell, S. D. (2015). Trophic compensation reinforces resistance: herbivory absorbs the increasing effects of multiple disturbances. Ecol. Lett. 18, 182–187. doi: 10.1111/ele.12405
González, A., and Loreau, M. (2009). The causes and consequences of compensatory dynamics in ecological communities. Annu. Rev. Ecol. Evol. Syst. 40, 393–414. doi: 10.1146/annurev.ecolsys.39.110707.173349
Grime, J. P. (1998). Benefits of plant diversity to ecosystems: immediate, filter and founder effects. J. Ecol. 86, 902–910. doi: 10.1046/j.1365-2745.1998.00306.x
Grimm, V., and Wissel, C. (1997). Babel or the ecological stability discussions: an inventory and analysis of terminology and a guide for avoiding confusion. Oecologia 1997, 323–334.
Grman, E., Lau, J. A., Schoolmaster, D. R., and Gross, K. L. (2010). Mechanisms contributing to stability in ecosystem function depend on the environmental context. Ecol. Lett. 13, 1400–1410. doi: 10.1111/j.1461-0248.2010.01533.x
Gross, K., Cardinale, B. J., Fox, J. W., Gonzalez, A., Loreau, M., Wayne Polley, H., et al. (2014). Species richness and the temporal stability of biomass production: a new analysis of recent biodiversity experiments. Am. Nat. 183, 1–12. doi: 10.1086/673915
Hallett, L. M., Hsu, J. S., Cleland, E. E., Collins, S. L., Dickson, T. L., Farrer, E. C., et al. (2014). Biotic mechanisms of community stability shift along a precipitation gradient. Ecology 95, 1693–1700. doi: 10.1890/13-0895.1
Hallett, L. M., Jones, S. K., MacDonald, A. A. M., Jones, M. B., Flynn, D. F. B., Ripplinger, J., et al. (2016). codyn: an r package of community dynamics metrics. Methods Ecol. Evol. 7, 1146–1151. doi: 10.1111/2041-210X.12569
Harley, C. D. G., and Helmuth, B. S. T. (2003). Local- and regional-scale effects of wave exposure, thermal stress, and absolute versus effective shore level on patterns of intertidal zonation. Limnol. Oceanogr. 48, 1498–1508.
Harley, C. D. G., Hughes, A. R., Hultgren, K. M., Miner, B. G., Sorte, C. J. B., Thornber, C. S., et al. (2006). The impacts of climate change in coastal marine systems. Ecol. Lett. 9, 228–241. doi: 10.1111/j.1461-0248.2005.00871.x
Harley, C. D. G., Anderson, K. M., Demes, K. W., Jorve, J. P., Kordas, R. L., Coyle, T. A., et al. (2012). Effects of climate change on global seaweed communities. J. Phycol. 48, 1064–1078. doi: 10.1111/j.1529-8817.2012.01224.x
Harrison, X. A., Donaldson, L., Correa-Cano, M. E., Evans, J., Fisher, D. N., Goodwin, C. E. D., et al. (2018). A brief introduction to mixed effects modelling and multi-model inference in ecology. PeerJ. 2018, 1–32. doi: 10.7717/peerj.4794
Haughey, E., Suter, M., Hofer, D., Hoekstra, N. J., McElwain, J. C., Lüscher, A., et al. (2018). Higher species richness enhances yield stability in intensively managed grasslands with experimental disturbance. Sci. Rep. 8, 1–10. doi: 10.1038/s41598-018-33262-9
Hillebrand, H., Bennett, D. M., and Cadotte, M. W. (2008). Concepts and synthesis emphasizing new ideas to stimulate research in ecology consequences of dominance: a review of evenness effects on local and regional ecosystem processes. Ecology. 89, 1510–1520. doi: 10.1890/07-1053.1
Hoffmann, A., and Santelices, B. (1997). Flora marina de Chile continental. Santiago: Ediciones Universidad Católica de Chile, 434.
Hooper, D. U., Adair, E. C., Cardinale, B. J., Byrnes, J. E. K., Hungate, B. A., Matulich, K. L., et al. (2012). A global synthesis reveals biodiversity loss as a major driver of ecosystem change. Nature 486, 105–108. doi: 10.1038/nature11118
Iken, K. (2012). “Grazers on benthic seaweeds,” in Seaweed biology, eds C. Wiencke and K. Bischof (Berlin: Springer), 157–175. doi: 10.1007/978-3-642-28451-9
Ives, A. R., and Carpenter, S. R. (2007). Stability and diversity of ecosystems. Science 317, 58–62.
Ives, A. R., Klug, J. L., and Gross, K. (2000). Stability and species richness in complex communities. Ecol. Lett. 3, 399–411. doi: 10.1046/j.1461-0248.2000.00144.x
Jara, H. F., and Moreno, C. A. (1984). Herbivory and structure in a midlittoral rocky community: a case in southern Chile. Ecology 65, 28–38. doi: 10.2307/1939455
Kéfi, S., Domínguez-García, V., Donohue, I., Fontaine, C., Thébault, E., and Dakos, V. (2019). Advancing our understanding of ecological stability. Ecol. Lett. 22, 1349–1356. doi: 10.1111/ele.13340
Kigel, J., Konsens, I., Segev, U., and Sternberg, M. (2021). Temporal stability of biomass in annual plant communities is driven by species diversity and asynchrony, but not dominance. J. Veg. Sci. 32, 1–12. doi: 10.1111/jvs.13012
Kordas, R. L., and Harley, C. D. G. (2016). Demographic responses of coexisting species to in situ warming. Mar. Ecol. Prog. Ser. 546, 147–161. doi: 10.3354/meps11620
Kordas, R. L., Dudgeon, S., Storey, S., and Harley, C. D. G. (2014). Intertidal community responses to field-based experimental warming. Oikos 124, 888–898. doi: 10.1111/oik.00806
Klug, J. L., Fischer, J. M., Ives, A. R., and Dennis, B. (2000). Compensatory dynamics in planktonic community responses to pH perturbations. Ecology 81, 387–398. doi: 10.2307/177435
Ko, Y. W., Sung, G. H., and Kim, J. H. (2008). Estimation for seaweed biomass using regression: a methodological approach. Algae 23, 289–294.
Lamy, T., Koenigs, C., Holbrook, S. J., Miller, R. J., Stier, A. C., and Reed, D. C. (2020). Foundation species promote community stability by increasing diversity in a giant kelp forest. Ecology 101:5. doi: 10.1002/ecy.2987
Langenheder, S., Bulling, M. T., Prosser, J. I., and Solan, M. (2012). Role of functionally dominant species in varying environmental regimes: evidence for the performance-enhancing effect of biodiversity. BMC Ecol. 12:14. doi: 10.1186/1472-6785-12-14
Leary, D. J., and Petchey, O. L. (2009). Testing a biological mechanism of the insurance hypothesis in experimental aquatic communities. J. Anim. Ecol. 78, 1143–1151. doi: 10.1111/j.1365-2656.2009.01586.x
Lehman, C. L., and Tilman, D. (2000). Biodiversity, stability, and productivity in competitive communities. Am. Nat. 156, 534–552. doi: 10.1086/303402
Littler, M. M., and Littler, D. S. (1984). Relationships between macroalgal functional form groups and substrata stability in a subtropical rocky-intertidal system. J. Exp. Mar. Biol. Ecol 74, 13–34. doi: 10.1016/0022-0981(84)90035-2
Loreau, M. (2000). Biodiversity and ecosystem functioning: recent theoretical advances. Oikos 91, 3–17. doi: 10.1034/j.1600-0706.2000.910101.x
Lopez, G. (2019). Effect of wild-harvest on a commercially important seaweed: A case study of Mazzaella laminarioides (Rhodophyta, Gigartinaceae) in south-central Chile. [Dissertation/PhD Thesis]. Hawai: University of Hawai’i at Mānoa.
Loreau, M., and Hector, A. (2001). Partitioning selection and complementarity in biodiversity experiments. Nature 412, 72–76. doi: 10.1038/35083573
Loreau, M., and De Mazancourt, C. (2008). Species synchrony and its drivers: neutral and nonneutral community dynamics in fluctuating environments. Am. Nat. 172:2. doi: 10.1086/589746
Loreau, M., and de Mazancourt, C. (2013). Biodiversity and ecosystem stability: a synthesis of underlying mechanisms. Ecol. Lett. 16, 106–115. doi: 10.1111/ele.12073
Loreau, M., Barbier, M., Filotas, E., Gravel, D., Isbell, F., Miller, S. J., et al. (2021). Biodiversity as insurance: from concept to measurement and application. Biol. Rev. 96, 2333–2354. doi: 10.1111/brv.12756
Loreau, M., Sapijanskas, J., Isbell, F., and Hector, A. (2012). Niche and fitness differences relate the maintenance of diversity to ecosystem function: comment. Ecology 93, 1482–1487. doi: 10.1890/11-0792.1
Maggi, E., Bertocci, I., Vaselli, S., and Benedetti-Cecchi, L. (2011). Connell and Slatyer’s models of succession in the biodiversity era. Ecology 92, 1399–1406. doi: 10.1890/10-1323.1
Menge, B. A. (1976). Organization of the New England rocky intertidal community: role of predation, competition, and environmental heterogeneity. Ecol. Monog. 46, 355–393. doi: 10.2307/1942563
Miller, R. J., Lafferty, K. D., Lamy, T., Kui, L., Rassweiler, A., and Reed, D. C. (2018). Giant kelp, Macrocystis pyrifera, increases faunal diversity through physical engineering. Proc. Royal Soc. B. 285:1874. doi: 10.1098/rspb.2017.2571
Montecinos, A., Broitman, B. R., Faugeron, S., Haye, P. A., Tellier, F., and Guillemin, M. L. (2012). Species replacement along a linear coastal habitat: phylogeography and speciation in the red alga Mazzaella laminarioides along the south east pacific. BMC Evol. Biol. 12, 1–17. doi: 10.1186/1471-2148-12-97
Moreno, C. A., and Jaramillo, E. (1983). The role of grazers in the zonation of intertidal macroalgae of the Chilean coast. Oikos 41:73. doi: 10.2307/3544348
Moreno, C. A., Sutherland, J. P., and Jara, H. F. (1984). Man as a predator in the intertidal zone of southern Chile. Oikos 42:155. doi: 10.2307/3544787
Moreno, C. A. (2001). Community patterns generated by human harvesting on Chilean shores: a review. Aquat. Conserv.-Mar. Freshw. Ecosyst. 11, 19–30.
Navarrete, S. A., Wieters, E. A., Broitman, B. R., and Castilla, J. C. (2005). Scales of benthic – pelagic coupling and the intensity of species interactions: from recruitment limitation to top-down control. Proc. Natl. Acad. Sci. 102, 18046–18051. doi: 10.1073/pnas.0509119102
Nielsen, K. J., and Navarrete, S. A. (2004). Mesoscale regulation comes from the bottom-up: intertidal interactions between consumers and upwelling. Ecol. Lett. 7, 31–41. doi: 10.1046/j.1461-0248.2003.00542.x
O’Brien, J. M., and Scheibling, R. E. (2018). Low recruitment, high tissue loss, and juvenile mortality limit recovery of kelp following large - scale defoliation. Mar. Biol. 165, 1–19. doi: 10.1007/s00227-018-3423-y
Paine, R. T. (1974). Intertidal community structure: experimental studies on the relationship between a dominant competitor and its principal predator. Oecologia 15, 93–120. doi: 10.1007/BF00345739
Pérez-Mayorga, D. M., Ladah, L. B., Zertuche-González, J. A., Leichter, J. J., Filonov, A. E., and Lavín, M. F. (2011). Nitrogen uptake and growth by the opportunistic macroalga Ulva lactuca (Linnaeus) during the internal tide. J. Exp. Mar. Biol. Ecol 406, 108–115. doi: 10.1016/j.jembe.2011.05.028
Petraitis, P. S., and Dudgeon, S. R. (2020). Declines over the last two decades of five intertidal invertebrate species in the western North Atlantic. Commun. Biol. 3, 1–7. doi: 10.1038/s42003-020-01326-0
Pimm, S. L. (1984). The complexity and stability of ecosystems. Nature 307, 321–326. doi: 10.1038/307321a0
Polley, H. W., Wilsey, B. J., and Derner, J. D. (2003). Do plant species evenness and plant density influence the magnitude of selection and complementarity effects in annual plant species mixtures? Ecol. Lett. 6, 248–256.
Poore, A. G. B., Campbell, A. H., Coleman, R. A., Edgard, G. J., Jormalainen, V., Reynolds, P. L., et al. (2012). Global patterns in the impact of marine herbivores on benthic primary producers. Ecol. Lett. 15, 912–922. doi: 10.1111/j.1461-0248.2012.01804.x
Richards, S. A. (2005). Testing ecological theory using the information-theoretic approach: examples and cautionary results. Ecology 86, 2805–2814.
Richards, S. A. (2008). Dealing with overdispersed count data in applied ecology. J. Appl. Ecol. 2008, 218–227. doi: 10.1111/j.1365-2664.2007.01377.x
Rivadeneira, M. M., Fernández, M., and Navarrete, S. A. (2002). Latitudinal trends of species diversity in rocky intertidal herbivore assemblages: spatial scale and the relationship between local and regional species richness. Mar. Ecol. Prog. Ser. 245, 123–131. doi: 10.3354/meps245123
R Core Team, (2020). R: A language and environment for statistical computing (v.4.0.2) [Computer software]. R Foundation for Statistical Computing. Vienna: R Core Team.
Roberts, E. A., and Bracken, M. E. S. (2021). Intertidal canopy-forming seaweeds modulate understory seaweed photoprotective compounds. J. Phycol. 57, 645–654. doi: 10.1111/jpy.13118
Romanuk, T. N., Beisner, B. E., Martinez, N. D., and Kolasa, J. (2006). Non-omnivorous generality promotes population stability. Biol. Lett. 2, 374–377. doi: 10.1098/rsbl.2006.0464
Rosenberg, C., and Ramus, J. (1982). Ecological growth strategies in the seaweeds Gracilaria foliifera (Rhodophyceae) and Ulva sp. (Chlorophyceae): soluble nitrogen and reserve carbohydrates. Mar. Biol. 66, 251–259. doi: 10.1007/BF00397030
Santelices, B. (1990). Patterns of organizations of intertidal and shallow subtidal vegetation in wave exposed habitats of central Chile. Hydrobiologia 192, 35–57. doi: 10.1007/BF00006226
Santelices, B., Montalva, S., and Oliger, P. (1981). Competitive algal community organization in exposed intertidal habitats from central Chile. Mar. Ecol. Prog. Ser. 6, 267–276. doi: 10.3354/meps006267
Sasaki, T., and Lauenroth, W. K. (2011). Dominant species, rather than diversity, regulates temporal stability of plant communities. Oecologia 166, 761–768. doi: 10.1007/s00442-011-1916-1
Sasaki, T., Lu, X., Hirota, M., and Bai, Y. (2019). Species asynchrony and response diversity determine multifunctional stability of natural grasslands. J. Ecol. 107, 1862–1875. doi: 10.1111/1365-2745.13151
Säterberg, T., Jonsson, T., Yearsley, J., Berg, S., and Ebenman, B. (2019). A potential role for rare species in ecosystem dynamics. Sci. Rep. 9:11107. doi: 10.1038/s41598-019-47541-6
Sousa, W. P. (1979). Disturbance in marine intertidal boulder fields: the nonequilibrium maintenance of species diversity. Ecology 60, 1225–1239.
Sousa, W. P. (1980). The responses of a community to disturbance: the importance of successional age and species’ life histories. Oecologia 45, 72–81. doi: 10.1007/BF00346709
Stachowicz, J. J., Bruno, J. F., and Duffy, J. E. (2007). Understanding the effects of marine biodiversity on communities and ecosystems. Annu. Rev. Ecol. Evol. Syst. 38, 739–766. doi: 10.1146/annurev.ecolsys.38.091206.095659
Stachowicz, J. J., Graham, M., Bracken, M. E. S., and Szoboszlai, A. I. (2008). Diversity enhances cover and stability of seaweed assemblages: the role of heterogeneity and time. Ecology 89, 3008–3019. doi: 10.1890/07-1873.1
Steneck, R. S., and Dethier, M. N. (1994). A functional group approach to the structure of algal-dominated communities. Oikos 69:476. doi: 10.2307/3545860
Strathmann, R. R., Hughes, T. P., Kuris, A. M., Lindeman, K. C., Morgan, S. G., Pandolfi, J. M., et al. (2002). Evolution of local recruitment and its consequences for marine populations. Bull. Mar. Sci. 70, 377–396.
Suárez, J. L., Hansen, M., Urtubia, U., Lenz, M., Valdivia, N., and Thiel, M. (2020). Season-dependent effects of ocean warming on the physiological performance of a native and a non-native sea anemone. J. Exp. Mar. Biol. Ecol. 522:151229. doi: 10.1016/j.jembe.2019.151229
Tejada-Martínez, D., López, D. N., Bonta, C. C., Sepúlveda, R. D., and Valdivia, N. (2016). Positive and negative effects of mesograzers on early-colonizing species in an intertidal rocky-shore community. Ecol. Evol. 6, 2045–7758. doi: 10.1002/ece3.2323
Thibaut, L. M., and Connolly, S. R. (2013). Understanding diversity-stability relationships: towards a unified model of portfolio effects. Ecol. Lett. 16, 140–150. doi: 10.1111/ele.12019
Tilman, D., Reich, P. B., and Knops, J. M. H. (2006). Biodiversity and ecosystem stability in a decade-long grassland experiment. Nature 441, 629–632. doi: 10.1038/nature04742
Underwood, A. J. (2000). Experimental ecology of rocky intertidal habitats: what are we learning? J. Exp. Mar. Biol. Ecol. 250, 51–76. doi: 10.1016/S0022-0981(00)00179-9
Underwood, A. J., Denley, E. J., and Moran, M. J. (1983). Experimental analyses of the structure and dynamics of mid-shore rocky intertidal communities in New South Wales. Oecologia 56, 202–219. doi: 10.1007/BF00379692
Valdivia, N., Golléty, C., Migné, A., Davoult, D., and Molis, M. (2012). Stressed but stable: canopy loss decreased species synchrony and metabolic variability in an intertidal hard-bottom community. PLoS One 7:5. doi: 10.1371/journal.pone.0036541
Valdivia, N., González, A. E., Manzur, T., and Broitman, B. R. (2013). Mesoscale variation of mechanisms contributing to stability in rocky shore communities. PLoS One 8:1. doi: 10.1371/journal.pone.0054159
Valdivia, N., Aguilera, M. A., Navarrete, S. A., and Broitman, B. R. (2015). Disentangling the effects of propagule supply and environmental filtering on the spatial structure of a rocky shore metacommunity. Mar. Ecol. Prog. Ser. 538, 67–79. doi: 10.3354/meps11493
Valdivia, N., Aguilera, M., and Broitman, B. R. (2020). High dimensionality of the stability of a marine benthic ecosystem. Front. Mar. Sci. 7:569650. doi: 10.3389/fmars.2020.569650
Valdivia, N., López, D. N., Fica-Rojas, E., Catalán, A. M., Aguilera, M. A., Araya, M., et al. (2021). Stability of rocky intertidal communities, in response to species removal, varies across spatial scales. Oikos 130, 1385–1398. doi: 10.1111/oik.08267
Valencia, E., de Bello, F., Galland, T., Adler, P. B., Lepš, J., E-Vojtkó, A., et al. (2020). Synchrony matters more than species richness in plant community stability at a global scale. Proc. Natl. Acad. Sci. U.S.A. 117, 24345–24351. doi: 10.1073/pnas.1920405117
Van Meerbeek, K., Jucker, T., and Svenning, J. C. (2021). Unifying the concepts of stability and resilience in ecology. J. Ecol. 109, 3114–3132. doi: 10.1111/1365-2745.13651
van Ruijven, J., and Berendse, F. (2005). Diversity–productivity relationships: initial effects, long-term patterns, and underlying mechanisms. Proc. Natl. Acad. Sci. U.S.A. 102, 695–700. doi: 10.1073/pnas.0407524102
Witman, D. J., Cusson, M., Archambault, P., Pershing, A. J., and Mieszkowska, N. (2008). The relation between productivity and species diversity in temperate arctic marine ecosystems. Ecology 89, 66–80. doi: 10.1890/07-1201.1
Yachi, S., and Loreau, M. (1999). Biodiversity and ecosystem productivity in a fluctuating environment: the insurance hypothesis. Proc. Natl. Acad. Sci. U.S.A. 96, 1463–1468. doi: 10.1073/pnas.96.4.1463
Keywords: canopy-forming species, facilitation, habitat loss, community stability, disturbance, understory seaweeds, seaweed ecology
Citation: Fica-Rojas E, Catalán AM, Broitman BR, Pérez-Matus A and Valdivia N (2022) Independent Effects of Species Removal and Asynchrony on Invariability of an Intertidal Rocky Shore Community. Front. Ecol. Evol. 10:866950. doi: 10.3389/fevo.2022.866950
Received: 31 January 2022; Accepted: 13 May 2022;
Published: 13 June 2022.
Edited by:
Viktoriia Radchuk, Leibniz Institute for Zoo and Wildlife Research (LG), GermanyReviewed by:
Samuel Ross, Okinawa Institute of Science and Technology Graduate University, JapanCopyright © 2022 Fica-Rojas, Catalán, Broitman, Pérez-Matus and Valdivia. This is an open-access article distributed under the terms of the Creative Commons Attribution License (CC BY). The use, distribution or reproduction in other forums is permitted, provided the original author(s) and the copyright owner(s) are credited and that the original publication in this journal is cited, in accordance with accepted academic practice. No use, distribution or reproduction is permitted which does not comply with these terms.
*Correspondence: Eliseo Fica-Rojas, ZWxpaXNlby5zcEBnbWFpbC5jb20=
Disclaimer: All claims expressed in this article are solely those of the authors and do not necessarily represent those of their affiliated organizations, or those of the publisher, the editors and the reviewers. Any product that may be evaluated in this article or claim that may be made by its manufacturer is not guaranteed or endorsed by the publisher.
Research integrity at Frontiers
Learn more about the work of our research integrity team to safeguard the quality of each article we publish.