- 1Institute of Zoology, Poznań University of Life Sciences, Poznań, Poland
- 2Department of Zoology and Animal Ecology, University of Life Sciences, Lublin, Poland
Perceived predation risk to offspring may have similar ultimate community-level impacts to those of consumptive trophic interactions. The present study investigated the behavioral effects of common carp Cyprinus carpio–an omnivorous fish capable of triggering an ecosystem shift to an algae-dominated state–on anurans, using a natural experiment conducted in a system of fish-stocked ponds. We compared oviposition patterns and larval densities of anurans and abundance of zooplankton and phytoplankton in ponds where fish were virtually absent and ponds where common carp was dominant. All studied anuran species bred in fish-poor ponds, while in ponds with high fish densities most of them oviposited infrequently or virtually did not breed. Oviposition habitat selection coupled with fish trophic pressure resulted in diametrically different tadpole densities between fish-poor and fish-dominated ponds. The alimentary tract contents of tadpoles of three locally common anurans, Pelobates fuscus, Hyla orientalis, and Rana temporaria, contained large numbers of unicellular algae, but also significant numbers of zooplanktonic grazers. According to stable nitrogen isotope analyses, tadpoles occupied a trophic level similar to a primary consumer, snail Lymnaea stagnalis, indicating that they fed mainly on algae. While total biomass of crustacean zooplankton did not differ between pond types, chlorophyll a concentrations were low in fish-poor ponds compared to fish-dominated ponds and negatively related to total tadpole biomass. Our findings indicate that scarcity of anuran larvae, resulting mainly from the behavioral responses of breeding anurans to fish predation risk, may facilitate algal production, although ecosystem shifts in the presence of fish occur along more pathways than a top-down cascade.
Introduction
Recent research suggests that behavioral (non-consumptive) effects of predators on prey fitness components can be as important as trophic (consumptive) interactions (Lima, 1998; Schmitz et al., 2004; Preisser et al., 2005). Such effects have consequences not only for the affected prey, but may influence populations of distantly linked species by propagating across trophic levels in a manner analogous to trophic cascades (e.g., McIntosh and Townsend, 1996; Beckerman et al., 1997; Romare and Hansson, 2003; Schmitz et al., 2004; but see Vonesh et al. (2009)). Both top predators and medium-trophic-level omnivores may trigger “behavioral cascades” spreading through food webs down to primary production, although top-down cascades driven by omnivores naturally involve fewer trophic levels. The magnitude of the trophic cascades generated by omnivores has been debated because they feed at more than one trophic level, and thus community-scale effects are likely to be dampened (Polis and Strong, 1996). However, their impact on herbivores can still be strong, while increases in the abundance of primary producers are additionally stimulated by recycling of nutrients originating at higher trophic levels, mimicking or reinforcing a cascade process (Vanni and Layne, 1997; Okun et al., 2008; Fahimipour et al., 2019).
Most studies on behaviorally mediated trophic cascades have focused on how prey engaging in anti-predator behaviors at the expense of foraging, such as avoidance of risky areas, reduced activity, or increased vigilance, benefits certain lower trophic levels (reviewed in Werner and Peacor (2003)). However, behavioral responses need not directly accompany trophic interactions between consumers and their prey, but can be stage-structured, i.e., mediated by the behavior of life stages that are not themselves threatened by predation (Wesner, 2019). A good example is amphibians with larval aquatic stages and (predominantly) terrestrial adult stages. As parents increase their fitness by reducing future predation risk to their offspring, breeding females typically avoid oviposition sites where predators of their eggs or larvae are present (Resetarits, 1996; reviewed in Buxton and Sperry (2017)). These behavioral effects have been shown to strongly influence amphibian distribution in small-scale experimental manipulations (Resetarits et al., 2004; Vonesh et al., 2009). However, large-scale field studies on the behavioral adjustments of amphibians to perceived predation risk to offspring and their trophic consequences in relatively large, speciose water bodies are lacking. Consequently, little is known of whether such effects have a potential to affect trophic cascades in complex food webs, i.e., to mitigate/enhance changes in the structure and abundance of primary producers, ultimately driven by predators threatening larval amphibians.
We investigated behavioral interactions between an omnivorous fish, common carp Cyprinus carpio, and anurans, and their potential role in top-down cascades in the food webs of large, semi-natural ponds. Common carp (hereafter: carp) is globally invasive, and its introductions have been linked to significant ecosystem alterations, such as shifts from a clear-water to an algae-dominated turbid state (Richardson et al., 1990; Bajer et al., 2016). Carp prefers to feed on benthic macroinvertebrates, but also exploits plant material and crustacean zooplankton (Sibbing, 1988; Kloskowski, 2011b), including cladoceran grazers, which play a vital role in controlling phytoplankton. Reduction of grazing zooplankton provides a trophic cascade mechanism leading to algal blooms (Khan, 2003; Kloskowski, 2011c; but see Qin and Threlkeld (1990)). Enhanced internal loading largely associated with sediment displacement due to bottom stirring by carp can also contribute to ecosystem shifts (Parkos et al., 2003; Weber and Brown, 2009; Nieoczym and Kloskowski, 2014). Carp can escape the controlling effects of piscivorous fish by elevating turbidity and nutrient levels and attaining a large body size providing a size-refuge from predators (Crivelli, 1981; Wahl et al., 2011). Notably, carp impact on zooplanktonic grazers can be alleviated by its trophic pressure on secondary consumers, i.e., invertebrate predators of zooplankton (Richardson et al., 1990; Parkos et al., 2003; but see Shen et al. (2021)). Despite its slow approach toward food and lack of oral teeth (Sibbing, 1988), carp has been demonstrated to readily feed on tadpoles in experimental settings and to exclude fish-vulnerable amphibians from waters where it achieves a size allowing predation on tadpoles (Kloskowski, 2011a,c). Questions arise about the behavioral component of carp-amphibian interactions and the role of amphibian larvae in the cascading of carp impact down trophic levels. In temperate regions, most lentic tadpoles are assumed to rely on algae (Seale and Beckvar, 1980; Waringer-Löschenkohl and Schagerl, 2001); as they can reach high densities, some studies have shown a reduction in phytoplankton and algal periphyton in the presence of tadpoles (reviewed in Hocking and Babbitt (2014)). Hence, fish-mediated disruption of phytoplankton regulation by tadpoles could ease ecosystem shifts to an algae-dominated state. However, the role of tadpoles in algae regulation is not unambiguous and may vary between taxa (Altig et al., 2007). While tadpoles of some species are able to digest bloom-forming and/or toxic algae (Seale and Beckvar, 1980; Pryor, 2003), they may also facilitate the growth of nuisance algae; e.g., inedible macroalgae may be enhanced by tadpole consumption of edible epiphytic diatoms or avoidance of filaments (Savage, 1952; Kupferberg, 1997). Moreover, it has been pointed out that tadpoles are opportunistic consumers, also feeding on invertebrate grazers of algae, and thus potentially releasing algae from invertebrate predation (Kupferberg, 1997; see also Schiesari et al. (2009), Arribas et al. (2014)).
We examined anuran oviposition preferences and larval densities in ponds with differing fish status. We checked the diet composition of anuran tadpoles using alimentary tract analyses and assessed the relative trophic positions of fish and tadpoles using nitrogen stable isotope measurements. The stable isotope ratio of nitrogen 15N/14N (δ15N) systematically increases with each trophic step within the food web and is thus a reliable indicator of an organism’s trophic level within a given environment (Minagawa and Wada, 1984; Schoeninger and DeNiro, 1984). To examine the role of anuran larvae in phytoplankton control, we compared the abundance of crustacean zooplankton and phytoplankton between ponds with low densities of tadpoles at high densities of carp and fish-poor ponds with high abundances of tadpoles. We predicted that breeding anurans would be discouraged from oviposition in fish-containing ponds, with the exception of fish-tolerant species. Consequently, anuran tadpoles were expected to occur in insufficient densities to cause an appreciable reduction of algae in the presence of fish. More specifically, we expected high phytoplankton levels in ponds dominated by fish and low phytoplankton levels in fish-poor ponds with high abundance of tadpoles. Provided that the effects of tadpole grazing on algae outweighed those of indirect phytoplankton facilitation, the behavioral cascade generated by amphibian avoidance of fish-dominated habitats would complement and accelerate processes of pond ecosystem state shift driven by fish top-down (via zooplankton predation) and bottom-up (nutrient enrichment) influences.
Materials and Methods
Study System
Field research was carried out on large shallow ponds, resembling natural, semi-permanent lakes and used for extensive culture of carp in eastern Poland (51°17–33′ N, 22°15–26′ E), from early April to mid-June in 2006 and 2007. Data were collected from 29 ponds (12 ponds in 2006 and 17 in 2007), filled in early spring and drained in late autumn. The ponds were morphologically similar despite varying water surface area (2–8 ha), with mean depth of about 0.9–1.2 m and uniform terrestrial buffers (grassed levees). The ponds were nutrient-subsidized, with broken cereal grains added as supplementary food for fish, approximately every 3 days starting in May, in the amount of about 1% of the assumed current biomass of fish in the ponds. Natural colonization of ponds by other wildlife was unrestricted, encouraged by the presence of emergent vegetation along the shores. The ponds were of regular shape and similar in morphology. The proportion of pond surface area covered by emergent aquatic macrophytes varied among ponds (range about 0.1–0.5, as quantified from digitized aerial photographs), but all ponds contained beds of emergent vegetation, several meters wide, which could serve as egg-laying habitat and refuges for amphibians. Water depth became shallower toward the shore, but except for ditches regulating water supply, it was fairly uniform across the pond, so that rooted submerged macrophytes were able to colonize the entire pond bottom, while scattered patches of emergent vegetation occasionally appeared in the central part of the pond.
As they were artificially stocked with carp and maintained in a similar manner, the ponds offered an opportunity for a large-scale natural experiment to examine the effects of fish on pond trophic webs. The ponds could be divided into two types during the study period. 1. “Fish-dominated ponds” (n = 19), stocked with one- or 2-year-old carp, 12–22 cm total length (weight about 30–330 g) in late March-early April. The standing biomasses of carp were relatively high, about 100–300 kg/ha, but still below the total biomass levels achieved by carp in invaded ecosystems (reviewed in Weber and Brown (2009)). Since large-sized piscivorous fish were absent, the ponds supported relatively simple food webs structured by omnivorous fish. Large predatory insects and some piscivorous birds could occupy a higher trophic position in the ponds, but could not control carp due to its large individual body size (Kloskowski and Trembaczowski, 2015). 2. “Fish-poor ponds” used as “controls” (n = 10), which remained practically fishless over the study period apart from sparse populations of wild fish that were able to invade the ponds. Water intakes were screened (mesh size 1–2 mm in fish-poor ponds, 5 mm in fish-dominated ponds) to prevent intrusion of wild fish, so unstocked small fish that appeared in the ponds were of no ecological importance in the study period. Also, the fish-poor ponds were stocked with carp larvae (at an individual body mass of 1.5–3 mg) by local fisheries in late May and early June, so the study was terminated in both years on June 10, no more than 2–3 weeks after the introduction of larvae to individual ponds. We assumed that during the first 2–3 weeks the stocked larvae were too small to affect pond food web dynamics; however, carp larvae may shift to grazing on small crustacean zooplankton soon after the onset of exogenous feeding (Lechelt and Bajer, 2016). The study ponds were haphazardly chosen from a larger set of ponds interspersed in four clusters, scattered 10–50 km from one another. From three of the clusters, we used 2 and 2, 11 and 2, and 1 and 6 fish-dominated and fish-poor ponds, respectively, in the research; in the fourth cluster five fish-dominated ponds were examined. Each pond was in close proximity to at least one pond of each fish status (<1 km apart), so sampling of alternative habitat patches did not require long-distance movements by breeding anuran females. The two pond types did not differ in depth or in emergent vegetation cover; however, although the ranges overlapped, fish-dominated ponds were generally larger in surface area than fish-poor ponds (means ± SE, 5.1 ± 0.6 vs. 3.1 ± 0.3 ha, respectively).
Surveys of Oviposition Habitat Preferences and Tadpole Densities
The ponds were searched for spawn of six anuran species commonly breeding in the study area: Pelobates fuscus, Bufo bufo, Bufotes viridis, Hyla orientalis, Rana temporaria, and Rana arvalis. All these species can be involved in algae control because they have gill filters, enabling selection of small suspended particles, and keratinized mouth-parts which allow them to scrape at surfaces (Savage, 1952; Kenny, 1969). Four visits to each pond were conducted between 8.00 and 18.00 h at approximately weekly intervals between April 5 and May 6. Based on irregular pond visits in March and mid-May, we assumed that the survey period encompassed all or most of the egg-laying period of the species, as they all had one breeding peak in the spring, except for B. viridis, which is known to have a prolonged reproductive season (Berger, 2000). When detected late after laying, spawn clumps of the two Rana species could not be reliably distinguished in the field, so they were combined in the analyses (the two species often co-occurred in the same ponds). Two other locally common anuran taxa, Bombina bombina and Pelophylax spp. (Pelophylax esculenta complex), were not considered because they typically bred later in the season. All vegetated areas within the pond perimeter, except for the interior of dense reedbed patches, were searched for egg masses by systematic wading; shallow areas with unvegetated substrate were also checked for B. viridis spawn. Oviposition was recorded as presence/absence, since it was not feasible to identify individual egg masses within their communal aggregates, especially for bufonids. Estimates of egg mass densities were obtained only for Rana spp., based on counts of spawn clumps conducted while wading 1–5 m from the shoreline along two 50-m transects haphazardly placed in vegetated areas on opposite pond margins. Locations of egg masses were marked with plastic strips tied to emergent vegetation. Spawn losses due to carp predation were not observed (Kloskowski, 2020), so we assumed that egg predation did not bias our estimates of oviposition habitat selection. The results were compared with activity trap catches of tadpoles (see below). Generally, trapping did not reveal the presence of any species not detected earlier by egg mass surveys, except one pond in which B. bufo spawn had been overlooked.
Tadpole densities in the ponds were estimated using submerged 1-L funnel (100 mm at the large end and 23 mm at the inward-directed opening) activity traps, at approximately 2-week intervals between April 20 and June 10 (four sampling sessions per pond, 10 traps per session). Traps were placed horizontally on the bottom or on submerged macrophytes, at the interface between open water and emergent vegetation to sample both habitats, and retrieved after 24 h. The distance from the shore varied from one to several meters depending on the width of the shoreline vegetation at a given site. The traps were set roughly equidistant from one another, at least 20 m apart. Captured tadpoles were counted and wet-weighed after removing excess water. Again, R. temporaria and R. arvalis were pooled to genus. Species richness was derived from the presence–absence of species during any of the sampling sessions (either spawn searches or tadpole trapping).
Analyses of Tadpole Gut Contents
Three regionally common anuran species, P. fuscus, H. orientalis, and R. temporaria, known to be vulnerable to fish predation but differing in breeding phenology, were sampled for conventional dietary analyses. Tadpoles at Gosner stage 30–33 (Gosner, 1960) were collected opportunistically in late May-early June 2006 and 2007. Since R. temporaria tadpoles were difficult to distinguish from R. arvalis, they were collected from ponds where only R. temporaria spawn had been found. Other anurans were collected as well, but their samples were insufficient for reliable diet estimation. Freshly dead individuals, typically those which succumbed in traps during trapping sessions, were preserved in 4% formalin. The intestines were removed, and the alimentary tract contents, omitting the posterior portion of the gut, were examined microscopically in a Sedgewick–Rafter counting chamber (unicellular organisms were counted from subsamples). We aimed to determine the functional role of tadpoles from the composition of ingested food (phytophagous vs. zoophagous, feeding on algae or on grazers on algae), and not interspecific differences in diet and habitat use. Hence, taxonomic identification was made to the lowest practical level. Ciliates, which numerically constituted <1% of unicellular food, and detritus were omitted from the analyses. Owing to size disparity between invertebrate prey (especially macroinvertebrates) and algae, food composition (based on numerical percentages of the taxa ingested) was calculated separately for these two groups. For simplicity, all taxa traditionally considered as algae were included as such.
Stable Isotope Analyses
The trophic levels of carp and tadpoles of the species sampled for dietary analyses were compared using the stable isotope of nitrogen 15N. To avoid issues with estimating relative trophic positions due to spatial variation in baseline isotope signatures (Cabana and Rasmussen, 1996) of individual pond food webs, tadpoles were collected for SIA from a subset of ponds belonging to one pond cluster, supplied with water from the same river (the ponds also received water from precipitation) and with sediments consisting of similar matter (mixed organic and mineral components). We did not make baseline adjustments, as in an earlier study we found no significant between-pond differences in the δ15N values (mean ≈ 3.2) of phytophagous leaf beetles Donacia cinerea, the lowest trophic rank primary consumers sampled in the study area (Kloskowski and Trembaczowski, 2015). Here, to assess the relative trophic levels of tadpoles within the food web, we used snails Lymnaea stagnalis as long-living consumers at the base of the food web (Brönmark et al., 1991; Post, 2002; Schiesari et al., 2009). However, they showed some between- and within-pond variation in δ15N signals (see section “Results”).
Carp were collected from the end of April until the end of May. Tadpoles were sampled on the same occasions as specimens for gut content analyses (late May-early June), and similarly, were mainly (except 1–2 specimens of each species) from fish-poor ponds due to the shortage of tadpoles in fish-dominated ponds. Snails were collected along with tadpoles. Samples were individually frozen upon collection and later dried and homogenized in a mortar; white muscles were used in carp, while tadpoles and snails were ground whole (snail shells were removed).
The δ15N of the samples was measured by Elemental Analysis-Isotope Ratio Mass Spectrometry at the Iso-Analytical Laboratory (Cheshire, United Kingdom). Results are expressed relative to atmospheric nitrogen.
Zooplankton and Phytoplankton Assessment
Microcrustacean zooplankton was sampled in the last week of May of each year. Samples were taken from four sites roughly evenly distributed over the open water zone in each pond, in similar weather conditions, between 10:00 and 14:00 h. The entire water column was sampled with a 7 L tube sampler. Zooplankters were concentrated with a 55-mm-mesh net and preserved in a formalin–glycerin solution. Cladocerans were identified to genus when possible, and copepods to order. Copepod nauplii were omitted from analyses due to their small size. The zooplankters were enumerated in a Sedgewick-Rafter chamber and measured under a light microscope. Dry weights were derived from length–weight relationships (Dumont et al., 1975; Bottrell et al., 1976). Densities of large-bodied cladocerans (predominantly Daphnia spp., Scapholeberis mucronata, Moina spp., Diaphanosoma brachyurum, and Sida crystallina) and of small-bodied cladocerans (predominantly Bosmina longirostris, Ceriodaphnia spp., Alona spp., Chydorus sphaericus) were estimated separately, because large cladocerans are particularly effective at maintaining water clarity and grazing on phytoplankton, while at the same time they are often preferentially exploited by fish (e.g., Brooks and Dodson, 1965; Pace, 1984; Hambright and Hall, 1992; Potužák et al., 2007).
Chlorophyll a concentrations were used as a proxy for phytoplankton biomass. In each of the ponds water was sampled at two sites 1–2 m from the shoreline vegetation between June 6 and 10. 500-mL water samples were collected from mid-depth, excluding the near-bottom water column on the assumption that near-bottom chlorophyll a would be affected by resuspension of sediment particles by carp in fish-dominated ponds. Water was filtered through Whatman GF/C filters. Chlorophyll a content was determined spectrophotometrically (ethanol extraction method; DU 640 spectrophotometer, Beckman Instruments Inc., United States). Water transparency was measured with a 12-cm Secchi disc at the deepest sites in each pond in the last week of May.
Statistics
All statistics were conducted using Genstat 15 (VSN International, 2012). Most of the analyses were comparisons of the biomass or numerical densities of key food web components between fish-dominated and fish-poor ponds. Binomially distributed data, such as anuran species occurrence in ponds, were analyzed using Generalized Linear Mixed Models (GLMMs) with a logit link function. To examine differences in anuran species richness with respect to fish status in the pond, species number was modeled as a binomially distributed variable with the maximum number of species possible (n = 5) as the denominator. In the binomial models, any overdispersion in the data was controlled using the Williams procedure (Williams, 1982). Continuous response variables, such as zooplankton abundance (biomass or numerical densities), as well as chlorophyll a and water transparency, were analyzed using residual maximum likelihood (REML) models. Residual plots were visually evaluated to ensure that each dataset met the assumption of normally distributed residual errors. Significance of the only fixed term (fish status in the pond or total tadpole biomass) was assessed by the Wald test. Means were compared using standard error of the difference. As the pond clusters were scattered 10–40 km apart, cluster identity was fitted as a random intercept to account for potential autocorrelations. Although we assumed that ecological conditions in the ponds did not differ between the study years, as they were determined by the fish stocking regime, study year was initially considered as a fixed effect, but then omitted as insignificant to simplify the models. Similarly, pond surface area and the proportion of emergent vegetation were not included because preliminary analyses suggested that they had no impact on oviposition habitat selection by anurans. The effects of total tadpole biomass on zooplankton and chlorophyll a were also tested, but in separate models because tadpole biomass and fish status were strongly related. For analyses of the effects of tadpoles on phytoplankton and zooplankton (see below), we used the relative total biomass of tadpoles assessed during the last sampling session (June 5–10). At this time tadpole biomass was at its highest; also, tadpole sampling in June was concurrent with sampling of chlorophyll a and zooplankton. The relative total biomass was strongly correlated with the total number of tadpoles captured per 10 traps during June sampling (Pearson r = 0.904, P < 0.001).
The relative numerical and biomass densities of tadpoles were compared between pond types using REML repeated measures analysis with trapping session as the repeated factor. Multiple comparisons were carried out using the least significant difference (LSD) test (significance level 5%). We omitted the data from the first trapping session (April) because in most ponds no tadpoles were caught. Differences in δ15N levels between fish, the three anuran species, and Lymnaea snails were assessed using a one-factor analysis of variance (ANOVA), followed by post-hoc Tukey’s tests. Comparisons of anuran species richness within pond types were conducted using the non-parametric Wilcoxon-test due to small sample sizes.
Results
Oviposition Habitat Selection by Anurans
Spawn of P. fuscus and B. viridis was virtually absent from the fish-dominated ponds (each species was recorded in only one pond). Only one egg mass of H. orientalis was found in each of three of the fish-dominated ponds, while they were numerous (>>10) in each of the fish-poor ponds where spawn presence was noted. The occurrence of Rana spp. and B. bufo egg masses did not differ between the two pond types (Table 1 and Figure 1). Although Rana spawn was found in most fish-dominated ponds as well, the fish-poor ponds supported significantly higher densities of egg clumps (means 19.8 ± SE 9.0 vs. 83.4 ± 13.1 per 100 m shoreline; REML, Wald χ2 = 15.05, df = 1, P < 0.001). Anuran species richness based on egg mass presence/absence was higher in fish-poor than in fish-dominated ponds (binomial GLMM, Wald χ2 = 25.39, df = 1, P < 0.001; Figure 2). In three fish-dominated ponds no spawn or tadpoles were recorded.
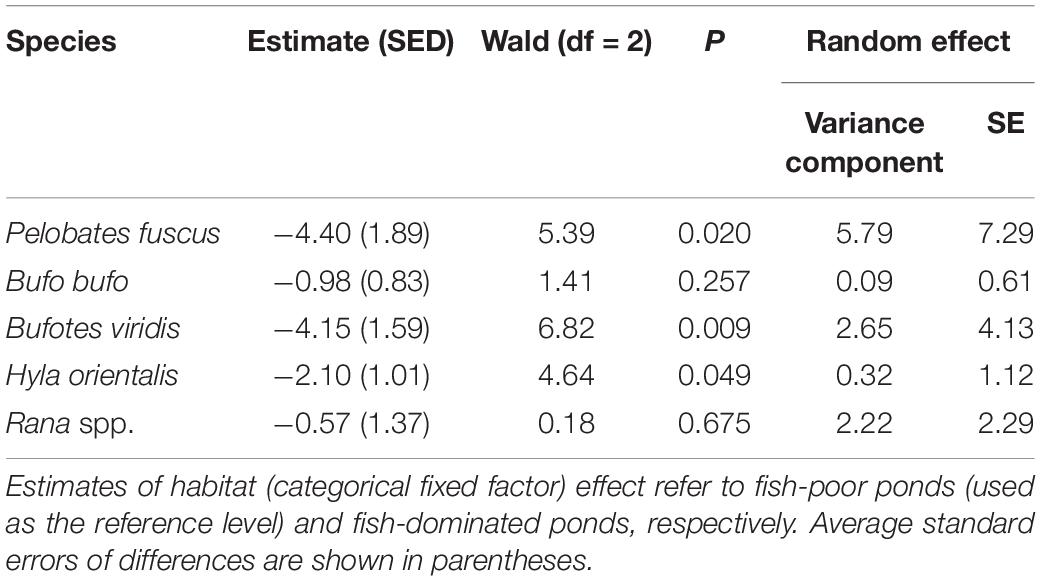
Table 1. Results of the fixed part of GLMM analyses (binomial errors, logit link function) relating presence/absence of anuran spawn to oviposition habitat type.
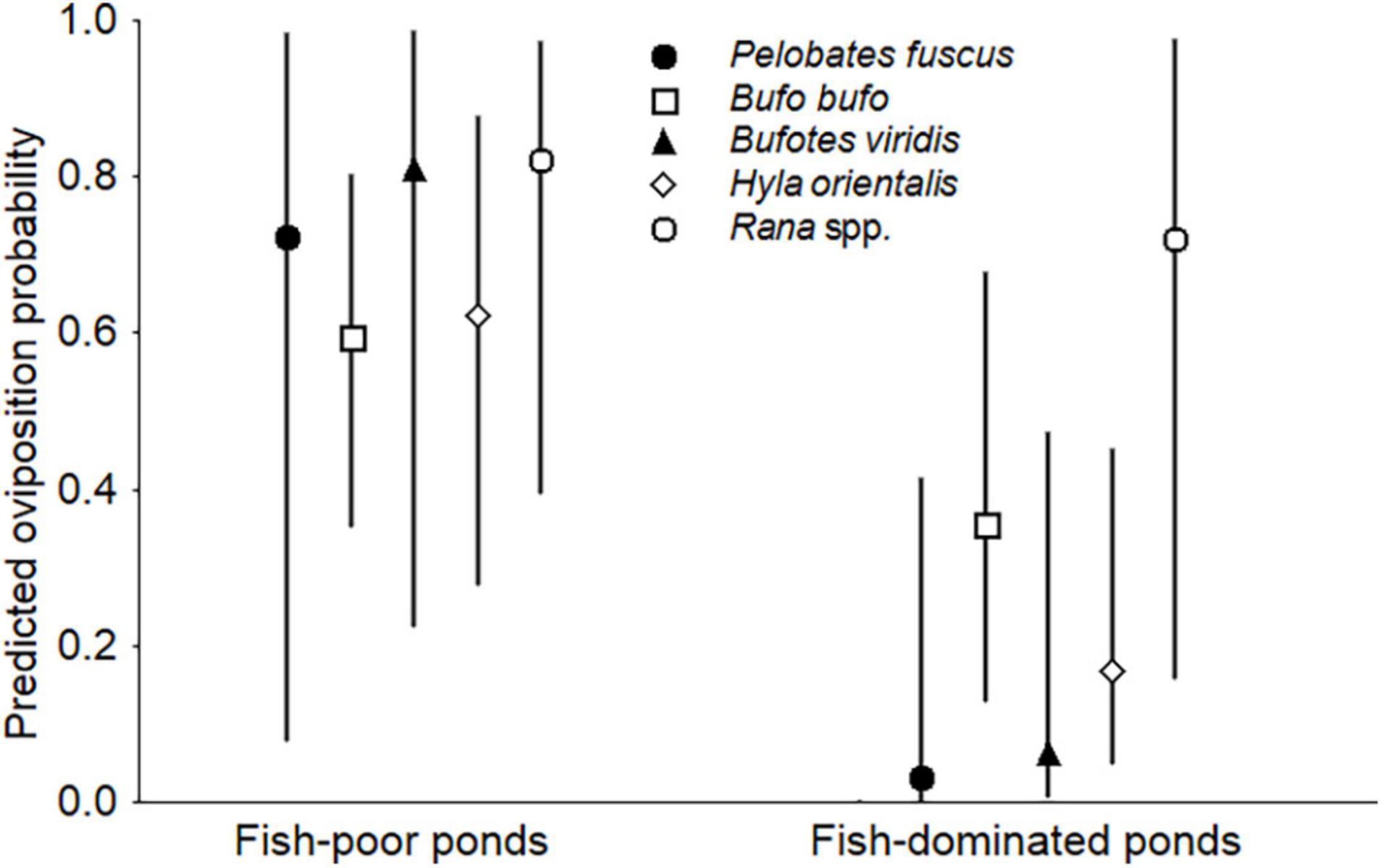
Figure 1. Mean proportional occurrence of anuran taxa in fish-poor and fish-dominated ponds (proportion of ponds with taxon present). Symbols and error bars represent back-calculated means and ± 95% confidence intervals, respectively, from binomial GLMM.
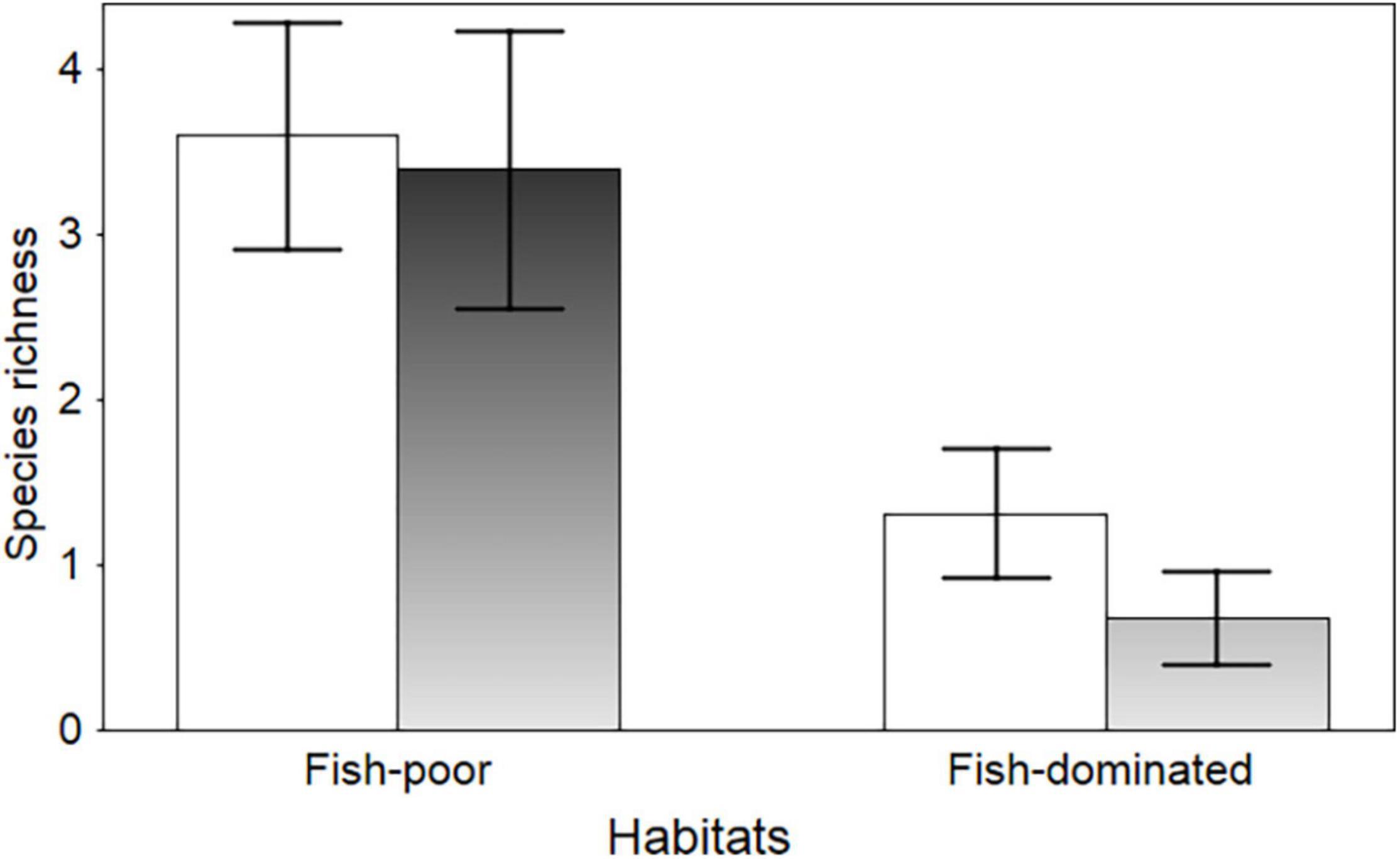
Figure 2. Anuran species richness based on spawn surveys (open bars) and on trapping of tadpoles (filled bars) in fish-poor and fish-dominated ponds. Data shown are means and ± 95% confidence intervals.
Residual maximum likelihood repeated measures analysis showed that the numerical density and total biomass of tadpoles per pond was clearly higher in fish-poor ponds than in fish-dominated ponds (F = 9.16, df = 1, 26.9, P = 0.005 and F = 8.42, df = 1, 27, P = 0.007, respectively; Figure 3). The time effect was significant only for tadpole biomass (F = 12.66, df = 2, 26, P < 0.001). In both models, there was a significant interaction between time and fish status (both P ≤ 0.027), with tadpole abundance/biomass increasing over the study period in fish-poor ponds. Larval anuran species richness showed a similar pattern (binomial GLMM, Wald χ2 = 37.21, df = 1, P < 0.001; Figure 2). Anuran species richness based on spawn surveys was highly correlated with species richness based on trapping of tadpoles (Pearson r = 0.918, P < 0.001). The two measures of species richness did not differ for fish-poor ponds (Wilcoxon test, Z = 1.34, P = 0.178), while in fish-dominated ponds tadpole species richness was consistently lower than species richness from spawn surveys (Z = 2.93, P = 0.003). Pelophylax tadpoles captured during June sampling were not considered, but they occurred nearly exclusively in fish-poor ponds.
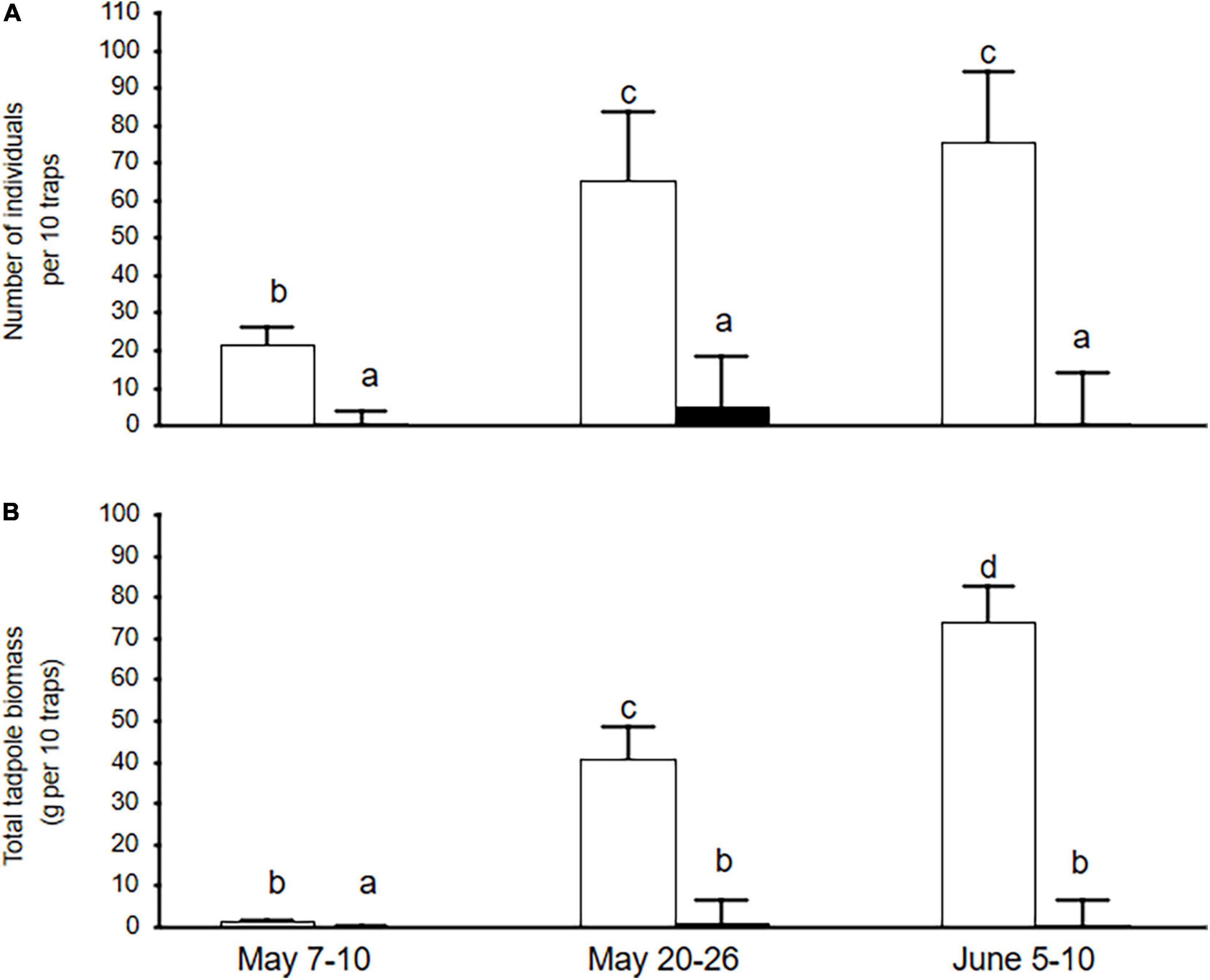
Figure 3. Total numerical (A) and biomass (B) densities (means ± SE) of tadpoles in fish-poor (open bars) and fish-dominated (filled bars) ponds (REML repeated measures analysis). Data were based on three trapping sessions at approximately 2-week intervals. Different letters indicate significant differences between means according to the LSD test.
Tadpole Diet
Unicellular algae were the most abundant category of food ingested, present in all examined alimentary tracts. The total numbers of ingested cells in the analyzed portions of the guts were on average about 86.8 × 103 (± SE 17.7 × 103) cells in P. fuscus, 36.3 × 103 (± 8.2 × 103) in H. orientalis and 78.8 × 103 (± 20.1 × 103) cells in R. temporaria. The guts of some P. fuscus and H. orientalis also contained filamentous algae (Chlorophyta). Chlorophyta, Bacillariophyceae and Raphidophyceae dominated algae remains (together numerically >96% in in the algal diet composition of all studied anurans; Table 2). The guts of all specimens except for a few H. orientalis contained rotifers. The alimentary tracts of most tadpoles contained crustacean zooplankton, especially cladocerans (means ± SE, from 11.2 ± 2.6 individuals per gut in R. temporaria to 20.9 ± 4.2 in P. fuscus). Ostracoda were also common in the diet (from 5.3 ± 1.3 individuals per gut in R. temporaria to 6.5 ± 2.1 in P. fuscus). Insects, mainly dipterans, were present but infrequent in the alimentary tracts of all species studied (Table 2).
Stable Isotopes
The δ15N values of consumers collected from the ponds showed a significant separation (ANOVA F = 7.60, df = 4, 54, P < 0.001). Post-hoc Tukey’s HSD with unequal n-tests revealed that carp was the only species significantly different (more 15N enriched) from all other species sampled (all P < 0.017) (Figure 4). Tadpole isotopic values were similar to those of Lymnaea snail tissues (all P ≥ 0.334) and did not differ among anuran species (all P ≥ 0.457) (Figure 4). The mean differences in δ15N signals between carp and anurans ranged from 3.0‰ (H. orientalis) to 1.9‰ (P. fuscus).
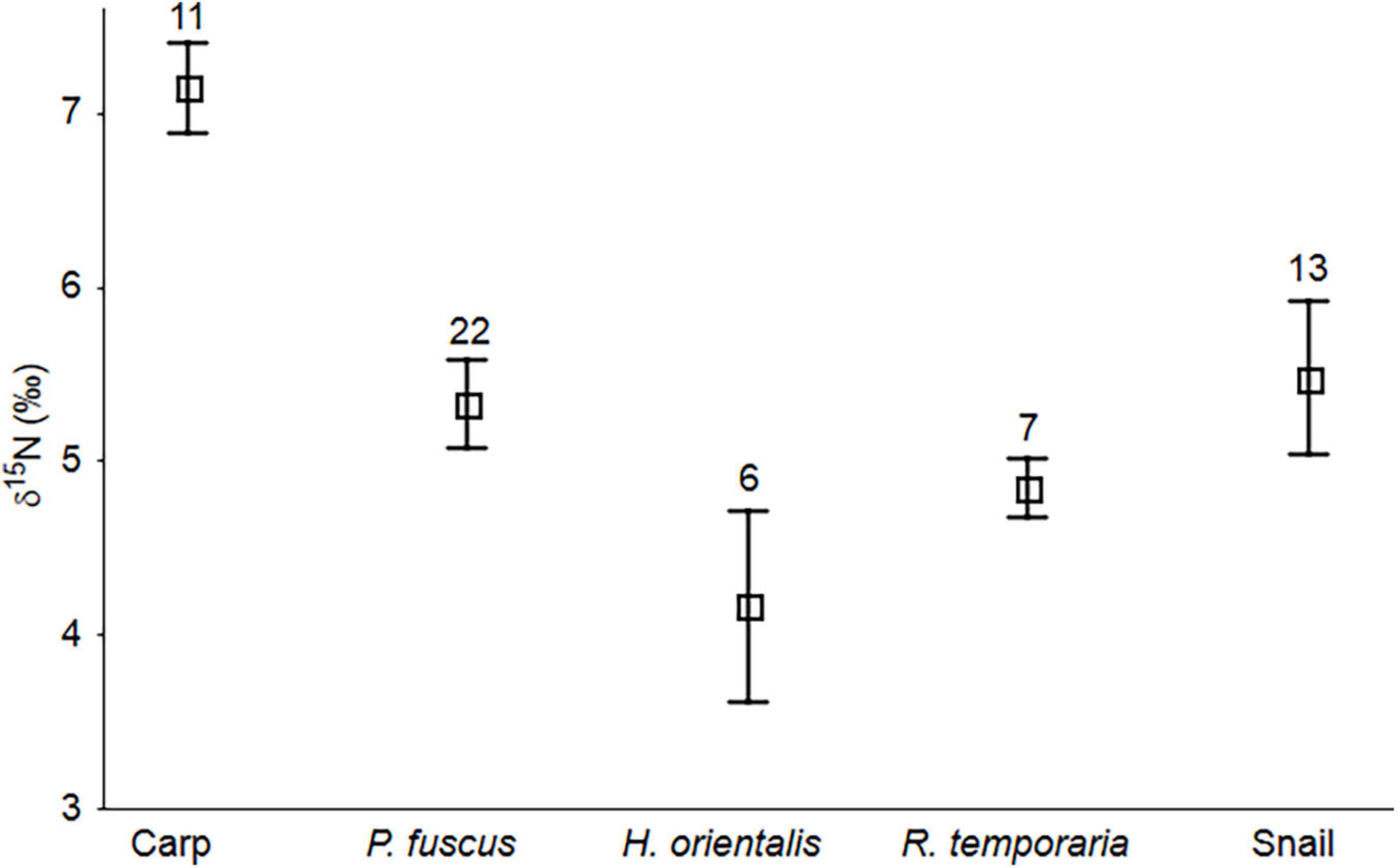
Figure 4. Mean (± SE) stable nitrogen isotope values of fish (common carp Cyprinus carpio), anuran tadpoles (Pelobates fuscus, Hyla orientalis, and Rana temporaria) and snails Lymnaea stagnalis. Sample sizes are shown above the whiskers. Common carp was the only species differing (significantly more enriched in 15N) from the other consumers collected from the study ponds.
Zooplankton and Phytoplankton Abundance
We found no differences in the total biomass of crustacean zooplankton (means ± SE, 0.46 ± 0.30 vs. 0.93 ± 0.36 mg L–1, REML, Wald χ2 = 0.21, df = 1, P = 0.644) between fish-dominated ponds and fish-poor ponds. Large-bodied cladocerans did not significantly differ in densities depending on the fish status of the ponds (50.3 ± 6.6 vs. 39.8 ± 12.4 individuals L–1, respectively; Wald χ2 = 0.47, df = 1, P = 0.491); nor did copepods (74.2 ± 15.9 vs. 38.7 ± 11.5 individuals L–1, respectively; F = 3.28, df = 1, 27, P = 0.081). Small cladocerans tended to be more abundant in fish-dominated ponds (275.9 ± 53.8 vs. 21.1 ± 98.8 individuals L–1, respectively; Wald χ2 = 4.32, df = 1, P = 0.038). Total biomass of zooplankton, copepods, and cladocerans, considered separately, was unaffected by tadpole biomass density (Wald χ2 ≤ 2.60, all P ≥ 0.124).
Chlorophyll a concentrations were clearly higher in fish-dominated ponds than in fish-poor ponds (Wald χ2 = 7.80, df = 1, P = 0.018; Figure 5) and tended to decrease as tadpole biomass density increased (Wald χ2 = 6.08, df = 1, P = 0.038). Secchi depth of fish-poor ponds was nearly twice that of the fish-dominated ponds, means ± SE, 127.2 ± 8.4 cm (in some ponds the Secchi disc was visible on the bottom) vs. 67.6 ± 5.5 cm, respectively; Wald χ2 = 32.37, df = 1, P < 0.001. Blooms of algae were observed in most of the fish-dominated ponds after the termination of the study, in July and August.
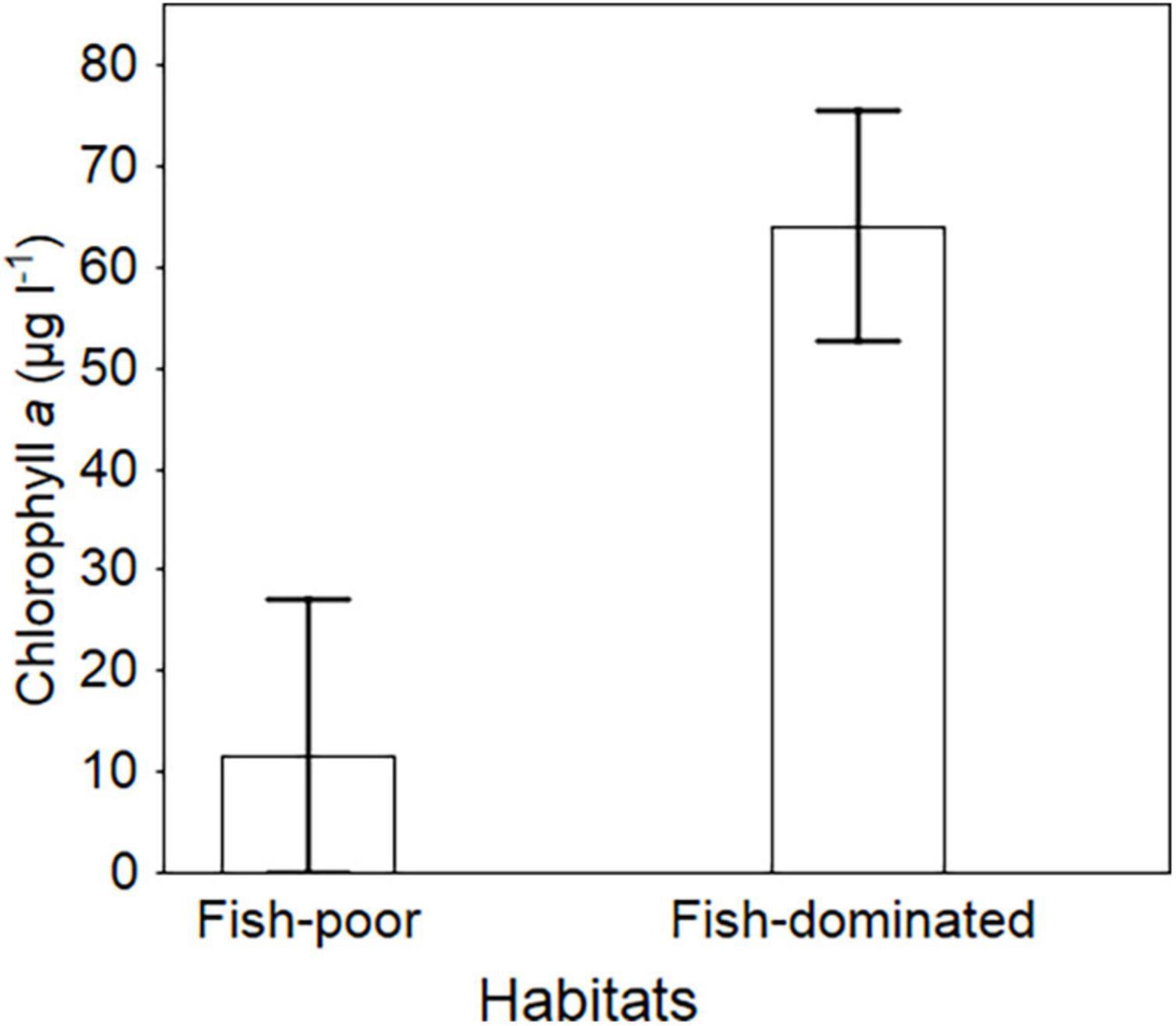
Figure 5. Residual maximum likelihood (REML)-predicted mean (± SE) chlorophyll a concentrations in fish-poor and fish-dominated ponds.
Discussion
Our study demonstrates strong behavioral effects of fish on anuran occurrence in ponds. Besides B. bufo, a species assumed to be unpalatable to cyprinid fishes (Sinsch et al., 2009; Kloskowski, 2011a), only Rana spawn occurred more commonly in fish-dominated ponds, but the densities of Rana egg clumps were markedly lower than in fish-poor ponds. The study design did not allow us to disentangle life-stage-specific effects, i.e., breeding site selection (behavioral) vs. larval mortality (trophic). However, in the fish-dominated ponds that were completely avoided by individual species or even all anuran species, actual predation obviously never occurred. Thus, behavioral effects on breeding anuran females were evidently more important. Additionally, amphibian larvae in the presence of fish tend to change habitat from open water to the littoral margins (Kenison et al., 2016; Kloskowski et al., 2020), potentially relieving open-water phytoplankton. The role of actual predation is unknown, so it is not clear whether fish could entirely eliminate fish-vulnerable anurans from the fish-dominated ponds where their egg masses had been observed; ample littoral vegetation offered structural refuges allowing a certain fraction of tadpoles to survive (Kloskowski et al., 2020). Anuran richness based on tadpole catches was lower than that based on spawn surveys, indicating that fish predation supplemented the behavioral effects, although it cannot be ruled out that trapping was less effective at species detection than searching for egg masses. Overall, total fish effects on anuran breeding success were catastrophic, with tadpole biomass about three orders of magnitude lower in fish-dominated ponds than in fish-poor ponds in June. While in the latter ponds total biomass densities of tadpoles increased over the study, in fish-dominated ponds they remained at a steady low level. Consequently, any anurans able to persist in fish-dominated ponds were unlikely to functionally compensate for the virtual absence of fish-vulnerable species. It should be noted that fish also exert a strong adverse impact on aquatic insects feeding on zooplankton, as well as on large insect predators (larval Anisoptera and Dytiscidae), which can control larval amphibian populations (Wellborn et al., 1996). It is unclear to what degree insect scarcity in ponds containing fish can be attributed to the same antipredator strategy as that of breeding anurans (Šigutová et al., 2021).
Assuming the average isotopic increment of about 3.4‰ between trophic levels in aquatic ecosystems (Minagawa and Wada, 1984; Post, 2002), carp and tadpoles differed in trophic position by less than one trophic step. Our results show that an omnivore predator such as carp, which does not specialize in feeding on large-bodied, evasive prey, may have a strong deterrent effect on anurans, largely or entirely excluding some species from otherwise suitable habitats. Although carp from the study ponds relied largely on animal food, the low δ15N values were apparently affected by the contribution of seeds and detritus to its diet (Crivelli, 1981; Khan, 2003; Kloskowski, 2011b). Given carp’s foraging versatility, the isotopic signatures may not fully reflect its ability to feed on aquatic macrozoa and its potential threat to amphibian larvae (cf. Kloskowski, 2011c).
Chlorophyll a concentrations indicative of phytoplankton abundance were clearly higher in fish-dominated ponds than in fish-poor ponds. Carp is known to feed on zooplankton (Sibbing, 1988; Khan, 2003; Kloskowski, 2011b), but it would be difficult to ascribe high phytoplankton levels to mere suppression of zooplanktonic grazers (cf. Richardson et al., 1990; Parkos et al., 2003; Wahl et al., 2011). The abundance of crustacean zooplankton and its two most important taxonomic and functional groups did not differ depending on fish status, except for higher densities of small cladocerans in fish-dominated ponds. Zooplankton was sampled during daytime hours, so the data could have been affected by diel migration of zooplankton in the presence of fish (e.g., Gliwicz, 1986; Lauridsen and Buenk, 1996); however, underestimation of zooplankton densities could not explain the higher phytoplankton production in fish-dominated ponds. The fish-poor ponds supported high densities of larval anurans, and even if zooplankton was controlled by tadpoles instead of fish, this did not lead to an increase in phytoplankton. We cannot rule out that fish larvae present in fish-poor ponds affected zooplankton densities, but this would only point to the significance of herbivores other than zooplankton. Carp may affect zooplankton–phytoplankton relations in a more subtle way, by size-selective predation on zooplankton groups, e.g., large fish can be ineffective in handling small plankters (Sibbing, 1988; Richardson et al., 1990; Nieoczym and Kloskowski, 2014). Carp-driven shifts from a clear water phase to an algae-dominated turbid state can be also explained by internal loading due to benthivory (Parkos et al., 2003; Weber and Brown, 2009; Nieoczym and Kloskowski, 2014; but see Qin and Threlkeld (1990)), especially in ecosystems with external nutrient addition, like our fish-dominated ponds. However, fish-dominated and fish-poor ponds did not differ in nutrient levels, as indicated by the total nitrogen and total phosphorus concentrations (Nieoczym et al., 2020). Also, provided that large daphnids persist, phytoplankton can be held at low densities despite high nutrient concentrations in ponds (Fott et al., 1980). Bottom-feeding by fish may also adversely influence submerged vegetation, thus boosting algal growth (Kloskowski, 2011c; Bajer et al., 2016).
High numbers of unicellular algae and the occasional occurrence of filamentous species were recorded in tadpole alimentary tracts. Thus, the strong deterrent impact of carp on breeding anurans, associated with high phytoplankton abundance in fish-dominated ponds, suggests the presence of a behavioral cascade mechanism releasing algal primary producers from tadpole grazing. The question remains as to the degree to which algal communities are regulated by tadpoles. Larval anuran can vary considerably in their impact on ecosystem functioning (Arribas et al., 2014). Some species feed to a large extent by grazing on surfaces (Brönmark et al., 1991; Kupferberg, 1997), which may reduce their role in algae control compared to herbivorous zooplankton. Most tadpoles, however, especially in the absence of fish, were shown to stay in the open-water zone of the ponds (Kloskowski et al., 2020), where submerged plants (as substrates for grazing) were scarce in spring, so filter feeding in the water column was likely a major foraging mode. Also, epiphytic grazing may increase the availability of light and carbon dioxide for submerged vegetation, counteracting the effects of fish on the macrophyte–phytoplankton balance (Williams et al., 2002). We did not find toxic algae in tadpole food, which could indicate that tadpole grazing may by insufficient for effective control of algae blooms (Kupferberg, 1997). However, in our study tadpoles were sampled in late May-early June, while algae blooms occurred in fish-dominated ponds later in the season, after tadpoles of most anuran species had completed metamorphosis and left the ponds. Zooplankton, especially cladocerans, was a common prey of tadpoles as well, indicating that they may play dual roles as phytoplankton-feeders and macrophagous predators, including grazers of phytoplankton in their diet (Petranka and Kennedy, 1999; Altig et al., 2007; Arribas et al., 2014). However, δ15N measurements showed that the trophic positions of anuran tadpoles were similar to those of Lymnaea snails, assumed to be basal consumers of pond food chains (but see Doi et al. (2010)). These findings suggest that, apart from certain interspecific differences in trophic levels, the anurans studied to a large extent feed as primary consumers. Foraging by tadpoles on planktonic herbivores may serve as intraguild predation (Polis and Holt, 1992), only partially relieving algae from top-down grazer control. If predation on invertebrate phytoplankton-feeders was of greater importance in organizing the pond community than microphagous suspension feeding, phytoplankton productivity would be expected to increase with tadpole density. Instead, the reverse pattern was observed, although it may have been biased by the effects of carp.
The exploitation of multiple trophic levels by carp and not least its ecosystem engineering through bioturbation and excretion create multiple pathways of impact on primary productivity and of ecosystem transition to an algae-dominated turbid state (Matsuzaki et al., 2007; Vilizzi et al., 2015). In contrast, despite some uncertainty regarding feeding habits, pond tadpoles appear to stabilize the ecosystem through consumption of algae and can be a meaningful component of ecosystem resistance to state shifts toward turbid regimes. As the period of larval anuran presence in the ponds is relatively short, cropping by tadpoles cannot prevent algal blooms later in the season. Larval amphibian populations are largely limited by fish; hence they are unlikely to buffer the effects of fish in permanent, fish-abundant lakes. However, they may be of importance in less permanent water bodies where fish occur only temporarily or at low densities. Under low fish predation pressure, despite the resulting high abundance of predatory insects, total biomass densities of tadpoles can exceed those of aquatic macroinvertebrates in ponds (Kloskowski, 2010, 2011a). Our results indicate that largely behaviorally mediated elimination of anuran larvae may provide a stage-structured cascade mechanism accompanying trophic top-down effects of fish. Anuran avoidance of ponds containing fish may facilitate algae via another pathway: tadpoles readily consume fecal material (Steinwascher, 1978; Waringer-Löschenkohl and Schagerl, 2001), and thus may also mitigate the stimulation of algae growth by nutrients from fish excreta. The present results are correlational, based on a natural experiment in which we could separate carp effects in otherwise near-natural conditions. While our system had the complexity of natural settings usually lacking in experimental research, more manipulative studies are required to examine whether amphibian larvae, if able to survive in significant numbers in the presence of fish, would slow down the ecosystem shift to an algae-dominated state. Further manipulative research should also attempt to elucidate the character and role of interactions between amphibian larvae and zooplanktonic grazers in phytoplankton regulation.
Data Availability Statement
The raw data supporting the conclusions of this article will be made available by the authors, without undue reservation.
Ethics Statement
The animal study was reviewed and approved by the Polish Ministry of the Environment.
Author Contributions
JK and MN collected the data. JK conceptualized the manuscript, analyzed the data, and wrote the manuscript with significant input from MN. Both authors approved the final version.
Funding
This project was funded by a grant from the Polish Ministry of Science and Higher Education (MNiSW 2 P04G05030).
Conflict of Interest
The authors declare that the research was conducted in the absence of any commercial or financial relationships that could be construed as a potential conflict of interest.
Publisher’s Note
All claims expressed in this article are solely those of the authors and do not necessarily represent those of their affiliated organizations, or those of the publisher, the editors and the reviewers. Any product that may be evaluated in this article, or claim that may be made by its manufacturer, is not guaranteed or endorsed by the publisher.
Acknowledgments
We are thankful to the pond owners for their cooperation and to the numerous students and volunteers who assisted in collecting field data. M. Adamczuk analyzed the tadpole gut contents.
References
Altig, R., Whiles, M. R., and Taylor, C. L. (2007). What do tadpoles really eat? Assessing the trophic status of an understudied and imperiled group of consumers in freshwater habitats. Freshw. Biol. 52, 386–395. doi: 10.1111/j.1365-2427.2006.01694.x
Arribas, R., Díaz-Paniagua, C., and Gomez-Mestre, I. (2014). Ecological consequences of amphibian larvae and their native and alien predators on the community structure of temporary ponds. Freshw. Biol. 59, 1996–2008. doi: 10.1111/fwb.12402
Bajer, P. G., Beck, M. W., Cross, T. K., Koch, J. D., Bartodziej, W. M., and Sorensen, P. W. (2016). Biological invasion by a benthivorous fish reduced the cover and species richness of aquatic plants in most lakes of a large North American ecoregion. Glob. Chang. Biol. 22, 3937–3947. doi: 10.1111/gcb.13377
Beckerman, A. P., Uriarte, M., and Schmitz, O. J. (1997). Experimental evidence for a behavior-mediated trophic cascade in a terrestrial food chain. Proc. Natl. Acad. Sci. U.S.A. 94, 10735–10738. doi: 10.1073/pnas.94.20.10735
Bottrell, H. H., Duncan, A., Gliwicz, Z. M., Grygierek, E., Herzig, A., Hillbricht-Ilkowska, A., et al. (1976). A review of some problem in zooplankton production studies. Nor. J. Zool. 24, 419–456. doi: 10.2166/wst.2012.862
Brönmark, C., Rundle, S. D., and Erlandsson, A. (1991). Interactions between freshwater snails and tadpoles: competition and facilitation. Oecologia 87, 8–18. doi: 10.1007/BF00323774
Brooks, J. L., and Dodson, S. I. (1965). Predation, body size, and composition of plankton. Science 150, 28–35. doi: 10.1126/science.150.3692.28
Buxton, V. L., and Sperry, J. H. (2017). Reproductive decisions in anurans: a review of how predation and competition affects the deposition of eggs and tadpoles. Bioscience 67, 26–38. doi: 10.1093/biosci/biw149
Cabana, G., and Rasmussen, J. B. (1996). Comparison of aquatic food chains using nitrogen isotopes. Proc. Natl. Acad. Sci. U.S.A. 93, 10844–10847. doi: 10.1073/pnas.93.20.10844
Crivelli, A. (1981). The biology of the common carp. Cyprinus carpio L. in the Camargue, southern France. J. Fish Biol. 18, 271–290.
Doi, H., Yurlova, N. I., Kikuchi, E., Shikano, S., Yadrenkina, E. N., Vodyanitskaya, S. N., et al. (2010). Stable isotopes indicate individual level trophic diversity in the freshwater gastropod Lymnaea stagnalis. J. Mollus. Stud. 76, 384–388. doi: 10.1093/mollus/eyq020
Dumont, H. J., Van de Velde, I., and Dumont, S. (1975). The dry weight estimate of biomass in a selection of Cladocera, Copepoda and Rotifera from the plankton, periphyton and benthos of continental waters. Oecologia 19, 75–97. doi: 10.1007/BF00377592
Fahimipour, A. K., Levin, D. A., and Anderson, K. E. (2019). Omnivory does not preclude strong trophic cascades. Ecosphere 10:e02800. doi: 10.1002/ecs2.2800
Fott, J., Pechar, L., and Pražáková, M. (1980). Fish as a factor controlling water quality in ponds. Dev. Hydrobiol. 2, 255–261.
Gliwicz, M. Z. (1986). Predation and the evolution of vertical migration in zooplankton. Nature 320, 746–748. doi: 10.1038/320746a0
Gosner, K. L. (1960). A simplified table for staging anuran embryos and larvae with notes on identification. Herpetologica 16, 183–190.
Hambright, K. D., and Hall, R. O. (1992). Differential zooplankton feeding behaviors, selectivities, and community impacts of two planktivorous fishes. Environ. Biol. Fish. 35, 401–411. doi: 10.1007/BF00004992
Hocking, D. J., and Babbitt, K. J. (2014). Amphibian contributions to eco-system services. Herpetol. Conserv. Biol. 9, 1–17. doi: 10.5479/si.00810282.242
Kenison, E. K., Litt, A. R., Pilliod, D. S., and McMahon, T. E. (2016). Role of habitat complexity in predator–prey dynamics between an introduced fish and larval Long-toed Salamanders (Ambystoma macrodactylum). Can. J. Zool. 94, 243–249. doi: 10.1139/cjz-2015-0160
Kenny, J. (1969). Feeding mechanisms in anuran larvae. J. Zool. 157, 225–246. doi: 10.1111/j.1469-7998.1969.tb01699.x
Khan, T. A. (2003). Dietary studies on exotic carp (Cyprinus carpio L.) from two lakes of western Victoria, Australia. Aquat. Sci. 65, 272–286. doi: 10.1007/s00027-003-0658-5
Kloskowski, J. (2010). Fish farms as amphibian habitats: factors affecting amphibian species richness and community structure at carp ponds in Poland. Environ. Conserv. 37, 187–194. doi: 10.1017/S0376892910000494
Kloskowski, J. (2011b). Differential effects of age-structured common carp (Cyprinus carpio) stocks on pond invertebrate communities: implications for recreational and wildlife use of farm ponds. Aquacult. Int. 19, 1151–1164. doi: 10.1007/s10499-011-9435-y
Kloskowski, J. (2011c). Impact of common carp Cyprinus carpio on aquatic communities: direct trophic effects versus habitat deterioration. Fund. Appl. Limnol. 178, 245–255. doi: 10.1127/1863-9135/2011/0178-0245
Kloskowski, J. (2011a). Consequences of the size structure of fish populations for their effects on a generalist avian predator. Oecologia 166, 517–530. doi: 10.1007/s00442-010-1862-3
Kloskowski, J. (2020). Better desiccated than eaten by fish: distribution of anurans among habitats with different risks to offspring. Freshw. Biol. 65, 2124–2134. doi: 10.1111/fwb.13608
Kloskowski, J., Nieoczym, M., and Stryjecki, R. (2020). Between-habitat distributions of pond tadpoles and their insect predators in response to fish presence. Hydrobiologia 847, 1343–1356. doi: 10.1007/s10750-020-04190-5
Kloskowski, J., and Trembaczowski, A. (2015). Fish reduce habitat coupling by a waterbird: evidence from combined stable isotope and conventional dietary approaches. Aquat. Ecol. 49, 21–31. doi: 10.1007/s10452-015-9501-z
Kupferberg, S. (1997). Facilitation of periphyton production by tadpole grazing: functional differences between species. Freshw. Biol. 37, 427–439. doi: 10.1046/j.1365-2427.1997.00170.x
Lauridsen, T. L., and Buenk, I. (1996). Diel changes in the horizontal distribution of zooplankton in the littoral zone of two shallow eutrophic lakes. Arch. Hydrobiol. 137, 161–176. doi: 10.1127/archiv-hydrobiol/137/1996/161
Lechelt, J. D., and Bajer, P. G. (2016). Elucidating the mechanism underlying the productivity-recruitment hypothesis in the invasive common carp. Aquat. Invasions 11, 469–482. doi: 10.3391/ai.2016.11.4.11
Lima, S. L. (1998). Nonlethal effects in the ecology of predator-prey interactions. Bioscience 48, 25–34. doi: 10.2307/1313225
Matsuzaki, S. S., Usio, N., Takamura, N., and Washitani, I. (2007). Effects of common carp on nutrient dynamics and littoral community composition: roles of excretion and bioturbation. Fund. Appl. Limnol. 168, 27–38. doi: 10.1127/1863-9135/2007/0168-0027
McIntosh, A. R., and Townsend, C. R. (1996). Interactions between fish, grazing invertebrates and algae in a New Zealand stream: a trophic cascade mediated by fish-induced changes to grazer behaviour? Oecologia 108, 174–181. doi: 10.1007/BF00333229
Minagawa, M., and Wada, E. (1984). Stepwise enrichment of 15N along food chains: further evidence and the relation between δ15N and animal age. Geochim. Cosmochim. Acta 48, 1135–1140. doi: 10.1016/0016-7037(84)90204-7
Nieoczym, M., and Kloskowski, J. (2014). The role of body size in the impact of common carp Cyprinus carpio on water quality, zooplankton, and macrobenthos in ponds. Int. Rev. Hydrobiol. 99, 212–221. doi: 10.1002/iroh.201301644
Nieoczym, M., Mencfel, R., Gorzel, M., and Kloskowski, J. (2020). Reduced abundance but increased diversity of chironomid larvae under higher trophic pressure from fish in semi-permanent ponds. Limnologica 82:125778. doi: 10.1016/j.limno.2020.125778
Okun, N., Brasil, J., Attayde, J. L., and Costa, I. A. (2008). Omnivory does not prevent trophic cascades in pelagic food webs. Freshw. Biol. 53, 129–138. doi: 10.1111/j.1365-2427.2007.01872.x
Pace, M. L. (1984). Zooplankton community structure, but not biomass, influences the phosphorus–chlorophyll a relationship. Can. J. Fish. Aquat. Sci. 41, 1089–1096. doi: 10.1139/f84-128
Parkos, J. J. III, Santucci, J., Victor, J., and Wahl, D. H. (2003). Effects of adult common carp (Cyprinus carpio) on multiple trophic levels in shallow mesocosms. Can. J. Fish. Aquat. Sci. 60, 182–192. doi: 10.1139/f03-011
Petranka, J. W., and Kennedy, C. A. (1999). Pond tadpoles with generalized morphology: Is it time to reconsider their functional roles in aquatic communities? Oecologia 120, 621–631. doi: 10.1007/s004420050898
Polis, G. A., and Holt, R. D. (1992). Intraguild predation: the dynamics of complex trophic interactions. Trends Ecol. Evol. 7, 151–154. doi: 10.1016/0169-5347(92)90208-S
Polis, G. A., and Strong, D. R. (1996). Food web complexity and community dynamics. Am. Nat. 147, 813–846. doi: 10.1086/285880
Post, D. M. (2002). Using stable isotopes to estimate trophic position: models, methods, and assumptions. Ecology 83, 703–718. doi: 10.1111/j.1095-8649.2012.03251.x
Potužák, J., Hùda, J., and Pechar, L. (2007). Changes in fish production effectivity in eutrophic fishponds—impact of zooplankton structure. Aquacult. Int. 15, 201–210. doi: 10.1007/s10499-007-9085-2
Preisser, E. L., Bolnick, D. I., and Benard, M. F. (2005). Scared to death? The effects of intimidation and consumption in predator–prey interactions. Ecology 86, 501–509. doi: 10.1890/04-0719
Pryor, G. S. (2003). Growth rates and digestive abilities of bullfrog tadpoles (Rana catesbeiana) fed algal diets. J. Herpetol. 37, 560–566. doi: 10.1670/153-02n
Qin, J., and Threlkeld, S. T. (1990). Experimental comparison of the effects of benthivorous fish and planktivorous fish on plankton community structure. Arch. Hydrobiol. 119, 121–141.
Resetarits, W. J. (1996). Oviposition site choice and life history evolution. Am. Zool. 36, 205–215. doi: 10.1093/icb/36.2.205
Resetarits, W. J., Rieger, J. F., and Binckley, C. A. (2004). Threat of predation negates density effects in larval gray treefrogs. Oecologia 138, 532–538. doi: 10.1007/s00442-003-1466-2
Richardson, W. B., Wickham, S., and Threlkeld, S. (1990). Foodweb response to the experimental manipulation of a benthivore (Cyprinus carpio), zooplanktivore (Menidia beryllina) and benthic insects. Arch. Hydrobiol. 119, 143–165.
Romare, P., and Hansson, L.-A. (2003). A behavioral cascade: top-predator induced behavioral shifts in planktivorous fish and zooplankton. Limnol. Oceanogr. 48, 1956–1964. doi: 10.4319/lo.2003.48.5.1956
Savage, R. M. (1952). Ecological, physiological and anatomical observations on some species of anuran tadpoles. Proc. Zool. Soc. Lond. 122, 467–514. doi: 10.1111/j.1096-3642.1952.tb00322.x
Schiesari, L., Werner, E. E., and Kling, G. W. (2009). Carnivory and resource-based niche differentiation in anuran larvae: implications for food web and experimental ecology. Freshw. Biol. 54, 572–586. doi: 10.1111/j.1365-2427.2008.02134.x
Schmitz, O. J., Krivan, V., and Ovadia, O. (2004). Trophic cascades: the primacy of trait-mediated indirect interactions. Ecol. Lett. 7, 153–163. doi: 10.1111/j.1461-0248.2003.00560.x
Schoeninger, M. J., and DeNiro, M. J. (1984). Nitrogen and carbon isotopic composition of bone collagen from marine and terrestrial animals. Geochim. Cosmochim. Acta 48, 625–639. doi: 10.1016/0016-7037(84)90091-7
Seale, D. B., and Beckvar, N. (1980). The Comparative Ability of Anuran Larvae (Genera: Hyla. Bufo and Rana) to Ingest Suspended Blue-Green Algae. Copeia 1980, 495–503. doi: 10.2307/1444527
Shen, R., Gu, X., Chen, H., Mao, Z., Zeng, Q., and Jeppesen, E. (2021). Silver carp (Hypophthalmichthys molitrix) stocking promotes phytoplankton growth by suppression of zooplankton rather than through nutrient recycling: an outdoor mesocosm study. Freshw. Biol. 66, 1074–1088. doi: 10.1111/fwb.13700
Sibbing, F. A. (1988). Specializations and limitations in the utilization of food resources by the carp, Cyprinus carpio: a study of oral food processing. Environ. Biol. Fish. 22, 161–178. doi: 10.1007/BF00005379
Šigutová, H., Harabiš, F., Šigut, M., Vojar, J., Choleva, L., and Dolný, A. (2021). Specialization directs habitat selection responses to a top predator in semiaquatic but not aquatic taxa. Sci. Rep. 11:18928. doi: 10.1038/s41598-021-98632-2
Sinsch, U., Schneider, H., and Tarkhnishvili, D. N. (2009). “Bufo bufo Superspezies – Erdkröten – Artenkreis,” in Handbuch der Reptilien und Amphibiens Europas, Band 5/II, ed. K. Grossenbacher (Wiebelsheim: Aula Verlag), 191–335.
Steinwascher, K. (1978). Interference and exploitation competition among tadpoles of Rana utricularia. Ecology 59, 1039–1046. doi: 10.2307/1938556
Vanni, M. J., and Layne, C. D. (1997). Nutrient recycling and herbivory as mechanisms in the “top–down” effect of fish on algae in lakes. Ecology 78, 21–40. doi: 10.2307/2265976
Vilizzi, L., Tarkan, A. S., and Copp, G. H. (2015). Experimental Evidence from Causal Criteria Analysis for the Effects of Common Carp Cyprinus carpio on Freshwater Ecosystems: a Global Perspective. Rev. Fish. Sci. Aquacult. 23, 253–290. doi: 10.1080/23308249.2015.1051214
Vonesh, J. R., Kraus, J. M., Rosenberg, J. S., and Chase, J. M. (2009). Predator effects on aquatic community assembly: disentangling the roles of habitat selection and post-colonization processes. Oikos 118, 1219–1229. doi: 10.1111/j.1600-0706.2009.17369.x
Wahl, D. H., Wolfe, M. D., Santucci, V. J., and Freedman, J. A. (2011). Invasive carp and prey community composition disrupt trophic cascades in eutrophic ponds. Hydrobiologia 678, 49–63. doi: 10.1007/s10750-011-0820-3
Waringer-Löschenkohl, A., and Schagerl, M. (2001). Algal Exploitation by Tadpoles — an Experimental Approach. Int. Rev. Hydrobiol. 86, 105–125. doi: 10.1002/1522-2632(200101)86:1<105::aid-iroh105>3.0.co;2-v
Weber, M. J., and Brown, M. L. (2009). Effects of common carp on aquatic ecosystems 80 years after “carp as a dominant”: ecological insights for fisheries management. Rev. Fish. Sci. 17, 524–537. doi: 10.1080/10641260903189243
Wellborn, G. A., Skelly, D. K., and Werner, E. E. (1996). Mechanisms creating community structure across a freshwater habitat gradient. Annu. Rev. Ecol. Syst. 27, 337–363. doi: 10.1146/annurev.ecolsys.27.1.337
Werner, E. E., and Peacor, S. D. (2003). A review of trait-mediated indirect interactions in ecological communities. Ecology 84, 1083–1100. doi: 10.1890/0012-9658(2003)084[1083:arotii]2.0.co;2
Wesner, J. (2019). Using stage-structured food webs to assess the effects of contaminants and predators on aquatic–terrestrial linkages. Freshw. Sci. 38, 928–935. doi: 10.1086/706103
Williams, A. E., Moss, B., and Eaton, J. (2002). Fish induced macrophyte loss in a shallow lakes: top-down and bottom up processes in mesocosm experiments. Freshw. Biol. 47, 2216–2232. doi: 10.1046/j.1365-2427.2002.00963.x
Keywords: amphibian larvae, behaviorally mediated trophic cascades, fear ecology, predator-prey interactions, stable isotopes
Citation: Kloskowski J and Nieoczym M (2022) Strong Behavioral Effects of Omnivorous Fish on Amphibian Oviposition Habitat Selection: Potential Consequences for Ecosystem Shifts. Front. Ecol. Evol. 10:856258. doi: 10.3389/fevo.2022.856258
Received: 17 January 2022; Accepted: 21 March 2022;
Published: 13 April 2022.
Edited by:
Lawrence Hurd, Washington and Lee University, United StatesReviewed by:
Matthew Pintar, Florida International University, United StatesDavid Boukal, University of South Bohemia in České Budějovice, Czechia
Copyright © 2022 Kloskowski and Nieoczym. This is an open-access article distributed under the terms of the Creative Commons Attribution License (CC BY). The use, distribution or reproduction in other forums is permitted, provided the original author(s) and the copyright owner(s) are credited and that the original publication in this journal is cited, in accordance with accepted academic practice. No use, distribution or reproduction is permitted which does not comply with these terms.
*Correspondence: Janusz Kloskowski, janusz6kl@gmail.com