- 1Department of Neurobiology, Physiology and Behavior, University of California, Davis, Davis, CA, United States
- 2Department of Fisheries, Wildlife, and Conservation Science, Oregon State University, Corvallis, OR, United States
- 3Department of Integrative Biology, Oregon State University, Corvallis, OR, United States
Regulation of energetic expenditure in a changing environment, considered here as allostatic load, is central to organism-environment interactions. The value of responses that modify behavior or physiology in coping strategies is often measured in terms of energetic benefits. In this study, the total energetic cost incurred by Gambel’s white-crowned sparrows, Zonotrichia leucophrys gambelii, was assessed using heart-rate transmitters. The use of heart rate was validated as a proxy for metabolic rate via flow-through respirometry. Applying heart rate as an indicator of allostatic load, we confirmed that ambient temperature under wintering conditions influences allostatic load. However, baseline corticosterone, proposed to mediate physiological responses to variation in allostatic load, does not appear to vary with heart rate or temperature in captivity, or with temperature under ambient conditions in the field. The relationship between allostatic load and plasma corticosterone levels was also investigated by manipulating feeding effort for captive Gambel’s white-crowned sparrows using a sand-excavation challenge that approximated a type of foraging work that these birds normally perform in the wild. This experiment was designed to test the hypothesis that experimentally increased allostatic load induces elevation in baseline corticosteroids. We did not find support for this hypothesis. We suggest that the adrenocortical response to increased allostatic load may be limited to overload or environmental conditions that meaningfully threaten energy imbalance, indicating new targets for further research.
Introduction
The model of allostatic load outlined by McEwen and Wingfield (2003) emphasizes variation in energetic demand as a key driving force underlying glucocorticoid regulation of physiological and behavioral adaptations to a changing environment. Allostatic load, in this model, refers to the cumulative cost of allostasis, maintaining stability through change. Energy expenditure varies both predictably and unpredictably over the life of an animal. It is important for an individual to maintain neutral energy balance whenever possible despite fluctuations in demand, resources available and body condition (e.g., Broggi et al., 2019). Environmental factors are expected to be a primary source of variation in energy requirements, particularly in wintering animals (Wingfield and Ramenofsky, 2011; Broggi et al., 2019), when costs and trade-offs associated with breeding, molt, and migration are absent. When environmental factors create a psychological or physiological perturbation, it can activate the emergency life-history stage (ELHS, Wingfield et al., 1998), which consists of a series of behavioral and physiological responses, regulated primarily by glucocorticoids, that mobilize resources and promote individual survival at the expense of other life-history strategies (Romero and Wingfield, 2016). The observation of organismal responses to fluctuations in winter weather offers an excellent opportunity to examine the overall energy demands an individual experiences at any moment that is a function of resources available in the environment, body condition, social status, etc. (Korte et al., 2005).
The model of allostatic load (McEwen and Wingfield, 2003) makes two independent assumptions that may be drawn upon for their testable predictions and alternatives. Assumption 1 states that costly events cause allostatic load to increase, at least up to a threshold for ELHS activation, which triggers compensatory mechanisms to reduce allostatic load. Alternatively, costly events could directly result in minor compensatory changes that reduce existence energy or routine energetic expenditures (e.g., by lower body temperature or reduced non-feeding activity) that keep allostatic load stable until such options are exhausted. This alternative would maximize the perturbation resistance potential (PRP), i.e., the difference between allostatic load and the cumulative resources available internally and in the environment (Wingfield et al., 2011, 2017).
Assumption 2 states that increased allostatic load is associated with elevation in circulating baseline glucocorticoid levels, at least up to a threshold for the ELHS.
Alternatively, if allostatic load changes (Assumption 1), plasma glucocorticoids may not change in parallel. Because the role of glucocorticoids during the ELHS transition is well established, sudden sustained or repeated increases may trigger the ELHS only when allostatic load approaches the limit of energy availability rather than gradually rising to a threshold. This alternative would link glucocorticoid action to PRP as opposed to allostatic load.
Heart rate is a common proxy for estimating metabolic rate in the field (reviewed in Butler et al., 2004; Green, 2011). While, heart rate (fH) and oxygen consumption are generally correlated, unpredicted changes in stroke volume and/or oxygen extraction can result in estimation errors (particularly when one is used to predict the other). Although fH and oxygen consumption are related, the consistency of this relationship is unclear. Relationships have been noted within individuals across seasons, as well as across individuals and species. Variation in the relationship can be related to body mass (BM; Green, 2011), but allometric scaling does not always account for observed differences (e.g., Portugal et al., 2009). The selection of appropriate scaling indices is, in itself, problematic (Packard and Boardman, 1999). In mammals, heart rate typically scales with mass (BM–0.26) meaning fH decreases as BM increases (White and Kearney, 2014). On the other hand, mammalian V̇O2, volume of oxygen consumed per unit time, increases with mass (BM0.71) though the allometric scaling relationship of V̇O2 can vary with circumstance (White and Kearney, 2014). Procedures for handling scaling are diverse, including modeling body mass as a covariate, calculating mass exponents using a subset of data taken under constant conditions, or trying a range of possible mass exponents and selecting a value that explains the most variability.
Here we first performed experiments on captive, adult Gambel’s white-crowned sparrows (Zonotrichia leucophrys gambelii) to validate the use of heart rate telemetry as a proxy for energy expenditure through simultaneous respirometry to evaluate the extent that these approaches reliably co-varied. We then evaluated intra- and inter-individual variations in fH and explored a role for body mass in explaining this variability. Next, we employed this technique and others to investigate McEwen and Wingfield’s (2003) model of allostatic load.
To evaluate Assumption 1, we determined whether typical fluctuations in outdoor temperatures during winter in Davis, California influenced allostatic load among captive and free-living birds. Ambient temperatures were usually below the laboratory-determined thermoneutral zone for this species (King, 1964). Allostatic load, in this case, was averaged over a 24-h period, and measured using fH. Real-time heart rate data has the advantage of offering insight into how ambient temperature influenced fH and to what extent compensation occurred within a 24-h average. Free-living birds experienced complex meteorological events and exploited microhabitat variation whereas captive birds were housed in sheltered outdoor aviaries with limited exposure to extraneous variables (e.g., wind) but experienced natural variation in ambient temperature and humidity. To evaluate Assumption 2, we compared heart rate telemetry data to measurements of baseline plasma corticosterone (the major glucocorticoid in birds). We also examined ambient temperature for relationships with baseline corticosterone on the basis of Assumption 1.
In the second part of this study, we experimentally manipulated energy expenditure to increase allostatic load to test for its impact on baseline plasma levels of corticosterone independent of ambient temperature thereby further evaluating Assumption 2. Energy expenditure has been manipulated in several species in captivity. Techniques previously applied to adult birds include perch removal, lever pressing, weight-carrying and motion-sensing feeders with pre-programmed frequency or probability of food release used to manipulate the effort required for food acquisition (Bautista et al., 1998; Wiersma et al., 2005; Johns et al., 2018). Physiological costs associated with normal seasonal changes of life-history stages, e.g., egg laying, have also been examined (Vézina et al., 2006). However, energetic demand associated with physiological change is problematic as a mode of “manipulation” because costs may change concurrently with a suite of allostatic shifts across life-history stage transitions potentially including changes at multiple levels of the hypothalamic-pituitary-adrenal (HPA) axis. For example, glucocorticoid receptor expression can change seasonally, which complicates interpretation of concurrent hormonal shifts (Lattin and Romero, 2014; Krause et al., 2015; Wingfield, 2018). Methods that involve manipulation of effort within season offer superior control of internal confounds. However, the unrealistic nature of challenges like perch removal or unpredictable food release, relative to a songbird’s evolutionary history and life experience, may confound an energetic manipulation with a cognitive cue that may act as a classical “stressor.” This is particularly important in teasing apart the predictions of allostatic load from our understanding of the classical stress response.
The aim here was to experimentally increase workload for captive Gambel’s white-crowned sparrows to mimic variation in foraging effort required in the field. Substrate-scratching is a mode of foraging behavior described in a variety of songbirds (Greenlaw, 1977). A study of free-living New World sparrows showed that increasing the amount of litter on top of food increases the frequency of engagement in prolonged feeding bouts, as measured by number of “scratches” per bout in those areas (Hailman, 1984).
Animals may also compensate for increased workload by diminishing energetic investment in other activities or functions. This issue is minimized in a captive setting where other energetic demands are absent. Heart rate telemetry could not be used in the second set of experiments due to problems using our transmitters inside small cages. Therefore, the verification of Assumption 2 relies heavily on the validity of Assumption 1, i.e., experimental workload can be applied as a direct proxy for allostatic load. To probe the validity of Assumption 1 in this context, we collected and analyzed 24 h video recordings to determine whether patterns of daytime energy expenditure changed as a result of foraging workload manipulation. We also measured food consumption and change in body mass as further indicators of energetic status. To evaluate Assumption 2, we tested baseline plasma corticosterone levels during a manipulated workload regime and with respect to activity level and consumption metrics within and across treatment regimes. Exploration of these outcomes has implications for understanding not only relationships between allostatic load and corticosterone, but also for individual variation.
Materials and Methods
General Methods Applied Across Studies
Bird Capture and Housing
Gambel’s white-crowned sparrows (hereafter, GWCS; Z. l. gambelii) winter in large numbers at our study sites in and around Davis, CA, United States (38.5°N, 121.8°W, Supplementary Figure 1, Chilton et al., 1995). We captured all birds in the winter months (November to February) using mist nets or Potter traps that were pre-baited with seeds (under guidelines of the State of California and USFWS collecting permits). All procedures and housing of captive birds were approved by the University of California Davis IACUC. For each bird, we measured morphological characteristics including length of the tarsus, beak (nare to tip) and flattened wing chord to the nearest 0.1 mm using calipers. We weighed birds (±0.1 g) using a Pesola scale.
All GWCS were initially housed in flight cages (1.8 m × 1 m × 1.8 m), typically within an outdoor aviary at UC Davis. The aviary was semi-enclosed, open to the outside air and naturally lit, but was sheltered from rain and prevailing winds by a roof and concrete walls on two sides. While in flight cages, birds were fed a diet consisting of Mazuri small bird maintenance feed (PMI Nutrition), wild bird seed, and vitamin-enriched grit ab libitum. We provided water in bottles as well as in cups with the latter mostly used by the birds for bathing. Birds were allowed to acclimate to captivity for at least 3 weeks prior to the initiation of experiments or transfer to individual cages (Wingfield et al., 1982).
Blood Sampling
We collected a small blood sample by puncturing the alar vein within 3 min of capture using a 26-gauge needle. We collected blood into heparin-coated microhematocrit tubes. Blood samples were briefly stored on ice, then centrifuged at approximately 170 G for 5 min. Plasma was aspirated and stored at −35°C until used for hormone assays. Baseline corticosterone samples from wintering GWCS were collected at multiple sites in Davis, CA between 2007 and 2014.
Corticosterone Assay
Circulating corticosterone (ng/mL) was measured by radioimmunoassay following Wingfield et al. (1992). Briefly, plasma was added in volumes ranging from 5 to 30 μL in combination with distilled water to achieve a consistent volume of 200 μL. 2,000 CPM of tritiated corticosterone (Perkin Elmer NET399250UC) was added to each sample to evaluate extraction efficiency of individual samples. After overnight equilibration, steroids were extracted with 4 mL of freshly re-distilled dichloromethane for 2 h. The organic phase was aspirated and dried under a stream of nitrogen gas in a water bath at 35°C, then reconstituted in 550 μL of phosphate-buffered saline with gelatin (PBSG). A 100 μL aliquot was removed and scintillation fluid added (Perkin Elmer Ultima Gold: 6013329) to evaluate percent recovery of the labeled hormone for each sample. Duplicate 200 μL aliquots were equilibrated overnight at 4°C with 100 μL (approx. 10,000 CPM) of tritiated corticosterone and 100 μL of antibody (100× dilution of Esoterix Inc. B3-163). Unbound steroid was stripped from solution by adding 500 μL of dextran-coated charcoal for 10 min at 4°C, followed by refrigerated centrifugation at 3000 RPM. Supernatant (containing antibody-bound hormone) was decanted and vortexed with scintillation fluid before counting for 10 min or within 2% accuracy on a Beckman 6500 liquid scintillation counter. Each assay included two solvent blanks and a standard sample (1,000 pg of unlabeled corticosterone). The mean recovery efficiency was 87.4% and coefficients of inter- and intra-assay variation were 16.1 and 12.9%, respectively. The mean lower detection limit was 18.05 pg/tube.
Heart Rate Telemetry and Signal Processing
SP2000 heart rate transmitters (Sparrow Systems, IL, United States) were attached using a protocol modified from Raim (1978). Each transmitter was attached to a small piece of cotton cloth (approximately 1.5 cm × 2.5 cm). Birds were briefly immobilized by isoflurane anesthesia. A small patch of contour feathers was trimmed (captive birds) or plucked (necessary for superior adhesion in field studies) from the dorsum, and two sterilized electrocardiogram (EKG) electrodes were inserted subcutaneously (3–4 mm) via two small incisions (1 mm) lateral to the dorsal midline and just posterior to the scapula. Incisions were closed with surgical adhesive and treated with lidocaine. The cloth holding the transmitter was glued to the dorsum using latex eyelash adhesive (Duo brand), with the remaining length of the electrodes tucked under the cloth.
Heart rate signals were received using an AR8200 receiver (AOR Ltd., Tokyo, JP) with a telescopic whip (captive) or handheld Yagi (field) antenna. Audio output was recorded to an R09-HR recorder in MP3 format or, in the case of validation studies, directly to disk in WAV format using Audition software (Adobe Systems, Inc., CA, United States).
Heart rate data were extracted and filtered from audio files using Vireo software, two scripts written for Scilab (Versailles, France) by Kelley and Bisson (2009), with modifications to accommodate a range of heart rate and noise characteristics specific to each experiment. Extraction and filtration were spot-checked manually by removing the carrier frequency using an FFT filter in Adobe Audition software (Adobe Systems, Inc., CA, United States) and hand-counting peaks associated with heart beats. Data were extracted at a resolution of 1 datum point per second, then averaged over 5-min periods to reduce autocorrelation and accommodate any time offsets relative to V̇O2.
Validation of Heart Rate as a Proxy for Allostatic Load
Respirometry
Rates of O2 consumption () of 7 captive adult GWCS (mean mass ± SEM: 30.18 ± 0.29 g; see Supplementary Tables 1, 2 for statistical analyses of mensural characters) were measured using open-flow respirometry. Room air was drawn at a rate of 900 mL/min from the bottom of a clear acrylic chamber (6L volume) fully air-tight except for an in-flow port at the top of the chamber (Supplementary Figure 2).
The respirometry chamber was equipped with food, water and a perch, just large enough for a bird to extend its wings, though too small to permit flight. A 6-cm fan was installed in the lid to prevent formation of dead spaces around the objects in the chamber. Pump, flow control, oxygen and carbon dioxide meters were included in a Foxbox (Sable Systems, Las Vegas, NV, United States). Water and CO2 were removed from air prior to oxygen measurement using a laboratory gas drying unit (2 5/8 × 11 3/8, Hammond Drierite Co., OH, United States) and soda lime in a smaller (3/5 × 8″) column. To correct for drift in the instruments, a multiplex unit was engaged for automatic base-lining with room air for 1-min every 10-min. The chamber was placed in the room where birds were normally housed, and each subject was able to view conspecifics throughout. All other equipment was in an adjacent room, which allowed the experimenter to remain hidden from the subject’s view during testing. Temperature in the bird room and adjacent to the Foxbox was measured using Sable thermistor probes connected to a universal interface (UI-2, Sable Systems). Respirometry data from the Foxbox were imported to a PC running Expedata software (v. 1.2.5, Sable Systems, Las Vegas, NV, United States). Oxygen measurements were corrected to STP (standard temperature and pressure) which were adjusted to baseline. Instantaneous V̇O2 was calculated using the manufacturer’s recommended procedures for the Expedata software. Data were averaged over 5 min periods to align with heart rate.
We validated the stability of measurements in the apparatus using nitrogen injection (Lighton, 2008). Briefly, a known rate of oxygen “consumption” is generated via displacement of oxygen using a small known flow-rate of pure nitrogen gas added to the air intake. This known rate of change is then compared with instantaneous V̇O2 calculated based on oxygen measurement to verify that the respirometry chamber is not a source of error.
Heart Rate Telemetry, Validation Protocol
After we attached the heart rate transmitters, birds were returned to their cages and allowed to recover from anesthesia for at least 1 h. They were then placed in the respirometry chamber and allowed to settle while the respirometry system equilibrated. Validations began immediately upon placing the bird in the chamber and continued for a period of 2 h. To obtain maximal heart rate elevation birds were intermittently disturbed by the experimenter entering the room and briefly tapping on the chamber. All experiments were conducted at the end of the day (15:00–17:00). Overhead fluorescent lights were turned off 20 min prior to the end of each trial to allow the bird to settle down for the night. This range of activity revealed heart rates, approximately 440–900 beats per minute (BPM), which were nearly comparable to that of a free-living bird. While frequent technical challenges limited the validation period to 2 h there were opportunities to collect additional data over the course of the one or more days spent in the respirometry chamber for several birds. These data were examined to evaluate intra-individual variability and possible sources of error.
Validation Comparison: Selection and Conversion of Existing Data
To compare our fH-V̇O2 validation data with existing data from other species, we performed a literature search to extract data for visual meta-analysis. These data included juvenile and adult birds from different species. Where findings did not support a single relationship across individuals (e.g., Gessaman, 1980), we selected individual relationships with minimum and maximum slopes separately for comparison. Because body mass varied widely across the data set, V̇O2 was converted to reflect units of ml/min/kg0.703 (Tieleman and Williams, 2000) and heart rate was converted to BPM/kg–0.26 (White and Kearney, 2014).
Measurement of Allostatic Load via Heart Rate Telemetry
Aviary Telemetry
Adult GWCS were captured during February of 2011 and 2012, processed and acclimated as described above. In the aviary, ambient temperature (°C) was monitored continuously using two thermistors taped to the walls of the cage that recorded to a PC running Expedata software (Sable Systems, Las Vegas, NV, United States). We used an average of the temperature readings (°C) from these two thermistors in our analyses. Transmitters were attached to 5 birds for each of three trial periods. The AR8200 receiver was programmed to cycle through each of the 5 transmitter frequencies at a rate of 1 channel per minute. Signals for each individual were recorded for 1 min out of every 5-min period. Heart rate was recorded over a period of 7 days or until the transmitters failed, after which they were promptly removed from the birds. Audio files were split into 1 min segments for analysis. Within each minute, the first and last 10-s were eliminated to prevent error due to channel switching. Heart rates were averaged over 30-min periods (6 × 1-min samples for each of the 5 birds) to reduce autocorrelation and minimize the influence of brief disturbances.
Heart rate was measured for 5 birds starting on March 18, 2011 (Trial 1), then for 5 different birds starting on April 2, 2011 (Trial 2). Between these two trials, a substantial and enduring spring warming occurred, such that the thermal profile for the second trial was dramatically different from the first. To investigate the occurrence of intra-individual variation in response to different temperature regimes, the birds from Trial 1 were followed again for Trial 3, starting on April 22, 2011. However, due to transmitter failures, replicate data from Trial 1 and Trial 3 were only successfully obtained from one individual, Bird #130. Total numbers of birds with successful heart rate data were n = 3 for Trial 1, n = 4 for Trial 2, and n = 3 for Trial 3, with the latter including Bird #130.
Field Telemetry
Adult GWCS were captured in Davis, CA (Supplementary Figure 1) during the spring (January – March; n = 10) and autumn (October – November; n = 9) of 2012. During the spring, birds were primarily captured at sites A, C, and F while in fall most birds were captured at site B (Supplementary Figure 1). We captured birds between 0700 and 1100AM. Birds were banded with an aluminum USFWS band and a unique set of color bands for later visual identification. Heart rate transmitters were attached as above on the day of capture. After birds recovered from the effects of anesthesia, they were held overnight for monitoring in a flight cage within the aviary. We then released each bird early the following morning at the site of their capture. Following the trials, some transmitters were removed at recapture and some of them fell off and were retrieved from the field.
Individual birds were followed in the field as continuously as possible and as closely as necessary to maintain optimal signal strength. In open terrain, the quality of the signal degraded at a distance of ∼30 m, with significant reductions in operable distance associated with heavy shrubs or ravines. Thus, it was not always possible to collect data at a distance sufficient to avoid disturbance of the flock by researchers. In these cases, the equipment was attached to a tripod or other available structure and were checked every 1–3 h to ensure that the bird was still present and that the equipment batteries were still functional. For overnight recording, the equipment was left attached to an automotive backup battery, packed inside a rain-proof sack with the antenna clamped to a tripod or fixed structure. Each bird was followed for at least 2 full days after release. In the rain, transmitter batteries became waterlogged and ceased functioning for periods of hours to days. This failure sometimes also occurred when birds bathed under drip-irrigation devices that were present in the vineyards that birds frequented. Occasionally, a bird’s signal was lost, usually after fleeing a disturbance, and could not be relocated for an extended period. Some transmitter frequencies were vulnerable to interference from unknown local sources such as intermittent static or total disruption. Finally, transmitters remained functional for variable lengths of time before failing and/or falling off.
Meteorological Data
Hourly data for statistical analyses were drawn from a weather station (38.536°N, 121.775°W) maintained by UC Davis that collects data for the California Irrigation Management Information System, Mesowest ID# CI0061. This station is located approximately 1 km north of our field site at an elevation of 18.3-m.
Heart Rate Signal Processing
Heart rate data were extracted from audio files as described above. Because signal quality was more variable for field data, heart rates were averaged over 1-min periods, retaining only bins for which more than 10 s of data were present after extracting data as previously described. One-minute bins were averaged over 60 min, at which point heart rate data were aligned with meteorological data. Hourly heart rate and temperature were averaged to obtain daily average heart rate (BPM). Averaging hourly bins, as opposed to 1-min bins, ensured that times of day in which the signal was noisiest (e.g., periods of active foraging) and therefore contained fewer 1-min data points (due to filtering) were not underrepresented in the average. Thus, all hourly bins were weighted equally regardless of the number of 1-min data points they contained.
Daily averages were defined as the 24-h period before and after sunrise. 24-h periods with <16 h worth of binned data were excluded from analysis. Because temperature was aligned with heart rate prior to averaging, only temperature data for periods with valid heart rate data were included in averages when evaluating relationships between these two variables.
Manipulation of Foraging Effort
Gambel’s white-crowned sparrows were acclimated to captivity as described above, then transferred indoors to individual cages (35 cm × 40 cm × 45 cm) and kept on a continuous short-day light cycle (8L:16D) at 21–23°C. Short day lengths were chosen so all birds, whether housed indoors or exposed to natural day length were on similar light/dark schedules. Mazuri food was distributed ab libitum and was split evenly between two circular trays on the floor of the cage (6-in × 1-in plastic pot saucers) that could accommodate a variety of substrates in addition to food (Supplementary Figure 3). Grit and water cups as well as a water bottle were installed in the cage walls; wild bird seed was removed from their diet. Each bird was housed in its home cage for all training and trials but were moved into a separate, adjacent room (hereafter, the trial room) for training and trials. In the trial room, all cages were arranged on two shelves, each holding two cages, which were thoroughly and evenly lit by fluorescent light fixtures positioned on the wall and ceiling. An array of 4 closed circuit television (CCTV) cameras was positioned to observe and record each bird’s behavior. The room was illuminated during dark hours by a single low-intensity LED nightlight (Limelight Nightlight, Austin Innovations, TX, United States). Cameras were also equipped with infrared LEDs that gave off some visible red light.
Trial Substrate and Training
We used natural white aquarium sand (Petco, CA, United States) for our testing medium because it was difficult but not impossible for birds to displace, and the food could be easily extracted from it at the end of the experiment to determine how much had been consumed. In this way we tested for the energetic cost of self-maintenance by increasing foraging effort. Birds accessed buried food with increasing amounts of aquarium sand in their food trays.
To train birds, we initially provided individuals with trays where food was fully visible. No sand was present initially during training, but several stones of natural white aquarium gravel were used to stabilize the tray and prevent spillage. The food was spread evenly over the bottom of the tray. Sand was then added daily in small, incremental amounts to the trays with a few pieces of food remaining visible to provide birds a visual cue. Trays were changed daily at which point remaining food was weighed to verify that all birds were eating. We also recorded videos to confirm that birds were successfully finding their food and not simply displacing it from the tray. At the end of the training, all birds had learned to search for fully buried food through a tray of sand that was approximately 2 cm deep. Following completion of the training, birds were returned to the general housing room and continued to receive their food in the same trays mixed with small amounts of sand and gravel for the duration of the study.
Manipulation Experiments
Birds were moved in pairs to the trial room, so that each bird was on the same shelf with a familiar neighbor. GWCS form flocks in winter and maintaining neighbor pairs may minimize social stress (Apfelbeck and Raess, 2008, but see Banerjee and Adkins-Regan, 2011). Birds were weighed and measured as above. Individuals were allowed to acclimate to the trial room for 24 h before starting a feeding treatment. We provided 6 g of food per day divided equally between two food trays and placed in the trays at the bottom of each cage. This amount was slightly more than the maximum that any bird was observed to eat each day when consumption was measured during pilot trials. Food was delivered just before lights off each day, so for Day 1 food was placed in the day prior. We then conducted one of two randomly assigned feeding treatments (“Easy” or “Hard” Supplementary Figure 4) on each set of neighbor pairs by shelf. This approach allowed birds to only see other individuals from the same treatment regimes. The Easy treatment consisted of trays with food, a few pieces of larger aquarium gravel (for weight) and no sand. The Hard treatment consisted of food that was entirely buried under ∼2 cm of sand. Exposure to initial treatments was continued for 3 days. On the third and last day, birds were removed from their cages shortly after the lights came on and a blood sample was taken (as described above) within 3 min of researchers entering the room. At the end of that same day, treatments were switched so that Easy treatments became Hard and Hard treatments became Easy, Supplementary Figure 4, which began the second 3-day treatment exposure. Blood samples were taken for measurement of baseline corticosterone at 9AM at the start of each experiment and every 3 days throughout each trial.
Activity Scoring
We recorded bird activity continuously using CCTV throughout each experiment. Videos were automatically saved as a series of 15-min files and we used this time period as the sampling unit for scoring. We initially performed behavioral scoring using only the daytime videos from Day 2 of each treatment, which was the day prior to blood sampling. We later increased the video scoring period to include some nocturnal observations (described below). Assistants were trained to score each file. To improve consistency, we used quantitative scores based on presence or absence of a criterion for a minimum of 10 s within a 15-min video segment. Presence of foraging behavior was defined by the bird occupying a food dish for longer than 10 s (actual digging or eating could not be clearly distinguished from the low-resolution CCTV video footage). We classified the presence of a specific type of hopping behavior (i.e., hopping from perch or floor up to walls or ceiling of the cage for longer than 10 s in each 15-min video) as “escape-type” behavior because individuals did not appear to be engaged in any significant foraging, exploration, or interaction during the periods when this behavior was manifested. A similar interpretation is noted for this species by Astheimer et al. (1992), however, this “escape” behavior may also represent a stereotyped response by individuals to cope with captivity (Rose et al., 2017).
Based on prior experience and other studies of this species, we initially assumed that birds were active only during daytime, and thus, only scored daytime videos. However, night videos were later examined to verify inactivity but revealed that birds were, in fact, variably active at night. Nocturnally active birds engaged in vigorous, escape-type hopping to walls or ceiling similar to that observed during the day. Consequently, nighttime activity was estimated by sampling one 15-min video per hour throughout the 16 h dark period, beginning approximately 15 min after lights-off. The total number of samples during which escape-type hopping activity was observed (with the maximum being the total number of hours of darkness, 16) is reported as the night activity score. Due to the differences in day and nighttime sampling procedures and duration, both day and night hopping data were converted to percentages of active intervals out of the total observed during that period. Raw scores (# intervals with hopping) were used for all statistical tests, but percentages are used in figures where day and nighttime trends are compared.
Statistical Analyses
JMP Pro (v. 11.2.1) or SAS (v. 9.4) (SAS Institute, NC, United States) were used for all statistical analyses. We used multiple linear regression to determine relationships among our variables of interest. Prior to all analyses, all variables were checked for normality and heteroskedasticity using the Shapiro-Wilks test. We further checked model assumptions by plotting residuals against predicted values.
Model Selection of Captive and Field Datasets for Heart Rate
To determine the overall linear relationship between fH and V̇O2, we used multiple linear regression. We used ANCOVA to test for differences in slopes between individual birds. Data were compared using mixed effect models with a Kenward-Rogers correction. Each hypothesis was tested separately for each dataset. Allometric effects were investigated through the inclusion of body mass or tarsus length as a fixed effect and as part of an interaction variable; variables were removed using stepwise selection when they did not explain significant variance (α = 0.05). Akaike’s information criterion (AICc) was compared during model selection to ensure that the final model provided the best fit. Competing models < 2 ΔAICc are included for comparison.
Assumption 1: We compared average daily heart rate to average daily temperature controlling for Bird ID, which was nested within trial (for captive data) or season (for field data) as a random effect.
Assumption 2: Recall that this assumption was only directly testable using the captive dataset. We compared baseline corticosterone (dependent variable) to average heart rate for the 24-h period prior to blood sampling and controlled for Bird ID nested within trial as a random effect.
Based on identified relationships between heart rate and temperature, Assumption 2 was indirectly tested using both captive and field datasets. To do this, we compared baseline corticosterone to average temperature (for the 24-h period before blood sampling) with Bird ID nested within trial as a random effect for captive data. In the field dataset, it was not necessary to include Bird ID in the model testing Assumption 2 because corticosterone was only collected once.
Data Analysis of Manipulation of Allostatic Load
We used linear mixed effect models to assess the influence of treatment (Easy or Hard), treatment exposure (1st or 2nd treatment within a trial), and their interaction, including post-treatment mass change (g), baseline corticosterone (ng/mL), and qualitative activity scores. We included individual bird ID as a random effect to control for repeated sampling.
While foraging workload was the key independent variable in our manipulation experiment on allostatic load, we chose to extend our analysis to include relevant metrics like activity score and change in body mass, evaluating relationships among outcomes. In these cases, baseline corticosterone was examined as the dependent variable, while activity, change in body mass over each trial, and their interaction were included as fixed effects in a linear mixed effects model. Because day and night activity are expected to be related, separate models were run to evaluate the effect of each with relation to the other variables.
Results
Heart Rate Validation
Heart rate and oxygen consumption were strongly correlated, with an overall equation of = 0.07 (±0.16) + 0.0039 (±0.0002)*fH (r2 = 0.68) for 6 birds (Figure 1). Neither log-transformation nor mass-adjustment of any kind improved this relationship. Slopes for individual birds were significantly different (p < 0.001) and ranged from 0.003 to 0.007. In the case of these two individuals, the data were either limited in range (consistently high heart rate) or diverged from linearity, reducing confidence in the calculation of slope. Sample sizes were insufficient to test for differences by sex and individual. However, when only sex was included in the model, it was not statistically significant.
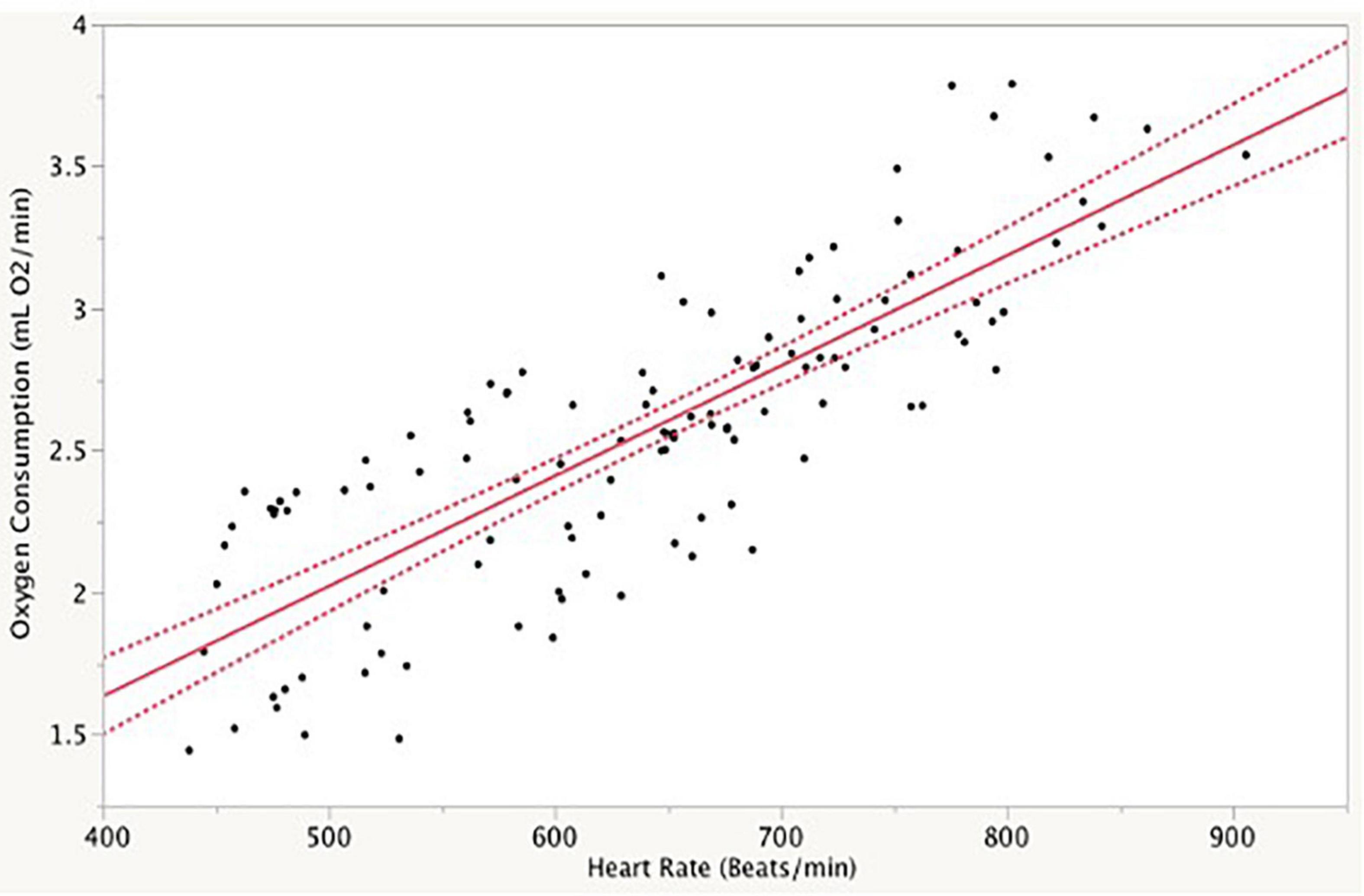
Figure 1. Relationship between oxygen consumption (mL O2/min) and heart rate (beats/min) of 6 white-crowned sparrows measured over a 2-h time period. Line indicates best fit regression with 95% confidence intervals. Each data point reflects a 5-min average.
In addition to inter-individual variation, anecdotal measurements made after the initial validation period indicated there was intra-individual variability. However, this variation was most apparent with regard to the intercept because slopes were largely similar. Pearson correlation coefficients within trials were almost universally high, and statistically redundant (r > 0.7). The relationship between fH and described previously for birds vary widely (Figure 2). Note that the relationship for GWCS lies within the major cluster of other species.
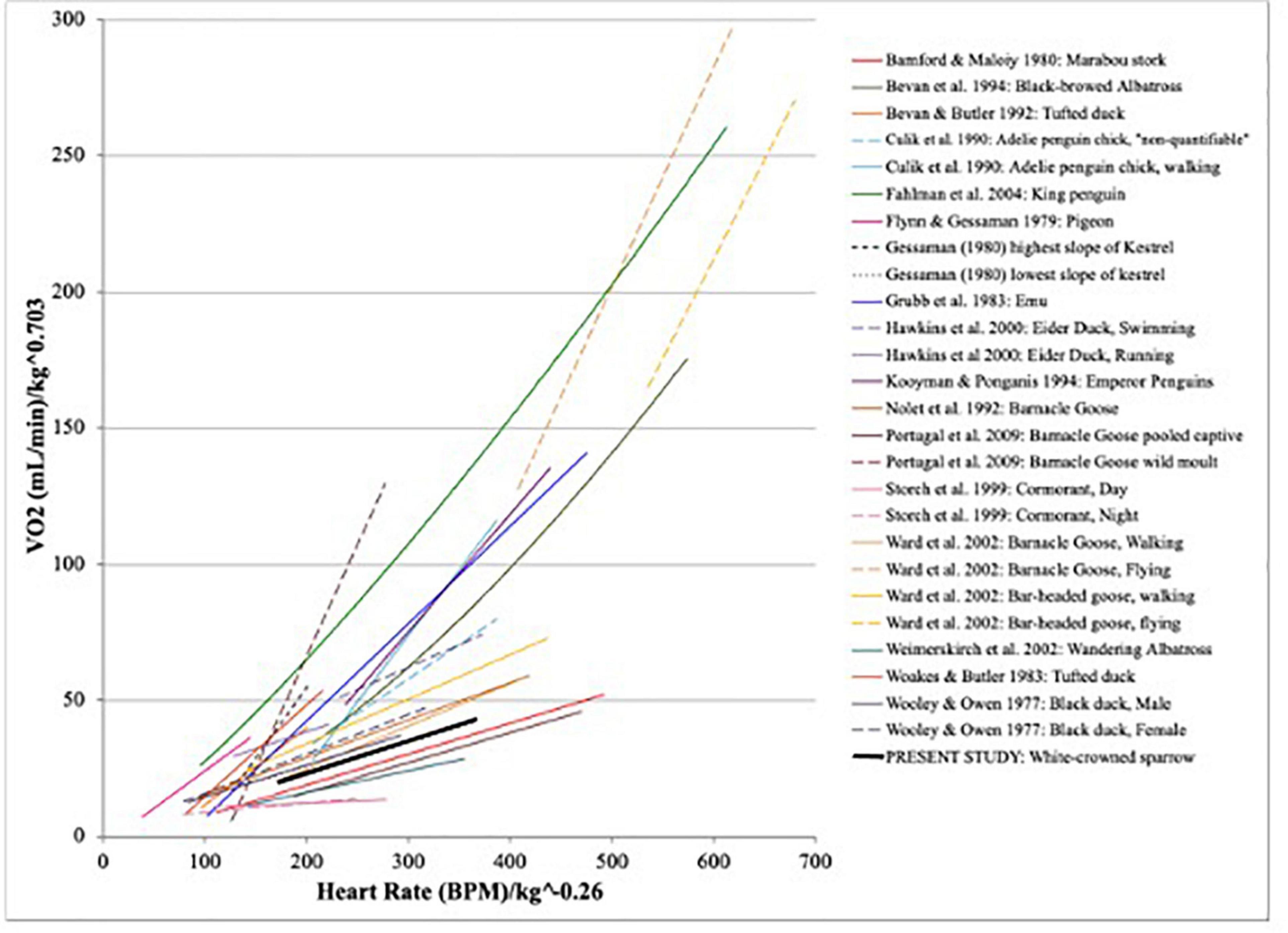
Figure 2. Previously reported relationships between heart rate and oxygen consumption for birds, with the relationship derived herein overlaid for comparison. Species described are: Leptoptilos crumeniferus (Bamford and Maloiy, 1980), Diomedea melanophrys (Bevan et al., 1994), Aythya Fuligula (Bevan and Butler, 1992), Pygoscelis adeliae (Culik et al., 1990), Aptenodytes patagonicus (Fahlman et al., 2004), Columba livia (Flynn and Gessaman, 1979), Falco sparverius (Gessaman, 1980), Dromiceius novaehollandiae (Grubb et al., 1983), Somateria mollissima (Hawkins et al., 2000), Aptenodytes forsteri (Kooyman and Ponganis, 1994), Branta leucopsis (Nolet et al., 1992), Branta leucopsis (Portugal et al., 2009), Phalacrocorax carbo (Storch et al., 1999), Branta leucopsis and Anser indicus (Ward et al., 2002), Diomedea exulans (Weimerskirch et al., 2002), Aythya fuligula (Woakes and Butler, 1983), and Anas rubripes (Wooley and Owen, 1977).
Testing Assumption 1: Did Temperature or Manipulation of Feeding Effort Influence Allostatic Load?
Temperature significantly predicted 24-h average heart rate for captive birds (F1, 40.6 = 12.8, p < 0.001; Figure 3 and Supplementary Table 1). Morphometric variation (body mass or tarsus length) did not significantly explain heart rate within our models (Supplementary Table 1). Removing Bird #130 (an influential outlier that was also the only bird we were able to successfully collect heart rate from two trials) had no effect on this relationship. Ambient temperature significantly predicted daily average heart rate for free-living birds (F1, 69.9 = 14.0, p < 0.001; Figure 4 and Supplementary Table 2). Neither body mass nor tarsus length significantly contributed to the model, alone or by interaction (Supplementary Table 2).
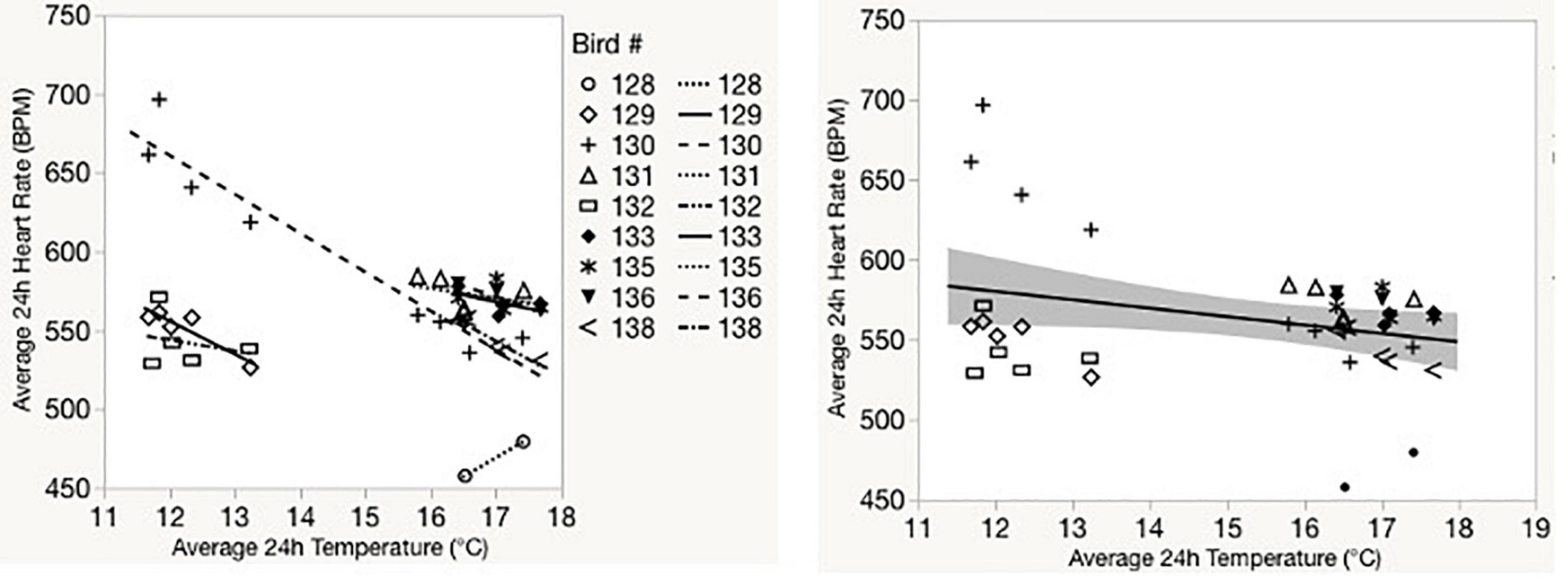
Figure 3. Standard regression lines showing relationships between 24-h average heart rate and temperature for individual birds (left) and for all birds (right) in captive experiments.
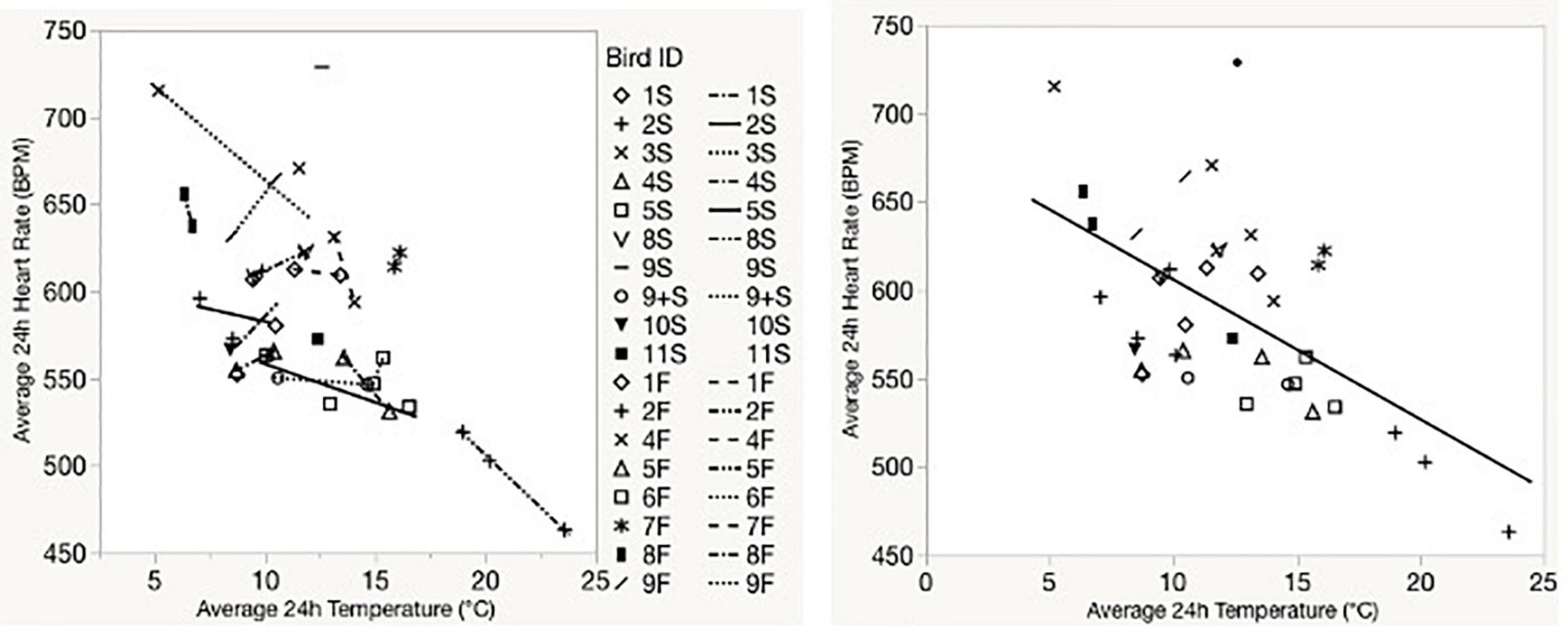
Figure 4. Standard regression lines showing relationships between 24-h average heart rate and temperature for individual birds (left) and for all birds (right) in field telemetry experiments. “F” and “S” in bird ID numbers reflect data collected during fall and spring field seasons, respectively.
Testing Assumption 2: Did Allostatic Load Predict Baseline Corticosterone?
When we compared plasma corticosterone to heart rate, tarsus, body mass, and their interaction, we found that there was no effect of average daily heart rate on corticosterone levels (F1, 10.1 = 0.0, p = 0.887; Figure 5 and Supplementary Table 3). There was also no effect of average daily daytime temperature on corticosterone levels among captive birds (F1, 10.9 = 0.0, p = 0.99; Figure 5A) or wild birds (F1, 16 = 0.3, p = 0.62; Figure 5B and Supplementary Tables 4, 5). Corticosterone levels were not significantly correlated with average temperature for the day on which the sample was taken, or with the average temperature from the night prior to sampling (Figure 6).
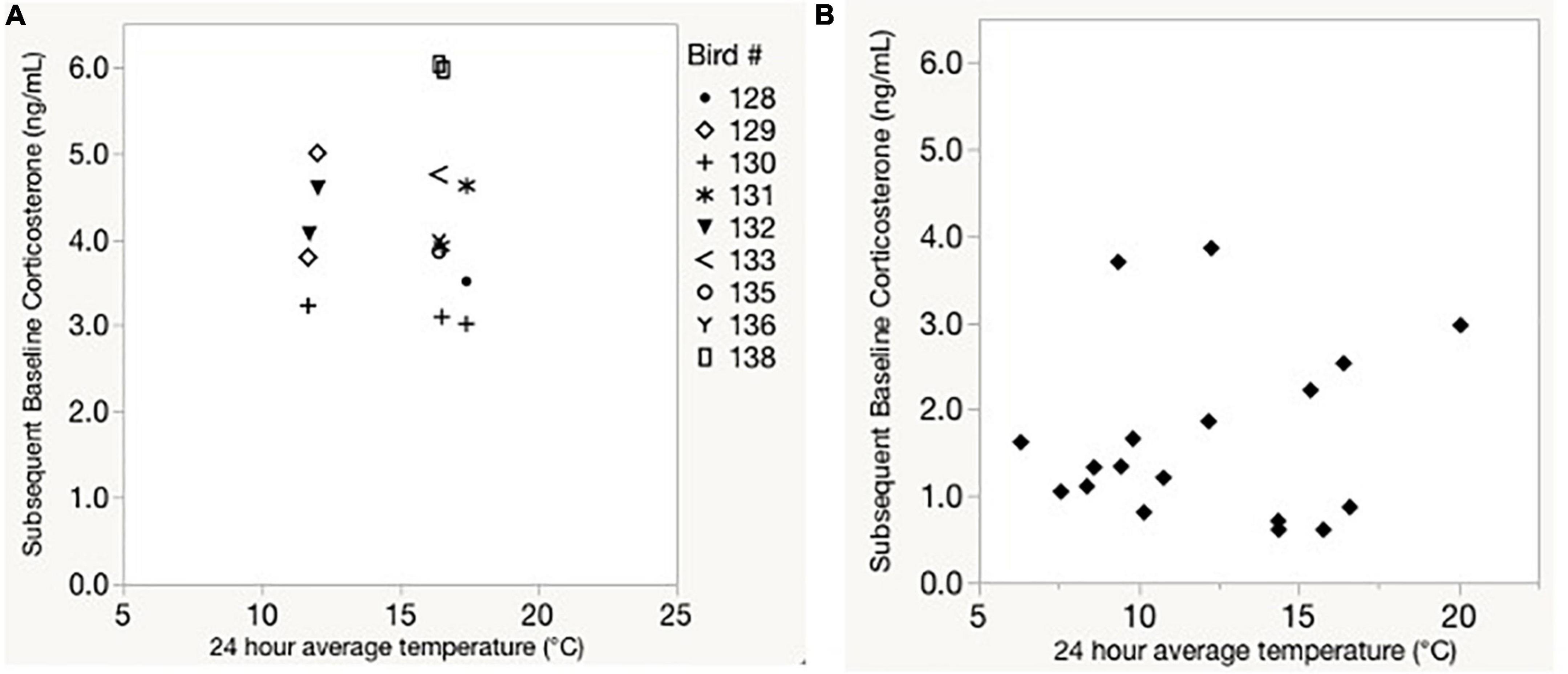
Figure 5. Baseline corticosterone vs. 24 h average temperature prior to blood sampling in captive (A, with repeated samples identified by bird ID) and field (B, with each point reflecting a unique bird ID). Aviary temperature was measured via thermistors placed on the cage, while field temperature was recorded by a local weather station.
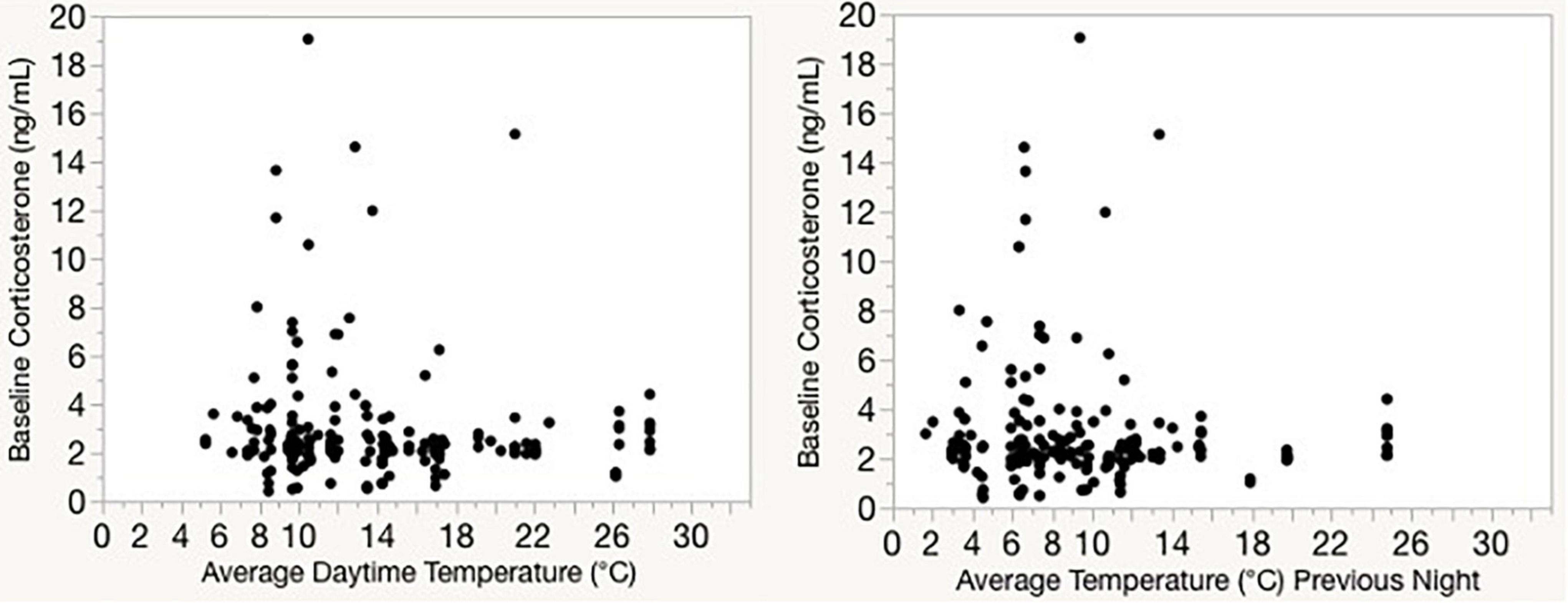
Figure 6. Baseline corticosterone samples from wintering Gambel’s white-crowned sparrows collected at multiple sites in Davis, CA between 2007 and 2014. Corticosterone levels are not significantly correlated with average temperature for the day on which the sample was taken (left), or with the average temperature from the night prior to sampling (right). Temperatures are obtained from a local weather station.
In manipulation experiments, workload treatment did not predict baseline corticosterone (F1, 13 = 0.1, p = 0.784). Exposure (1st vs. 2nd treatment) was a significant predictor in this model, with many birds having elevated baseline corticosterone during the first exposure regardless of workload treatment (F1, 13 = 4.9, p = 0.045; Figure 7). The interaction between treatment and exposure was not significant (F1, 13 = 1.8, p = 0.202).
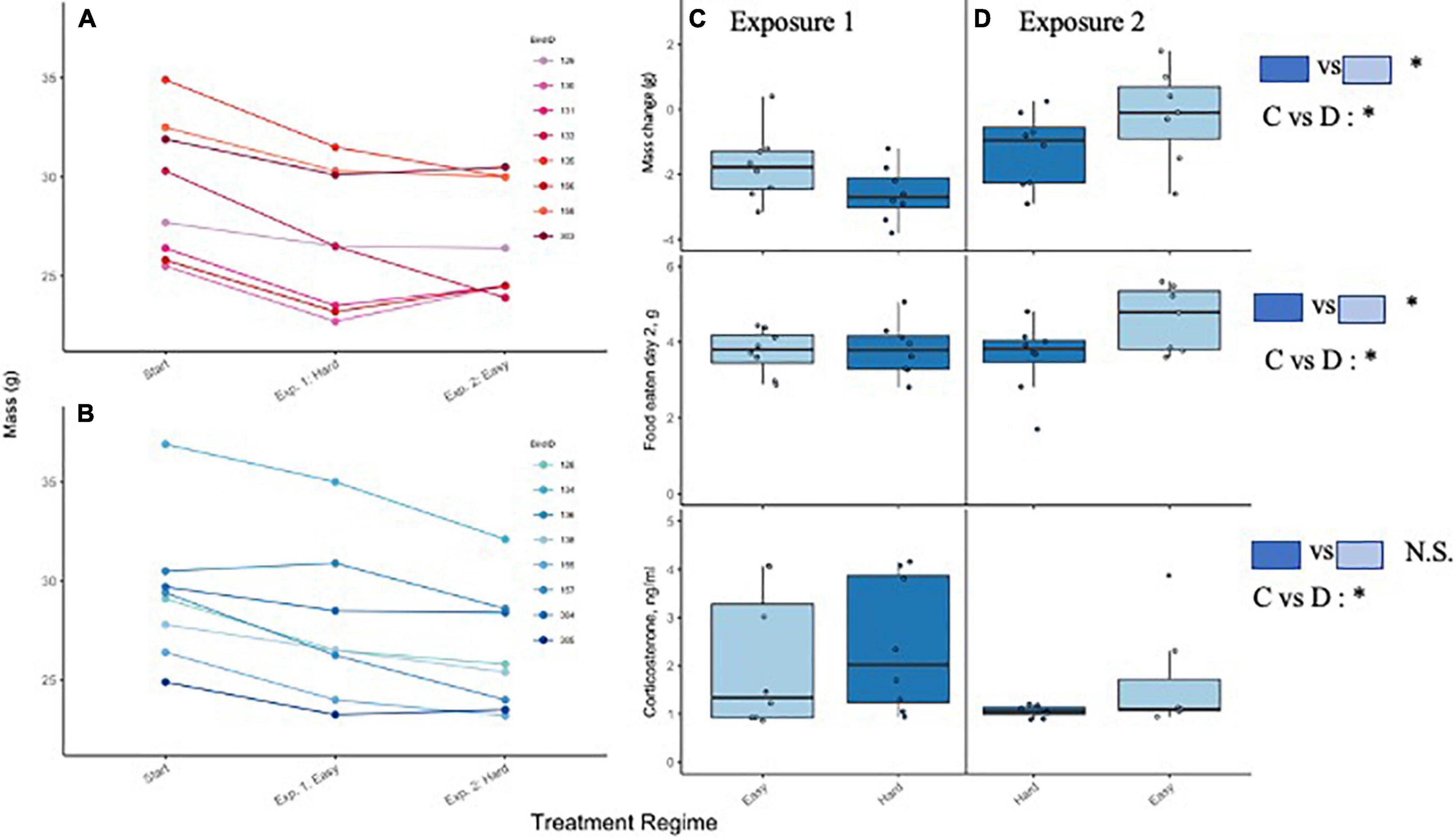
Figure 7. Left hand panels. Pattern of body mass change for each bird throughout the study. Panel (A) shows birds that received the easy workload regime during the first exposure, followed by the hard regime; panel (B) shows birds that received the reverse treatment order. Right hand panels. Change in body mass during trial was affected by workload treatment and 1st vs. 2nd exposure to treatment. Change in body mass during trial was affected by workload treatment regime (C,D top) and treatment order ((C,D) middle and bottom). Shading reflects treatment number, with treatments separated into C,D. Lower right hand panel. Baseline corticosterone (ng/mL) was not significantly influenced by workload (feeding regime) but was elevated during the first exposure regardless of regime. Most birds lost weight under all conditions.
Change in body mass differed by treatment (F1, 13 = 6.5, p = 0.024, lsmeans difference: Easy vs. Hard est. 0.96 ± 0.38 SE; Figure 7B) and exposure (F1, 13 = 14.8, p = 0.002, lsmeans difference: 1 vs. 2 est. = −1.45 ± 0.38 SE; 7C), but not their interaction (F1, 13 = 0.1, p = 0.835). Body mass declined almost uniformly during the first trial, but the Hard treatment generally incurred a larger weight loss regardless of exposure. When the Easy treatment was delivered during the second exposure, birds appeared to recover mass (Figure 7). The amount of food that birds ate (on D2) also differed by treatment (F1, 13 = 6.9, p = 0.021), but not by exposure (F1,13 = 3.2, p = 0.097) nor by treatment*exposure (F1,13 = 2.2, p = 0.160). Birds ate significantly more food during the Easy regime, but the magnitude of difference was small on the first exposure and most obvious during the second. Neither workload treatment or 1st/2nd exposure was associated with significant differences in escape-type hopping during daytime hours (treatment F1, 13 = 1.7, p = 0.213), lsmeans difference: Easy vs. Hard = −1.39 ± 0.11; exposure F1, 13 = 2.3, p = 0.155, lsmeans difference: 1 vs. 2 estimate = −1.61 ± 1.06; or their interaction (F1, 13 = 0.1, p = 0.820; Figure 8A) though there was a suggestive difference with potential reduction in escape-type hopping at night during the Hard treatment (treatment F1,13 = 4.1, p = 0.063; exposure F1, 13 = 0.0, p = 0.993 lsmeans estimate: treatment: 0.87 ± 0.43 SE; exposure: 0.00 ± 0.43; Figure 8B).
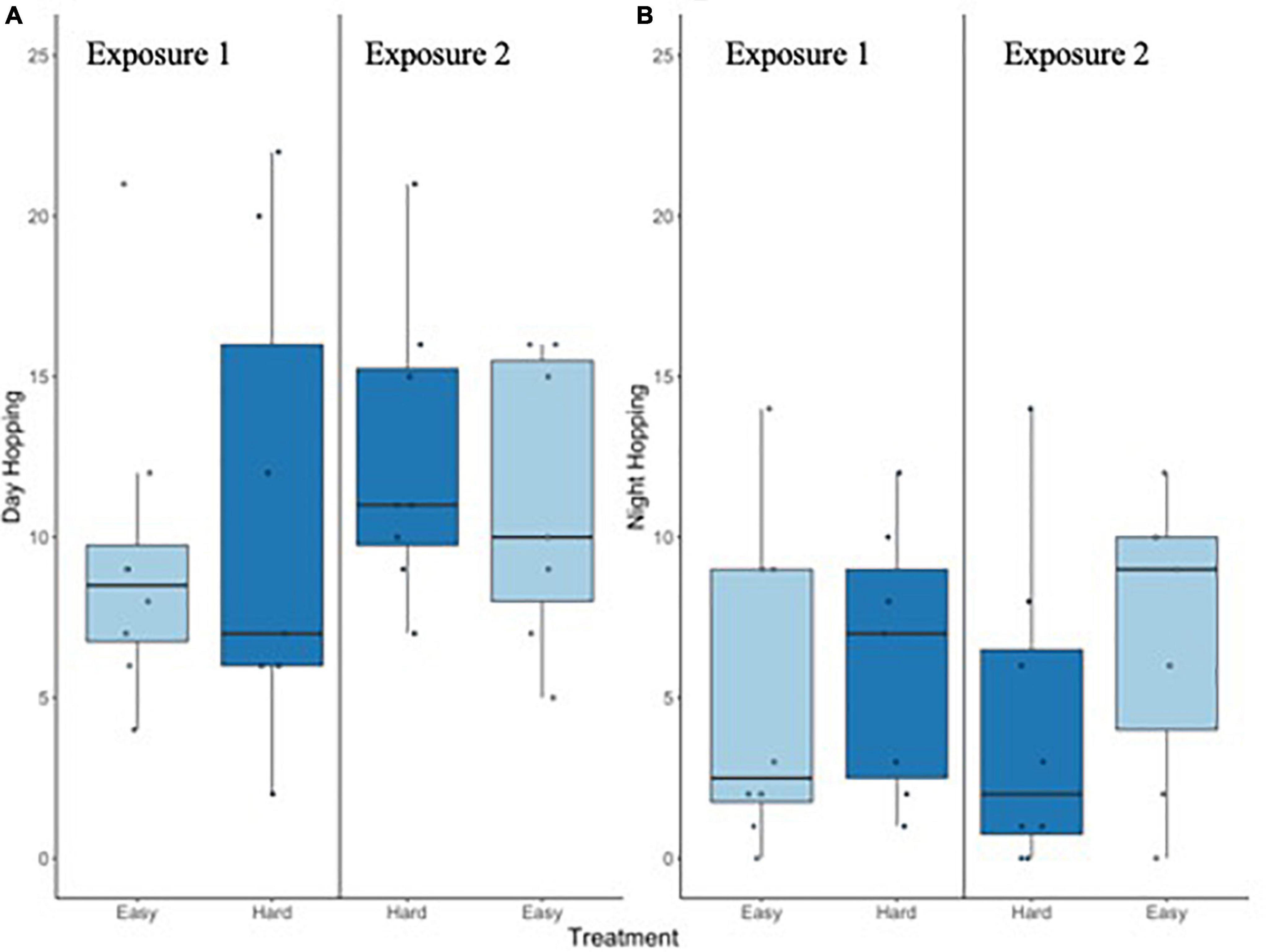
Figure 8. Number of 15-min intervals in which escape-type hopping was observed during daylight hours (A; all intervals scored) and at night (B; sampled hourly) on the day prior to blood sampling. This type of activity was not significantly affected by workload regime or exposure.
Additional Observations From Manipulation of Foraging Effort: Relationships Among Outcomes
Corticosterone was predicted by weight change (F1, 12 = 9.7, p = 0.009), but not escape-type hopping activity during the day (F1, 12 = 3.7, p = 0.080) or at night. The interaction between daytime activity and weight change was also a significant predictor of baseline corticosterone (F1, 12 = 11.8; p = 0.005). The significant relationship between corticosterone and weight change was driven by the interaction term; thus, we will focus on the interpretation of the interaction. Exploration of this interaction suggests a relationship between corticosterone and activity levels that flips based on extremity of weight change. If we bin weight change into two categories, for birds that lost a lot of weight (>1.5 g), elevated baseline corticosterone was mainly associated with high activity levels, while among birds with more stable body mass (<1.5 g change) those with elevated baseline corticosterone were characterized by low activity levels (Figure 9). In contrast, nocturnal activity and weight change were not significantly related in any models. Furthermore, examination of the data reveals a striking negative trend between day and nighttime activity with regard to the interaction discovered with the daytime data (Figures 8, 9).
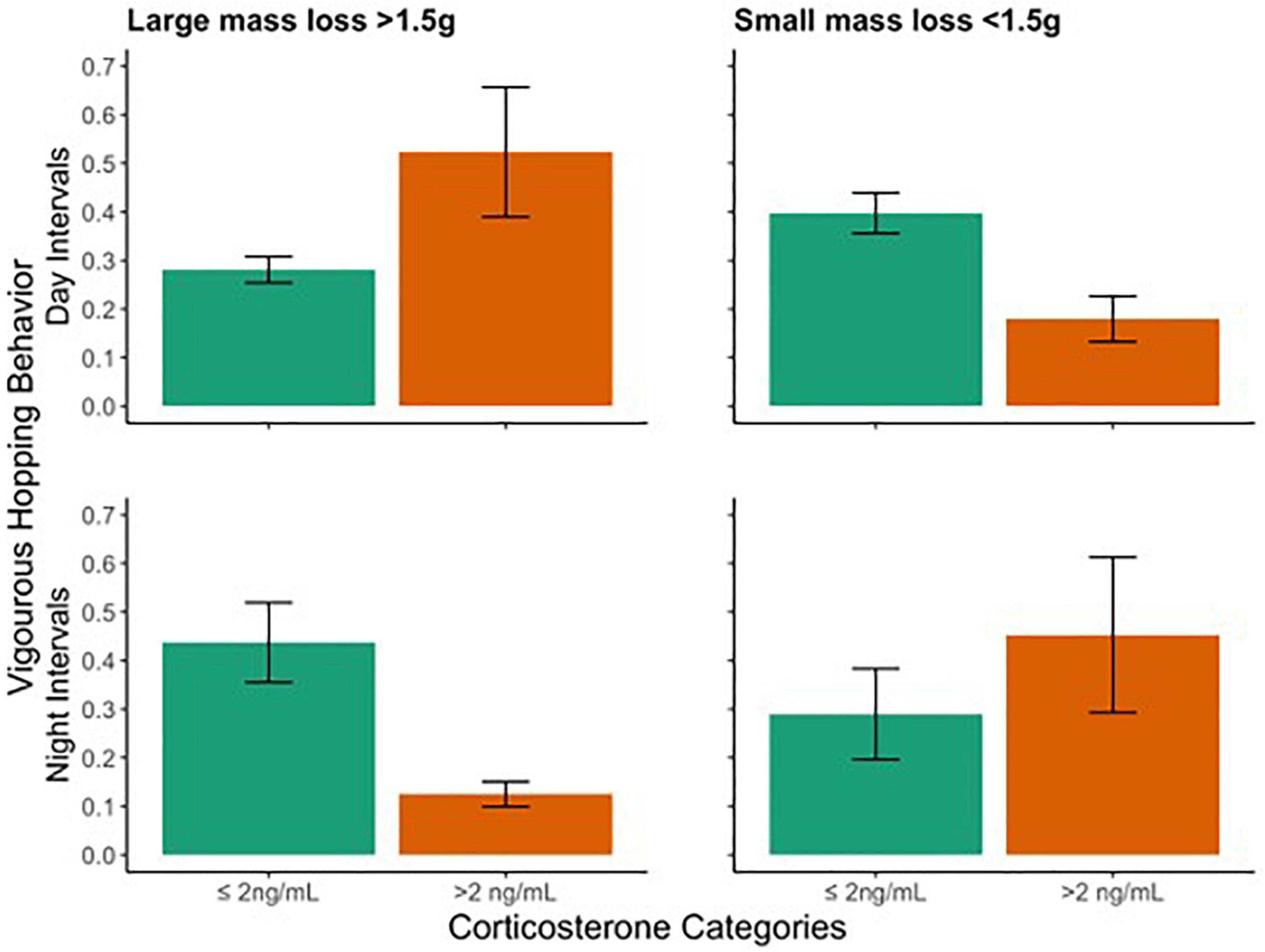
Figure 9. Difference between day (top panels) and night (bottom panels) escape-type hopping behavior with regard to baseline corticosterone for binned categories of large (left) and small (right) mass loss. Bars show means ± SEM for each group. Activity data are displayed as a percent of intervals counted. While no significant effects or interactions relate nighttime activity to baseline corticosterone, overall trends are opposite to the significant interaction between daytime activity, weight change, and corticosterone.
Discussion
Results from our validation experiments supported Assumption 1 in that changes in activity were reflected in changing V̇O2 and with associated change in heart rate. Heart rate telemetry further showed that variation in daily temperature influenced average daily heart rate and, thereby, allostatic load. These findings do not exclude the possibility that compensatory mechanisms, such as decreased voluntary activity (González-Gómez et al., 2011) or facultative hypothermia (McKechnie and Lovegrove, 2011), might mitigate such influence especially in relation to individual variation.
Interpretation of outcomes for manipulation of feeding effort with respect to Assumption 1 is less clear because we have no direct measure of energy expenditure. There is evidence to suggest that some birds may have engaged in compensatory strategies to reduce impact of the hard treatment on allostatic load, e.g., by eating less food or by reducing activity at night. However, these trends were far from clear or dramatic, with a much more sizeable influence of 1st/2nd exposure and associated interactions. The fact that most birds lost weight during most treatments, suggests that something other than behavioral compensation for increased workload is at play. However, we cannot eliminate the possibility that some compensation may have mitigated the impact of workload treatment on allostatic load.
Assumption 2 was not supported by our data in any experiment. We found no association between baseline corticosterone and allostatic load using either direct measurement of heart rate (aviary heart rate telemetry) or by inferring influence of temperature on allostatic load (aviary and field telemetry along with other field data) based on support for Assumption 1. We also found that increased foraging workload did not significantly affect baseline plasma corticosterone levels in captive birds. Body mass and tarsus length explained little of the variation in our models and thus were not considered influential either as determinants or as mediators/moderators of the response variables used to test this assumption. Nonetheless, body size is a potentially important source of error. Where inter-individual variation in heart rate is influenced by heart size, for example, rather than factors related to allostatic load, this variation impairs the power of the model we used to compare data between individuals in tests of Assumption 2. The influence of temperature on allostatic load may also vary with body size, but likely less systematically due to other factors such as microhabitat or feather condition. While this source of error is important, our failure to detect a relationship between baseline corticosterone and allostatic load in multiple different tests of Assumption 2 provides more robust grounds for its rejection.
Potential for Relationships at Other Levels of the Hypothalamic-Pituitary-Adrenal Axis
The framework underlying the allostasis model specifically suggests that circulating glucocorticoids should track allostatic load. However, in outlining the model, McEwen and Wingfield (2003) also stated that glucocorticoids function within a suite of “interconnected hormonal mediators.” Changes in glucocorticoid transport, local enzymatic control, receptor density or responsiveness may act independently to effect change on the organism (see also Ball and Balthazart, 2008). The primary plasma transport protein for glucocorticoids, corticosteroid binding globulin (CBG), is believed to both facilitate transport and prevent its action (Breuner and Orchinik, 2002), with the latter being the effect most widely accepted as outlined in the free hormone hypothesis (Mendel, 1989). Local control of hormone concentrations can occur through enzymatic breakdown or reconstitution via expression of 11β-hydroxysteroid dehydrogenase enzymes (Tomlinson et al., 2014; Pérez et al., 2020). Other molecules also co-vary with circulating levels of corticosterone across seasons (Breuner and Orchinik, 2001), but some changes can occur even when total corticosterone remains constant (Lynn et al., 2003; Sesti-Costa et al., 2012; Krause et al., 2015). Hence, the possibility that Assumption 2 may be fulfilled at a molecular level not explored in this study cannot be eliminated, and further investigation in this area could be a fruitful direction for future investigation. Nonetheless, systemic effects of hormone action are most parsimoniously regulated by change in hormone level. Tissue-level changes in hormone action are far more likely to be relevant to fine-tuning local control.
Context on Validation of Heart Rate as a Measure of Energy Expenditure
The oxygen consumption values observed for Gambel’s white-crowned sparrows are within the range expected for this species. King (1964) reported a standard metabolic rate for Z. l. gambelii of 2.43 mL/g*hr (1.16 mL/min) based on the average mass reported for his birds. This value is expectedly lower than the minimum reported here (mean minimum = 1.68 ± 0.13 mL/min) for fed birds at rest. Most studies comparing fH to V̇O2 tend to vary widely (Figures 1, 2) and did not typically compare slopes between individual animals. When individual slopes were tested, the application of a combined equation was sometimes rejected when individuals differed significantly. However, heart rate is not expected to explain 100% of the variance in oxygen consumption, a priori. While many studies have found consistent slopes among individuals, others found broad differences (Gessaman, 1980), or found slopes differ with flight (Ward et al., 2002) or captivity (Portugal et al., 2009). Even with the considerable variance in energetic expenditure that cannot be captured using heart rate there is still a positive relationship between fH and V̇O2 supported by the data from our study organism. Furthermore, if we adjust for large differences in body size across studies using mass exponents of 0.703 for avian field metabolic rate (Tieleman and Williams, 2000) and −0.26 for heart rate (White and Kearney, 2014), the observed relationship we found is consistent with other studies of different species, particularly when (mass-adjusted) ranges of heart rates were examined. While it is not possible to draw specific conclusions from comparisons across such a wide range of phyla, based on this rough visual comparison our data bear the greatest similarity to relationships established for walking ducks and geese (Figure 2).
Because V̇O2 is itself a proxy for direct calorimetry, even when (or if) heart rate varies predictably with V̇O2, the prediction of energy expenditure is necessarily imperfect (Walsberg and Hoffman, 2005, 2006). Even direct calorimetry itself is far from error-free, so the source of discrepancies between direct and indirect methods may never be fully understood (Speakman, 2014). In any case, whether reported as milliliters of oxygen or kilojoules, some error in estimating true energetic expenditure is a consequence of indirect calorimetry.
To our knowledge, no study has investigated a direct relationship between heart rate and energetic expenditure via direct calorimetry. Heart rate, when used as a measure of energy expenditure, is inarguably a blunt instrument. Small changes in energy expenditure, or small differences between animals may well be undetectable by fH. However, to the extent that relationships between environmental factors and fH are observable, the data presented in this paper justify the inference of a corresponding relationship between the same environmental factors and energy expenditure overall. This finding is in accordance with other studies that have utilized heart rate telemetry to evaluate energy expenditure in songbirds (Bowlin and Wikelski, 2008; Cyr et al., 2008; Steiger et al., 2009; Bisson et al., 2011, 2009; Romero and Wingfield, 2016). While heart rate data are not converted to units of oxygen consumption using the equation validated here, the consistency of correlation, particularly of the slope, both supports Assumption 1 and justifies the use of heart rate as a proxy measurement for energy expenditure when testing the model of allostatic load.
Context on the Response of Glucocorticoids to Weather Changes
Several studies have looked for relationships between glucocorticoid hormones and changing temperatures with variable results (reviewed in de Bruijn and Romero, 2018). Among relevant studies (excluding heat stress, heterotherms, and early development), the impact of temperature on circulating corticosterone remains mixed with, some finding glucocorticoids do vary with temperature (Huber et al., 2003; Frigerio, 2004; Dorn et al., 2014) while others did not or found responses varied with other factors (Wingfield et al., 1996, 1997, 2003; Romero et al., 2000; Lobato et al., 2008; Knutie and Pereyra, 2012). In contrast with the present study, all unequivocally positive results used fecal corticosteroid metabolites to evaluate glucocorticoid response, which may be an important distinction. While stable changes in baseline corticosteroid levels are expected to be observable in fecal metabolite levels, many other kinds of change, e.g., altered responsiveness to minor stressors in the environment or the amplitude of circadian peaks, can influence fecal metabolite levels but go undetected in an instantaneous sample of blood plasma (Ninnes et al., 2010; Sheriff et al., 2010). Fecal samples contain an amount of corticosteroid metabolites accumulated over the time period during which food material has passed through the gut of the animal. Thus, all endocrine events that occurred during this time can influence the amount of metabolites in a sample. Additionally, any factor that affects gut transit time can also influence the amount of metabolites found in any given sample (Goymann et al., 2006). If glucocorticoids were to increase abruptly in response to reduced perturbation resistance potential, as in the alternative to Assumption 2, this increase could be evident in fecal metabolite sampling but not in instantaneous plasma measurements of hormone, depending on sample timing.
Much of the other evidence commonly cited in favor of a role for glucocorticoids in mediating responses to weather is drawn from studies of extreme weather events (Rohwer and Wingfield, 1981; Wingfield et al., 1983; Schwabl et al., 1985; Rogers et al., 1993; Smith et al., 1994; Raouf et al., 2006). In these situations, cold temperature is only one part of a larger environmental perturbation that may involve compromised shelter and/or restricted access to food. Food restriction, in particular, is often cited as the most likely explanation for the observed hormone response (Schwabl et al., 1985). Additionally, changes to corticosterone were observed in the absence of notable temperature change (Rogers et al., 1993; Raouf et al., 2006) or appeared to be dependent on season or breeding condition (Wingfield et al., 1983; Ramenofsky et al., 1992). Other studies on white-crowned sparrows found limited effects of cold temperature in males of one subspecies (Wingfield et al., 1997), but no differences in corticosterone in any other group. Because their tests were run concurrently with photostimulation, it is interesting to note that temperature itself had direct inhibitory effects on gonadal recrudescence, which should minimize allostatic load by reducing the cost of gonadal growth. This potential compensatory strategy merits further consideration because it suggests that other physiological processes might, similarly, respond to temperature in these birds.
Even though a relationship between energy expenditure and temperature was observed in this study, if such direct physiological compensation were to differ among individuals, this could detract from the robustness of energy expenditure as an estimate of allostatic load. The presence of some confirmed relationships among negative results (Wingfield et al., 1997; Romero et al., 2000) suggests that there may be a threshold, or multiple interacting factors influencing how circulating glucocorticoids interact with temperature. A recent study of greylag geese (Anser anser) showed sex-based variation in the response of heart rate and core body temperature to ambient changes (Wascher et al., 2018), which supports the potential for complex interactions to mask impacts of temperature on corticosterone via changes in allostatic load.
Context and Additional Observations on Responses to Workload Manipulation
When we manipulated foraging workload, we observed no change in baseline plasma corticosterone. This contrasts with a recent test of workload on feather corticosterone [in growing feathers of female ducklings, Anas platyrhynchos (Johns et al., 2018)]. These authors found that when elevation of allostatic load was induced by obstacles and adding weight to individuals, corticosterone levels of feathers growing throughout the treatment period were increased. Like fecal corticosterone, feather measurements represent a longer term “sum” of corticosterone change in comparison with the “snapshot” of a plasma sample. In addition, the manipulations in this study were more extreme, likely inducing allostatic overload. The lack of associations of allostatic load with baseline plasma corticosterone observed in this study are, therefore, not inconsistent with existing data. Curiously, we did find a positive effect on baseline corticosterone in this study – not of workload treatment but of the first exposure, regardless of treatment. We also observed an interaction with the concurrent outcomes of daytime escape-type activity levels and weight loss when these were treated as independent variables that could influence corticosterone levels.
The effect of first exposure regardless of treatment suggests that something about the birds’ introduction to the experimental room had an effect on baseline corticosterone that persisted through the first 3 days of treatment. Even though plasma samples after the first exposure were higher than those sampled later, the direction of effect cannot be inferred because pre-trial samples were not collected. Initial exposure could have increased baseline corticosterone levels, after which they slowly returned to previous levels, but it is alternatively possible that hormone levels may have fallen steadily, on average, throughout the two trials.
Prior to experimental trials, all birds had been trained in the experimental room for periods of 1 week or more, so nothing about the environment was novel. If the training had created a negative association, we would expect birds to respond specifically to the “hard” workload regime on which they were trained at that time – this was not observed. While the effect of translocating birds within captivity has not been formally studied, the large elevation in baseline corticosterone associated with being brought into captivity has been found to extend for at least 3–4 days (Wingfield et al., 1982). If the experience of changing rooms were perceived as a “stressor” of this type, though much smaller in magnitude, then the time frame of acclimation does fit with the pattern observed here. However, given the regularity with which birds are transferred between rooms in captive experiments, it seems unlikely that a response of this kind would have eluded detection throughout the extensive history of study in this species.
Alternatively, baseline corticosterone levels may have steadily declined in response to the room transfer. The experimental room contained only 4 birds, whereas the housing room contained 16 birds in individual cages. The effect of housing density on songbirds is poorly understood but its effect on corticosterone has been found to depend on social rank (Nephew and Romero, 2003). Such responses are therefore likely to vary with social structure and seasonality. Our birds were maintained on short days, a photoperiod that is normally associated with wintering conditions when white crowned sparrows remain in loose flocks. The proximity of caged conspecifics is arguably similar to how birds are typically spaced in the field, at least during daylight hours. Average baseline plasma corticosterone levels measured in the context of other studies with housing conditions and photoperiod similar to those utilized here are comparable to the intermediate-to-higher values reported in this study (M. Ramenofsky, J.S. Krause, pers. com., e.g., Busch et al., 2008a,b). Taken together, these data show that a steady decline of corticosterone from a higher level prior to exposure 1 cannot be eliminated.
The interaction between weight loss and daytime activity level in predicting baseline corticosterone is unexpected but potentially meaningful. Astheimer et al. (1992) performed an entirely different experiment, in which the same species of birds were implanted with corticosterone, fed either ad-libitum or a restricted diet, and monitored for activity. These birds exhibited a relationship between corticosterone treatment and activity that was opposite for feed restricted birds compared with those fed ad-libitum. Specifically, corticosterone-implanted birds both increased activity during feed restriction, but were less active than controls during the ad-libitum feed regime. A previous study (Buttemer et al., 1991) with similar conditions showed that fasted and corticosterone-implanted birds all minimized metabolic rate by reducing episodes of nighttime arousal, but there was no additive effect of corticosterone-implantation with fasting; i.e., they either conserved at night or they did not. In the present study, neither high weight loss nor high corticosterone predicted night activity and the interaction between the two was also not statistically significant. While the study conducted by Astheimer et al. (1992) is radically different in design from the experiment performed here, there is logic in the comparison. In either interpretation of the correlation between baseline plasma corticosterone levels and order of exposure described above, the most likely explanation is one in which a classical “stressor” of some kind causes corticosterone levels to increase. Hence, although corticosterone is examined here as an outcome in reference to activity and change in body mass, it may be more appropriate to consider an alternate model in which baseline corticosterone levels, influenced by some stressor rather than implantation, may predict escape-type activity in a manner dependent on the nutritional status of the animal. When organized in this way, the outcomes of these two studies, at least with respect to daytime activity, are strikingly similar.
Conditional effects of the kind observed here are gaining recognition as a likely explanation for otherwise contradictory data, particularly with regard to the relationship between glucocorticoids and locomotion, which is also highly relevant to site abandonment following environmental perturbations, i.e., the “take it or leave it” decision (Wingfield and Ramenofsky, 1997). In mountain white-crowned sparrows (Z. l oriantha), corticosterone results in birds delaying return to a territory abandoned in response to bad weather conditions, but enhances foraging (rather than inducing abandonment) during fair weather (Breuner and Hahn, 2003; Breuner et al., 2013). In black-legged kittiwakes (Rissa tridactyla) corticosterone implants increased foraging and flying behavior only in birds with good body condition (Kitaysky et al., 2001a,b; Angelier et al., 2007). Similar data also exist for rats, in which corticosterone increases wheel-running only in food-restricted animals and furthermore is permissive of the increase associated with food restriction based on comparison with adrenalectomized controls (Duclos et al., 2009). Glucocorticoids are also permissive to locomotor effects of stimulant drugs in rodents (Marinelli et al., 1997), so all of these examples, inclusive of the present study, may reflect a permissive mechanism by which endocrine and environmental signals may be integrated to result in an optimal behavioral effect. A permissive role in mediating the effect of weight loss does not explain the additional and opposite effect of corticosterone in reducing daytime activity for animals that maintained weight (or were fed ad lib in Astheimer et al., 1992). This may occur via a separate but, similarly, integrative mechanism. Neuroendocrine peptides such as corticotropin releasing factor can also influence locomotor activity (Morley and Levine, 1982; Maney and Wingfield, 1998; Romero and Wingfield, 2016), and the extent to which such other factors might be involved in further mediation is unknown.
The three-way interaction observed in this study, particularly the important role played by weight loss as a conditional factor in determining the effect of corticosterone, is fundamentally compatible with a view of the model of allostatic load in which resource availability (EG in the original model), is equal in importance to allostatic load. Organization, evolution, and conceptualization of such a complex, integrated regulatory system within the framework of allostasis needs more investigation in the context of the organism in its environment.
Future Directions
This study finds evidence to support the hypothesis that fluctuations in average daily energy expenditure, here considered as allostatic load, occur and are related to ambient temperature in wintering GWCS. Despite multiple tests, no evidence is found of any relationship between routine fluctuations in allostatic load and circulating baseline corticosterone, as predicted by Wingfield and McEwen’s (2003) model. While it is possible that this negative result is spurious, or that other levels of the hypothalamic-pituitary-adrenal axis and/or associated proteins may be the true targets of regulation, these data are consistent with other studies in suggesting that a relationship, if or where present, may not be as simple or direct as portrayed in the original model.
Current results call for a re-examination of this model in view of the challenges presented here. If baseline circulating corticosterone does not increase gradually with routine allostatic load, there may instead be a threshold at which a response is generated and this threshold may vary across individuals in relation to condition, resource availability, and other factors that influence risk of energetic crisis. This raises the possibility that individual perturbation resistance potential may play a key role in determining when a threshold of allostatic load and available resources is reached. This would signal critically reduced perturbation resistance potential thus triggering a glucocorticoid response and the emergency life history stage. Further work examining the response of glucocorticoids to allostatic load should anticipate interactions that may serve to define that threshold.
Data Availability Statement
The raw data supporting the conclusions of this article will be made available by the authors, without undue reservation.
Ethics Statement
The animal study was reviewed and approved by Institutional Animal Care and Use Committee, University of California, Davis.
Author Contributions
KW designed the investigations, conducted the research, and writing of the final manuscript. SA analyzed the data and produced many of the figures. JW was involved in the design of the investigations, provided funding and was involved in writing. All authors contributed to the article and approved the submitted version.
Funding
The investigations presented here were funded by the University of California, Davis Endowment in Physiology and NSF grant numbers ARC – 0909133, BIO-1066241, and IOS-1558049 to JW.
Conflict of Interest
The authors declare that the research was conducted in the absence of any commercial or financial relationships that could be construed as a potential conflict of interest.
Publisher’s Note
All claims expressed in this article are solely those of the authors and do not necessarily represent those of their affiliated organizations, or those of the publisher, the editors and the reviewers. Any product that may be evaluated in this article, or claim that may be made by its manufacturer, is not guaranteed or endorsed by the publisher.
Acknowledgments
This work would not have been possible without the assistance of a great many people, especially Samin Khoshkhoo, Isaac Uvarov, Michaela McGuigan, Sarena Olson, Anastazia Capparelli, Amber Kanwer, Veronica Cordero, Madhulika Battu, Kristina Mardinian, Richard Ly, Courtney Runyan, Fei Hsuan Annie Chen, Fareeha Waheed, Amber Kanwer, Irene Arroyo-Lozano, Isaac Uvarov, Jesse Krause, Jonathan Pérez, and Zoltan Nemeth. We are also grateful for essential consultation and advice offered by J. Patrick Kelley, Jamie Cornelius, Tom Hahn, and Marilyn Ramenofsky.
Supplementary Material
The Supplementary Material for this article can be found online at: https://www.frontiersin.org/articles/10.3389/fevo.2022.855152/full#supplementary-material
Footnotes
References
Angelier, F., Clément-Chastel, C., Gabrielsen, G., and Chastel, O. (2007). Corticosterone and time–activity budget: an experiment with Black-legged kittiwakes. Horm. Behav. 52, 482–491. doi: 10.1016/j.yhbeh.2007.07.003
Apfelbeck, B., and Raess, M. (2008). Behavioral and hormonal effects of social isolation and neophobia in a gregarious bird species, the European starling (Sturnus vulgaris).”. Horm. Behav. 54, 435–441. doi: 10.1016/j.yhbeh.2008.04.003
Astheimer, L. B., Buttemer, W. A., and Wingfield, J. C. (1992). Interactions of corticosterone with feeding, activity and metabolism in passerine birds. Ornis Scand. 23, 355–365. doi: 10.2307/3676661
Ball, G. F., and Balthazart, J. (2008). Individual variation and the endocrine regulation of behaviour and physiology in birds: a cellular/molecular perspective. Philos. Trans. R. Soc. B Biol. Sci. 363, 1699–1710. doi: 10.1098/rstb.2007.0010
Bamford, O. S., and Maloiy, G. M. (1980). Energy metabolism and heart rate during treadmill exercise in the Marabou stork. J. Appl. Physiol. 49, 491–496. doi: 10.1152/jappl.1980.49.3.491
Banerjee, S. B., and Adkins-Regan, E. (2011). Effect of isolation and conspecific presence in a novel environment on corticosterone concentrations in a social avian species, the zebra finch (Taeniopygia guttata). Horm. Behav. 60, 233–238. doi: 10.1016/j.yhbeh.2011.05.011
Bautista, L. M., Tinbergen, J., Wiersma, P., and Kacelnik, A. (1998). Optimal foraging and beyond: how starlings cope with changes in food availability. Am. Nat. 152, 543–561. doi: 10.1086/286189
Bevan, R., Woakes, A., Butler, P., and Boyd, I. (1994). The use of heart rate to estimate oxygen consumption of free-ranging black-browed albatrosses Diomedea melanophrys. J. Exp. Biol. 193, 119–137. doi: 10.1242/jeb.193.1.119
Bevan, R. M., and Butler, P. J. (1992). The effects of temperature on the oxygen consumption, heart rate and deep body temperature during diving in the tufted duck Aythya fuligula. J. Exp. Biol. 163, 139–151. doi: 10.1242/jeb.163.1.139
Bisson, I.-A., Butler, L. K., Hayden, T. J., Kelley, P., Adelman, J. S., Romero, L. M., et al. (2011). Energetic response to human disturbance in an endangered songbird. Anim. Conserv. 14, 484–491. doi: 10.1111/j.1469-1795.2011.00447.x
Bisson, I.-A., Butler, L. K., Hayden, T. J., Romero, L. M., and Wikelski, M. C. (2009). No energetic cost of anthropogenic disturbance in a songbird. Proc. R. Soc. Lond. B Biol. Sci. 276, 961–969. doi: 10.1098/rspb.2008.1277
Bowlin, M. S., and Wikelski, M. (2008). Pointed wings, low wingloading and calm air reduce migratory flight costs in songbirds. PLoS One 3:e2154. doi: 10.1371/journal.pone.0002154
Breuner, C., and Orchinik, M. (2002). Plasma binding proteins as mediators of corticosteroid action in vertebrates. J. Endocrinol. 175, 99–112. doi: 10.1677/joe.0.1750099
Breuner, C. W., and Orchinik, M. (2001). Seasonal regulation of membrane and intracellular corticosteroid receptors in the house sparrow brain. J. Neuroendocrinol. 13, 412–420. doi: 10.1046/j.1365-2826.2001.00646.x
Breuner, C. W., and Hahn, T. P. (2003). Integrating stress physiology, environmental change, and behavior in free-living sparrows. Horm. Behav. 43, 115–123. doi: 10.1016/S0018-506X(02)00020-X
Breuner, C. W., Sprague, R. S., Patterson, S. H., and Woods, H. A. (2013). Environment, behavior and physiology: do birds use barometric pressure to predict storms? J. Exp. Biol. 216, 1982–1990. doi: 10.1242/jeb.081067
Broggi, J., Nilson, J. F., Koivula, K., Hohtola, E., and Nilsson, J. A. (2019). Mass or pace? Seasonal energy management in wintering boreal passerines. Oecologia 189, 339–351. doi: 10.1007/s00442-018-04332-6
Busch, D. S., Sperry, T. S., Peterson, E., Do, C.-T., Wingfield, J. C., and Boyd, E. H. (2008a). Impacts of frequent, acute pulses of corticosterone on condition and behavior of Gambel’s white-crowned sparrow (Zonotrichia leucophrys gambelii). Gen. Comp. Endocrinol. 158, 224–233. doi: 10.1016/j.ygcen.2008.07.010
Busch, D. S., Sperry, T. S., Wingfield, J. C., and Boyd, E. H. (2008b). Effects of repeated, short-term, corticosterone administration on the hypothalamo-pituitary-adrenal axis of the white-crowned sparrow (Zonotrichia leucophrys gambelii). Gen. Comp. Endocrinol. 158, 211–223. doi: 10.1016/j.ygcen.2008.06.004
Butler, P. J., Green, J. A., Boyd, I. L., and Speakman, J. R. (2004). Measuring metabolic rate in the field: the pros and cons of the doubly labelled water and heart rate methods. Funct. Ecol. 18, 168–183. doi: 10.1111/j.0269-8463.2004.00821.x
Buttemer, W. A., Astheimer, L. B., and Wingfield, J. C. (1991). The effect of corticosterone on standard metabolic rates of small passerine birds. J. Comp. Physiol. B 161, 427–431. doi: 10.1007/BF00260804
Chilton, G., Baker, M. C., Barrentine, C. D., and Cunninghan, M. A. (1995). “White-crowned Sparrow (Zonotrichia leucophrys),” in The Birds of North America Online, ed. A. Poole (Ithaca, NY: Cornell Lab of Ornithology).
Culik, B., Woakes, A. J., Adelung, D., Wilson, R. P., Coria, N. R., and Spairani, H. J. (1990). Energy requirements of Adélie penguin (Pygoscelis adeliae) chicks. J. Comp. Physiol. B 160, 61–70. doi: 10.1007/BF00258763
Cyr, N. E., Wikelski, M., and Romero, L. M. (2008). Increased energy expenditure but decreased stress responsiveness during molt. Physiol. Biochem. Zool. 81, 452–462. doi: 10.1086/589547
de Bruijn, R., and Romero, L. M. (2018). The role of glucocorticoids in the vertebrate response to weather. Gen. Comp. Endocrinol. 269, 11–32. doi: 10.1016/j.ygcen.2018.07.007
Dorn, S., Wascher, C. A. F., Möstl, E., and Kotrschal, K. (2014). Ambient temperature and air pressure modulate hormones and behaviour in raylag geese (Anser anser) and northern bald ibis (Geronticus eremita). Behav. Process. 108, 27–35. doi: 10.1016/j.beproc.2014.08.026
Duclos, M., Gatti, C., Bessière, B., and Mormède, P. (2009). Tonic and phasic effects of corticosterone on food restriction-induced hyperactivity in rats. Psychoneuroendocrinology 34, 436–445. doi: 10.1016/j.psyneuen.2008.10.008
Fahlman, A., Handrich, Y., Woakes, A. J., Bost, C.-A., Holder, R., Duchamp, C., et al. (2004). Effect of fasting on the VO2-fh relationship in king penguins, Aptenodytes patagonicus. Am. J. Physiol. Regul. Integr. Comp. Physiol. 287, R870–R877. doi: 10.1152/ajpregu.00651.2003
Flynn, R. K., and Gessaman, J. A. (1979). An evaluation of heart rate as a measure of daily metabolism in pigeons (Columba livia). Comp. Biochem. Physiol. A Physiol. 63, 511–514. doi: 10.1016/0300-9629(79)90185-3
Frigerio, D. (2004). Excreted corticosterone metabolites co-vary with ambient temperature and air pressure in male raylag geese (Anser anser). Gen. Comp. Endocrinol. 137, 29–36. doi: 10.1016/j.ygcen.2004.02.013
Gessaman, J. A. (1980). An evaluation of heart rate as an indirect measure of daily energy metabolism of the American kestrel. Comp. Biochem. Physiol. A Physiol. 65, 273–289. doi: 10.1016/0300-9629(80)90030-4
González-Gómez, P. L., Ricote-Martinez, N., Razeto-Barry, P., Cotorás, I. S., and Bozinovic, F. (2011). Thermoregulatory cost affects territorial behavior in hummingbirds: a model and its application. Behav. Ecol. Sociobiol. 65, 2141–2148. doi: 10.1007/s00265-011-1222-2
Goymann, W., Trappschuh, M., Jensen, W., and Schwabl, I. (2006). Low ambient temperature increases food intake and dropping production, leading to incorrect estimates of hormone metabolite concentrations in European stonechats. Horm. Behav. 49, 644–653. doi: 10.1016/j.yhbeh.2005.12.006
Green, J. A. (2011). The heart rate method for estimating metabolic rate: review and recommendations. Comp. Biochem. Physiol. A Mol. Integr. Physiol. 158, 287–304. doi: 10.1016/j.cbpa.2010.09.011
Greenlaw, J. S. (1977). Taxonomic distribution, origin, and evolution of bilateral scratching in ground-feeding birds. Condor 79, 426–439. doi: 10.2307/1367722
Grubb, B., Jorgensen, D. D., and Conner, M. (1983). Cardiovascular changes in the exercising emu. J. Exp. Biol. 104, 193–201. doi: 10.1242/jeb.104.1.193
Hailman, J. P. (1984). Effect of litter on leaf-scratching in emberizines. Wilson Bull. 96, 121–125.
Hawkins, P., Butler, P., Woakes, A., and Speakman, J. (2000). Estimation of the rate of oxygen consumption of the common eider duck (Somateria mollissima), with some measurements of heart rate during voluntary dives. J. Exp. Biol. 203, 2819–2832. doi: 10.1242/jeb.203.18.2819
Huber, S., Palme, R., and Arnold, W. (2003). Effects of season, sex, and sample collection on concentrations of fecal cortisol metabolites in red deer (Cervus elaphus). Gen. Comp. Endocrinol. 130, 48–54. doi: 10.1016/S0016-6480(02)00535-X
Johns, D. W., Marchant, T. A., Fairhurst, G. D., Speakman, J. R., and Clark, R. G. (2018). Biomarker of burden: feather corticosterone reflects energetic expenditure and allostatic overload in captive waterfowl. Funct. Ecol. 32, 345–357. doi: 10.1111/1365-2435.12988
Kelley, P., and Bisson, I.-A. (2009). Vireo Software. Available online at: https://web.archive.org/web/20210512024554/https://www.isabellebisson.com/software–applications (accessed March 25).
King, J. R. (1964). Oxygen consumption and body temperature in relation to ambient temperature in the white-crowned sparrow. Comp. Biochem. Physiol. 12, 13–24. doi: 10.1016/0010-406X(64)90044-1
Kitaysky, A. S., Wingfield, J. C., and Piatt, J. F. (2001a). Corticosterone facilitates begging and affects resource allocation in the black-legged kittiwake. Behav. Ecol. 12, 619–625. doi: 10.1093/beheco/12.5.619
Kitaysky, A. S., Kitaiskaia, E. V., Wingfield, J. C., and Piatt, J. F. (2001b). Dietary restriction causes chronic elevation of corticosterone and enhances stress response in red-legged kittiwake chicks. J. Comp. Physiol. B 171, 701–709. doi: 10.1007/s003600100230
Knutie, S. A., and Pereyra, M. E. (2012). A comparison of winter stress responses in cardueline finches. Auk 129, 479–490. doi: 10.1525/auk.2012.11241
Kooyman, G. L., and Ponganis, P. J. (1994). Emperor penguin oxygen consumption, heart rate and plasma lactate levels during graded swimming exercise. J. Exp. Biol. 195, 199–209. doi: 10.1242/jeb.195.1.199
Korte, S. M., Koolhaas, J. M., Wingfield, J. C., and McEwen, B. S. (2005). The Darwinian concept of stress: benefits of allostasis and costs of allostatic load and the trade-offs in health and disease. Neurosci. Biobehav. Rev. 29, 3–38. doi: 10.1016/j.neubiorev.2004.08.009
Krause, J. S., McGuigan, M. A., Bishop, V. R., Wingfield, J. C., and Meddle, S. L. (2015). Decreases in mineralocorticoid but not glucocorticoid receptor mRNA expression during the short arctic breeding season in free-living Gambel’s white-crowned sparrow (Zonotrichia leucophrys gambelii). J. Neuroendocrinol. 27, 66–75. doi: 10.1111/jne.12237
Lattin, C. R., and Romero, L. M. (2014). Seasonal variation in glucocorticoid and mineralocorticoid receptors in metabolic tissues of the house sparrow (Passer domesticus). Gen. Comp. Endocrinol. 14, 95–102. doi: 10.1016/j.ygcen.2014.05.033
Lighton, J. R. B. (2008). Measuring Metabolic Rates: A Manual for Scientists. New York, NY: Oxford University Press.
Lobato, E., Merino, S., Moreno, J., Morales, J., Tomás, G., Martínez- de la Puente, J., et al. (2008). Corticosterone metabolites in blue tit and pied flycatcher droppings: effects of brood size, ectoparasites and temperature. Horm. Behav. 53, 295–305. doi: 10.1016/j.yhbeh.2007.10.010
Lynn, S. E., Breuner, C. W., and Wingfield, J. C. (2003). Short-term fasting affects locomotor activity, corticosterone, and corticosterone binding globulin in a migratory songbird. Horm. Behav. 43, 150–157. doi: 10.1016/S0018-506X(02)00023-5
Maney, D. L., and Wingfield, J. C. (1998). Neuroendocrine suppression of female courtship in a wild passerine: corticotropin-releasing factor and endogenous opioids. J. Neuroendocrinol. 10, 593–599. doi: 10.1046/j.1365-2826.1998.00238.x
Marinelli, M., Rougé-Pont, F., Deroche, V., Barrot, M., Jésus-Oliveira, C. D., Moal, M. L., et al. (1997). Glucocorticoids and behavioral effects of psychostimulants. I: locomotor response to cocaine depends on basal levels of glucocorticoids. J. Pharmacol. Exp. Ther. 281, 1392–1400.
McEwen, B. S., and Wingfield, J. C. (2003). The concept of allostasis in biology and biomedicine. Horm. Behav. 43, 2–15. doi: 10.1016/S0018-506X(02)00024-7
McKechnie, A. E., and Lovegrove, B. G. (2011). Avian facultative hypothermic responses: a review. Condor 104, 705–724. doi: 10.1093/condor/104.4.705
Mendel, C. M. (1989). The free hormone hypothesis: a physiologically based mathematical model. Endocr. Rev. 10, 232–274. doi: 10.1210/edrv-10-3-232
Morley, J. E., and Levine, A. S. (1982). Corticotrophin releasing factor, grooming and ingestive behavior. Life Sci. 31, 1459–1464. doi: 10.1016/0024-3205(82)90007-8
Nephew, B. C., and Romero, L. M. (2003). Behavioral, physiological, and endocrine responses of starlings to acute increases in density. Horm. Behav. 44, 222–232. doi: 10.1016/j.yhbeh.2003.06.002
Ninnes, C. E., Waas, J. R., Ling, N., Nakagawa, S., Banks, J. C., Bell, D. G., et al. (2010). Comparing plasma and fecal measures of steroid hormones in Adelie penguins Pygoscelis adeliae. J. Comp. Physiol, B. 180, 83–94. doi: 10.1007/s00360-009-0390-0
Nolet, B. A., Butler, P. J., Masman, D., and Woakes, A. J. (1992). Estimation of daily energy expenditure from heart rate and doubly labeled water in exercising geese. Physiol. Zool. 65, 1188–1216. doi: 10.1086/physzool.65.6.30158275
Packard, G. C., and Boardman, T. J. (1999). The use of percentages and size-specific indices to normalize physiological data for variation in body size: wasted time, wasted effort? Comp. Biochem. Physiol. A Mol. Integr. Physiol. 122, 37–44. doi: 10.1016/S1095-6433(98)10170-8
Pérez, J. H., Swanson, R. E., Lau, H. J., Cheah, J., Bishop, V. R., Snell, K. R. S., et al. (2020). Tissue-specific expression of 11β-HSD and its effects on plasma corticosterone during the stress response. J. Exp. Biol. 223:jeb209346. doi: 10.1242/jeb.209346
Portugal, S. J., Green, J. A., Cassey, P., Frappell, P. B., and Butler, P. J. (2009). Predicting the rate of oxygen consumption from heart rate in barnacle geese Branta leucopsis: effects of captivity and annual changes in body condition. J. Exp. Biol. 212, 2941–2948. doi: 10.1242/jeb.034546
Raim, A. (1978). A radio transmitter attachment for small passerine birds. Bird Band. 1978, 326–332. doi: 10.2307/4512391
Ramenofsky, M., Gray, J. M., and Johnson, R. B. (1992). Behavioral and physiological adjustments of birds living in winter flocks. Ornis Scand. Scand. J. Ornithol. 23, 371–380. doi: 10.2307/3676663
Raouf, S. A., Smith, L. C., Brown, M. B., Wingfield, J. C., and Brown, C. R. (2006). Glucocorticoid hormone levels increase with group size and parasite load in cliff swallows. Anim. Behav. 71, 39–48. doi: 10.1016/j.anbehav.2005.03.027
Rogers, C. M., Ramenofsky, M., Ketterson, E. D., Nolan, V., and Wingfield, J. C. (1993). Plasma corticosterone, adrenal mass, winter weather, and season in nonbreeding populations of dark-eyed juncos (Junco hyemalis hyemalis). Auk 110, 279–285.
Rohwer, S., and Wingfield, J. C. (1981). A field study of social dominance, plasma levels of luteinizing hormone and steroid hormones in wintering Harris’ sparrows. Z. Für Tierpsychol. 57, 173–183. doi: 10.1111/j.1439-0310.1981.tb01321.x
Romero, L. M., Reed, J. M., and Wingfield, J. C. (2000). Effects of weather on corticosterone responses in wild free-living passerine birds. Gen. Comp. Endocrinol. 118, 113–122. doi: 10.1006/gcen.1999.7446
Romero, L. M., and Wingfield, J. C. (2016). Tempests, Poxes, Predators, and People: Stress in Wild Animals and How they Cope. Oxford: Oxford University Press.
Rose, N., Hancock Snusz, G., Brown, D. M., and Parsons, E. C. M. (2017). Improving captive marine mammal welfare in the United States: science-based recommendations for improved regulatory requirements for captive marine mammal care. J. Int. Wildlife Law Policy 20, 38–72. doi: 10.1080/13880292.2017.1309858
Schwabl, H., Wingfield, J. C., and Farner, D. S. (1985). Influence of winter on endocrine state and behavior in European blackbirds (Turdus merula). Z. Für Tierpsychol. 68, 244–252. doi: 10.1111/j.1439-0310.1985.tb00127.x
Sesti-Costa, R., Ignacchiti, M. D. C., Chedraoui-Silva, S., Marchi, L. F., and Mantovani, B. (2012). Chronic cold stress in mice induces a regulatory phenotype in macrophages: correlation with increased 11β-hydroxysteroid dehydrogenase expression. Brain. Behav. Immun. 26, 50–60. doi: 10.1016/j.bbi.2011.07.234
Sheriff, M. J., Krebs, C. J., and Boonstra, R. (2010). Assessing stress in animal populations: do fecal and plasma glucocorticoids tell the same story? Gen. Comp. Endocrinol. 166, 614–619. doi: 10.1016/j.ygcen.2009.12.017
Smith, G. T., Wingfield, J. C., and Veit, R. R. (1994). Adrenocortical response to stress in the common diving petrel, Pelecanoides urinatrix. Physiol. Zool. 67, 526–537. doi: 10.1086/physzool.67.2.30163862
Speakman, J. R. (2014). Should we abandon indirect calorimetry as a tool to diagnose energy expenditure? Not yet. Perhaps not ever. Commentary on Burnett and Grobe (2014). Mol. Metab. 3, 342–344. doi: 10.1016/j.molmet.2014.04.003
Steiger, S. S., Kelley, J. P., Cochran, W. W., and Wikelski, M. (2009). Low metabolism and inactive lifestyle of a tropical rain forest bird investigated via heart-rate telemetry. Physiol. Biochem. Zool. 82, 580–589. doi: 10.1086/605336
Storch, S., Gremillet, D., and Culik, B. M. (1999). The telltale heart: a non-invasive method to determine the energy expenditure of incubating great cormorants Phalacrocorax carbo carbo. Ardea 87, 207–215.
Tieleman, B. I., and Williams, J. B. (2000). The adjustment of avian metabolic rates and water fluxes to desert environments. Physiol. Biochem. Zool. 73, 461–479. doi: 10.1086/317740
Tomlinson, S., Arnall, S. G., Munn, A., Bradshaw, S. D., Maloney, S. K., Dixon, K. W., et al. (2014). Applications and implications of ecological energetics. Trends Ecol. Evol. 29, 280–290. doi: 10.1016/j.tree.2014.03.003
Vézina, F., Speakman, J. R., and Williams, T. D. (2006). Individually variable energy management strategies in relation to energetic costs of egg production. Ecology 87, 2447–2458. doi: 10.1890/0012-9658(2006)87[2447:ivemsi]2.0.co;2
Walsberg, G. E., and Hoffman, T. C. M. (2005). Direct calorimetry reveals large errors in respirometric estimates of energy expenditure. J. Exp. Biol. 208, 1035–1043. doi: 10.1242/jeb.01477
Walsberg, G. E., and Hoffman, T. C. M. (2006). Using direct calorimetry to test the accuracy of indirect calorimetry in an ectotherm. Physiol. Biochem. Zool. 79, 830–835. doi: 10.1086/505514
Ward, S., Bishop, C. M., Woakes, A. J., and Butler, P. J. (2002). Heart rate and the rate of oxygen consumption of flying and walking barnacle geese (Branta leucopsis) and bar-headed geese (Anser indicus). J. Exp. Biol. 205, 3347–3356. doi: 10.1242/jeb.205.21.3347
Wascher, C. A. F., Kotrschal, K., and Arnold, W. (2018). Free-living greylag geese adjust their heart rates and body core temperatures to season and reproductive context. Sci. Rep. 8:2142. doi: 10.1038/s41598-018-20655-z
Weimerskirch, H., Shaffer, S. A., Mabille, G., Martin, J., Boutard, O., and Rouanet, J. L. (2002). Heart rate and energy expenditure of incubating wandering albatrosses: basal levels, natural variation, and the effects of human disturbance. J. Exp. Biol. 205, 475–483. doi: 10.1242/jeb.205.4.475
White, C. R., and Kearney, M. R. (2014). Metabolic scaling in animals: methods, empirical results, and theoretical explanations. Compr. Physiol. 4, 231–256. doi: 10.1002/cphy.c110049
Wiersma, P., Salomons, H. M., and Verhulst, S. (2005). Metabolic adjustments to increasing foraging costs of starlings in a closed economy. J. Exp. Biol. 208, 4099–4108. doi: 10.1242/jeb.0185
Wingfield, J. C. (2018). Environmental endocrinology: insights into the diversity of regulatory mechanisms in life cycles. Integr. Comp. Biol. 58, 790–799. doi: 10.1093/icb/icy081
Wingfield, J. C., Hahn, T. P., Maney, D. L., Schoech, S. J., Wada, M., and Morton, M. L. (2003). Effects of temperature on photoperiodically induced reproductive development, circulating plasma luteinizing hormone and thyroid hormones, body mass, fat deposition and molt in mountain white-crowned sparrows, Zonotrichia leucophrys oriantha. Gen. Comp. Endocrinol. 131, 143–158. doi: 10.1016/S0016-6480(02)00648-2
Wingfield, J. C., Hahn, T. P., Wada, M., Astheimer, L. B., and Schoech, S. (1996). Interrelationship of day length and temperature on the control of gonadal development, body bass, and fat score in white-crowned sparrows, Zonotrichia leucophrys gambelii. Gen. Comp. Endocrinol. 101, 242–255. doi: 10.1006/gcen.1996.0027
Wingfield, J. C., Hahn, T. P., Wada, M., and Schoech, S. J. (1997). Effects of day length and temperature on gonadal development, body mass, and fat depots in white-crowned sparrows, Zonotrichia leucophrys pugetensis. Gen. Comp. Endocrinol. 107, 44–62. doi: 10.1006/gcen.1997.6894
Wingfield, J. C., Kelley, J. P., and Angelier, F. (2011). What are extreme environmental conditions and how do organisms cope with them. Curr. Zool. 57, 363–374. doi: 10.1093/czoolo/57.3.363
Wingfield, J. C., Maney, D. L., Breuner, C. W., Jacobs, J. D., Lynn, S., Ramenofsky, M., et al. (1998). Ecological bases of hormone-behavior interactions: the “emergency life history stage.”. Am. Zool. 38, 191–206. doi: 10.1093/icb/38.1.191
Wingfield, J. C., Moore, M. C., and Farner, D. S. (1983). Endocrine responses to inclement weather in naturally breeding populations of white-crowned sparrows (Zonotrichia leucophrys pugetensis). Auk 100, 56–62. doi: 10.1093/auk/100.1.56
Wingfield, J. C., Pérez, J. H., Krause, J. S., Word, K. R., González-Gómez, P. L., Lisovski, S., et al. (2017). How birds cope physiologically and behaviorally with extreme climatic events. Phil. Trans. R. Soc. B 372:20160140. doi: 10.1098/rstb.2016.0140
Wingfield, J. C., and Ramenofsky, M. (1997). Corticosterone and facultative dispersal in responses to unpredictable events. Ardea 85, 155–166.
Wingfield, J. C., and Ramenofsky, M. (2011). “Hormone-behavior interrelationships of birds in response to weather,” in Advances in the Study of Behavior, eds H. J. Brockman, C. T. Snowdon, and T. J. Roper (Cambridge, MA: Academic Press), 93–188. doi: 10.1016/s1096-4959(01)00540-1
Wingfield, J. C., Smith, J. P., and Farner, D. S. (1982). Endocrine responses of white-crowned sparrows to environmental stress. Condor 84, 399–409. doi: 10.2307/1367443
Wingfield, J. C., Vleck, C. M., and Moore, M. C. (1992). Seasonal changes of the adrenocortical response to stress in birds of the Sonoran desert. J. Exp. Zool. 264, 419–428. doi: 10.1002/jez.1402640407
Woakes, A. J., and Butler, P. J. (1983). Swimming and diving in tufted ducks, Aythya fuligula, with particular reference to heart rate and gas exchange. J. Exp. Biol. 107, 311–329. doi: 10.1242/jeb.107.1.311
Keywords: allostatic load, glucocorticoid, heart rate, energy expenditure, oxygen consumption, temperature
Citation: Word KR, Austin SH and Wingfield JC (2022) Allostatic Load in Gambel’s White Crowned Sparrow, Zonotrichia leucophrys gambelii: Relationships With Glucocorticoids. Front. Ecol. Evol. 10:855152. doi: 10.3389/fevo.2022.855152
Received: 14 January 2022; Accepted: 12 April 2022;
Published: 04 May 2022.
Edited by:
John Cockrem, Massey University, New ZealandReviewed by:
L. Michael Romero, Tufts University, United StatesClaus Bech, Norwegian University of Science and Technology, Norway
Copyright © 2022 Word, Austin and Wingfield. This is an open-access article distributed under the terms of the Creative Commons Attribution License (CC BY). The use, distribution or reproduction in other forums is permitted, provided the original author(s) and the copyright owner(s) are credited and that the original publication in this journal is cited, in accordance with accepted academic practice. No use, distribution or reproduction is permitted which does not comply with these terms.
*Correspondence: John C. Wingfield, amN3aW5nZmllbGRAdWNkYXZpcy5lZHU=