- 1Department of Botany, University of Wisconsin-Madison, Madison, WI, United States
- 2Visiting Researcher, Northwest Fisheries Science Center, NOAA, Seattle, WA, United States
- 3Department of Botany and Arboretum, University of Wisconsin-Madison, Madison, WI, United States
Selectively planting native species could guide ecosystem development toward wetland restoration targets, once we understand how influential species function, alone and in combination. Knowing that Triglochin concinna (arrow grass, Juncaceae) accumulates N in its perennial roots, we asked how it would influence N dynamics on an excavated salt marsh plain at Tijuana Estuary, in southern California. We hypothesized that it would (a) accumulate N in roots and shoots, (b) reduce biomass of other marsh plain plants or, alternatively, (c) share N with neighbors as its litter decomposed and released N. We used 15N stable isotope enrichment to quantify N transfer between Triglochin and the marsh plain’s seven-species halophyte assemblage in field and greenhouse experiments. We also examined the effect of Triglochin on individual marsh plain species’ biomass and N accumulation. Triglochin had low shoot biomass (0.96 ± 0.5 g m−2 in field plots and 17.64 ± 2.2 g m−2 in greenhouse pots), high root:shoot ratios (4.3 in the field and 2.0 in the greenhouse), and high tissue N content (1.9 ± 0.2% in the field and 1.7 ± 0.1% in the greenhouse). Two productive perennials, Sarcocornia pacifica (pickleweed) and Frankenia salina (alkali heath), outgrew Triglochin; yet these biomass dominants produced 44%–45% less shoot biomass in greenhouse pots with Triglochin than without. However, we did not find this reduction in the field where roots were unconfined. In the greenhouse, δ15N values were higher for species grown with 15N-enriched Triglochin, indicating that this species made N available to its neighbors. The δ15N values for plants grown in the field exceeded background levels, also indicating that the marsh plain assemblage took up N released by Triglochin. We conclude that Triglochin can influence the restoration of salt marsh vegetation by accumulating N and releasing its tissue N to neighbors as leaves and roots decompose, while simultaneously reducing the biomass of neighbors. The seasonally deciduous Triglochin is low in shoot biomass, yet competitively superior in N uptake. Because this often-ignored species has limited tidal dispersal, we suggest restoration plantings, including tests of its ability to facilitate diversity where S. pacifica, the marsh plain dominant, might otherwise form monocultures.
Introduction
Coastal wetlands have been degraded for centuries but are being restored globally to regain valued ecosystem services (biodiversity support, erosion control, wildlife habitat, productivity, trophic support, and cultural uses; Gann et al., 2019). In southern California, urbanization, pollution (e.g., 2021 Huntington Beach oil spill), sea level rise, storm surges, and global warming Thorne et al., 2018; Armitage, 2021) continue to threaten remnant and restored salt marshes. Mitigation for impacts involves restoration (and creation) of tidal marshes and endangered species habitat, with strict criteria, but uncertain outcomes (Doherty et al., 2011; Adam, 2019).
Field experiments in wetland remnants and on newly graded marsh plains have revealed halophyte traits and methods for restoration (Zedler, 2001; Bonin and Zedler, 2008; Janousek et al., 2019). Understandably, research has focused on dominant plant species, especially Sarcocornia pacifica Standl. (Salicornia virginica L.) and the dynamics of nitrogen (N), which is often limited in restored marshes (Boyer and Zedler, 1998; Boyer et al., 2001; Ryan and Boyer, 2012; Cott et al., 2018). One method for non-mycorrhizal species to acquire N when planted in nutrient-poor substrates is N transfer from N-rich sources to N-poor tissues (Haystead et al., 1988; Moyer-Henry et al., 2006; He et al., 2009; Teste et al., 2015).
Non-dominant species can play a critical role in restoring N dynamics (Sullivan et al., 2007). Species with high N tissue content can accumulate biomass over time and strongly influence nutrient dynamics through seasonal uptake and release (Berendse, 1994). In southern California, Triglochin concinna Davy (Juncaceae, hereafter Triglochin), could be playing such a role. This subordinate species has perennial roots and rhizomes, with deciduous shoots. Its leaves emerge in fall and reach peak biomass by late spring; it flowers and fruits between January and June, and shoots die back by midsummer (Sullivan and Noe, 2001). Triglochin expands clonally and from seed (Keer and Zedler, 2002). In the nearby experimental “Tidal Linkage” marsh, Triglochin monocultures had low biomass (7.0 ± 1.9 g m−2, Callaway et al., 2003), low cover and height (~10–15 cm), and minimal layering (Keer and Zedler, 2002). In greenhouse pots, Triglochin had high N tissue content (17–52 mg g−1), high N uptake rates and roots that concentrated N (Sullivan and Zedler, 1999; Callaway et al., 2003; Sullivan et al., 2007; Morzaria-Luna and Zedler, 2014). Canopy cover of Triglochin and S. pacifica were negatively correlated in the field (Morzaria-Luna et al., 2004); and in a greenhouse experiment, S. pacifica and Triglochin competed, especially with prolonged stress (low water level and N supply) (Morzaria-Luna and Zedler, 2014). These observations and experimental results suggested a detailed study of its capabilities in mixed restoration plantings.
We hypothesized that Triglochin could influence community dynamics in a newly planted tidal restoration site by (a) accumulating N in roots and shoots, (b) reducing biomass of other marsh plain plants or, alternatively, (c) sharing N with neighbors as its litter decomposed and released N. We expected Triglochin to develop a “nitrogen reservoir” in a mostly-bare restoration site (by accumulating N in roots and shoots), and that it would transfer N to seven other planted species, as its deciduous leaves decompose. We set up a large field experiment in the Model Marsh at Tijuana Estuary in 2001 (Figure 1) and we duplicated the design in 2002 in greenhouse pots, where we could simulate tidal flushing. The greenhouse experiment served as a back-up, because in 2000 the Model Marsh lost over 5,000 plantings because it was not connected to tidal influence on schedule (see Zedler et al., 2003).
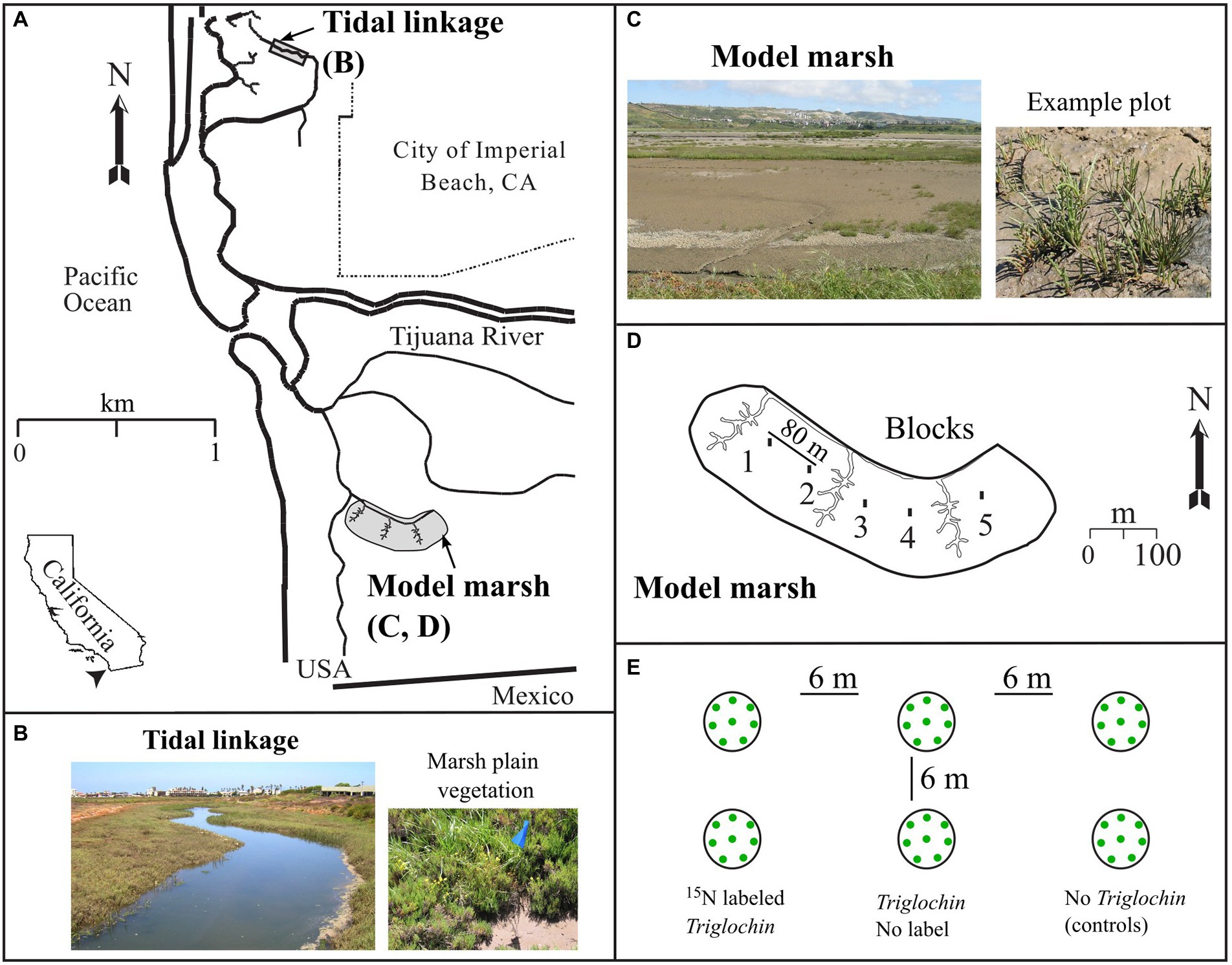
Figure 1. Location of the Tijuana River National Estuarine Research Reserve (NERR, “Tijuana Estuary,” 100 ha) in San Diego County, CA (A): Tidal Linkage (B) and Model Marsh (C) in 2003. (D) shows 5 blocks in the 8-ha Model Marsh and (E) seedling arrangement within each field plot in each block. Photos: J.B. Zedler (B) and H. Morzaria-Luna (C).
Methods
Southern California’s Mediterranean-type climate allows a year-round growing season for most salt marsh species, although there is generally a slow-growth period during cool winters. Rainfall and flooding occur in winter months and evaporation is high during the warm, dry summer. As a result, soil salinities are high (40–100 g L−1). The annual precipitation averages 26.6 cm; the average annual high temperature is 21°C and the average low 12.9°C (Zedler et al., 1986; Zedler, 2009).
We used 15N stable isotope enrichment to quantify N transfer between Triglochin and the 7-species assemblage in both field and greenhouse experiments. We also examined the effect of Triglochin on biomass and N accumulation by seven marsh plain halophytes (Table 1). Isotopic labeling has been previously used in marshes to analyze N retention and loss, measure N uptake by plant roots and soil microorganisms (Henry and Jefferies, 2003), analyze internal N transport in clonal plants from the parent ramet to the offspring ramet via the rhizome (Do, 2018), trace N transformation pathways, including long-term storage (Erler et al., 2010; Messer et al., 2017), and to identify consumers of marsh plants and algae (Kwak and Zedler, 1997; Levin et al., 2006).
Initially, we obtained background 15N values of the eight common salt marsh plain species from the reference Tidal Linkage (Figure 1). Then, in field and greenhouse experiments, we labeled Triglochin plants using 15N enriched fertilizer and incorporated them into assemblages with the remaining 7-species. N transfer was measured as 15N movement from Triglochin tissues to other species, and evidenced by an increase in δ15N in marsh plain halophyte tissues relative to background levels. Following the senescence of Triglochin, we assessed uptake of 15N by other plants after 14 months’ growth in the field and 13 months in the greenhouse.
We investigated N transfer between Triglochin and seven other marsh plain species (Table 1) in experimental plantings in the 8-ha restored Model Marsh (32.5458 N, −117.1210 W, Figure 1). The marsh was excavated in 1999 to expose buried salt marsh soil to an elevation range of 0.30–0.80 m NGVD. It was connected to an existing tidal channel in February 2000. The sediment was sandy (75.1 ± 2.3% sand, 17.3 ± 1.7% silt, and 7.6 ± 1.1% clay) and organic matter was 4.5 ± 0.13% in February 2001 (Zedler et al., 2003; O’Brien and Zedler, 2006). Spartina foliosa was planted across the site near the tidal channel.
Background 15N abundance
Background 15N abundance in test species was determined from the literature and from plants collected in the Tidal Linkage (Figure 1; Supplementary Text S1). On December 12, 2002, we collected shoot and root samples from one individual of each species. Plants were refrigerated, taken to the laboratory, and rinsed with deionized (DI) water over a 500-μm sieve to eliminate soil and salts while preserving the root mass. We separated roots and shoots for each plant. In the case of Frankenia salina, Batis maritima, and S. pacifica, any stem fragments with green tissue were classified as shoot throughout the experiment. Tissue N content (%N) and atoms % 15N in shoot and roots were determined directly on dried (60°C for 48 h) and ground tissue samples, after milling through a 40 mesh (420-μm openings). Analysis was by a Carla Erba 1,110 Elemental analyzer coupled to a Finnigan MAT 252 Isotope Ratio Mass Spectrometer at the Stable Isotope Ratio Facility for Environmental Research in the University of Utah. Precision (based on internal standards) was ±0.07‰ for 15N.
Plant 15N labeling
We applied the 15N enrichment approach due to the overlap between Triglochin’s isotope natural abundance values (δ15N) and values from other common salt marsh species (Table 2). Additionally, Tijuana Estuary receives sewage overflow during storm events, which is enriched in 15N and results in higher δ15N across the food web relative to natural marshes, which could confound natural abundance levels (Moseman et al., 2004).
Seedlings for the greenhouse and field experiments were grown in 2001 in our greenhouse at San Diego State University, CA. Seeds were collected from the Tidal Linkage in fall–winter 2000, air dried, and stored at 5°C until planting. On November 1, 2001, seeds of all marsh plain species (Table 1), except the annual Salicornia bigelovii, were germinated in flats filled with topsoil covered in a ~ 2-cm layer of potting soil. Seedlings were then transplanted individually into sterile 5×5-cm plastic pots and grown for 8 months, watering with freshwater two to three times a week as needed. We germinated S. bigelovii seeds 2 months prior to the start of each experiment.
To label Triglochin plants, we followed the method proposed by Hart (1990). On August 20, 2002, we transported the 60 largest Triglochin plants from San Diego to UW-Madison. Soil was washed off the roots with DI water using a pressure hose. We standardized plant size by removing all but three randomly chosen shoots. Roots were cut to 7-cm length, then 10 large roots were separated and remaining root mass was cut to 2-cm length. Plants were rinsed again in DI water, blotted with paper, weighed, and measured as the length of the tallest shoot.
Triglochin propagules were planted in sterile square plastic pots 9.6-cm tall (112.36 cm2 area), filled with sterilized clay soil (35.7% clay, 56.4% silt, and 7.9% sand) similar to marsh plains in southern California (Zedler, 1982). The soil had: 5.6 pH, 5 ppm NO3, 0.24 ppm P, and 2 ppm K (UW-Madison Soil and Plant Analysis Laboratory). We randomly arranged the pots in plastic trays in the greenhouse (Supplementary Figure S1). We supplemented natural light with 1,000-watt high-pressure sodium lamps in a 14:10-h photoperiod. Trays were filled with 2 g L−1 Proline™ synthetic sea water, to 2 cm below the top of the pots, to maintain soil saturation. We added DI water to offset evaporation and transpiration.
We fertilized Triglochin pots with Technigro™. 15N natural abundance was 0.368 ± 0.002 atoms % 15N, so we aimed to enrich plant tissue to 1.102 atoms % 15N. The water-soluble Technigro™ fertilizer has a 20 N: 9 P: 20 K ratio, trace elements, and 8.5% N (as CO(NH2)2) and 11.5% NO3 (as KNO3). This fertilizer was enriched with 99% 15N ammonium nitrate (NH4NO3), which was 35% N (Isotec, Inc. Miamisburg OH). Salt marsh sediments release mineralized nitrogen predominantly as ammonium, which is the main source of nitrogen uptake by marsh plants (Gardner et al., 1991; Chambers et al., 1992). We applied the enriched fertilizer to each pot at a rate of 5 g N m−2 by dissolving the fertilizer in 240 ml of water. The fertilizer was added in two 2.5 g N m−2 applications on September 9 and on September 23, 2002. Following fertilization and algal proliferation, we treated each pot with ZeroTolZT, a broad spectrum algicide containing 27% H2O2, in a 1:100 concentration, on September 25, then repeated on September 27 with a 1:300 concentration. On October 7 the plants were transplanted to larger plastic pots, 14-tall × 15.2-cm diameter (153.4-cm−2 area), filled with sterilized clay soil. We covered each pot’s soil with Perlite© to limit algal growth. After transplanting, we applied another dose of 15N enriched fertilizer (15 g m−2, mixed as described above); each pot was irrigated with 2 ml of the liquid mixture on October 25 and on November 1. In total, each plant received 0.133 g N 15N enriched.
On November 22, we began to salt-harden plants and eliminate freshwater algae. Over 2 weeks, we applied dilute seawater in increasing concentrations: 4, 6, 15, and 35 g L−1 every 3 days. In between seawater additions, we added DI water and monitored soil surface salinities as interstitial water squeezed through filter paper onto a refractometer. Once soil surface salinities were > 35 g L−1 salt, pots were watered with DI water only. On December 3, plants were removed from the pots and we carefully washed each plant over a 350-μm sieve with DI water to clean the root mass.
We blotted the labeled 25 Triglochin plants, obtained wet weight, and measured the height of the tallest shoot to analyze 15N label uptake. of these, 20 Triglochin plants were analyzed whole and 5 were separated into green shoots, senesced shoots, inflorescences, and roots. Then, they were then dried at 60°C for at least 48 h and weighed. We determined Tissue N content and atoms % 15N as with the background samples (as above). We determined recovery of the 15N label following Cabrera and Kissel (1989) as:
The remaining labeled Triglochin plants were held in DI water at 5°C for use in the field and greenhouse experiments.
Field experiment design
To test for N uptake and N transfer from Triglochin to seven halophytes, we set up a complete block experiment on December 11–12, 2002 on the unvegetated marsh plain of the Model Marsh surface ~20 m away from the edge of the S. foliosa plantings (Figure 1). The 5 blocks were ~ 80 m apart, and each block encompassed four 0.04-m−2 plots. In each plot, we planted one individual each of S. pacifica, F. salina, Jaumea carnosa, S. esteroa, L. californicum, B. maritima, and 4 S. bigelovii seedlings in a circle, with position assigned randomly. Four S. bigelovii seedlings were planted to compensate for their small size. In each block, we established 4 plots ±Triglochin (n = 2), i.e., 2 control plots with no Triglochin (−Triglochin; extrapolated density = 273 plants m−2) and 2 plots +Triglochin, planted with 15N-labeled Triglochin plants (extrapolated density = 328 plants m−2). The ±Triglochin plots were 6 m apart. Each +Triglochin plot held a pair of plants with approximately the same wet weight (36.49 ± 0.66 g). We assessed mortality on January 6 and replaced dead plants. Strong winter storms in 2003 during a mild El Niño/Southern Oscillation delivered >16 cm of heavy rainfall (NWS 2004), which dislodged S. bigelovii seedlings. After storms ceased, we replaced missing seedlings on January 30 and again on April 11, 2003. No further seedlings were replaced, due to transpiration stress as temperatures rose to 27°C in April (NOAA, 2003).
We determined soil salinity from 5-cm deep cores collected next to the treatment plots on December 12, 2002, May 19, 2003, and March 23, 2004; interstitial water was minimal, so we mixed soil pastes by drying soil samples at 60°C, mixed with DI to make a saturated soil paste (Richards, 1954), and expressed the filtrate onto a refractometer. The experiment was terminated March 23–26, 2004.
Prior to harvesting, we counted survivors (with green tissue). We collected live and dead shoots on the surface of the plot. We extracted roots using a corer 22 × 25 cm (diameter × length). Cores were taken to the lab and washed over a series of four stacked 500-μm mesh sieves to collect roots. Shoots and roots were rinsed with DI water to remove soil and salts and then stored at 5°C. Individual species’ rhizomes were separated from the root mass with dissection needles; fine roots were combined for each plot. Shoot and root samples were oven-dried at 60°C for 2 days and weighed to obtain dry weight. We analyzed whole plant %N and atoms % 15N (shoot and root tissue combined) from all plants in +Triglochin plots as described in the previous section. The N pool for each plot was calculated based on root, shoot, algal turf, and fine root biomass and tissue N content. Root:shoot ratio was calculated per plot and by species.
Greenhouse experiment design
We repeated the design of the field experiment except that we had to divide treatment replicates between two UW greenhouses to fit available space. Shading differed somewhat and one greenhouse averaged 7°C higher in ambient temperature. Evapotranspiration and soil saturation were not measured but appeared to vary with temperature.
We grew seedlings for 20+ pots in San Diego and flew them to UW-Madison, then transplanted them to sterilized plastic pots 22 × 22 cm (diameter x length) filled with sterilized clay soil. Pairs of Triglochin in each pot weighed on average 59.85 ± 1.28 g. To introduce microflora, we inoculated each pot with a small amount (~10 g) of marsh soil taken from a natural marsh in Tijuana Estuary. We assessed mortality after 2 weeks, when only S. bigelovii plants had died and were replaced. Three subsequent replants all died within a week, so we stopped replanting after March 16, 2003, when transpiration stress made S. bigelovii seedling survival unlikely.
To salt-harden seedlings, starting January 2, 2003 we watered pots with artificial Proline™ seawater (2, 4, 6, 15, and 35 g L−1 salt). In between seawater additions, we watered with DI water. Once soil surface interstitial salinities were > 35 g L−1 salt, pots were watered with DI water only. On April 8, plants were placed inside 11-L plastic containers and assigned to each of two greenhouses. Starting May 11, we alternated between dry and wet soil by filling containers with either 3 or 1.5 l of DI water every 2 weeks. We used 1,000-watt high pressure sodium lamps to supplement natural light in a 14-h photoperiod.
Triglochin produced seeds that germinated continually, so we maintained pot density by pulling up seedlings on October 17, December 4, December 18, 2003, and February 1, 2004. Seedlings were left on the soil surface to maintain each pot’s N pool. On October 17, Triglochin plants were pruned of standing dead biomass and the canopy of the other species was shaken to remove dead tissue. We fragmented this plant biomass manually and returned it to the pots. We measured interstitial soil surface salinity on June 26, August 8, September 19, 2003, and February 20, 2004. As possible, soil was returned to each pot after measuring salinity. Salinity in the bottom 5 cm of each pot was measured on February 20, 2004 while harvesting the experiment. Litter and Triglochin seedlings present at the end of the experiment were collected separately for each pot. We processed plant material as with the field experiment (see above), to determine whole plant biomass (shoots + roots), %N and atoms % 15N for +Triglochin treatments and for six plants from −Triglochin pots (three from each UW greenhouse). We pooled Triglochin seedlings to provide enough material for %N and 15N analysis. The N pool for each pot was calculated based on root, shoot, litter, fine root biomass, and tissue N content. Root:shoot ratio was calculated per plot and by species.
Data analysis
We quantified the isotopic composition relative to the 15N/14N atmospheric ratio. Results are expressed as parts per thousand (‰) differences from the corresponding standard:
higher δ values indicate a greater proportion of the heavy 15N isotope.
Differences among ± Triglochin treatments in shoot and root biomass, root:shoot ratio, %N, and δ15N values were analyzed using a two-way ANOVA with interactions. Total shoot biomass excluded litter and total root biomass excluded fine root weight; we also eliminated Triglochin from +Triglochin treatments to assess its effect on the 7-species assemblage. We specified block and greenhouse effects as factors. We analyzed individual species performance between plots and pots ± Triglochin using a multistratum ANOVA with the dependent variable (shoot and root biomass, root:shoot ratio, %N, and δ15N) nested within plots or pots.
Dependent variables that did not meet the assumptions of ANOVA were transformed: biomass was transformed using x’ = √x + ⅜ to stabilize the variance, and root:shoot data were log-transformed (Zar, 1999). Tukey’s Honest Significant Difference (HSD) was computed for post-hoc pairwise multiple comparisons. Statistical analyses were performed using R statistical framework (R Development Core Team, 2012) and the package MASS (Venables and Ripley, 2002). Means ±1 SE are reported in the text.
Results
All results are deposited in the Knowledge Network for Biocomplexity repository.1 Table 2 summarizes all results from two-way ANOVAs on shoot and root biomass, root:shoot ratio, %N, and δ15N values.
Background 15N abundance at the tidal linkage (planted in 1997)
Delta values from shoot samples were low and ranged from −0.2 to 6.7‰ (Table 3). Triglochin shoots had the highest δ15N value and B. maritima was depleted. Root samples of F. salina, J. carnosa, L. californicum, and S. pacifica were depleted relative to shoots. The δ15N values found for F. salina and S. pacifica were lower than records from other sites (Table 3); we found no published information for other species. Tissue N contents in shoots (Table 3) were highest for Triglochin (4.5%), and ranged from 0.8 to 1.6% for other species. N content in Triglochin root tissue was also higher (2.9%) than for other species (~0.7%).
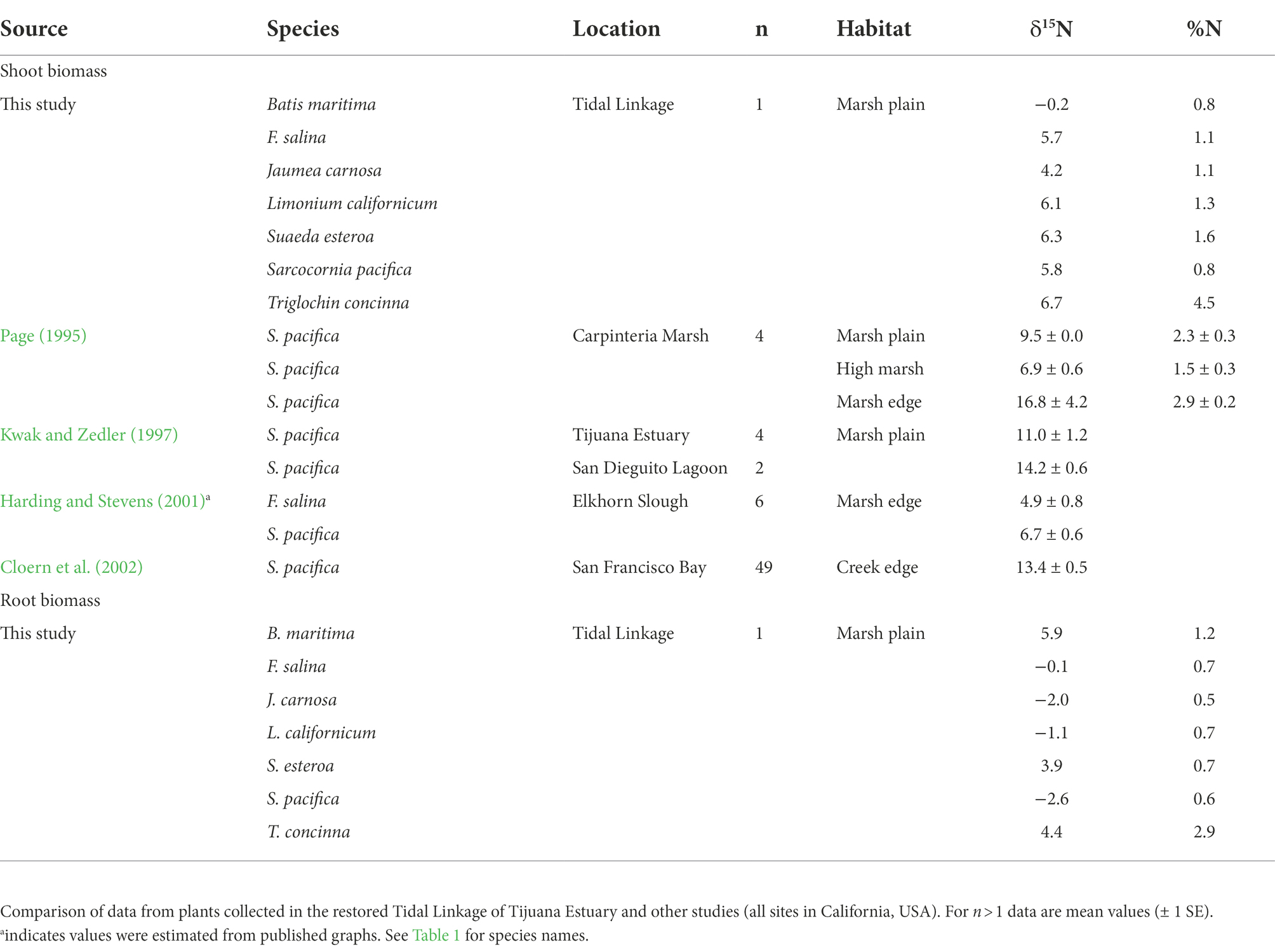
Table 3. Shoot and root stable isotope ratios (‰) and N concentrations (%) from marsh plain halophytes.
Triglochin labeling
Biomass of Triglochin plants increased 20.3 ± 1.0 g wet weight by the end of the labeling process. The mean δ value in labeled tissue was 656 ± 13.2‰ and N tissue content was 1.9 ± 0.3%. There was no difference in δ15N values between tissue types but N content in senesced shoots was 0.9 ± 0.01 compared to 2.2 ± 0.05% in live tissue. The recovery rate of the 15N label was on average 14.4 ± 0.3%.
Field experiment at the Model Marsh
Two years after it was opened to tidal flows, the soil surface salinity did not vary between blocks and it averaged 56.4 ± 2.5 g L−1 in December 2002, 72.4 ± 6.5 g L−1 in May 2003, and 70.1 ± 4.9 g L−1 in March 2004, reflecting seasonal differences.
Plant survival at the end of the experiment, in 2004, was high: 100% for B. maritima, F. salina, and S. pacifica; 65% in −Triglochin plots and 74% in +Triglochin plots. 75% for J. carnosa and S. esteroa, but only 14% for S. bigelovii. Four of the S. bigelovii plants collected were natural recruits. At the end of the experiment, only one −Triglochin plot and three +Triglochin plots still contained the original species assemblage.
Unless specified, total plot biomass for +Triglochin treatments considers all plants, including Triglochin; also, “roots” includes rhizomes throughout. Biomass did not differ between ± Triglochin treatments, despite different planting density (n = 10 in −Triglochin vs. n = 12 in +Triglochin plots). Scaling from 0.04 m−2 plots, mean shoot biomass was 363.6 ± 41.3 g m−2 in −Triglochin and 352.44 ± 58.1 g m−2 in +Triglochin plots. Shoot biomass varied among species (Figure 2; F = 77.42, p < 0.001; Supplementary Table S1). S. pacifica was the dominant; with 57.6 ± 3.7% of all shoot biomass per plot. Although Triglochin plants were still alive at the end of the experiment, most had little shoot biomass (0.96 ± 0.5 g m−2) and showed evidence of herbivory.
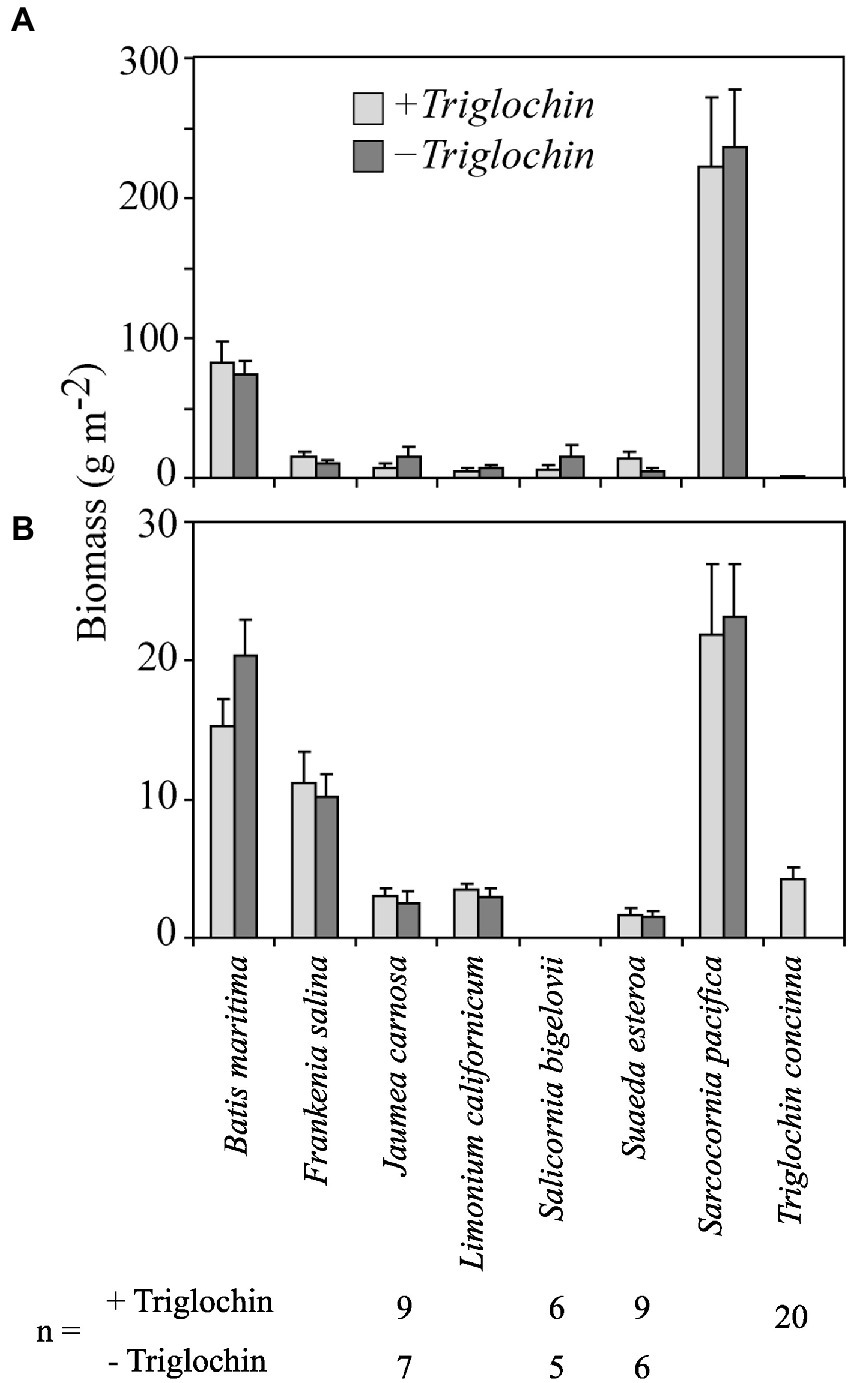
Figure 2. Mean (A) shoot and (B) root biomass from the field experiment (+ 1 SE); unless specified, n = 10. Means by species grown in 7-species assemblages with (+Triglochin) or without (−Triglochin) 15N-enriched Triglochin concinna; S. bigelovii biomass is total biomass. There were no significant differences between treatments ± Triglochin for either shoot or root biomass.
Root biomass was 60.6 ± 5.5 in −Triglochin and 56.4 ± 7.2 g m−2 in +Triglochin plots. S. pacifica and B. maritima contributed 31.7 ± 2.6 and 36.7 ± 3.1%, respectively, of total root biomass within plots (Figure 2; Supplementary Table S1). Triglochin root biomass was 4.2 ± 0.9 g m−2. Fine root biomass was high in both −Triglochin, 33.6 ± 4.4, and + Triglochin, 51.4 ± 8.9 g m−2 treatments. Root:shoot ratio did not vary either among ± Triglochin treatments. Average root:shoot ratios were 0.4 ± 0.04 in −Triglochin and 0.5 ± 0.1 in +Triglochin plots. Root:shoot ratio differed among species (Supplementary Table S2; F = 28, p < 0.0001). S. pacifica had the lowest root:shoot ratio (0.09) and Triglochin the highest (4.3), largely due to reduced shoot biomass. No litter was found in the plots.
Labeled Triglochin plants retained the 15N label after 14 months in the Model Marsh (December 2002–March 2004). Delta values were significantly different between ± Triglochin plots (F = 6.582, p = 0.0116) and between species (F = 143.8, p < 0.01). Delta values for Triglochin were 343.1 ± 39.9‰, on average 50% lower than δ15N values in plants immediately after labeling (656 ± 13.2‰). Delta values for other species ranged from 5 to 26‰ (Figure 3) and were consistently higher than background levels for plants of similar age collected in the Tidal Linkage (Table 3). Seedlings that germinated in field plots had high δ15N values (25‰) and 0.4%N. Average tissue N content was highest for Triglochin, 1.7 ± 0.1% N (Figure 3; F = 5.23, p = 0.01) and was not significantly different from background levels (Table 3). The N pool in +Triglochin plots was 5.9 ± 0.9 g m−2. The contribution of individual species to the N pool followed biomass patterns; S. pacifica accumulated 44 ± 2.0% of total N.
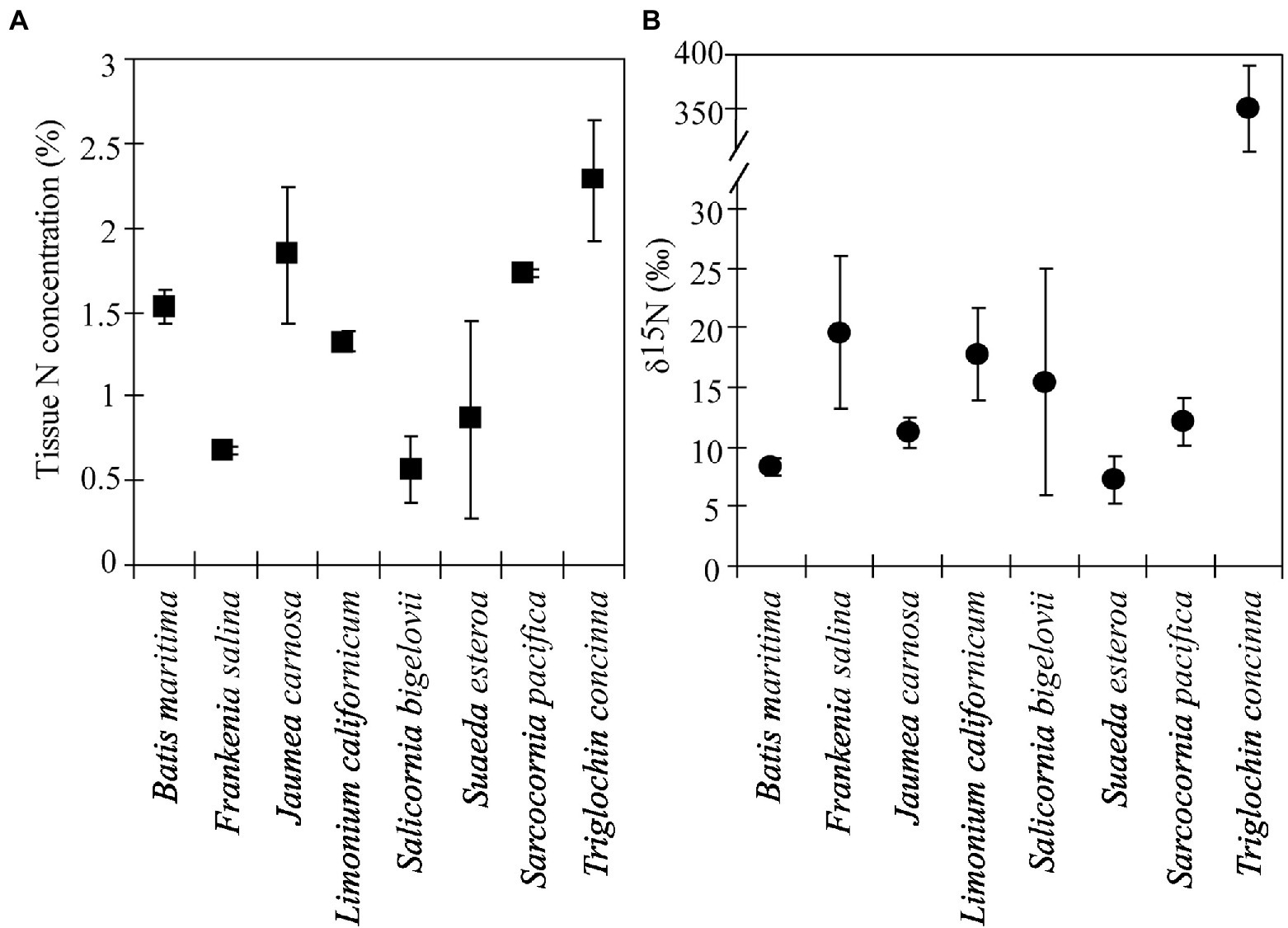
Figure 3. Tissue N concentrations (A) and δ15N values (B) for species grown December 2002–March 2004 in 7-species assemblages with 15N-enriched Triglochin concinna in the restored Friendship Marsh (± 1 SE, n = 2). Means by species.
Greenhouse experiment
Growing conditions in the greenhouse differed from the field, in part because pots confined plant roots. Soil surface salinity (5-cm depth) did not vary between pots in the two greenhouses; the average was 66.5 ± 5.3 g L−1 in June, 98.5 ± 2.4 g L−1 in August, 74.5 ± 5.3 g L−1 in September 2003, 53.5 ± 5.9 in February 2004. Salinity in the bottom 5 cm of pots was 13.1 ± 2.3 in the lower vs. 16.4 ± 2.1 g L−1 in the less-shaded greenhouse. Still the greenhouse results supported those of the field test.
As in the field, only S. bigelovii had a low survival rate (10%); all other plants survived. In both ± Triglochin pots, collected S. bigelovii plants were small (< 5 cm tall) and none was branching. At the end of the experiment, only five −Triglochin pots still contained the complete species assemblage. One −Triglochin pot was contaminated by a Triglochin seed that germinated and grew to ~10 cm before being removed at the end of the experiment.
As found in the field, there was a significant overall effect of ± Triglochin treatments on shoot biomass (Figure 4). Mean shoot biomass, scaling from 0.04-m−2 pots, was significantly higher in −Triglochin pots (373.4 ± 22.9) than +Triglochin (225.8 ± 21.3 g m−2; F = 22.16, p < 0.001). This difference can be attributed to S. pacifica, whose shoot biomass was 44% lower in +Triglochin than in −Triglochin pots (52.5 ± 7.4 vs. 120.4 ± 18.6 g m−2; F = 5.44, p < 0.0001); F. salina shoot biomass followed a similar pattern (Figure 4). Sarcocornia pacifica showed a significant difference in shoot biomass between ± Triglochin treatments. Triglochin shoot biomass was 17.64 ± 2.2 g m−2. Litter collected in +Triglochin pots was mostly Triglochin and J. carnosa senesced shoots (180.7 ± 23.3 g m−2); in −Triglochin pots litter was mostly F. salina and J. carnosa senesced leaves (95 ± 13.2 g m−2).
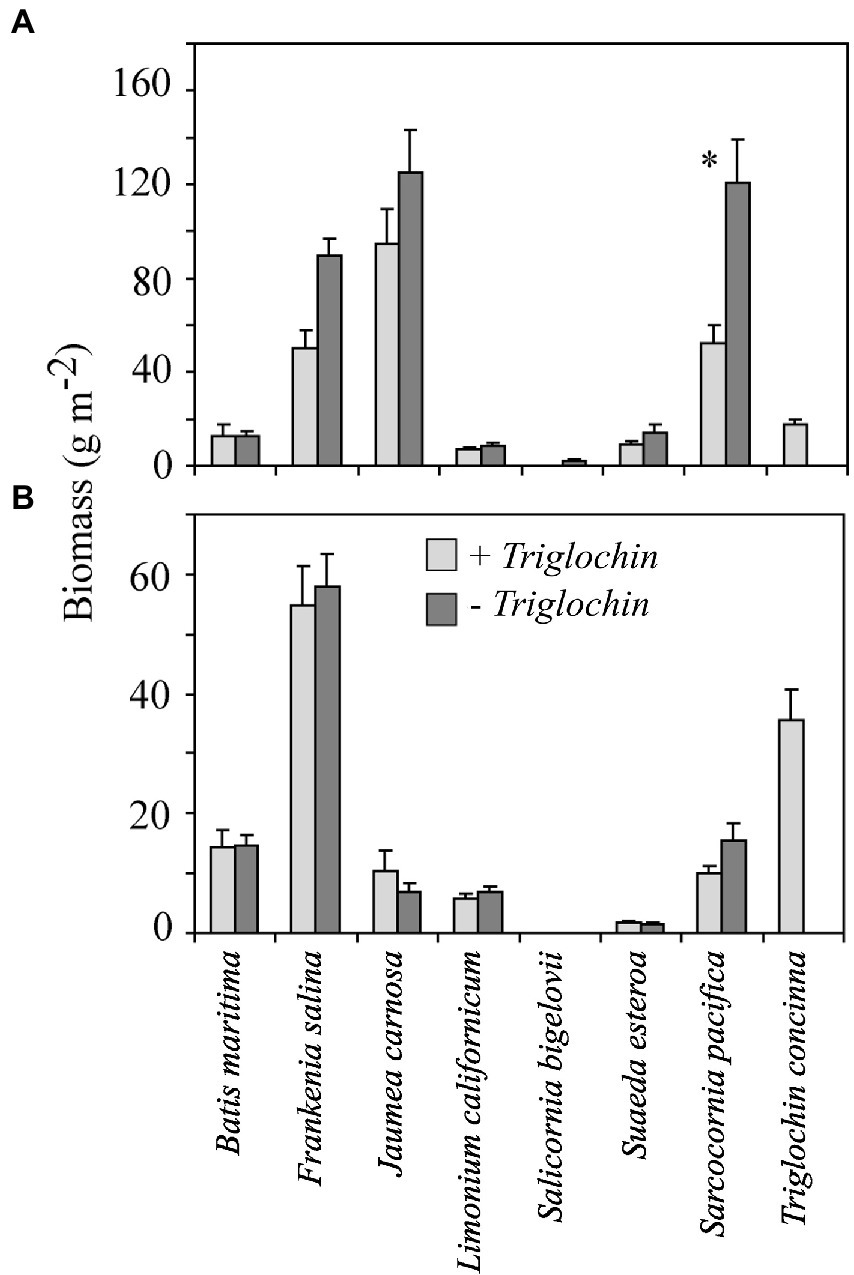
Figure 4. Mean shoot (A) and root (B) biomass from the greenhouse experiment (± 1 SE); unless specified n = 10. Means by species grown in 7- species assemblages with (+Triglochin) or without (−Triglochin) 15N-enriched Triglochin concinna; S. bigelovii biomass is total. In top graph (*) indicates means were significantly different between ± Triglochin treatments (Tukey HSD, p = 0.05). Total shoot biomass was significantly higher in -Triglochin pots; there was no difference among treatments for root biomass.
Neither shoot nor root biomass differed between greenhouses. Root biomass did not vary between ± Triglochin treatments (96.9 ± 10.5 − Triglochin vs. 102.8 ± 6.0 g m−2 + Triglochin). F. salina root biomass was significantly higher than other species (54.73 ± 6.74 g m−2; F = 96.45, p < 0.001; Supplementary Table S1), including Triglochin root biomass (35.6 ± 5.1 g m−2). Fine root biomass was similar in ± Triglochin treatments (121.1 ± 11.7 in −Triglochin and 147.1 ± 10.3 g m−2 in +Triglochin). Root:shoot ratio was significantly higher in +Triglochin treatments, average ratio was 0.5 ± 0.04 in −Triglochin and 0.7 ± 0.1 in +Triglochin pots (F = 6.14, p = 0.02; Supplementary Table S2). Triglochin had the highest root:shoot ratio (2.0) and J. carnosa the lowest (0.08). Litter production was similar between greenhouses, although litter collected in the cooler greenhouse was moist and appeared more decomposed than litter from the warmer greenhouse.
Delta values were significantly higher for species in +Triglochin pots (Figure 5; F = 13.49, p < 0.0001; Supplementary Table S3). Delta values in pots −Triglochin ranged between −2.6 and 6.8‰ and between 231.1 ± 23.2‰ for +Triglochin pots. These values for Triglochin were on average 65% lower than δ15N values found in plants right after labeling (656 ± 13.2‰). Triglochin seedlings that germinated from seeds produced during the experiment had high δ15N values, 158.8 ± 21.9‰. Delta values for other species in +Triglochin pots ranged between 4–80‰. Delta values were highest for L. californicum (89.5 ± 13‰) and lowest for B. maritima (15 ± 23.2‰). B. maritima had a high root:shoot ratio, with little growth aboveground. Delta values for plants grown in +Triglochin pots were consistently higher than background levels and values for other studies (Table 3; Supplementary Table S3). Delta values of litter in +Triglochin pots were lower than for live shoots (29.1 ± 34.8‰); δ15N values in litter from −Triglochin pots were 1.5 ± 0.4‰.
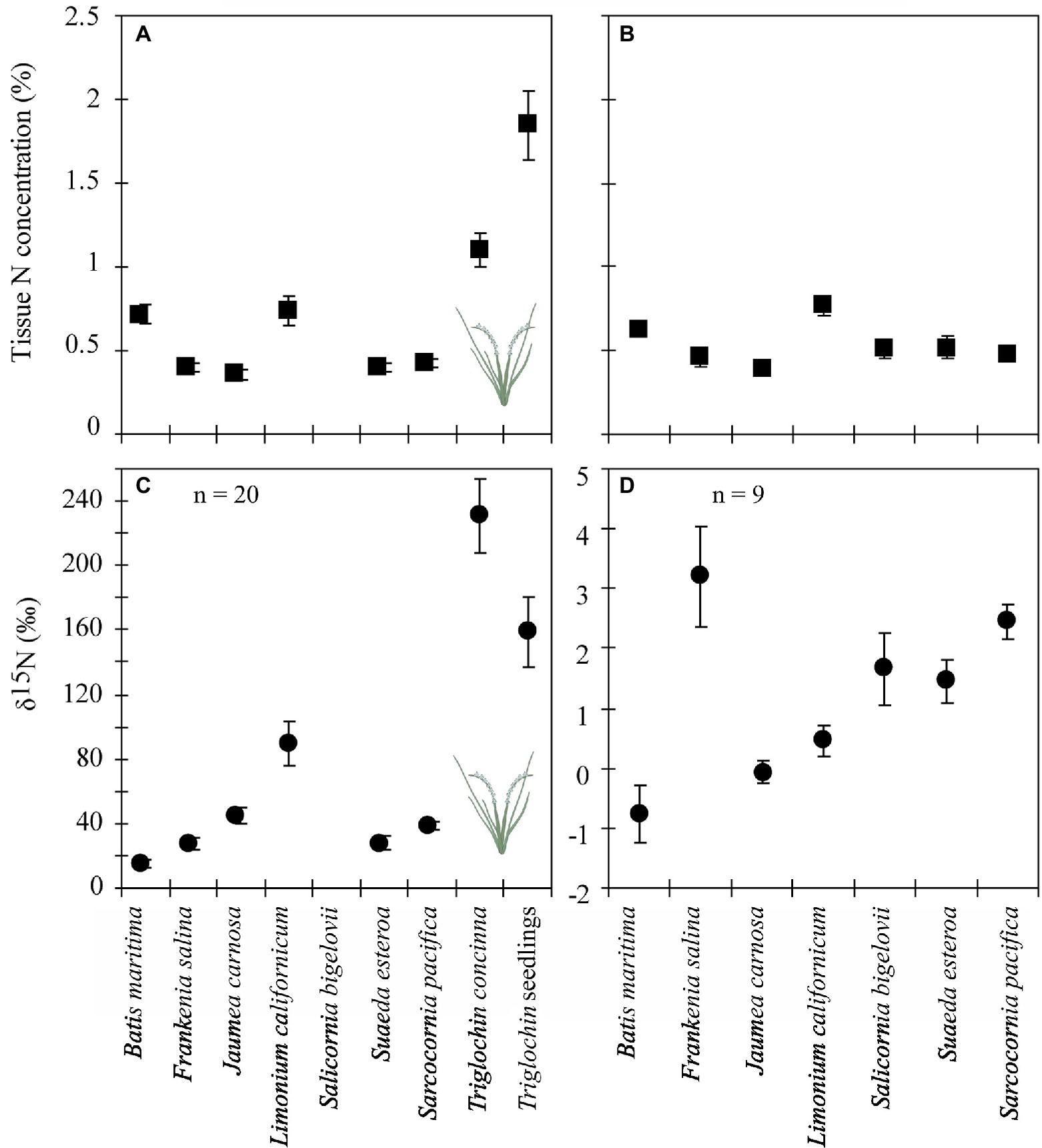
Figure 5. Tissue N concentrations (●, A & B) and δ15N values (◼, C & D) for species grown December 2002–February 2004 in 7-species assemblages with N-enriched Triglochin concinna (A & C) or without (B & D). Unless specified, n = 10. Means by species, including Triglochin seedlings that germinated during the experiment. Note different y-axis scales in panels C & D.
There were no differences in whole plant tissue N content between ± Triglochin treatments (Figure 5). There was a significant difference between the two greenhouses (F = 2.6, p < 0.001); % N was lower in the greenhouse that was warmer and received more light (0.6 ± 0.03 vs. 0.7 ± 0.1% N). As in the field experiment, tissue N content was highest in Triglochin seedlings that germinated during the experiment 1.9 ± 0.2% (F = 59.7, p < 0.001); followed by Triglochin and L. californicum (Figure 5). Percent N in litter was similar in −Triglochin, 0.8 ± 0.1, and + Triglochin, 0.73 ± 0.09% N. Tissue N content was positively related to root:shoot ratio (r = 0.39, F = 4.9, p < 0.001); suggesting higher N content in roots than shoots.
Overall, the N pool was lower in −Triglochin than in +Triglochin pots (F = 8.09, p = 0.01); this difference can be attributed to the addition of Triglochin plants. Triglochin plants and seedlings accumulated 0.6 ± 0.03 g N m−2 (Figure 6). We combined N-pools for the remaining species and we found N accumulation in tissue of the 7-species assemblage was higher in −Triglochin than in +Triglochin pots (2.17 ± 0.08 vs. 1.37 ± 0.11 g N m−2; F = 10.89, p < 0.001). This difference can be attributed to the increase in F. salina and S. pacifica shoot biomass in −Triglochin pots. There was a significant effect of greenhouse on the N pools among pots; on average, the N pool was on average higher in pots in the cooler greenhouse (4.66 ± 0.12 vs. 3.53 ± 0.05 g N m−2; F = 11.91, p = 0.003).
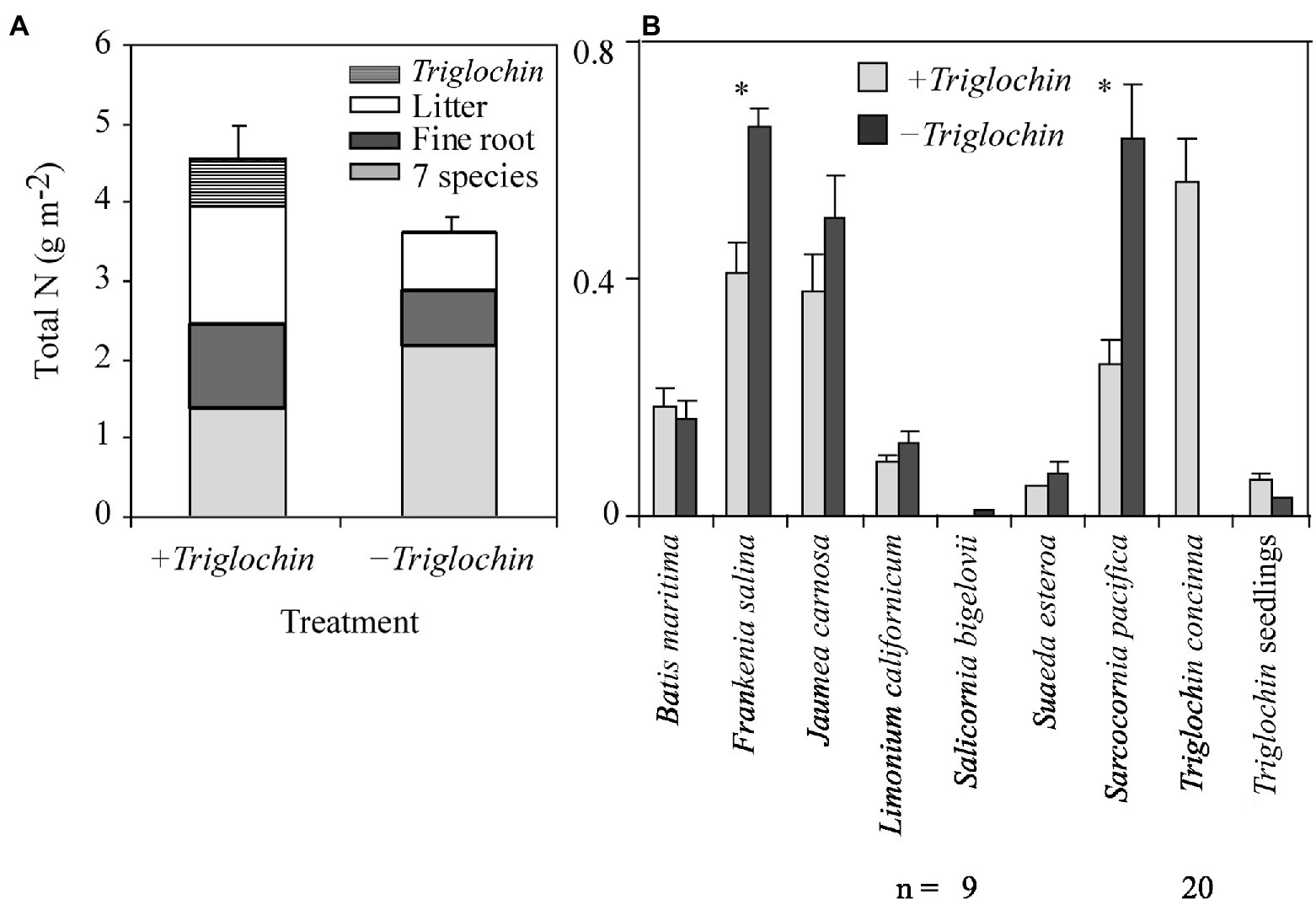
Figure 6. Total N accumulation (Mean + 1 SE), December 2002–February 2004, of 7- species assemblages with or without 15N-enriched Triglochin concinna in the greenhouse. Total means by ± Triglochin treatments (A) and the contribution of the individual species, by treatment (B) unless specified n = 10. Stacked bars are divided to indicate, fine root, and litter. Asterisks above data bars indicate that means were significantly different between treatments (Tukey HSD, p = 0.05).
Discussion
We learned that Triglochin can transfer N to seven other marsh plain species when planted as seedlings into a nearly-bare tidal plain (restored to tidal influence in 2000) as well as when grown in greenhouse pots. Enriched 15N isotopes showed that N from Triglochin is transferred to other marsh species (Figures 3, 5). We also confirmed previous experimental findings that Triglochin reduces assemblage biomass both above and below ground (Figure 4) and that assemblages with Triglochin have greater N accumulation (Figure 6). We found that although Triglochin has low biomass, cover, height, and layering in the salt marsh plain (Keer and Zedler, 2002; Callaway et al., 2003; Doughty et al., 2021), it performs two important ecosystem functions, N-storage and N-sharing. Thus, Triglochin has similarities with legumes that host biological N fixation and share N (Hamilton, 2012), and with cycads that contribute N to surrounding soils via roots and associated N-fixing cyanobacteria (Marler and Calonje, 2020). Our research also confirmed that isotope enrichment of salt marsh plants is a powerful tool for tracking the fate of N in the ecosystem (Whitcraft, 2007), clarifying ambiguities in natural abundance data, and serving as independent evidence of N transfer (Hart and Lovvorn, 2002). By being conducted in a National Estuarine Research Reserve (Figure 1) that focuses on salt marsh restoration, our realistic test of species-based functioning in the Model Marsh enhances knowledge and practice for restoring Californian tidal marshes.
Triglochin takes up and stores N
A reservoir of N is important where N is limiting, as in newly excavated, formerly buried substrates (Valiela and Teal, 1974; Boyer and Zedler, 1998). We found that Triglochin had high uptake of the 15N label, and high N content and retention (Figures 3, 5, 6), likely through recycling or internal translocation. These findings are consistent with previous results showing that Triglochin can limit growth and N accumulation of other plants in the 7-species assemblage (Table 1), particularly the biomass dominants S. pacifica and F. salina (Sullivan and Zedler, 1999; Callaway et al., 2003; Sullivan et al., 2007; Morzaria-Luna and Zedler, 2014). Our results support the hypothesis that Triglochin is a superior competitor for N and a functionally important halophyte that dominates N cycling, as proposed by (Zedler and Callaway, 1999; Zedler, 2005).
We demonstrated that a subordinate, low-biomass, species can take up more N than other marsh halophytes in a regional model restoration site. The whole plant N content of Triglochin was 1–5 times as high as that of other species in greenhouse and field experiments. Sullivan and Zedler (1999) and Callaway et al. (2003) also found high N in Triglochin shoots (1.7%–5.2%). Although waterlogged soils lose N through denitrification, Triglochin appears to have a competitive advantage in such soil, because it was able to grow substantial root biomass deep into the 10–12 cm of waterlogged soil at the base of of 38-cm tall greenhouse pots (Sullivan et al., 2007). Plant nitrogen uptake also responds to drought, flooding, salt stress and nitrogen fertilization (Morzaria-Luna and Zedler, 2014; Bai et al., 2017).
Nitrogen has important influences in salt marshes, some of which can be attributed to succulent Triglochin spp. with leaves having abundant free amino acids and low cell-wall content, preferred by several bird species as food (Popp and Albert, 1980; Naidoo, 1994). At the same time, herbivory is deterred by cyanogenic glucosides taxiphyllin and triglochinin that Triglochin spp. synthesizes (Majak et al., 1980). These compounds contain N (Nielsen and Moller, 1999), and feeding causes the cyanogenic glucosides to degrade rapidly into an aldehyde or ketone, cyanide, and sugar (Jones et al., 2000). Anecdotally, crushing the shoots of this Triglochin releases a strong smell that suggests cyanide (Alonso-Amelot and Oliveros, 2000).
Triglochin shares some of its N with neighbors
Our field and greenhouse experiments showed that seven halophyte species and labeled Triglochin had higher δ15N values (Figures 3, 5) than plants grown without labeled Triglochin. Values also exceeded previously reported natural abundances (Table 3). Thus, the labeled δ15N from Triglochin tissue was transferred to the plant tissue of the other common marsh halophytes. The 15N content in vascular plant tissue is a reflection of the N sources assimilated during the growing season (Shearer and Kohl, 1986; Johannisson and Högberg, 1994). Multiple mechanisms could account for the observed transfer of δ15N label from Triglochin to other plants. Although litter collected from +Triglochin pots was predominantly Triglochin senesced shoots, litter N content was similar in ± Triglochin pots (~0.8%); litter δ15N values in +Triglochin pots were also lower (60%) than live Triglochin shoots. This is consistent with death, decomposition, and remineralization of Triglochin shoots in the sediments and subsequent plant uptake (White and Howes, 1994a,b).
Labeled N could also reach other plant species by root exudates, leaching, or bacterial remineralization (Paynel et al., 2001; Levin et al., 2006), or by simple mass flow or diffusion of ions and simple amino acids along a concentration gradient (Teste et al., 2015). Although we did not measure N or δ15N in the sediment, the rapid transformation of inorganic N to plant organic N is a major mechanism for short-term nitrogen retention (White and Howes, 1994a). Root decomposition could also contribute to N transfer; seasonal translocation of N from above to belowground biomass during senescence is potentially important to internal N cycling of other tidal marsh species, like Spartina alterniflora (Morris, 1980; Hopkinson and Schubauer, 1984). Physiological integration where N is transferred to ramets in clonal plants, such as Triglochin, was recently confirmed in marsh plants (Do, 2018).
Triglochin retains N and can limit neighbor plant growth
While sharing its N, Triglochin can also limit the growth of its neighbors. Plants grown in pots +Triglochin had reduced shoot biomass; the dominants, S. pacifica and F. salina were particularly affected. Plants grown in +Triglochin pots had on average 44% less S. pacifica and 45% less F. salina shoot biomass than in –Triglochin pots by the end of the greenhouse experiment (Figure 4). These findings are consistent with earlier results (Table 3), Shoot N content in samples collected by Callaway et al. (2003) adjacent to our field plots ranged from 3.1 ± 0.2 to 5.1 ± 0.1% for Triglochin, and between 7.7 (J. carnosa) and 17.4% (F. salina) for the remaining species. Biomass did not differ between ±Triglochin in field plots (Figure 2), unlike in greenhouse pots with limited root space.
At least three mechanisms could account for the decreased growth of plants grown with Triglochin we observed here: direct competition, allelopathy, and indirect interactions that favor other species. In companion greenhouse and field experiments, we tested for competition between Triglochin and S. pacifica with varied N and water levels, using biomass to assess outcomes. Although there was evidence of facilitation in a short-term (6 mo.) experiment, the year-long interaction showed competition across the stress gradient (Morzaria-Luna and Zedler, 2014). Species-level traits explained responses of both Triglochin (with its high N uptake) and S. pacifica (with its large and perennial aboveground biomass). N supply was the only factor that affected outcomes; neither inundation nor time of interaction had any effect. By reducing the growth of its neighbors, Triglochin could facilitate canopy gaps, where the annual S. bigelovii could persist (Varty and Zedler, 2008). We attribute the observed reduction in shoot growth of plants in +Triglochin treatments to competition that we tracked using labeled N, as recommended by McKane et al. (2002). Although %N in plant tissue did not vary between ±Triglochin treatments, total N accumulation in F. salina and S. pacifica was higher in −Triglochin pots (0.6 ± 0.03 and 0.7 ± 0.1 g N m−2) than in +Triglochin (0.4 ± 0.05 and 0.3 ± 0.04 g N m−2) suggesting the additional growth of these plants was due to enhanced N availability.
The impact of N released from litter might be highest for S. bigelovii, the only annual species on the salt marsh plain (Zedler et al., 1999). N released by Triglochin during summer could be taken up by S. bigelovii seedlings, with senescing S. bigelovii releasing N in winter and spring, when Triglochin can take it up. Salicornia bigelovii might also take up higher amounts of N, since it was able to remove 23% of total N applied in a microcosm experiment (Brown et al., 1999). Lindig-Cisneros et al. (2003) found that where N was added to a restored S. foliosa marsh, S. bigelovii recruited rapidly, reaching biomass of up to 80 g m2. Thus, Triglochin could be extremely important in facilitating both the recruitment and establishment of S. bigelovii seedlings. Further research could assess the impact of seasonal Triglochin litter decomposition on S. bigelovii seedlings.
Implications for restoring the salt marsh plant community
Dominant species are often listed as restoration targets, and they are typically defined as those with extensive canopy cover or higher aboveground biomass (Zedler, 2001). In this study, however, we focused on a subordinate species that is hard to find after its leaves have senesced and washed away with the outgoing tide. Triglochin is often ignored; here, we confirm that it can dominate assemblages by accumulating N in its roots and shoots, even when it produces very little cover or biomass, and that it can share some of its N with neighbors and simultaneously limit their biomass. We had expected N-sharing or competition, but not both. The evidence, however, is compelling.
N-accumulation by Triglochin and subsequent effects on plant growth have immediate implications for the restoration of the plant community composition and its ecosystem functions. The salt marsh plain is dynamic, so species are not likely to remain where they are planted. A clonal species like Triglochin could concentrate N over a large area, translocating N between shoots and roots, creating long-lived nutrient-enriched patches and contributing significantly to spatial variability in available N (Baye, 2007; Do, 2018). The differential ability of plant species to compete for nutrients within nutrient-rich patches created by Triglochin could shift species abundances and increase diversity (Grime, 1997). As Triglochin shoots die back by mid-summer, they could decompose faster and release more nutrients than surrounding species because of their high N content (Quested et al., 2003). In greenhouse pots +Triglochin, 32% of the total N pool was contained in the litter (Figure 6).
Restoration is underway in several southern California salt marshes, to help compensate for major historical losses and in anticipation of future needs as sea level rises (SLR) and other anthropogenic impacts (Thorne et al., 2018). Even under the projected “low SLR scenario” for Tijuana Estuary, higher marsh vegetation would be submerged by the end of this century; and under the “high SLR scenario” all tidal marsh habitats would convert to mudflat by 2110 (Thorne et al., 2018). Thus, the upper margins of wetland salt marshes will need to extend into what is now upland. Soils that are low in N and other components (nutrients, mycorrhizae, seed banks) can be amended to support future salt marsh vegetation, but the most efficient plans will be based on knowledge of interactions, such as the ability of Triglochin to capture and share N with its native neighbors (Figures 3, 5).
We recommend planting Triglochin to increase plant diversity directly, adding seedlings and seeds to restoration plantings, and to restored and remnant marshes where Triglochin was more abundant historically (1989–2004 data show high variability at Tijuana Estuary (Zedler and West, 2008). We also recommend planting Triglochin to increase diversity indirectly, by reducing dominance where tidal action is being restored or created. We suggest collecting Triglochin seeds during peak months of fruiting (May–June) to build up a reserve for future use, e.g., longevity tests during storage beyond 3 years (Sullivan and Noe, 2001). At the Tidal Linkage, Triglochin recruitment was limited even though adult plants were nearby (Lindig-Cisneros and Zedler, 2002). Restoration plantings could omit biomass dominants (especially S. pacifica) to allow Triglochin to “creep” across the marsh plain with less competition. Additional considerations are needed for large-scale plantings that could be needed following the catastrophic loss of an entire salt marsh (e.g., through erosion, burial, oil or other toxic spills).
We continue to endorse Callaway’s (2005) call for “rigorously designed scientific experiments that identify cause-effect relationships for the development of salt marshes.” We suggest that including Triglochin in restoration can support the development and maintenance of desired ecosystem functions, especially in bare graded substrates and sandy soils, where N is likely to be limiting. We encourage long-term, strategic research and planning as a southern California “insurance policy” for salt marsh restoration.
Data availability statement
The datasets presented in this study can be found in online repositories. The names of the repository/repositories and accession number(s) can be found at: https://knb.ecoinformatics.org/view/doi:10.5063/F1V12367.
Author contributions
HM-L: conceptualization, methodology, field experiments, laboratory analysis, data analysis, data curation, writing—original draft, review and editing, visualization. JZ: conceptualization, methodology, data analysis, writing—review and editing, supervision, project administration, funding acquisition. Both authors contributed to the article and approved the submitted version.
Funding
Funding to construct the Friendship Marsh came to the Southwest Wetlands Interpretation Association from the US Fish and Wildlife Service and the California State Coastal Conservancy. The experimental work at Tijuana Estuary was funded in part by an NSF grant DEB0212005 to JZ, J. Callaway, and S. Madon, by an NSF-IGERT grant (9870703), from a DOC/NOAA National Estuarine Research Reserves fellowship (NA070R266) and a Society of Wetland Scientists student award. HM-L was supported by a CONACyT graduate scholarship (134519).
Acknowledgments
We thank four reviewers for comments on the manuscript. We thank S. Howard, M. Keener, M. Martinez, and B. Weller from the Pacific Estuarine Research Laboratory at SDSU for help in field sampling. J. Clemans, L. Hummel, I. Kabera, I. Kaplan, H. Kinmonth, and R. Luna-Godoy assisted in sample processing and greenhouse work at UW-Madison. J. M. Dorantes helped with document editing.
Conflict of interest
The authors declare that the research was conducted in the absence of any commercial or financial relationships that could be construed as a potential conflict of interest.
Publisher’s note
All claims expressed in this article are solely those of the authors and do not necessarily represent those of their affiliated organizations, or those of the publisher, the editors and the reviewers. Any product that may be evaluated in this article, or claim that may be made by its manufacturer, is not guaranteed or endorsed by the publisher.
Supplementary material
The Supplementary material for this article can be found online at: https://www.frontiersin.org/articles/10.3389/fevo.2022.851055/full#supplementary-material
Footnotes
References
Adam, P. (2019). “Salt marsh restoration” in Coastal Wetlands. eds. G. M. E. Perillo, E. Wolanski, D. R. Cahoon, and C. S. Hopkinson (Elsevier)
Alonso-Amelot, M. E., and Oliveros, A. (2000). A method for the practical quantification and kinetic evaluation of cyanogenesis in plant material. Application to Pteridium aquilinum and Passiflora capsularis. Phytochem. Anal. 11, 309–316.
Armitage, A. R. (2021). Perspectives on maximizing coastal wetland restoration outcomes in anthropogenically altered ecosystems. Estuaries Coast 44, 1699–1709. doi: 10.1007/s12237-021-00907-4
Bai, J., Jia, J., Huang, C., and Jia, J. (2017). Selective uptake of nitrogen by Suaeda salsa under drought and salt stresses and nitrogen fertilization using 15N. Ecol. Eng. 102, 542–545. doi: 10.1016/j.ecoleng.2017.02.046
Baye, P. (2007). Selected tidal marsh plant species of the San Francisco estuary: a field identification guide. San Francisco Estuary invasive Spartina project. Berkeley: California State Coastal Conservancy.
Berendse, F. (1994). “Ecosystem stability, competition, and nutrient cycling” in Biodiversity and Ecosystem Function. eds. E.-D. Schulze and H. A. Mooney (Berlin, Heidelberg: Springer Berlin Heidelberg)
Bonin, C., and Zedler, J. (2008). Southern California salt marsh dominance relates to plant traits and plasticity. Estuar. Coasts 31, 682–693. doi: 10.1007/s12237-008-9057-4
Boyer, K. E., Fong, P., Vance, R. R., and Ambrose, R. F. (2001). Salicornia virginica in a southern California salt marsh: seasonal patterns and a nutrient-enrichment experiment. Wetlands 21, 315–326. doi: 10.1672/0277-5212(2001)021[0315:SVIASC]2.0.CO;2
Boyer, K. E., and Zedler, J. B. (1998). Effects of nitrogen additions on the vertical structure of a constructed cordgrass marsh. Ecol. Appl. 8, 692–705. doi: 10.1890/1051-0761(1998)008[0692:EONAOT]2.0.CO;2
Brown, J. J., Glenn, E. P., Fitzsimmons, K. M., and Smith, S. E. (1999). Halophytes for the treatment of saline aquaculture effluent. Aquaculture 175, 255–268. doi: 10.1016/S0044-8486(99)00084-8
Cabrera, M. L., and Kissel, D. E. (1989). Review and simplification of calculations in nitrogen-15 tracer studies. Fertil. Res. 20, 11–15. doi: 10.1007/BF01055396
Callaway, J. C. (2005). The challenge of restoring functioning salt marsh ecosystem. J. Coast. Res. SI(40), 24–36.
Callaway, J. C., Sullivan, G., and Zedler, J. B. (2003). Species-rich plantings increase biomass and nitrogen accumulation in a wetland restoration experiment. Ecol. Appl. 13, 1626–1639. doi: 10.1890/02-5144
Chambers, R. M., Harvey, J. W., and Odum, W. E. (1992). Ammonium and phosphate dynamics in a Virginia salt marsh. Estuaries 15, 349–359. doi: 10.2307/1352782
Cott, G. M., Caplan, J. S., and Mozdzer, T. J. (2018). Nitrogen uptake kinetics and saltmarsh plant responses to global change. Sci. Rep. 8:5393. doi: 10.1038/s41598-018-23349-8
Cloern, J. E., Canuel, E. A., and Harris, D. (2002). Stable carbon and nitrogen isotope composition of aquatic and terrestrial plants of the San Francisco Bay estuarine system. Limnol. Oceanogr. 47, 713–729. doi: 10.4319/lo.2002.47.3.0713
Do, T. H. (2018). Applying Stable Isotope Techniques to Assess Nutrient and Carbon Dynamics in Salt Marshes. Hamburg: Staats-und Universitätsbibliothek Hamburg Carl von Ossietzky.
Doherty, J. M., Callaway, J. C., and Zedler, J. B. (2011). Diversity–function relationships changed in a long-term restoration experiment. Ecol Appl 21, 2143–2155. doi: 10.1890/10-1534.1
Doughty, C. L., Ambrose, R. F., Okin, G. S., and Cavanaugh, K. C. (2021). Characterizing spatial variability in coastal wetland biomass across multiple scales using UAV and satellite imagery. Remote Sens Ecol Conserv. 7, 411–429. doi: 10.1002/rse2.198
Erler, D. V., Eyre, B. D., and Davison, L. (2010). Temporal and spatial variability in the cycling of nitrogen within a constructed wetland: a whole-system stable-isotope-addition experiment. Limnol. Oceanogr. 55, 1172–1187. doi: 10.4319/lo.2010.55.3.1172
Gann, G. D., McDonald, T., Walder, B., McDonald, T., Walder, B., Aronson, J., et al. (2019). International principles and standards for the practice of ecological restoration. Restor. Ecol. 27, S1–S46. doi: 10.1111/rec.13035,27
Gardner, W. S., Seitzinger, S. P., and Malczyk, J. M. (1991). The effects of sea salts on the forms of nitrogen released from estuarine and freshwater sediments: does ion pairing affect ammonium flux? Estuaries 14, 157–166. doi: 10.2307/1351689
Grime, J. P. (1997). Biodiversity and ecosystem function: the debate deepens. Science 277, 1260–1261. doi: 10.1126/science.277.5330.1260
Hamilton, T. L. (2012). Defining the ecological interactions that drove the evolution of biological nitrogen fixation. Ph.D. Dissertation. Biochemistry, Montana State University.
Hart, S. C. (1990). Control of decomposition processes and nutrient flow in a California forest and grassland. University of California, Berkeley.
Harding, E. K., and Stevens, E. (2001). Using stable isotopes to assess seasonal patterns of avian predation across a terrestrial-marine landscape. Oecologia 129, 436–444. doi: 10.1007/s004420100729
Hart, E. A., and Lovvorn, J. R. (2002). Interpreting stable isotopes from macroinvertebrate foodwebs in saline wetlands. Limnol. Oceanogr. 47, 580–584. doi: 10.4319/lo.2002.47.2.0580
Haystead, A., Malajczuk, N., and Grove, T. S. (1988). Underground transfer of nitrogen between pasture plants infected with vesicular-arbuscular mycorrhizal fungi. New Phytol. 108, 417–423. doi: 10.1111/j.1469-8137.1988.tb04182.x
He, X., Xu, M., Qiu, G. Y., and Zhou, J. (2009). Use of 15N stable isotope to quantify nitrogen transfer between mycorrhizal plants. J. Plant Ecol. 2, 107–118. doi: 10.1093/jpe/rtp015
Henry, H. A. L., and Jefferies, R. L. (2003). Plant amino acid uptake, soluble N turnover and microbial N capture in soils of a grazed Arctic salt marsh. J. Ecol. 91, 627–636. doi: 10.1046/j.1365-2745.2003.00791.x
Hopkinson, C. S., and Schubauer, J. P. (1984). Static and dynamic aspects of nitrogen cycling in the salt marsh graminoid Spartina alterniflora. Ecology 65, 961–969. doi: 10.2307/1938068
Janousek, C. N., Thorne, K. M., and Takekawa, J. Y. (2019). Vertical zonation and niche breadth of tidal marsh plants along the northeast pacific coast. Estuar. Coast 42, 85–98. doi: 10.1007/s12237-018-0420-9
Johannisson, C., and Högberg, P. (1994). 15N abundance of soils and plants along an experimentally induced forest nitrogen supply gradient. Oecologia 97, 322–325. doi: 10.1007/BF00317321
Jones, P. R., Andersen, M. D., Nielsen, J. S., Andersen, M. D., Nielsen, J. S., Høj, P. B., et al. (2000). The biosynthesis, degradation, transport and possible function of cyanogenic. Evol. Metab. Pathways 34:191. doi: 10.1016/S0079-9920(00)80008-8
Keer, G. H., and Zedler, J. B. (2002). Salt marsh canopy architecture differs with the number and composition of species. Ecol. Appl. 12, 456–473. doi: 10.1890/1051-0761(2002)012[0456:SMCADW]2.0.CO;2
Kwak, T. J., and Zedler, J. B. (1997). Food web analysis of southern California coastal wetlands using multiple stable isotopes. Oecologia 110, 262–277. doi: 10.1007/s004420050159
Levin, L. A., Neira, C., and Grosholz, E. D. (2006). Invasive cordgrass modifies wetland trophic function. Ecology 87, 419–432. doi: 10.1890/04-1752
Lindig-Cisneros, R., Desmond, J. S., Boyer, K. E., and Zedler, J. B. (2003). Wetland restoration thresholds: can a degradation transition be reversed with increased effort? Ecol. Appl. 13, 193–205. doi: 10.1890/1051-0761(2003)013[0193:WRTCAD]2.0.CO;2
Lindig-Cisneros, R., and Zedler, J. B. (2002). Halophyte recruitment in a salt marsh restoration site. Estuaries 25, 1174–1183. doi: 10.1007/BF02692214
Majak, W., RE, M. D., Van Ryswyk, A. L., and Hall, J. W. (1980). Seasonal variation in the cyanide potential of Arrowgrass (Triglochin maritima). Can. J. Plant Sci. 60, 1235–1241. doi: 10.4141/cjps80-176
Marler, T. E., and Calonje, M. (2020). Two cycad species affect the carbon, nitrogen, and phosphorus content of soils. Horticulturae 6:24. doi: 10.3390/horticulturae6020024
McKane, R. B., Johnson, L. C., Shaver, G. R., Nadelhoffer, K. J., Rastetter, E. B., Fry, B., et al. (2002). Resource-based niches provide a basis for plant species diversity and dominance in arctic tundra. Nature 415, 68–71. doi: 10.1038/415068a
Messer, T. L., Burchell, M. R., Böhlke, J. K., and Tobias, C. R. (2017). Tracking the fate of nitrate through pulse-flow wetlands: a mesocosm scale 15N enrichment tracer study. Ecol. Eng. 106, 597–608. doi: 10.1016/j.ecoleng.2017.06.016
Morris, J. T. (1980). The nitrogen uptake kinetics of Spartina alterniflora in culture. Ecology 61, 1114–1121. doi: 10.2307/1936831
Morzaria-Luna, H., Callaway, J. C., Sullivan, G., and Zedler, J. B. (2004). Relationship between topographic heterogeneity and vegetation patterns in a Californian salt marsh. J. Veg. Sci. 15, 523–530. doi: 10.1111/j.1654-1103.2004.tb02291.x
Morzaria-Luna, H. N., and Zedler, J. B. (2014). Competitive interactions between two salt marsh halophytes across stress gradients. Wetlands 34, 31–42. doi: 10.1007/s13157-013-0479-9
Moseman, S. M., Levin, L. A., Currin, C., and Forder, C. (2004). Colonization, succession, and nutrition of macrobenthic assemblages in a restored wetland at Tijuana estuary, California. Estuar. Coast. Shelf Sci. 60, 755–770. doi: 10.1016/j.ecss.2004.03.013
Moyer-Henry, K. A., Burton, J. W., Israel, D. W., and Rufty, T. W. (2006). Nitrogen transfer between plants: a 15N natural abundance study with crop and weed species. Plant Soil 282:7. doi: 10.1007/s11104-005-3081-y
Naidoo, G. (1994). Growth, water and ion relationships in the coastal halophytes Triglochin bulbosa and T. striata. Environ. Exp. Bot. 34, 419–426. doi: 10.1016/0098-8472(94)90024-8
Nielsen, J. S., and Moller, B. L. (1999). Biosynthesis of cyanogenic glucosides in Triglochin maritima and the involvement of cytochrome P450 enzymes. Arch. Biochem. Biophys. 368, 121–130. doi: 10.1006/abbi.1999.1258
NOAA (2003). Local climatological data. Annual summary with comparative data. San Diego, California.
O’Brien, E. L., and Zedler, J. B. (2006). Accelerating the restoration of vegetation in a Southern California salt marsh. Wetl. Ecol. Manag. 14, 269–286. doi: 10.1007/s11273-005-1480-8
Page, H. M. (1995). Variation in the natural abundance of 15N in the halophyte, Salicornia virginica, associated with groundwater subsidies of nitrogen in a southern California salt-marsh. Oecol. Berlin 104, 181–188. doi: 10.1007/BF00328583
Paynel, F. J., Murray, P., and Bernard Cliquet, J. (2001). Root exudates: a pathway for short-term N transfer from clover and ryegrass. Plant Soil 229, 235–243. doi: 10.1023/A:1004877214831
Popp, M., and Albert, R. (1980). Free amino acids and nitrogen content in halophytes from the Neusiedlersee region, Austria. Flora 170, 229–239. doi: 10.1016/S0367-2530(17)31208-2
Quested, H. M., Cornelissen, J. H. C., and Press, M. C. (2003). Litter decomposition of sub-arctic plant species with differing nitrogen economies: the potential functional role of hemiparasites. Ecology 84, 3209–3221. doi: 10.1890/02-0426
R Development Core Team (2012). R: A Language and Environment for Statistical Computing. Ver. 2.15.2. Vienna, Austria: R Foundation for Statistical Computing.
Richards, L. A. (1954). Diagnosis and improvement of saline and alkali soils. Soil Sci. 78:154. doi: 10.1097/00010694-195408000-00012
Ryan, A. B., and Boyer, K. E. (2012). Nitrogen further promotes a dominant salt marsh plant in an increasingly saline environment. J. Plant Ecol. 5, 429–441. doi: 10.1093/jpe/rts001
Shearer, G., and Kohl, D. H. (1986). N2-fixation in field settings: estimations based on natural 15N abundance. Aust. J. Plant Physiol. 13, 699–756.
Sullivan, G., Callaway, J. C., and Zedler, J. B. (2007). Plant assemblage composition explains and predicts how biodiversity affects salt marsh functioning. Ecol. Monogr. 77, 569–590. doi: 10.1890/06-1947.1
Sullivan, G., and Noe, G. B. (2001). “Coastal wetland plant species of southern California” in Handbook for restoring tidal wetlands. ed. J. B. Zedler (Boca Raton, FL: CRC Press)
Sullivan, G., and Zedler, J. B. (1999). Functional redundancy among tidal marsh halophytes: a test. Oikos 84, 246–260. doi: 10.2307/3546719
Teste, F. P., Veneklaas, E. J., Dixon, K. W., and Lambers, H. (2015). Is nitrogen transfer among plants enhanced by contrasting nutrient-acquisition strategies? Plant Cell Environ. 38, 50–60. doi: 10.1111/pce.12367
Thorne, K., MacDonald, G., Guntenspergen, G., Ambrose, R., Buffington, K., Dugger, B., et al. (2018). US Pacific coastal wetland resilience and vulnerability to sea-level rise. Sci. Adv. 4:eaao3270. doi: 10.1126/sciadv.aao3270
Valiela, I., and Teal, J. M. (1974). Nutrient Limitation in Salt Marsh Vegetation. eds. R. J. Reimold and W. H. Queen New York: Academic Press.
Varty, A., and Zedler, J. (2008). How waterlogged microsites help an annual plant persist among salt marsh perennials. Estuar. Coasts 31, 300–312. doi: 10.1007/s12237-007-9019-2
Whitcraft, C. R. (2007). in Wetland plant influence on sediment ecosystem structure and trophic function. ed. L. A. Levin (Ann Arbor, United States: University of California, San Diego)
White, D. S., and Howes, B. L. (1994a). Long-term 15N-nitrogen retention in the vegetated sediments of a New England salt marsh. Limnol. Oceanogr. 39, 1878–1892. doi: 10.4319/lo.1994.39.8.1878
White, D. S., and Howes, B. L. (1994b). Translocation, remineralization, and turnover of nitrogen in the roots and rhizomes of Spartina alterniflora (Gramineae). Am. J. Bot. 81, 1225–1234.
Zedler, J. B. (1982). The ecology of southern California salt marshes: A community profile. U.S. Fish and Wildlife Service, Biological Service Program, FWS/OBS-81/54, 110.
Zedler, J. B. (2005). Restoring wetland plant diversity: a comparison of existing and adaptive approaches. Wetl. Ecol. Manag. 13:5. doi: 10.1007/s11273-003-5014-y
Zedler, J. B. (2009). How frequent storms affect wetland vegetation: a preview of climate-change impacts. Front. Ecol. Environ. 8, 540–547. doi: 10.1890/090109
Zedler, J. B., and Callaway, J. C. (1999). Tracking wetland restoration: Do mitigation sites follow desired trajectories? Restor. Ecol. 7, 69–73. doi: 10.1046/j.1526-100X.1999.07108.x
Zedler, J. B., Callaway, J. C., Desmond, J. S., Callaway, J. C., Desmond, J. S., Vivian-Smith, G., et al. (1999). Californian salt-marsh vegetation: an improved model of spatial pattern. Ecosystems 2, 19–35. doi: 10.1007/s100219900055
Zedler, J. B., Covin, J., Nordby, C., Covin, J., Nordby, C., Williams, P., et al. (1986). Catastrophic events reveal the dynamic nature of salt-marsh vegetation in Southern California. Estuaries 9, 75–80. doi: 10.2307/1352195
Zedler, J. B., Morzaria-Luna, H., and Ward, K. (2003). The challenge of restoring vegetation on tidal, hypersaline substrates. Plant Soil 253, 259–273. doi: 10.1023/A:1024599203741
Keywords: California, nitrogen sharing, roots, 15N tracer, tidal salt marsh, Triglochin concinna, N-cycling, wetland restoration
Citation: Morzaria-Luna H and Zedler JB (2022) Salt marsh restoration surprise: A subordinate species accumulates and shares nitrogen while outcompeting salt marsh dominants. Front. Ecol. Evol. 10:851055. doi: 10.3389/fevo.2022.851055
Edited by:
Orsolya Valkó, Hungarian Academy of Sciences, HungaryReviewed by:
Glenn Guntenspergen, United States Department of the Interior, United StatesRéka Kiss, Hungarian Academy of Sciences, Hungary
Copyright © 2022 Morzaria-Luna and Zedler. This is an open-access article distributed under the terms of the Creative Commons Attribution License (CC BY). The use, distribution or reproduction in other forums is permitted, provided the original author(s) and the copyright owner(s) are credited and that the original publication in this journal is cited, in accordance with accepted academic practice. No use, distribution or reproduction is permitted which does not comply with these terms.
*Correspondence: Hem Nalini Morzaria-Luna, aGVtbmFsaW5pQGNlZG8ub3Jn
†Present address: Hem Nalini Morzaria-Luna, Intercultural Center for the Study of Deserts and Oceans, CEDO Inc., Tucson, AZ, United States
Visiting Researcher, Northwest Fisheries Science Center, NOAA, Seattle, WA, United States