- 1Subterranean Research and Groundwater Ecology (SuRGE) Group, Trace and Environmental DNA (TrEnD) Lab, School of Molecular and Life Sciences, Curtin University, Perth, WA, Australia
- 2Trace and Environmental DNA (TrEnD) Lab, School of Molecular and Life Sciences, Curtin University, Perth, WA, Australia
- 3School of Biological Sciences, The University of Western Australia, Crawley, WA, Australia
- 4Collections and Research Centre, Western Australian Museum, Welshpool, WA, Australia
- 5The Institute for Geoscience Research, School of Earth and Planetary Sciences, Curtin University, Perth, WA, Australia
Groundwater environments interact with and support subterranean biota as well as superficial aquatic and terrestrial ecosystems. However, knowledge of subterranean energy flows remains incomplete. Cross-boundary investigations are needed to better understand the trophic structures of groundwater ecosystems and their reliance on carbon inputs from aboveground. In this study we used carbon and nitrogen stable isotope analyses combined with radiocarbon fingerprints to characterise organic flows in groundwater ecosystems. We coupled these data with DNA metabarcoding of the gut contents of stygofauna to further elucidate organic matter (OM) sources and shifts in diet preferences. Samples were collected from the arid zone Sturt Meadows calcrete aquifer under low rainfall (LR) and high rainfall (HR) conditions. Bayesian modelling of Δ14C, δ13C, and δ15N data indicated that primary consumers (copepods) incorporated mainly particulate organic carbon (POC) under LR but during HR shifted to root derived material (either exudates or direct root grazing). By contrast, diets of secondary consumers (amphipods) were dominated by root material under both LR and HR. Our DNA metabarcoding-based results indicate that amphipods relied primarily on root inputs from perennial trees (likely Eucalyptus and Callitris) during the dry season (LR). Under HR, diets of both amphipods and copepods also included organic material derived from a broad range of more shallow rooted shrubs, and ephemeral herbs and grasses. Our findings illustrate the complexity of functional linkages between groundwater biota and surface terrestrial ecosystems in environments where aboveground productivity, diversity and OM flux to groundwater are intimately linked to often episodic rainfall.
Introduction
Groundwater is the largest active freshwater resource on Earth, and a key component of the global hydrological cycle as well as a matrix for unique and diverse subterranean ecosystems (Gibert et al., 1990; Gleeson et al., 2016). Groundwater also sustains surficial (aboveground) aquatic and terrestrial ecosystems. The ecological connectivity between above- and underground biomes is exemplified by groundwater-dependent ecosystems (GDEs), which rely on either the surface expression or subsurface presence of groundwater to sustain biodiversity, growth and productivity (Gou et al., 2015). GDEs thus encompass diverse environments and associated biotic communities, including riparian and floodplain vegetation, wetlands and estuaries, hyporheic ecosystems, springs, aquifers and caves (Murray et al., 2003). Most studies of GDEs have focused on water fluxes and the maintenance of riparian and floodplain vegetation (e.g., Eamus et al., 2016). While it is often assumed that subterranean fauna in shallow groundwaters are dependent on carbon and nutrient inputs from this vegetation (Venarsky et al., 2022), there have been remarkably few studies that have determined whether particular plant species are more or less important for sustaining subterranean aquatic fauna, or how these may vary seasonally.
Subterranean environments often provide oligotrophic conditions, with total organic C concentrations ∼ 1 mg/L (Saccò et al., 2020a). Consequently, the growth and productivity of aquatic biota (stygofauna) is thought to be largely dependent on external inputs of carbon and nutrients from primary producers at or near the surface (Kløve et al., 2011; Venarsky et al., 2022). Organic matter (OM) in groundwaters is usually derived from the hyporheic and surficial aquatic and terrestrial zones via water flow, percolation, or animals and plant material falling directly into the aquifer (Humphreys, 2006). Surficial vegetation and their associated root systems have long been considered to provide crucial nutrients and carbon to both subterranean micro- (e.g., microbes and protozoans) and macro-organisms (e.g., stygofauna and cave fishes) (Hancock and Boulton, 2008). Root exudates also provide bioavailable carbon for microbes in soil and groundwater (Liu et al., 2019); in particular, aquatic root mats have been observed to provide reliable habitats and food sources for cave invertebrates (Eberhard and Spate, 1995; Jasinska and Knott, 2000). Several rare and endemic stygofaunal species have been discovered amongst tree roots in caves (e.g., Nerilla sp. at Christmas Island, Humphreys and Eberhard, 2001). Further, the density and diversity of the plant species that form root mats correlates to stygofaunal richness/abundance in caves and aquifers (Hoch and Howarth, 1989; English et al., 2000; Perriam et al., 2008; Hick, 2021). However, the trophic links between vegetation assemblages and different stygofaunal groups are unknown.
Worldwide, increasing over-extraction and contamination of groundwater resources coupled with direct and indirect climate change impacts (including but not limited to droughts, altered aquifer recharge, reduced surface productivity, changing dynamics of OM flux, and functional cascade effects) are threatening the complex matrix of habitats and biota associated with GDEs (Mammola et al., 2019; Sánchez-Fernández et al., 2021). It has been estimated that almost two thirds of the world’s largest aquifers (21 out of 37) have exceeded their sustainable limits (Richey et al., 2015). Negative feedbacks result from declines in groundwater quantity and quality further exacerbating declines in the diversity and functioning of surface ecosystems, which in turn alters and restricts groundwater recharge and transport of carbon subsidies to subterranean food webs (Green et al., 2011; Amanambu et al., 2020). These multi-layered pressures are particularly evident in arid regions, which are naturally exposed to high water deficits owing to low rainfall and high evapotranspiration rates (Lian et al., 2021).
Arid regions account for almost a third of the total global land area (31%, Salem, 1989), including almost 70% of the Australian continent (Khan, 2008). Australian arid zones harbour a vast range of groundwater ecosystems, including the Pilbara region of north-western Australia, which is estimated to host the highest stygofaunal diversity in the world (Saccò et al., 2019a and references therein). The Sturt Meadows calcrete aquifer, also located in inland Western Australia, is arguably one of the most studied groundwater ecosystems in the world, with more than thirty manuscripts published in the last 15 years (Saccò et al., 2019a and references therein). Here, functional ecological modelling has helped elucidate the role of rainfall and aquifer recharge in shaping microbial diversity and metabolisms, together with stygofaunal niche occupation trends and food web interactions (Saccò et al., 2019b, 2020a,b,2021a). However, baseline information on the role of surficial vegetation in mediating organic inputs across the ecosystem is still lacking. In this study, we use state-of-the-art isotope analyses (Bayesian modelling involving three proxies: Δ14C, δ13C, and δ15N) coupled with molecular approaches (DNA metabarcoding on the guts of primary and secondary consumers) to unravel the consumption of surficial vegetation by stygofaunal communities over two contrasting rainfall regimes at the Sturt Meadows aquifer. Our broad objective is to develop a more holistic understanding of the ecological functioning of groundwater communities by widening knowledge of terrestrial inputs in the aquifer and improving the accuracy of ecological modelling at Sturt Meadows. Specifically, we seek to: (i) quantify the proportion of terrestrial vegetation within diets of stygofaunal copepods and amphipods, (ii) characterise the diversity of plant-derived material in guts of primary (copepods) and secondary (amphipods) consumers, and (iii) investigate how plant-based sources differed between periods of low versus high rainfall.
Materials and Methods
Detailed description of the study area, fieldwork procedures, sample preparation and pre-treatments, and isotopic (Δ14C, δ13C, and δ15N) and molecular analyses (DNA metabarcoding on guts) can be found in Saccò et al. (2019b, 2021a). In brief, stygofaunal plant-derived dietary proportions were estimated by using previously published data through conventional stable isotope analysis (SIA; δ13C and δ15N, from Saccò et al., 2019b) but coupled with radiocarbon data (Δ14C, from Saccò et al., 2021a). This analytical design allows greater discrimination in carbon fingerprints between sources and consumers than stable isotopes alone (Larsen et al., 2018). DNA metabarcoding of stygofaunal plant-derived gut contents was undertaken for this study and the new data generated allows for presence-absence diet characterisations of food sources. All the analyses were repeated for low (LR) and high (HR) rainfall sample batches to compare trends across contrasting rainfall periods.
Fieldwork and Sample Preparation
Fieldwork was carried out at the Sturt Meadows calcrete aquifer, in the northeast Yilgarn, Western Australia (28°41′ S 120°58′ E, Figure 1A). The aquifer is comprised of a calcrete deposit developed via precipitation of groundwater calcium carbonate in palaeodrainage channels during the Late Eocene to Early Oligocene (37–30 Mya) (Morgan, 1993). The surrounding area is dominated by unconsolidated Quaternary surficial deposits (Saccò et al., 2020b). Two main types of geological units can be distinguished at the study area: calcretes and clayey formations (see Saccò et al., 2020a for further details). Lithological profiles show topsoil over vadose calcrete. The latter extends from the surface to the water table and overlies phreatic calcrete containing groundwater. This in turn sits above and is associated with sands and clays which also act as an aquifer (Bradford, 2010).
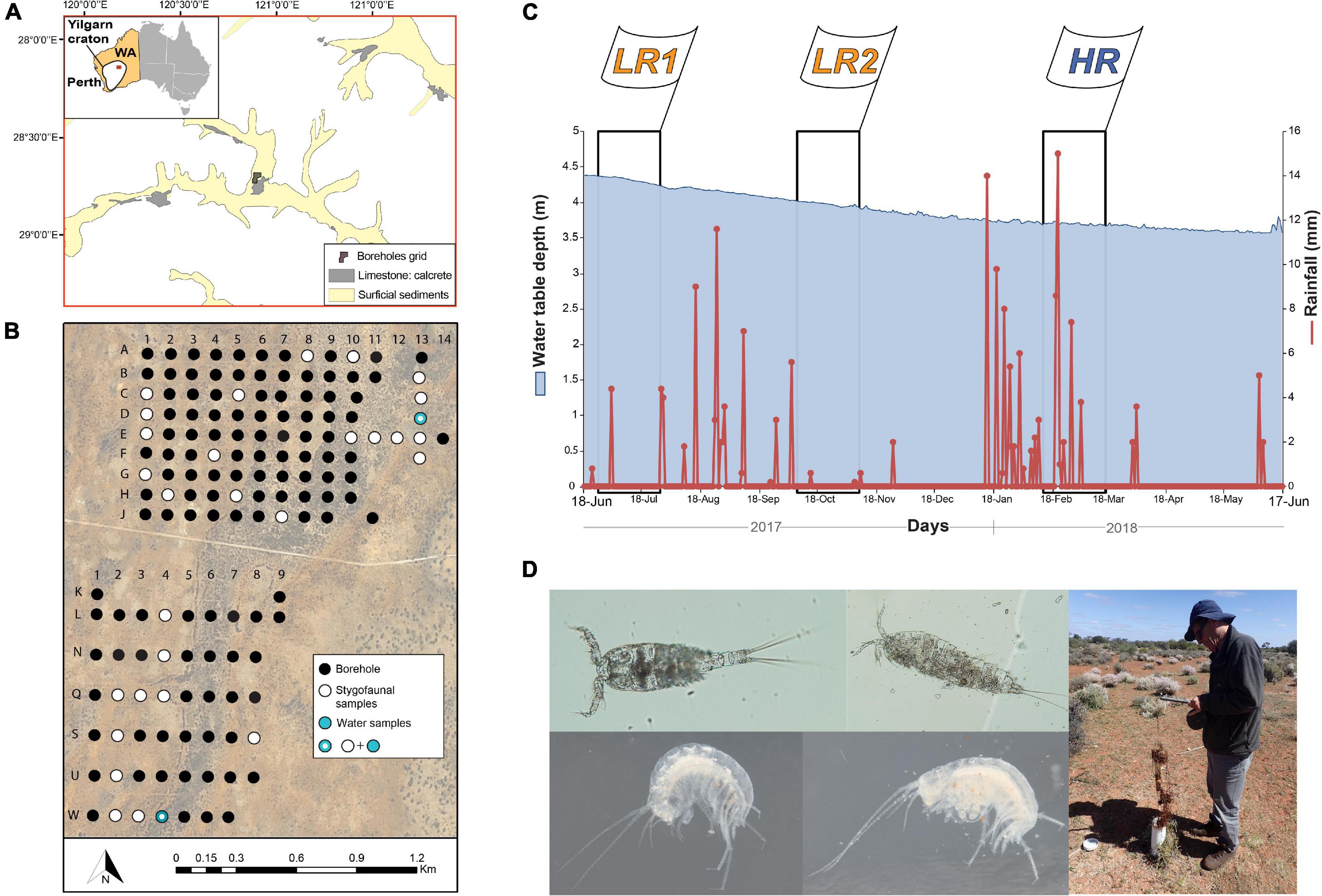
Figure 1. Location the Sturt meadows aquifer (A) and illustration of the borehole grid and samples collected (B); weather station data from bore E7 adapted from Saccò et al. (2020b) (C) and (D) photographs illustrating specimens belonging to the taxa Copepoda Cyclopoida (C, top left), Copepoda Harpacticoida (H, top middle), Amphipoda Scutachiltonia axfordi King, 2012 (AM1, bottom left) and Amphipoda Yilgarniella sturtensis (AM2, bottom middle), and also the root material obtained through sample collection in the field (right). Ground water levels (in mm) are illustrated in light blue and rainfall events (in mm) are shown in red in Figure 1C; flags indicate the dates of LR1 (26/07/2017), LR2 (07/11/2017), and HR (17/03/2018) campaigns, and the black rectangles illustrate the cumulative rainfall and water depth trend for the 30 days before samplings [for rainfall periods category thresholds refer to Hyde et al. (2018)].
The Sturt Meadows aquifer is very shallow (2–4 m below the surface) and readily accessible through a bore grid comprised of 115 bore holes (Figure 1B). The mean permeability of the calcrete is similar to that of sand (1.9–4.6 × 10–4m s–1; Anaconda, 2001), suggesting an average porosity of ∼25% (Allford et al., 2008). Sampling occurred over three campaigns belonging to two different hydrological seasons as described by Hyde et al. (2018): low rainfall (LR – LR1: 26/07/2017; LR2: 07/11/2017) and high rainfall (HR – 17-18/03/2018) (Figure 1C).
The surface vegetation of the broader area is dominated by open Acacia woodlands, primarily Acacia aneura (F.Muell. ex Benth.; family Fabaceae) interspersed with lowland shrubs and grasses (Poaceae) (Allford et al., 2008). Occasional eucalypts and Melaleuca (Myrtaceae) are found in creek zones (Hamano et al., 2011). The calcrete formation at Sturt Meadows sits upstream of a salt lake, Lake Raeside, which like many lakes within the region is surrounded by stands of Callitris columellaris (e.g., O’Donnell et al., 2021).
Stygofaunal specimens were collected from the bores by hauling a weighted plankton net (mesh 100 μm) five times through the water column following the site-specific procedure published by Allford et al. (2008). Four crustacean taxa were considered for the present study: Copepoda Cyclopoida (C) and Copepoda Harpacticoida (H) - both primary consumers - and Amphipoda Scutachiltonia axfordi King, 2012 (AM1) and Amphipoda Yilgarniella sturtensis King, 2012 (AM2) – previously identified as secondary consumers (Saccò et al., 2021a; Figure 1D). All biological and water samples were kept frozen (−20°C) in darkness until laboratory processing. The specimens were counted and identified via optical microscopy and specific taxonomic keys.
To investigate direct potential sources of plant-derived OM in the aquifer, we separated plant root material from water samples (Figure 1D) and sediment during the sorting of fauna in the laboratory. Each sample was pooled according to sampling campaign (LR1, LR2 – merged as LR - or HR). For particulate organic carbon (POC), 2 L of water were collected from bores D13 and W4 (Figure 1B) and then filtered through pre-combusted GF/F filters. POC was analysed for 14C, δ13C, and δ15N as described in Saccò et al. (2021a).
Stable Isotope and Radiocarbon Analyses
δ13C and δ15N SIA of bulk homogenised samples of sediment, roots, POC and stygofauna (AM1, AM2, C, and H) were analysed with a continuous flow isotope ratio mass spectrometer (CF-IRMS, Delta V Plus, Thermo Scientific Corporation, Madison, WI, United States), interfaced with an elemental analyser (Thermo Fisher Flash 2000 HT EA, Thermo Electron Corporation, United States). These tests were performed at the Australian Nuclear Science and Technology Organisation (ANSTO, Sydney) (see Saccò et al., 2019b for further details). 14C content of the same samples was determined using the accelerator mass spectrometry (AMS) STAR Facility at ANSTO (Sydney) (see Saccò et al., 2020c, 2021a for further details).
Gut DNA Metabarcoding
Specimens of AM1, AM2, C, and H were used for gut DNA metabarcoding analysis targeting plant material (primer trnL g-h). Prior to DNA extraction, stygofaunal animals (three to five individuals per pool; n = 84) were placed in petri dishes containing ultrapure water and UV sterilized in a UV oven for 10 min to eliminate any environmental DNA species that may be contained on the exoskeleton. Details on the DNA extraction, controls, sequencing and blasting for stygofaunal gut analysis are described in detail in Saccò et al. (2021a). A minimum threshold of five OTU counts was established for the presence-absence analysis. Only taxa known to occur in the Goldfields Region of Western Australia (Florabase; Western Australian Herbarium, 1988) were considered in this study.
Statistical Analyses
Relative contributions from dietary items were estimated using SIMM (Stable Isotope Mixing Models, R-package “simmr”). We used a triple proxy approach (δ13C, δ15N, and Δ14C) as described in Saccò et al. (2020c) coupled with carbon and nitrogen concentration data as priors (see Supplementary Table 1 for details). Specific trophic discrimination factors have not yet been determined for stygofauna. We thus used the widely accepted discrimination values of 3.46 ± 2‰ for nitrogen and 0.5 ± 1‰ for carbon for all bulk tissues (Zanden and Rasmussen, 2001). Δ14C is free from isotopic fractionation due to the internal correction by the δ13C of −25‰ (Ishikawa et al., 2013).
Results
Plant-Derived Food Web Incorporations
Primary consumers copepods (C and H) showed distinctive dietary patterns under LR compared to HR. During LR, POC was the most abundant food source (C: 47.1 ± 24.9%; H: 44.8 ± 23.6%), followed by roots (C: 33.7 ± 14.3%; H: 34.9 ± 13.5%) and sediment (C: 19.2 ± 10.8 %; H: 20.3 ± 10.2%) (Figures 2A1,A2), while roots dominated the diets of copepods under HR (C: 63.4 ± 0.9%; H: 59.6 ± 0.8%) (Figures 2B1,B2). By contrast, the base organic source of secondary consumers amphipods (AM1 and AM2) was dominated by roots under both rainfall regimes (LR, AM1: 61 ± 11.8%; AM2: 39.9 ± 9.8%; HR, AM1: 55.1 ± 7.9%; AM2: 65.8 ± 8.9%). The relative proportion of POC was ∼two-fold (from 14.5 to 32.5%) during HR for AM1 when compared to LR (Figures 2A3,A4,B3,B4). Contributions of sediment, cyclopoids and harpacticoids to AM1 and AM2 diets were always below 10%, with the exception of sediment during LR for AM2, which accounted for 22.2 ± 6.9% (Supplementary Table 2). POC contributions across all taxa and rainfall regimes correlated negatively with incorporations of sediment and roots, indicating that the SIMM could not discern between the different sources. In contrast, positive correlations between roots and sediment indicated that stygofauna tend to target either one source or the other (Supplementary Figure 1).
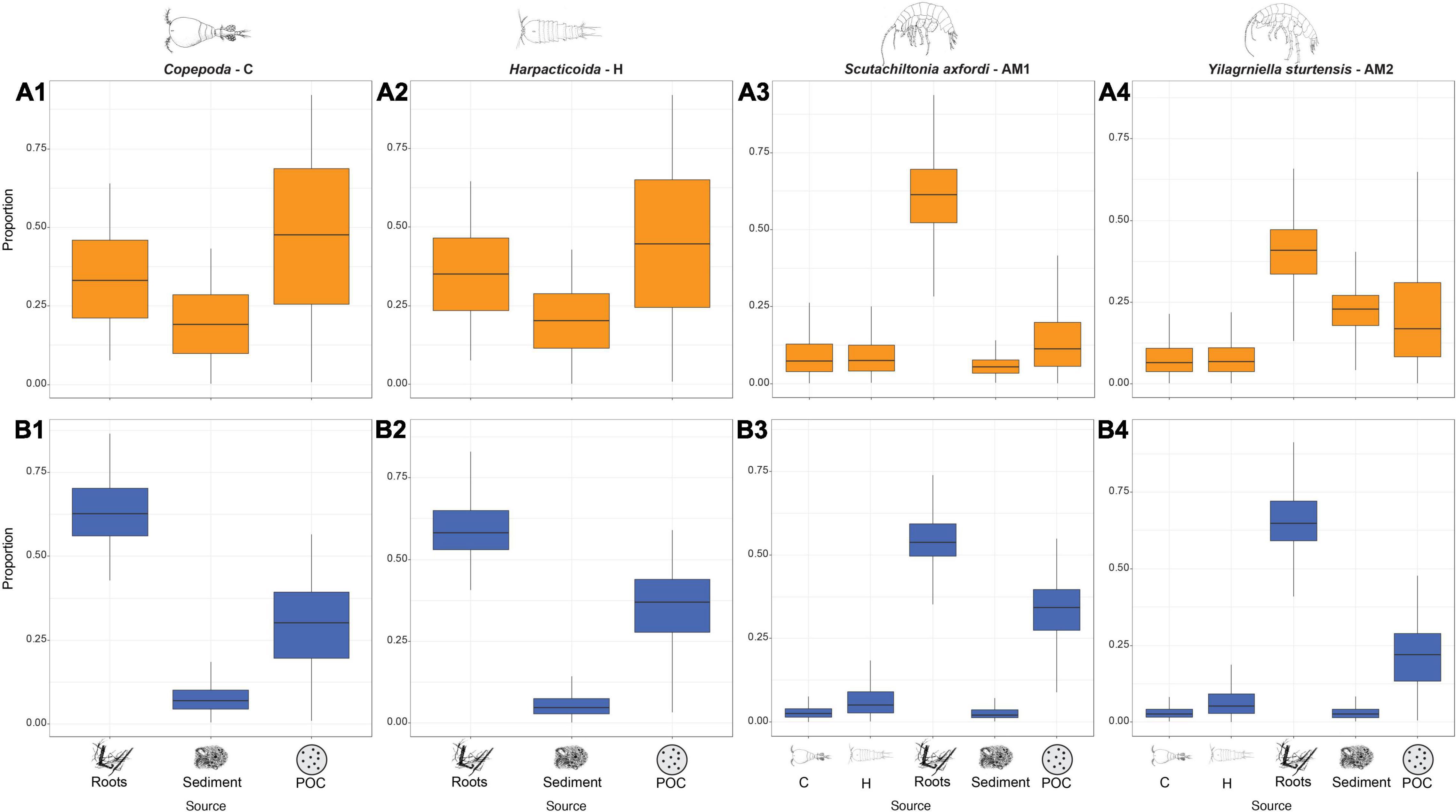
Figure 2. Modelled basal plant-derived OM contributions to the diets of Copepoda Cyclopoida (C), Copepoda Harpacticoida (H), Amphipoda Scutachiltonia axfordi (AM1) and Amphipoda Yilgarniella sturtensis (AM2), during LR (A1–4 respectively) and HR (B1–4 respectively). Cyclopoida and Harpacticoida were included in the diet modelling of AM1 and AM2 because they are potential organic incorporation of amphipods’ diets and were previously considered in Saccò et al. (2019b). See Supplementary Table 1 for the values (mean ± SD) of the estimated contributions of the diet items.
Plant-Based Diet Preferences
DNA metabarcoding of the guts of consumers revealed eight plant families belonging to four life forms (herb, tree, shrub, and grass) varying OM quality across the two rainfall regimes (Table 1). During LR, gut contents of amphipods AM1 and AM2 were dominated by two plant families: Myrtaceae (locally dominated by the species from the genus Eucalyptus) (AM1 and AM2), and Cupressaceae, genus Callitris (AM2). Under HR, amphipod AM1 was associated with four families (Fabaceae, Myrtaceae, Poaceae, and Scrophuliariaceae). By contrast, only two of the grass/herbaceous families (Poaceae and Polygonaceae) were identified in copepods H guts. During HR, Poaceae were detected most frequently (in H, AM1 and AM2), and together with Scrophulariaceae (likely Eremophila species) were the only two families present in more than one stygofaunal taxa at the same time; the rest of the families were uniquely detected in one taxon at a time. In addition, during HR only the family Poaceae was detected both in primary (H) and secondary (AM1 and AM2) consumers; Asteraceae (C), Cupressaceae (C), Polygonaceae (H), and Rutaceae (H) were only detected in primary consumers copepods, and Brassicaceae (AM2), Fabaceae (AM1), Myrtaceae (AM1) and Scrophulariaceae (AM1 and AM2) were detected in secondary consumers amphipods. Overall, long-lived tree life forms (Callitris and Eucalyptus) dominated the stygofaunal gut contents under LR, whilst all the other families characterising life forms other than trees (with the exception of Fabaceae, which include the locally dominant Acacia) were detected exclusively during HR.
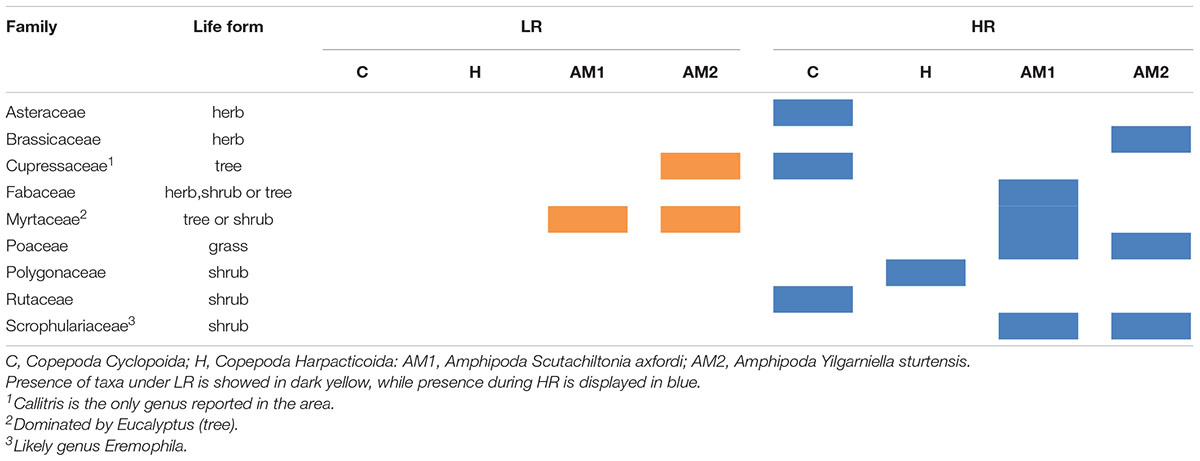
Table 1. Presence-absence data of plant-derived material in stygofaunal guts extrapolated from DNA metabarcoding analyses.
Discussion
This study, to our knowledge, provides the first evidence based on isotopic modelling combined with gut content analysis that surface derived plant-material plays a critical role in sustaining stygofauna of shallow groundwater ecosystems of the arid zone (Figure 3). We found that roots and other plant material constitute a key organic source for the subterranean ecosystem at Sturt Meadows, in an arid and highly dynamic hydrological environment characterised by oligotrophic conditions and truncated food webs due to the lack of autochthonous primary producers (Saccò et al., 2021b). Stygofauna, including oligochaetes, copepods and amphipods, are routinely found amongst submerged roots at Sturt Meadows (Humphreys; personal communication), suggesting that trees provide favourable niches for their biological cycles. This study using gut analyses combined with isotopic modelling confirms that tree roots are both habitat and source of OM for stygofauna, as has been previously suggested in several groundwater ecosystems in inland Western (Jasinska et al., 1996) and Eastern continental Australia (Hancock and Boulton, 2008), as well as Tasmania (Jasinska and Knott, 2000). The native cypress Callitris, a shallow-rooted but very long-lived tree (Cullen and Grierson, 2009; O’Donnell et al., 2021) was detected in amphipods AM2 (LR) and copepods C (HR). Recent investigations of the Sturt Meadows stygofaunal community suggested a substantial shift in the OM incorporated by amphipods (AM1 and AM2) from root-based during LR to a POC-based diet under HR (Saccò et al., 2021a). However, the “triple proxy approach” (δ13C, δ15N, and Δ14C) used in this study indicates that, despite a two-fold increase in the predicted amount of POC incorporated under HR compared to LR, roots constitute the main plant-derived organic source for amphipods under both rainfall regimes. The differences between the findings of the conventional and “triple proxy approach” can be ascribed to the ability of radiocarbon data to improve the tracing of the carbon flows, especially when multiple potential sources are available in the system as a result of seasonal changes (i.e., rainfall-driven subterranean inputs). While comparable results were generated by both approaches under LR, once the system receives mixed terrestrially-derived inflows of OM during HR (see Saccò et al., 2020a), the outputs of the two models differed substantially. Further discussion on the cost-benefit evaluation of the different designs, including the potential incorporation of prior qualitative information in form of DNA metabarcoding data, can be found in Saccò et al. (2020c).
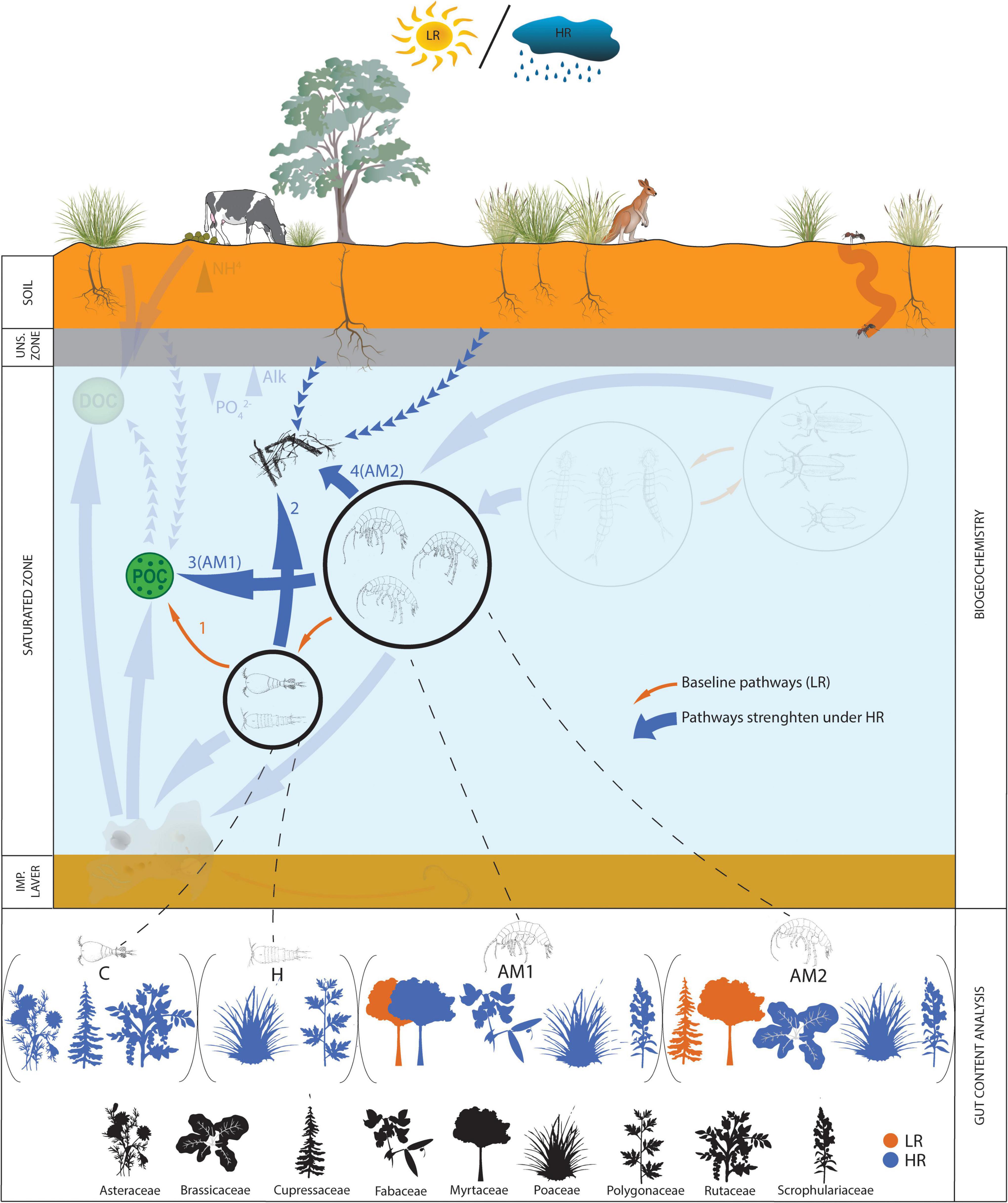
Figure 3. Updated conceptual model from Saccò et al. (2021a), with incorporation of information on the plant-derived consumption of primary (C and H) and secondary consumers (AM1 and AM2). The orange arrows illustrate the baseline biochemical paths which were frequent during LR, while bigger blue arrows underline those transitions strengthened under HR. Transparent figures and arrows refer to dynamics already described in Saccò et al. (2021a). The key biochemical findings are numbered as follow: (1) POC provides the main potential plant-derived organic input in copepods’ diets under LR; (2) under HR, copepods shift toward a diet dominated by roots and associated microbiota; (3) amphipods AM1 increase POC incorporations (∼ two-fold) during HR, while (4) amphipods AM2 increase the proportion of their root-based diets after rainfall (HR). Dashed lines lead to the characterisation (presence of plant families in C, H, AM1 and AM2 under LR and HR) of the plant-derived material identified in stygofaunal guts via DNA metabarcoding analysis.
Interestingly, plant-derived OM incorporated by copepods during LR followed a slightly different trend. POC was the most abundant dietary item during LR, suggesting potential trophic niche partitioning with amphipods (Saccò et al., 2020b). Previous radiocarbon dating of POC under both rainfall regimes showed old (LR: 1160 ± 70 BP; HR: 3605 ± 35 BP, Saccò et al., 2021a) rather than modern inputs (contrarily to roots, see Saccò et al., 2021a), suggesting that ancient carbon in sediment provides the bulk of this organic fraction in the groundwater. However, POC in groundwater is conventionally composed by a mixture of leaves, dead wood, guano, and animal carrion (Venarsky et al., 2022). This was confirmed in our analysis as the SIMM was not able to discriminate between differential consumptions of POC, roots and/or sediment (Supplementary Figure 1). Further in-depth and fine scale investigations on the different POC fractions – coarse (CPOC, >1 mm) and fine (FPOC, 1 mm > FPOC > 0.45 μm) – and relative contributions to stygofaunal diets at Sturt Meadows will help unravel the flows of particulate OM in the aquifer.
This study elucidates the complex matrix of interactions among plants and groundwater ecosystems. Stygofaunal gut contents analysis indicates that OM from phreatophytic trees such as Eucalyptus or Callitris underpin stygofauna diets under both rainfall regimes. Shorter lived species, such as shallow rooted herbs, grasses and shrubs, were only evident in guts after HR. There are possibly two reasons as to why this is the case. First, OM production by grasses and many herbs in the arid zone is confined to after rainy periods. Second, rainfall-driven inputs of these species seem to play a key role in shaping the organic flows at Sturt Meadows (Saccò et al., 2021a). The detection of stygofauna species-specific preferences for particular types and species of plant material suggests that trophic behaviours are shaped by differential nutrient/prey availability. In-depth genomic (i.e., transcriptomics, Beasley-Hall et al., 2021) and genetic (i.e., species-specific eDNA, Saccò et al., 2022) studies are necessary to understand patterns shaping both surficial and subterranean realms. Predicted altered rainfall regimes and increased aridity for the region will likely have an impact on this delicate balance (Lian et al., 2021), affecting both the groundwater-dependent surficial vegetation, and the subterranean biota that depend on plant material as a food source. In arid inland Australia, vegetation is often entirely dependent on groundwater sources, which are increasingly being impacted by resource development e.g., agriculture and mining (Nevill et al., 2010). These regions also often host unique stygofauna (Guzik et al., 2017) that have co-evolved with GDEs and associated natural organic sources. Consequently, future research priorities should focus on the resilience of the subterranean and GDEs to climate change, an impelling task given the uniqueness of these fauna and the risk of their extinction associated with anthropogenic impacts.
Data Availability Statement
The datasets presented in this study can be found in online repositories. The names of the repository/repositories and accession number(s) can be found below: https://datadryad.org/stash, https://doi.org/10.5061/dryad.kkwh70s66.
Author Contributions
MS: conceptualisation, methodology, validation, formal analysis, investigation, writing – original draft, and project administration. MC: investigation, curation, formal analysis, and writing – review and editing. PN and AB: investigation, writing – review and editing, and funding acquisition. WH: investigation and writing – review and editing. PG: resources and writing – review and editing. NW: methodology, validation, investigation, and writing – review and editing. All authors contributed to the article and approved the submitted version.
Funding
MS and NW are supported by the BHP Social Investment Fund, eDNA for Global Biodiversity (eDGES) programme. PN was supported by the Australian Research Council Industrial Transformation Training Centre for Mine Site Restoration (project number ICI150100041). We acknowledge financial support from the Australia Research Council (Linkage Project LP140100555) and the Australian Government’s National Collaborative Research Infrastructure Strategy (NCRIS) for the Centre for Accelerator Science at the Australian Nuclear Science and Technology Organisation. This work was supported by resources provided by the Pawsey Supercomputing Centre with funding from the Australian Government and the Government of Western Australia.
Conflict of Interest
The authors declare that the research was conducted in the absence of any commercial or financial relationships that could be construed as a potential conflict of interest.
Publisher’s Note
All claims expressed in this article are solely those of the authors and do not necessarily represent those of their affiliated organizations, or those of the publisher, the editors and the reviewers. Any product that may be evaluated in this article, or claim that may be made by its manufacturer, is not guaranteed or endorsed by the publisher.
Acknowledgments
We wish to acknowledge the traditional custodians of the land, the Wongai people, and their elders, past, present and emerging. We acknowledge and respect their continuing culture and the contribution they make to the life of the Yilgarn region. We thank Flora, Peter, and Paul Axford of Sturt Meadows Station for their kindness and generosity in providing both accommodation and access to their property. MS was supported by a Curtin International Postgraduate Research Scholarship (CIPRS) and an AINSE postgraduate scholarship (PGRA).
Supplementary Material
The Supplementary Material for this article can be found online at: https://www.frontiersin.org/articles/10.3389/fevo.2022.854591/full#supplementary-material
References
Allford, A., Cooper, S. J., Humphreys, W. F., and Austin, A. D. (2008). Diversity and distribution of groundwater fauna in a calcrete aquifer: does sampling method influence the story? Invert. Syst. 22, 127–138. doi: 10.1071/IS07058
Amanambu, A. C., Obarein, O. A., Mossa, J., Li, L., Ayeni, S. S., Balogun, O., et al. (2020). Groundwater system and climate change: present status and future considerations. J. Hydrol. 589:125163. doi: 10.1016/j.jhydrol.2020.125163
Anaconda (2001). Mt Margaret Nickel Cobalt Project: Public Environment Review and Public Environmental Report. Perth, Australia: Anaconda Nickel Limited.
Beasley-Hall, P. G., Bertozzi, T., Bradford, T. M., Foster, C. S., Jones, K., Tierney, S. M., et al. (2021). Stressed out underground?: Transcriptomics illuminates the genome-wide response to heat stress in surface and subterranean diving beetles. bioRxiv [preprint]. doi: 10.1101/2021.12.12.470823
Bradford, T. M. (2010). Modes of Speciation in Subterranean Diving Beetles from a Single Calcrete Aquifer in Central Western Australia. Doctoral dissertation. Adelaide, SA: University of Adelaide.
Cullen, L. E., and Grierson, P. F. (2009). Multi-decadal scale variability in autumn-winter rainfall in south-western Australia since 1655 AD as reconstructed from tree rings of Callitris columellaris. Clim. Dyn. 33, 433–444. doi: 10.1007/s00382-008-0457-8
Eamus, D., Fu, B., Springer, A. E., and Stevens, L. E. (2016). Groundwater Dependent Ecosystems: Classification, Identification Techniques And Threats Integrated Groundwater Management. Cham: Springer. doi: 10.1007/978-3-319-23576-9_13
Eberhard, S. M., and Spate, A. (1995). Cave Invertebrate Survey: Towards An Atlas Of Nsw Cave Fauna. Report to the Department Of Urban Affairs And Planning And The Australian. Canberra: Heritage Commission.
English, V., Blyth, J., Jasinska, E., Mutter, L., Bastian, L., Holmes, P., et al. (2000). Interim Recovery Plan: Aquatic Root Mat Community of Caves of the Swan Coastal Plain, 2000–2003. Perth, WA: Department of Conservation and Land Management (WA) and Department of Environment and Heritage.
Gibert, J., Dole-Olivier, M. J., Marmonier, P., and Vervier, P. (1990). “Surface water-groundwater ecotones,” in The Ecology and Management of Aquatic-Terrestrial Ecotones, eds R. J. Naiman and H. Décamps (London: UNESCO/Parthenon Publishing), 199–225.
Gleeson, T., Befus, K. M., Jasechko, S., Luijendijk, E., and Cardenas, M. B. (2016). The global volume and distribution of modern groundwater. Nat. Geosci. 9, 161–167. doi: 10.1038/ngeo2590
Gou, S., Gonzales, S., and Miller, G. R. (2015). Mapping potential groundwater-dependent ecosystems for sustainable management. Groundwater 53, 99–110. doi: 10.1111/gwat.12169
Green, T. R., Taniguchi, M., Kooi, H., Gurdak, J. J., Allen, D. M., Hiscock, K. M., et al. (2011). Beneath the surface of global change: impacts of climate change on groundwater. J. Hydrol. 405, 532–560. doi: 10.1016/j.jhydrol.2011.05.002
Guzik, M. T., Austin, A. D., Cooper, S. J., Harvey, M. S., Humphreys, W. F., Bradford, T., et al. (2017). Is the Australian subterranean fauna uniquely diverse? Invert. Syst. 24, 407–418. doi: 10.1071/IS10038
Hamano, H., Saito, N., Tanaka, Y., Kato, S., Tahara, K., Takahashi, N., et al. (2011). Factors affecting distribution of nutrient in tree organs of eucalyptus camaldulensis in arid lands of western australia. J. Chem. Eng. Japan 44, 653–661. doi: 10.1252/jcej.10we237
Hancock, P. J., and Boulton, A. J. (2008). Stygofauna biodiversity and endemism in four alluvial aquifers in eastern Australia. Invert. Syst. 22, 117–126. doi: 10.1071/IS07023
Hick, A. J. (2021). Preliminary Assessment of Subterranean Fauna Habitat for the Gnarabup Tourism Development, Leeuwin-Naturaliste Region, Western Australia. Victoria Park, WA: Director Invertebrate Solutions Pty Ltd.
Hoch, H., and Howarth, F. G. (1989). Six new cavernicolous cixiid planthoppers in the genus solonaima from australia (homoptera: fulgoroidea). Syst. Entomol. 14, 377–402. doi: 10.1111/j.1365-3113.1989.tb00291.x
Humphreys, W. F., and Eberhard, S. (2001). Subterranean fauna of christmas island. indian ocean. Helictite 37, 59–74.
Humphreys, W. F. (2006). Aquifers: the ultimate groundwater-dependent ecosystems. Austr. J. Bot. 54, 115–132. doi: 10.1071/BT04151
Hyde, J., Cooper, S. J., Humphreys, W. F., Austin, A. D., and Munguia, P. (2018). Diversity patterns of subterranean invertebrate fauna inmcalcretes of the yilgarn region. Western Australia. Mar. Fresh. Res. 69, 114–121. doi: 10.1071/MF17005
Ishikawa, N. F., Hyodo, F., and Tayasu, I. (2013). Use of carbon-13 and carbon-14 natural abundances for stream food web studies. Ecol. Res. 28, 759–769. doi: 10.1007/s11284-012-1003-z
Jasinska, E. J., Knott, B., and McComb, A. R. (1996). Root mats in ground water: a fauna-rich cave habitat. J. North Am. Benthol. Soc. 15, 508–519. doi: 10.2307/1467802
Jasinska, E., and Knott, B. (2000). “Root driven faunas in cave waters,” in Ecosystems of the World. Subterranean Ecosystems, eds H. Wilkens, D. Culver, and W. Humphreys (Amsterdam: Elsevier).
Khan, S. (2008). Managing climate risks in Australia: options for water policy and irrigation management. Austr. J. Exp. Agric. 48, 265–273. doi: 10.1071/EA06090
Kløve, B., Ala-Aho, P., Bertrand, G., Boukalova, Z., Ertürk, A., Goldscheider, N., et al. (2011). Groundwater dependent ecosystems. part I: hydroecological status and trends. Environ. Sci. Policy 14, 770–781. doi: 10.1016/j.envsci.2011.04.002
Larsen, T., Yokoyama, Y., and Fernandes, R. (2018). Radiocarbon in ecology: insights and perspectives from aquatic and terrestrial studies. Methods Ecol. Evol. 9, 181–190. doi: 10.1111/2041-210X.12851
Lian, X., Piao, S., Chen, A., Huntingford, C., Fu, B., Li, L. Z., et al. (2021). Multifaceted characteristics of dryland aridity changes in a warming world. Nat. Rev. Earth Environ. 2, 232–250. doi: 10.1038/s43017-021-00144-0
Liu, W., Jones, A. L., Gosse, H. N., Lawrence, K. S., and Park, S. W. (2019). Validation of the chemotaxis of plant parasitic nematodes toward host root exudates. J. Nematol. 51:e2019–2063. doi: 10.21307/jofnem-2019-063
Mammola, S., Cardoso, P., Culver, D. C., Deharveng, L., Ferreira, R. L., Fišer, C., et al. (2019). Scientists’ warning on the conservation of subterranean ecosystems. BioScience 69, 641–650. doi: 10.1093/biosci/biz064
Morgan, K. H. (1993). Development, sedimentation and economic potential of palaeoriver systems of the Yilgarn Craton of Western Australia. Sed. Geol. 85, 637–656. doi: 10.1016/0037-0738(93)90106-F
Murray, B. R., Zeppel, M. J., Hose, G. C., and Eamus, D. (2003). Groundwater-dependent ecosystems in Australia: it’s more than just water for rivers. Ecol. Manage. Restorat. 4, 110–113. doi: 10.1046/j.1442-8903.2003.00144.x
Nevill, J. C., Hancock, P. J., Murray, B. R., Ponder, W. F., Humphreys, W. F., Phillips, M. L., et al. (2010). Groundwater-dependent ecosystems and the dangers of groundwater overdraft: a review and an Australian perspective. Pacific Conserv. Biol. 16, 187–208. doi: 10.1071/PC100187
O’Donnell, A. J., McCaw, W. L., Cook, E. R., and Grierson, P. F. (2021). Megadroughts and pluvials in southwest Australia: 1350–2017 CE. Clim. Dyn. 57, 1817–1831. doi: 10.1007/s00382-021-05782-0
Perriam, J., Tapsuwan, S., Burton, M., and Schilizzi, S. (2008). Value of the Yanchep Caves: Assessing Yanchep National Park Visitor’s Willingness to Pay for Environmental Improvement to the Caves. Canberra: CSIRO: Water for a Healthy Country National Research Flagship.
Richey, A. S., Thomas, B. F., Lo, M. H., Reager, J. T., Famiglietti, J. S., Voss, K., et al. (2015). Quantifying renewable groundwater stress with GRACE. Water Res. Res. 51, 5217–5238. doi: 10.1002/2015WR017349
Saccò, M., Blyth, A., Bateman, P. W., Hua, Q., Mazumder, D., White, N., et al. (2019a). New light in the dark-a proposed multidisciplinary framework for studying functional ecology of groundwater fauna. Sci. Total Environ. 662, 963–977. doi: 10.1016/j.scitotenv.2019.01.296
Saccò, M., Blyth, A. J., Humphreys, W. F., Kuhl, A., Mazumder, D., Smith, C., et al. (2019b). Elucidating stygofaunal trophic web interactions via isotopic ecology. PLos One 14:e0223982. doi: 10.1371/journal.pone.0223982
Saccò, M., Blyth, A., Humphreys, W. F., Middleton, J. A., Campbell, M., Mousavi-Derazmahalleh, M., et al. (2020a). Tracking down carbon inputs underground from an arid zone Australian calcrete. PLoS One 15:e0237730. doi: 10.1371/journal.pone.0237730
Saccò, M., Blyth, A. J., Humphreys, W. F., Karasiewicz, S., Meredith, K. T., Laini, A., et al. (2020b). Stygofaunal community trends along varied rainfall conditions: deciphering ecological niche dynamics of a shallow calcrete in Western Australia. Ecohydrology 13:e2150. doi: 10.1002/eco.2150
Saccò, M., Blyth, A. J., Humphreys, W. F., Cooper, S. J., Austin, A. D., Hyde, J., et al. (2020c). Refining trophic dynamics through multi-factor bayesian mixing models: a case study of subterranean beetles. Ecol. Evolu. 10, 8815–8826. doi: 10.1002/ece3.6580
Saccò, M., Blyth, A. J., Humphreys, W. F., Cooper, S. J., White, N. E., Campbell, M., et al. (2021a). Rainfall as a trigger of ecological cascade effects in an Australian groundwater ecosystem. Sci. Rep. 11, 1–15. doi: 10.1038/s41598-021-83286-x
Saccò, M., Blyth, A. J., Venarsky, M., and Humphreys, W. F. (2021b). Trophic Interactions in Subterranean Environments. Reference Module in Earth Systems and Environmental Sciences. Amsterdam: Elsevier. doi: 10.1016/B978-0-12-819166-8.00064-5
Saccò, M., Guzik, M. T., van der Heyde, M., Nevill, P., Cooper, S. J. B., Austin, A. D., et al. (2022). eDNA in subterranean ecosystems: applications, technical aspects, and future prospects. Sci. Total Environ. 820:153223. doi: 10.1016/j.scitotenv.2022.153223
Salem, B. B. (1989). Arid Zone Forestry: A Guide for Field Technicians. Rome: FAO Conservation Guide.
Sánchez-Fernández, D., Galassi, D. M., Wynne, J. J., Cardoso, P., and Mammola, S. (2021). Don’t forget subterranean ecosystems in climate change agendas. Nat. Clim. Change 11, 458–459. doi: 10.1038/s41558-021-01057-y
Venarsky, M., Kevin, S., Saccò, M., François, C., Simon, L., and Griebler, C. (2022). Groundwater Food Webs. Groundwater Ecology, 2nd Edn. Amsterdam: Elsevier.
Western Australian Herbarium (1988). FloraBase–The Western Australian Flora. Perth, WA: Department of Parks and Wildlife.
Keywords: groundwater, stygofauna, vegetation, organic matter sources, seasonal rainfall, DNA metabarcoding, stable isotope analysis, radiocarbon
Citation: Saccò M, Campbell MA, Nevill P, Humphreys WF, Blyth AJ, Grierson PF and White NE (2022) Getting to the Root of Organic Inputs in Groundwaters: Stygofaunal Plant Consumption in a Calcrete Aquifer. Front. Ecol. Evol. 10:854591. doi: 10.3389/fevo.2022.854591
Received: 14 January 2022; Accepted: 18 February 2022;
Published: 11 March 2022.
Edited by:
Tiziana Di Lorenzo, Research Institute on Terrestrial Ecosystems, National Research Council (CNR), ItalyReviewed by:
Jennifer Lesley Silcock, The University of Queensland, AustraliaSelim Kapur, Çukurova University, Turkey
Benjamin F. Schwartz, Texas State University, United States
Copyright © 2022 Saccò, Campbell, Nevill, Humphreys, Blyth, Grierson and White. This is an open-access article distributed under the terms of the Creative Commons Attribution License (CC BY). The use, distribution or reproduction in other forums is permitted, provided the original author(s) and the copyright owner(s) are credited and that the original publication in this journal is cited, in accordance with accepted academic practice. No use, distribution or reproduction is permitted which does not comply with these terms.
*Correspondence: Mattia Saccò, mattia.sacco@curtin.edu.au