- 1Department of Anthropology and Archaeology, University of Calgary, Calgary, AB, Canada
- 2Área de Conservación Guanacaste, La Cruz, Costa Rica
- 3Department of Anthropology, Dartmouth College, Hanover, NH, United States
- 4Department of Medical Genetics, Cumming School of Medicine, University of Calgary, Calgary, AB, Canada
Aeroscapes—dynamic patterns of air speed and direction—form a critical component of landscape ecology by shaping numerous animal behaviors, including movement, foraging, and social and/or reproductive interactions. Aeroecology is particularly critical for sensory ecology: air is the medium through which many sensory signals and cues propagate, inherently linking sensory perception to variables such as air speed and turbulence. Yet, aeroscapes are seldom explicitly considered in studies of sensory ecology and evolution. A key first step towards this goal is to describe the aeroscapes of habitats. Here, we quantify the variation in air movement in two successional stages (early and late) of a tropical dry forest in Costa Rica. We recorded air speeds every 10 seconds at five different heights simultaneously. Average air speeds and turbulence increased with height above the ground, generally peaked midday, and were higher overall at the early successional forest site. These patterns of lower air speed and turbulence at ground level and overnight have important implications for olfactory foraging niches, as chemotaxis is most reliable when air movement is low and steady. We discuss our results in the context of possible selective pressures and observed variation in the foraging ecology, behaviors, and associated morphologies of resident vertebrates, with a focus on mammals. However, these data also have relevance to researchers studying socioecology, invertebrate biology, plant evolution, community ecology and more. Further investigation into how animals use different forest types, canopy heights and partition activities across different times of day will further inform our understanding of how landscape and sensory ecology are interrelated. Finally, we emphasize the timeliness of monitoring aeroecology as global wind patterns shift with climate change and human disturbance alters forest structure, which may have important downstream consequences for biological conservation.
Introduction
Air is a dynamic and ever-changing medium, and aeroscapes (defined as patterns in air speed and direction; Vogel, 1996) are an integral part of terrestrial ecosystems. Efforts to integrate aeroscapes into the study of organismal behavior and ecology (collectively termed aeroecology; Kunz et al., 2008; Diehl, 2013) have revealed that animals react adaptively to variables such as air speed and turbulence (Frick et al., 2013; Diehl et al., 2017). These same factors are expected to have pronounced effects on the propagation and uptake of sensory information (Finelli et al., 2000; Muller-Schwarze, 2006). For example, greater air speeds will disperse the odors of plants and animals farther, but the resulting turbulence is likely to disrupt the spatial distribution of odor plumes, challenging the ability of organisms to navigate toward the odor source (i.e., anemotaxis; Murlis, 1997; Conover, 2007; Bingman and Moore, 2017). This tradeoff in signal propagation and efficacy is well-studied in insects, which optimize their flight paths in response to air speed while tracking odor plumes (Aluja et al., 1993; Cardé and Willis, 2008; Hennessy et al., 2020). At the same time, many mammals possess relatively complex olfactory systems, and they, too, are sensitive to variations in the aeroscape (Moulton, 1967; Svensson et al., 2014). For example, air speed is known to affect the olfactory orientation and behavior of carnivorans—red foxes, striped skunks, raccoons, polar bears, domestic dogs (Ruzicka and Conover, 2011, 2012; Togunov et al., 2017; Jinn et al., 2020)—as well as primates, such as ring-tailed lemurs (Cunningham et al., 2021). Still, there has been little effort to explore the spatiotemporal factors that govern a given aeroscape, or the effects of this variability on the aero-sensory ecology of mammals, especially in forest ecosystems.
Forests are complex habitats in the vertical and horizontal planes (Ennos, 1997), and this level of heterogeneity is reflected in the form of highly variable aeroscapes (Baynton, 1969; Heydel et al., 2014). For example, the understory is essentially sheltered from the winds affecting the upper canopy, which can create striking vertical disparities in air speeds (Aoki et al., 1978; McCay, 2003). Scant air movement in the understory is expected to favor efficient anemotaxis, but the magnitude of vertical variation in an aeroscape can be offset temporally—e.g., at night, when air is cooler and moving at diminished speed—or spatially as a function of standing forest biomass (Murlis et al., 2000; McCay, 2003). The essential limitation is that these factors are rarely measured simultaneously or folded into our understanding of mammalian aero-sensory ecology and evolution.
To contribute toward building this literature, we studied spatiotemporal variation in the aeroscape of a lowland tropical dry forest in Costa Rica. We recorded variation in air speed and turbulence as a function of: (1) vertical position and (2) diel periodicity. We measured each of these variables at two sites, chosen to reflect two habitat types—early and late successional forest. Our goal is to better understand how aeroscapes vary within a forest, and how this variation might mediate the distribution of odorant molecules through the aeroscape. This information is essential for understanding the selective pressures that have shaped the olfactory anatomy and behaviors of resident animals, as well as plant reproductive strategies.
Materials and Methods
Study Site
We collected data in Sector Santa Rosa of the Área de Conservación Guanacaste, northwestern Costa Rica. The site is a tropical dry forest with two distinct seasons: a dry season from December through May and a wet season from June through November (Campos, 2018; Janzen and Hallwachs, 2020; Melin et al., 2020). Sector Santa Rosa forests are primarily secondary, stemming from restoration and reforestation efforts that began in the 1970s (Janzen and Hallwachs, 2020), and forest composition and structure varies between early and late successional stages. Canopy height ranges from 6 to 15 m depending on successional stage; the canopy is typically taller in areas of later succession (Kalacska et al., 2004; Powers et al., 2009).
Data Collection
We used cup anemometers (WL-11; Scarlet Tech, Taipei, Taiwan) to collect air speed data from May to June 2021. The instruments have a sensitivity range of 0.6–50 m/s, a resolution of 0.1 m/s, and an accuracy of ±2%, per manufacturer specifications. We built and erected two scaffold towers: one in a late successional forest (10.838617, −85.614283; 1,086 m a.s.l.), and the other in an early successional forest (10.839383, −85.616383; 906 m a.s.l.). To each tower, we affixed five anemometers at heights of 0.5, 3.5, 5.5, 7.5, and 10 m (Figure 1). The devices were set to data-logging mode, recording average and maximum air speed in 10-s intervals.
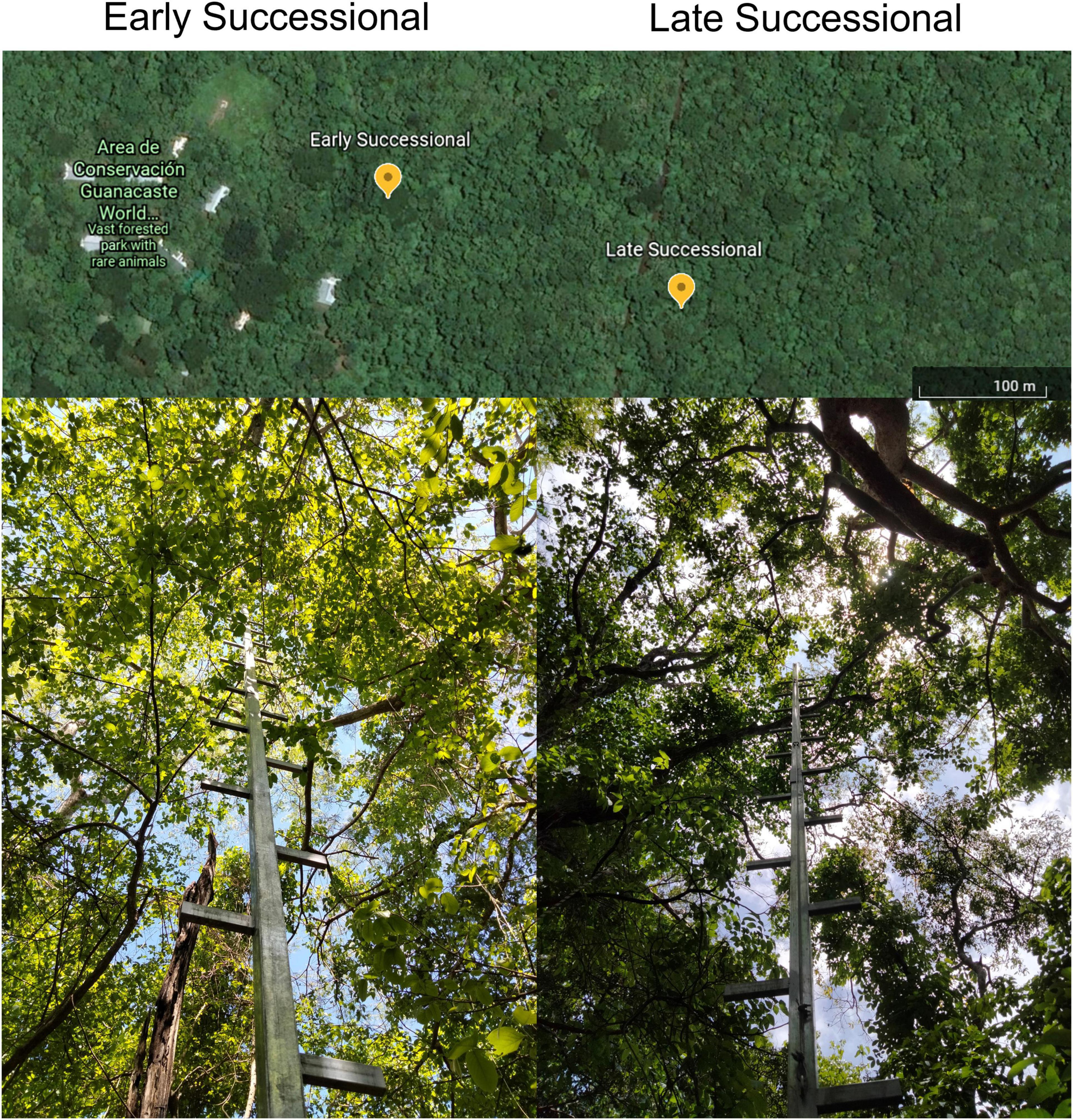
Figure 1. Scaffold system used to fix five anemometers per tower at heights of 0.5, 3.5, 5.5, 7.5, and 10 m. The anemometers were moved between our early successional forest site and our late successional forest site at 2 week intervals.
Data Analysis
We obtained the mean, median, and standard deviation of air speed for: (1) each sampling height and (2) time of day. We calculated air turbulence as standard deviation of air speed/mean air speed (McCay, 2003). The sensitivity threshold (0.6 m/s) of our anemometer risks a systematic bias against low air speeds, especially in the understory. Accordingly, we imputed values below this limit by using survival analyses, a method developed for the health sciences but adopted for analyzing environmental data with detection limits (Helsel, 2004). Using the survival (Therneau, 2021) and NADA (Lee, 2020) packages in R (v. 4.1.0., R Core Team, 2021), we constructed Kaplan–Meier estimates for data recorded at each site independently for each height and time of day, considering air speeds ≤0.6 m/s to be left-censored.
To detect significant differences in air speeds and turbulence as a function of vertical height and time of day, we conducted Cox proportional hazard modeling via the survival package in R. As these hazard models are designed for right-censored data, we transformed our data by using the “flipping” method of Helsel (2004), which subtracts all observed values from a constant to convert left-censored data to right-censored. Since the same column of wind is recorded simultaneously at all 5 observed heights for a given site, there is a high degree of collinearity between the height and time of day predictor variables, therefore we modeled each separately and present two models. To address our first aim, we modeled air speed as a function of vertical position, based on height from the ground (“Height”) in two different habitat types. To address our second aim, we modeled air speed as a function of diel periodicity (“Time of Day”) in two different habitat types. For both models, the outcome variable was the Kaplan–Meier estimate of average wind speed, and an interaction term of study site and height, or study site and time of day, was included as a predictor variable. For the Height model, the continuous variable distance from the ground (in meters) was used, while for the Time of Day model, because of the circular nature of temporal data, we employed a sinusoidal model with separate cos and sin terms (Simmons, 1990; Cazelles et al., 2008). Code for all analyses can be found at https://github.com/allegradepasquale/wind_speed_project.git.
Results
Vertical Variation
Air speeds increased as a positive function of vertical height, and mean air speeds differed between the study sites, with greater mean speeds recorded in the early successional forest (Figure 2 and Table 1). The interaction term of study site and height was also significant (Table 1). Variation in air turbulence followed a similar pattern: mean air turbulence scores varied as a positive function of vertical height and were greater in the early successional forest (Figure 2). Site differences in our measure of air turbulence were pronounced near ground level (0.5 m), but values tended to converge with increasing vertical height (Figure 2).
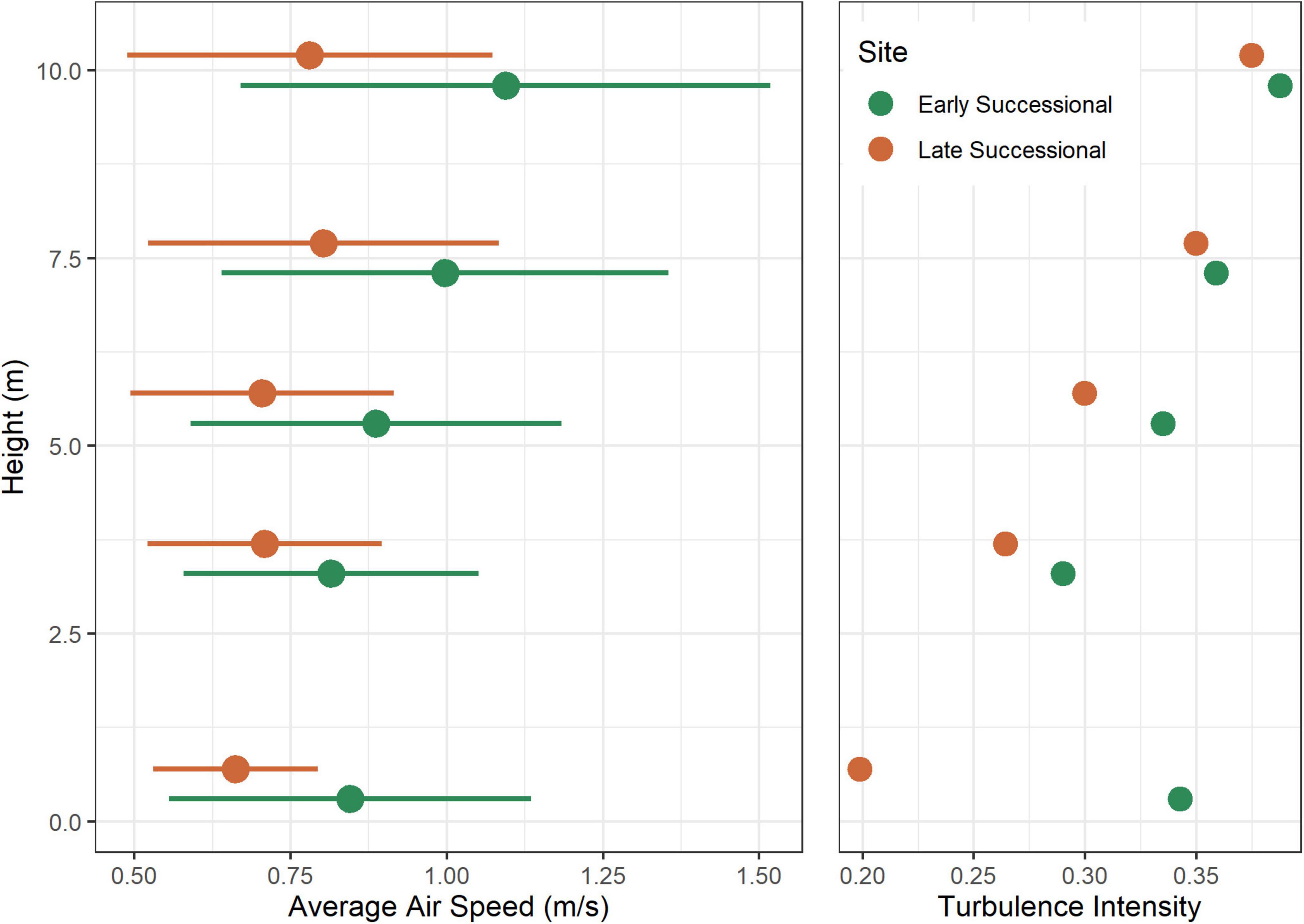
Figure 2. Average air speeds (±standard deviation) and turbulence score at different heights from the ground for our early successional and late successional forest sites, generated from Kaplan–Meier estimates.
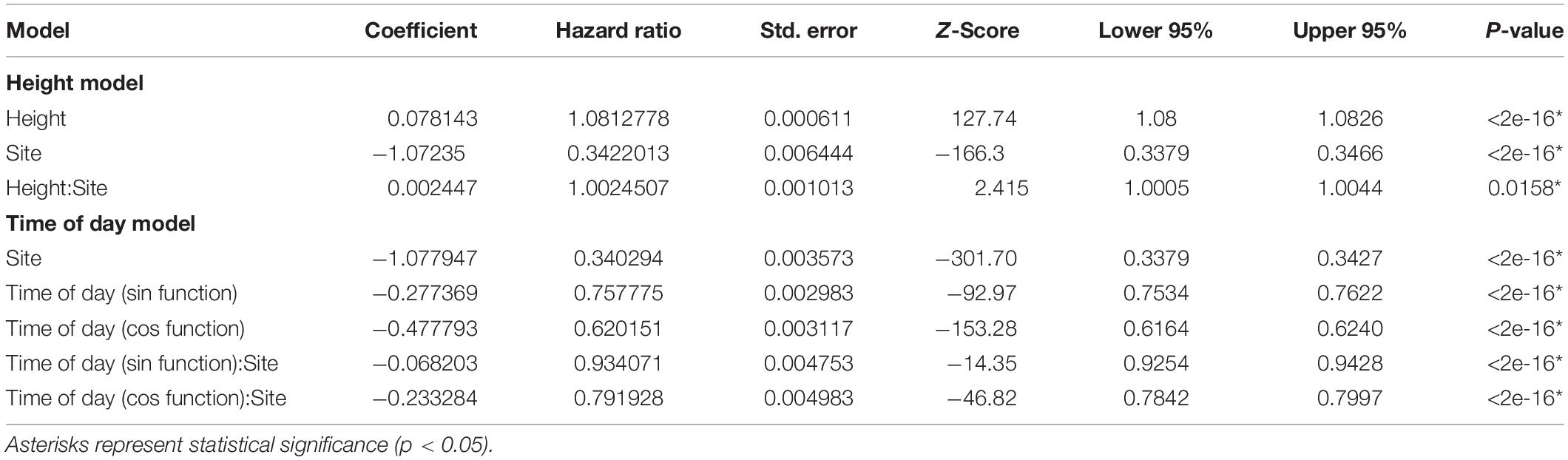
Table 1. Results of cox proportional hazard models for mean air speed by vertical position and time of day (i.e., diel cycle).
Temporal (Diel) Variation
Air speed varied significantly with time of day: winds were stronger from late morning to early afternoon and were lowest overnight (Figures 3, 4). As with our analyses of height, the main effect of study site, as well as the interaction between study site and time of day, were also significant (Figure 4 and Table 1). Turbulence scores were highest at midday and lowest overnight.
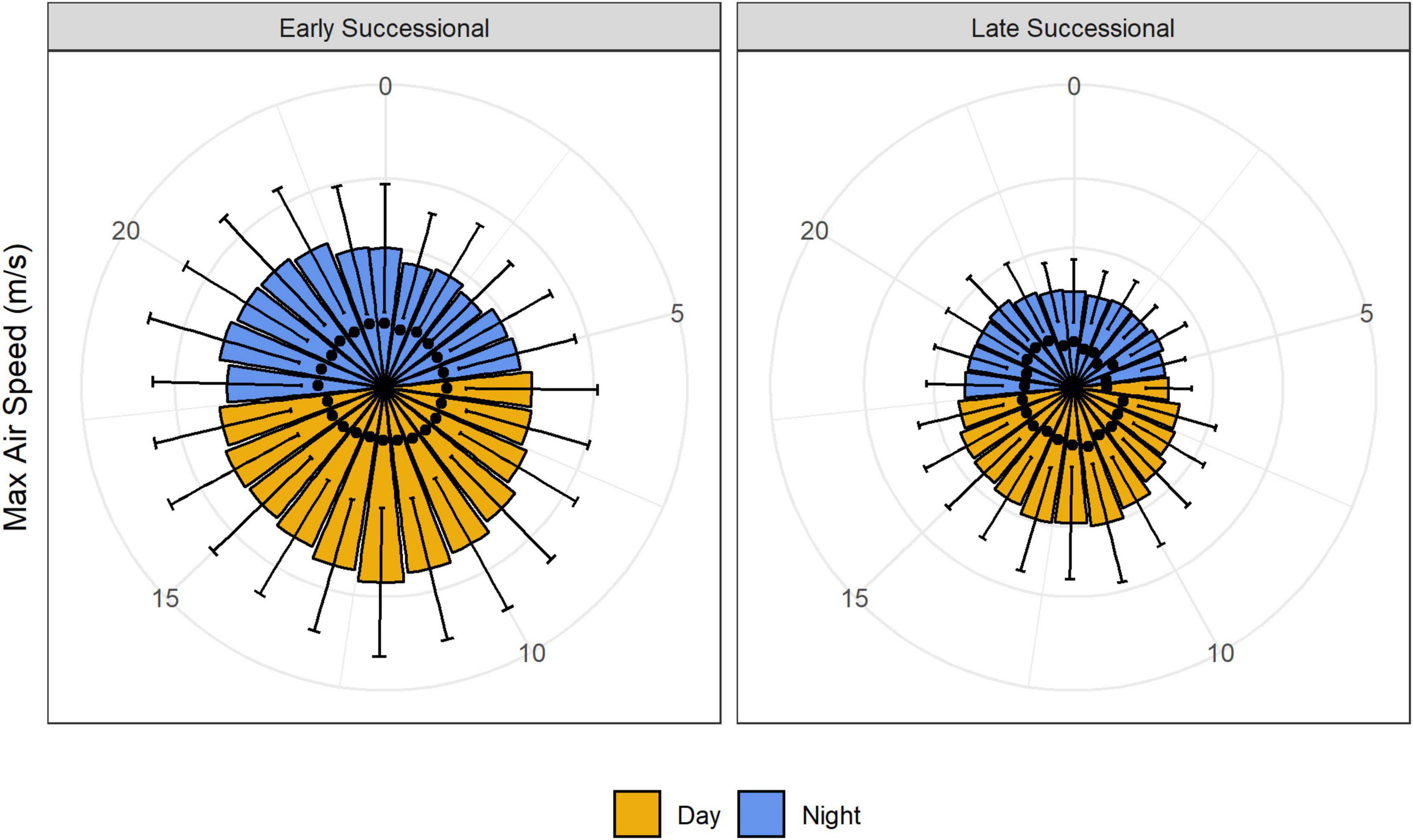
Figure 3. Maximum air speeds in meters per second at our early and late successional sites over the diel cycle. Black dots represent turbulence scores.
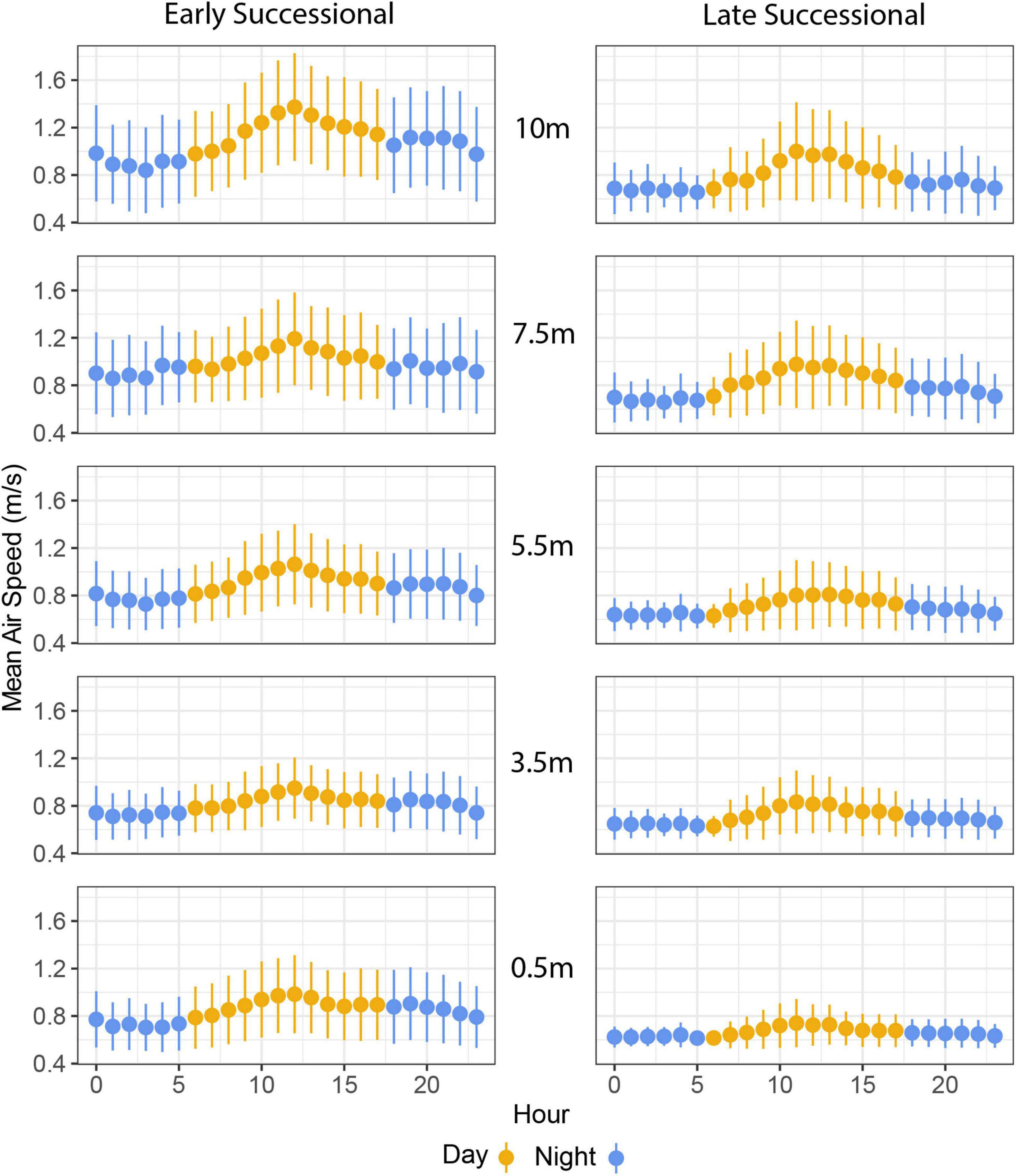
Figure 4. Mean air speeds at two forest sites as a function vertical height and diel periodicity. The x-axis begins at 00:00 h (midnight) and ends at 23:00 h (11:00 pm).
Discussion
Air movement is a dynamic aspect of landscape ecology that shapes the way animals experience the world. In this study, we quantified air movement in a tropical dry forest as a function of vertical position and diel periodicity in two forest types. We found that air speed and turbulence increased with height, peaked midday, and were lower in the late successional forest. Taken together, our findings suggest spatiotemporal predictability in the aeroscape of a tropical forest and motivate a discussion of how animals adapt to and exploit these patterns.
Vertical Variation in Aeroscapes
We detected a steep increase in air speeds as a function of vertical height, a result that replicates findings from other forest ecosystems (Baynton, 1969; Oliver and Mayhead, 1974; Aoki et al., 1978; Kruijt et al., 2000; McCay, 2003). The prevailing explanation for this pattern is that air movement is impeded near the ground by understory vegetation, tree trunks, and surface topography; however, the implications of such a gradient for olfactory ecology are underexplored. Fruits, for example, emit odors (generally lightweight, ephemeral volatile and semivolatile organic compounds) that are easily dispersed by air movement (Rodríguez et al., 2013; Nevo et al., 2018, 2020), although excessive air speeds can over-disperse odor compounds and disrupt anemotaxis (Svensson et al., 2014). Still, it follows that the vertical position of fruit will determine the probability and efficiency of long-distance odor detection and foraging by seed-dispersing mutualists (Santana et al., 2021). Anemotaxis toward food resources is well-studied among invertebrates (Zjacic and Scholz, 2022) and increasingly so among mammals. For example, experiments with bats have elicited klinotaxis in response to fruit odors (Thies et al., 1998; Korine and Kalko, 2005; Leiser-Miller et al., 2020; Brokaw et al., 2021; Brokaw and Smotherman, 2021), and a field experiment by Fleming et al. (1977) found that bats will deviate from their flyways by as much as 50 m to acquire fruits mounted to 1.5-m poles set up moments before sunset. Further, experiments with coatis and ring-tailed lemurs have shown that they can detect fruit from distances up to 20 m (Hirsch, 2010; Cunningham et al., 2021). These findings suggest that aero-sensory ecology via stimulus response can complement and extend the critical importance of spatial memory to the localization of foods (Janson, 1998; Janmaat et al., 2014; Dahmani et al., 2018). Looking forward, it stands to reason that our understanding of cognitive ecology will only be strengthened as we incorporate and integrate the systematic study of aeroscapes and other sensory landscapes into research frameworks.
Many animals use olfactory signals to communicate with one another for reproduction, dominance, and territory defense, as well as to discriminate conspecifics from heterospecifics. In mammals, these occur mainly through the deposition of scent marks (Johnson, 1973; Irwin et al., 2004; Kollikowski et al., 2019). Our results suggest that olfactory signals may remain more localized when deposited nearer to the ground than higher in the canopy. It is noteworthy, then, that olfactory communication appears to be particularly prevalent in terrestrial mammals, which have olfactory receptor gene repertoires that have undergone three times as much gene duplication than those of volant, arboreal, and aquatic mammals (Hughes et al., 2018). At the same time, low air speeds will limit long-distance dispersal of odors. It is possible that species and habitat-specific optima for olfactory signal height exist and may vary across taxa. For example, Ethiopian wolves, gray wolves, and pine martens use raised-leg urination, a behavior thought to reinforce territorial boundaries by dispersal of urinary odor plumes. Urine deposition above, rather than on, the ground may improve scent dispersal (Peters and Mech, 1975; Macdonald, 1980; Pulliainen, 1981; Alberts, 1992; Sillero-Zubiri and Macdonald, 1998). Turning to another mammalian radiation, many primate species that have evolved glands dedicated for scent deposition, including ring-tailed lemurs, mandrills, drills, and sifakas, are largely terrestrial (Delbarco-Trillo et al., 2011; Drea, 2015; Vaglio et al., 2016). Systematic study of olfactory signaling and receiving behaviors, along with investigation of co-occurring anatomical and genetic variation, in taxa occupying different vertical niches will allow this hypothesis to be tested. In general, the intersection of sensory and aeroecology holds untapped potential for better understanding the wide variation in animal sensory traits.
Temporal (Diel) Variation in Aeroscapes
We detected pronounced variation across the 24-h day, with peak and nadir air movements at midday and overnight, respectively. This pattern is almost certainly due to ambient temperature flux because warmer air moves faster and is less stable (Pleijel et al., 1996; McCay, 2003; Monteith and Unsworth, 2013; but c.f. Baynton, 1968, 1969; Rapp and Silman, 2012 for other patterns). Following similar arguments to those concerning vertical variation, nocturnal aeroscapes may be preferential for animals that detect and localize scents. Many nocturnal animals rely more on olfaction than vision, but the reasons are usually couched in the language of constraint—there is scant light at night, so vision is limited (Barton et al., 1995; Balkenius et al., 2006; Borges, 2018; Niimura et al., 2018). Underappreciated, however, is the idea that olfaction is more effective at night due to the relative stillness of air, especially in the understory, where air speeds and turbulence tend to be lowest (Murlis, 1997; Muller-Schwarze, 2006). Lack of wind produces a high signal-to-noise ratio for a given odor plume, resulting in a stronger olfactory signal. This nocturnal environment may thus select for olfactory-driven ecologies and social interactions, perhaps even in the absence of selection driven by the loss of visual ability (Drea et al., 2019).
Spatiotemporal Interactions
Vertical and diel variation interact to create diverse aeroscapes, ranging from the windiest and most turbulent environment of the upper canopy at midday, to the comparative stillness of the understory at night. Such interactions create opportunities for convergence. We found that aeroscapes in the daytime understory are comparable to those in the canopy at night, which raises the possibility of convergent olfactory signals and sensitivities in the animals that occupy these distinct niches (Barton, 2006; Valenta et al., 2013; Brokaw and Smotherman, 2020; Nevo and Ayasse, 2020). Interestingly, the olfactory ecology of nocturnal terrestrial frugivores may depend in part on wind-mediated fruit falls during daytime, suggesting vertical day-night integration of aeroecologies (Augspurger and Franson, 1987; Borah and Beckman, 2021). The upshot is that daytime canopy conditions are suboptimal for anemotaxis in the service of frugivory and seed dispersal, which may explain why so-called “bird-fruits” in the upper canopy emit little scent (Gautier-Hion et al., 1985; Howe, 1986; Lomáscolo et al., 2010; Valenta et al., 2018; Valenta and Nevo, 2020).
Some angiosperm plants, including Ficus and Nicotiana, exhibit diurnal rhythms in fruit and flower chemistry (Raguso et al., 2003; Borges et al., 2011; Burdon et al., 2015; Ripperger et al., 2019; Balducci et al., 2020) timed to maximize their availability and attractiveness for pollinators and seed dispersers. These diurnal rhythms may have evolved in response to diurnal patterns in air movement, such that compounds produced during the day may be heavier and more robust against turbulent conditions than those produced at night, which may be lighter and more easily propagated under calmer conditions (Alberts, 1992; Muller-Schwarze, 2006). Flowers, which are generally more delicate and ephemeral than fruits, are often produced early in the morning, which may reflect a compromise between protection from wind-damage and availability to vision-mediated pollinators such as bees and birds (Herrera, 1990; Bloch et al., 2017). Setting aside the aeroecology of frugivory and pollination, aeroscapes are also essential to another aspect of plant reproductive biology: wind dispersal (Kennedy, 1978; Friedman and Barrett, 2008). Flowering and the eventual abscission of wind-dispersed seeds is greatest at midday, when air movement is highest, which suggests some level of aerosensation (Bohrer et al., 2008; Wright et al., 2008; Caplat et al., 2012). Such hypotheses invite future testing.
Intraforest Variation
We recorded higher air speeds and greater turbulence in the early successional habitat compared to the later one, possibly reflecting the lower levels of standing biomass. This pattern is expected for habitats with greater levels of anthropogenic disturbance that have caused vegetative loss (Raynor, 1971; Muller-Schwarze, 2006; Klein et al., 2021). Such results have potentially important implications. For example, increased air movement may affect the relative colonization of wind-dispersed versus animal-dispersed plants in disturbed areas (Cadenasso and Pickett, 2001; Cubiña and Aide, 2006; Nathan et al., 2008), altering community dynamics, habitat suitability to frugivores, and reforestation efforts (Janzen, 1988; Vieira and Scariot, 2006; de la Peña-Domene et al., 2018; Camargo et al., 2020). Greater air movement and turbulence are also expected to negatively affect animals that rely on odor plumes (Shukla et al., 1990; Zhang et al., 1996; Gandu et al., 2004). Consequences include impediments to animal foraging and communication, as discussed above, as well as effects on predator-prey networks.
Sensory detection of predators often involves scent, and many species are particularly attuned to the odors of their relevant predators (Weldon, 1990; Kats and Dill, 1998; Apfelbach et al., 2005). Rats are particularly sensitive to 2,4,5-trimethylthiazoline, a component of red fox anal gland secretions (Laska et al., 2005) and mice, rats, and stoats, for example, have been shown to avoid carnivore and apex predator odors (Ferrero et al., 2011; Garvey et al., 2016). Indeed, higher wind speeds have been found to impede predator detection by mule deer (Bowyer et al., 2001) and other mammal species (Cherry and Barton, 2017). The sensory impact of air movement can be further compounded by spillover to the other senses: wind creates acoustic and visual noise, which may reduce detection of stimuli by other senses, further impeding predator detection (Hayes and Huntly, 2005; Carr and Lima, 2010; Francis et al., 2012). Overall, future work could usefully address the diverse ways that anthropogenically modified aeroscapes affect the aero-sensory ecology and habitat use of resident flora and fauna (Damschen et al., 2008). As these influences could ultimately affect species distributions (Bowyer and Kie, 2009; Breitbach et al., 2012), we urge the incorporation of aeroscapes into existing conservation and evolutionary frameworks (e.g., assessment of “edge effects” and “landscapes of fear”) and general inclusion into future studies of anthropogenic disturbance and deforestation/reforestation dynamics (Laundre et al., 2010).
Limitations and Future Directions
Our results offer insight into the variability of air movement within a heterogeneous landscape, although some caution must be noted. First, due to equipment limitations, we were unable to sample early and late successional forest sites simultaneously, and instead sampled them sequentially. This serial approach raises the possibility that temporal differences in climate, not habitat heterogeneity, were driving the difference that we observed between sampling locations. Further, due to the logistical challenges of erecting scaffolds in a protected habitat, we were unable to sample replicates of early and late successional forest conditions. The extent to which our two study locations exemplify such habitat conditions is therefore uncertain, and could usefully be explored in greater detail in future studies sampling multiple locations per forest type. Lastly, the detection limits of our anemometers prohibited direct measurement of the slowest air speeds. While prohibitively expensive for our study design, solid-state anemometers have the advantage of greater measurement range. Future studies may also benefit from incorporating variation in wind direction, as this also has important implications for olfactory-based orientation (Kennedy, 1978; Togunov et al., 2017; Jinn et al., 2020).
Our study contributes to the emerging field of aeroecology by quantifying variation in the aeroscape of a lowland tropical dry forest and drawing attention to the implications for sensory signal propagation, plant reproduction, and mammalian sensory evolution. This topic is timely as shifting air movement patterns due to anthropogenic climate change may disrupt aeroecological interactions, with the potential far-reaching effects on animal behavior, population dynamics, and species distributions (Usbeck et al., 2010; McInnes et al., 2011; Young et al., 2011; Lewis et al., 2015). Understanding the impacts of aeroscapes on sensory ecology and evolution will be key for predicting how animals will respond to changing environments.
Data Availability Statement
The raw data supporting the conclusions of this article will be made available by the authors, without undue reservation.
Author Contributions
AD contributed to the study design, led data collection and analysis, and wrote the manuscript. JH co-led data analysis alongside AD and contributed to the writing of the manuscript. CG contributed to the data collection, study design, and writing of the manuscript. ND contributed to the study design, data analysis, and writing of the manuscript. AM contributed to the study design, data collection and analysis, and writing of the manuscript. All authors contributed to the article and approved the submitted version.
Funding
This work was supported by the NSERC(RGPIN-2017-03782) and Canada Research Chairs Program (950-231257) to AM, National Geographic Early Career Research Grant (EC-59267R-19) to AD, and University of Calgary, Faculty of Arts (JH).
Conflict of Interest
The authors declare that the research was conducted in the absence of any commercial or financial relationships that could be construed as a potential conflict of interest.
Publisher’s Note
All claims expressed in this article are solely those of the authors and do not necessarily represent those of their affiliated organizations, or those of the publisher, the editors and the reviewers. Any product that may be evaluated in this article, or claim that may be made by its manufacturer, is not guaranteed or endorsed by the publisher.
Acknowledgments
We sincerely thank Roger Blanco, Maria Marta Chavarria, and the staff of the Área de Conservación Guanacaste for making this work possible. We also thank Saúl Cheves Hernandez and Danny Montiel for help with the construction of our scaffolds for this project.
References
Alberts, A. C. (1992). Constraints on the design of chemical communication systems in terrestrial vertebrates. Am. Nat. 139, S62–S89.
Aluja, M., Prokopy, R. J., Buonaccorsi, J. P., and Cardé, R. T. (1993). Wind tunnel assays of olfactory responses of female Rhagoletis pomonella flies to apple volatiles: effect of wind speed and odor release rate. Entomol. Exp. Appl. 68, 99–108. doi: 10.1111/j.1570-7458.1993.tb01693.x
Aoki, M., Yabuki, K., and Koyama, H. (1978). Micrometeorology of pasoh forest. Malayan Nat. J. 30, 149–159. doi: 10.1007/978-4-431-67008-7_11
Apfelbach, R., Blanchard, C. D., Blanchard, R. J., Hayes, R. A., and McGregor, I. S. (2005). The effects of predator odors in mammalian prey species: a review of field and laboratory studies. Neurosci. Biobehav. Rev. 29, 1123–1144. doi: 10.1016/j.neubiorev.2005.05.005
Augspurger, C. K., and Franson, S. E. (1987). Wind dispersal of artifical fruits varying in mass, area, and morphology. Ecology 68, 27–42. doi: 10.2307/1938802
Balducci, M. G., Van der Niet, T., and Johnson, S. D. (2020). Diel scent and nectar rhythms of an African orchid in relation to bimodal activity patterns of hawkmoth pollinators. Ann. Bot. 126, 1155–1164. doi: 10.1093/aob/mcaa132
Balkenius, A., Rosén, W., and Kelber, A. (2006). The relative importance of olfaction and vision in a diurnal and a nocturnal hawkmoth. J. Comp. Physiol. A 192, 431–437. doi: 10.1007/s00359-005-0081-6
Barton, R. A. (2006). Olfactory evolution and behavioral ecology in primates. Am. J. Primatol. 68, 545–558. doi: 10.1002/ajp.20251
Barton, R. A., Purvis, A., and Harvey, P. H. (1995). Evolutionary radiation of visual and olfactory brain systems in primates, bats and insectivores. Philos. Trans. R. Soc. London. Series B: Biol. Sci. 348, 381–392. doi: 10.1098/rstb.1995.0076
Baynton, H. W. (1968). The ecology of an elfin forest in Puerto Rico, 2. the microclimate of pico del oeste. J. the Arnold Arboretum 49, 419–430. doi: 10.5962/p.185747
Baynton, H. W. (1969). The ecology of an elfin forest in Puerto Rico, 3. Hilltop and forest influences on the microclimate of Pico del Oeste. J. Arnold Arboretum 50, 80–92.
Bingman, V. P., and Moore, P. (2017). “Properties of the atmosphere in assisting and hindering animal navigation,” in Aeroecology, eds P. Chilson, W. Frick, J. Kelly, and F. Liechti (Cham: Springer), doi: 10.1007/978-3-319-68576-2_6
Bloch, G., Bar-Shai, N., Cytter, Y., and Green, R. (2017). Time is honey: circadian clocks of bees and flowers and how their interactions may influence ecological communities. Philos. Trans. R. Soc. B: Biol. Sci. 372:20160256. doi: 10.1098/rstb.2016.0256
Bohrer, G., Katul, G. G., Nathan, R., Walko, R. L., and Avissar, R. (2008). Effects of canopy heterogeneity, seed abscission and inertia on wind-driven dispersal kernels of tree seeds. J. Ecol. 96, 569–580. doi: 10.1111/j.1365-2745.2008.01368.x
Borah, B., and Beckman, N. G. (2021). Studying seed dispersal through the lens of movement ecology. Oikos 2022, 1–13. doi: 10.1111/oik.08310
Borges, R. M. (2018). Dark matters: challenges of nocturnal communication between plants and animals in delivery of pollination services. Yale J. Biol. Med. 91, 33–42.
Borges, R. M., Ranganathan, Y., Krishnan, A., Ghara, M., and Pramanik, G. (2011). When should fig fruit produce volatiles? pattern in a ripening process. Acta Oecologica 37, 611–618. doi: 10.1016/j.actao.2011.06.003
Bowyer, R. T., McCullough, D. R., and Belovsky, G. E. (2001). Causes and consequences of sociality in mule deer. Alces 37, 371–402.
Bowyer, R., and Kie, J. (2009). Thermal landscapes and resource selection by black-tailed deer: implications for large herbivores. California Fish Game 95, 128–139.
Breitbach, N., Tillmann, S., Schleuning, M., Grünewald, C., Laube, I., Steffan-Dewenter, I., et al. (2012). Influence of habitat complexity and landscape configuration on pollination and seed-dispersal interactions of wild cherry trees. Oecologia 168, 425–437. doi: 10.1007/s00442-011-2090-1
Brokaw, A. F., and Smotherman, M. (2020). Role of ecology in shaping external nasal morphology in bats and implications for olfactory tracking. PLoS One 15:e0226689. doi: 10.1371/journal.pone.0226689
Brokaw, A. F., and Smotherman, M. (2021). Olfactory tracking strategies in a neotropical fruit bat. J. Exp. Biol. 224:jeb231829. doi: 10.1242/jeb.231829
Brokaw, A. F., Davis, E., Page, R. A., and Smotherman, M. (2021). Flying bats use serial sampling to locate odour sources. Biol. Lett. 17:20210430. doi: 10.1098/rsbl.2021.0430
Burdon, R. C. F., Raguso, R. A., Kessler, A., and Parachnowitsch, A. L. (2015). Spatiotemporal floral scent variation of penstemon digitalis. J. Chem. Ecol. 41, 641–650. doi: 10.1007/s10886-015-0599-1
Cadenasso, M. L., and Pickett, S. T. A. (2001). Effect of edge structure on the flux of species into forest interiors. Conservation Biol. 15, 91–97. doi: 10.1111/j.1523-1739.2001.99309.x
Camargo, P. H. S. A., Pizo, M. A., Brancalion, P. H. S., and Carlo, T. A. (2020). Fruit traits of pioneer trees structure seed dispersal across distances on tropical deforested landscapes: implications for restoration. J. Appl. Ecol. 57, 2329–2339. doi: 10.1111/1365-2664.13697
Campos, F. A. (2018). “A synthesis of long-term environmental change in Santa Rosa, Costa Rica,” in Primate Life Histories, Sex Roles, and Adaptability: Essays in Honour of Linda M. Fedigan, eds U. Kalbitzer and K. M. Jack (Berlin: Springer), 331–358. doi: 10.1007/978-3-319-98285-4_16
Caplat, P., Nathan, R., and Buckley, Y. M. (2012). Seed terminal velocity, wind turbulence, and demography drive the spread of an invasive tree in an analytical model. Ecology 93, 368–377. doi: 10.1890/11-0820.1
Cardé, R. T., and Willis, M. A. (2008). Navigational strategies used by insects to find distant, wind-borne sources of odor. J. Chem. Ecol. 34, 854–866. doi: 10.1007/s10886-008-9484-5
Carr, J. M., and Lima, S. L. (2010). High wind speeds decrease the responsiveness of birds to potentially threatening moving stimuli. Animal Behav. 80, 215–220. doi: 10.1016/j.anbehav.2010.04.021
Cazelles, B., Chavez, M., Berteaux, D., Ménard, F., Vik, J. O., Jenouvrier, S., et al. (2008). Wavelet analysis of ecological time series. Oecologia 156, 287–304. doi: 10.1007/s00442-008-0993-2
Cherry, M. J., and Barton, B. T. (2017). Effects of wind on predator-prey interactions. Food Webs 13, 92–97. doi: 10.1016/j.fooweb.2017.02.005
Cubiña, A., and Aide, T. M. (2006). The effect of distance from forest edge on seed rain and soil seed bank in a tropical pasture1. Biotropica 33, 260–267. doi: 10.1111/j.1744-7429.2001.tb00177.x
Cunningham, E. P., Edmonds, D., Stalter, L., and Janal, M. N. (2021). Ring-tailed lemurs (Lemur catta) use olfaction to locate distant fruit. Am. J. Phys. Anthropol. 175, 300–307. doi: 10.1002/ajpa.24255
Dahmani, L., Patel, R. M., Yang, Y., Chakravarty, M. M., Fellows, L. K., and Bohbot, V. D. (2018). An intrinsic association between olfactory identification and spatial memory in humans. Nat. Commun. 9:4162. doi: 10.1038/s41467-018-06569-4
Damschen, E. I., Brudvig, L. A., Haddad, N. M., Levey, D. J., Orrock, J. L., and Tewksbury, J. J. (2008). The movement ecology and dynamics of plant communities in fragmented landscapes. Proc. Natl. Acad. Sci. U S A. 105, 19078–19083. doi: 10.1073/pnas.0802037105
de la Peña-Domene, M., Martínez-Garza, C., Ayestarán-Hernández, L. M., and Howe, H. F. (2018). Plant attributes that drive dispersal and establishment limitation in tropical agricultural landscapes. Forests 9:620. doi: 10.3390/f9100620
Delbarco-Trillo, J., Burkert, B. A., Goodwin, T. E., and Drea, C. M. (2011). Night and day: the comparative study of strepsirrhine primates reveals socioecological and phylogenetic patterns in olfactory signals. J. Evol. Biol. 24, 82–98. doi: 10.1111/j.1420-9101.2010.02145.x
Diehl, R. H. (2013). The airspace is habitat. Trends Ecol. Evol. 28, 377–379. doi: 10.1016/j.tree.2013.02.015
Diehl, R. H., Peterson, A. C., Bolus, R. T., and Johnson, D. H. (2017). “Extending the habitat concept to the airspace,” in Aeroecology, eds P. B. Chilson, W. F. Frick, J. F. Kelly, and F. Liechti (Berlin: Springer), 47–69. doi: 10.1007/978-3-319-68576-2_3
Drea, C. M. (2015). D’scent of man: a comparative survey of primate chemosignaling in relation to sex. Horm. Behav. 68, 117–133. doi: 10.1016/j.yhbeh.2014.08.001
Drea, C. M., Goodwin, T. E., and Delbarco-Trillo, J. (2019). P-Mail: the information highway of nocturnal, but not diurnal or cathemeral, strepsirrhines. Folia Primatol. 90, 422–438. doi: 10.1159/000495076
Ennos, A. R. (1997). Wind as an ecological factor. Trends Ecol. Evol. 12, 108–111. doi: 10.1016/S0169-5347(96)10066-5
Ferrero, D. M., Lemon, J. K., Fluegge, D., Pashkovski, S. L., Korzan, W. J., Datta, S. R., et al. (2011). Detection and avoidance of a carnivore odor by prey. Proc. Natl. Acad. Sci. U S A. 108, 11235–11240. doi: 10.1073/pnas.1103317108
Finelli, C. M., Pentcheff, N. D., Zimmer, R. K., and Wethey, D. S. (2000). Physical constraints on ecological processes: a field test of odor-mediated foraging. Ecology 81, 784–797. doi: 10.1890/0012-9658(2000)081[0784:pcoepa]2.0.co;2
Fleming, T. H., Heithaus, E. R., and Sawyer, W. B. (1977). An experimental analysis of the food location behavior of frugivorous bats. Ecology 58, 619–627. doi: 10.2307/1939011
Francis, C. D., Kleist, N. J., Ortega, C. P., and Cruz, A. (2012). Noise pollution alters ecological services: enhanced pollination and disrupted seed dispersal. Proc. R. Soc. B: Biol. Sci. 279, 2727–2735. doi: 10.1098/rspb.2012.0230
Frick, W. F., Chilson, P. B., Fuller, N. W., Bridge, E. S., and Kunz, T. H. (2013). “Aeroecology,” in Bat Evolution, Ecology, and Conservation, eds R. A. Adams and S. C. Pedersen (Berlin: Springer), 149–167. doi: 10.1007/978-1-4614-7397-8_8
Friedman, J., and Barrett, S. C. H. (2008). A phylogenetic analysis of the evolution of wind pollination in the angiosperms. Int. J. Plant Sci. 169, 49–58. doi: 10.1086/523365
Gandu, A. W., Cohen, J. C. P., and de Souza, J. R. S. (2004). Simulation of deforestation in eastern Amazonia using a high-resolution model. Theoretical Appl. Climatol. 78, 123–135. doi: 10.1007/s00704-004-0048-5
Garvey, P. M., Glen, A. S., and Pech, R. P. (2016). Dominant predator odor triggers caution and eavesdropping behavior in a mammalian mesopredator. Behav. Ecol. Sociobiol. 70, 481–492. doi: 10.1007/s00265-016-2063-9
Gautier-Hion, A., Duplantier, J.-M., Quris, R., Feer, F., Sourd, C., Decoux, J.-P., et al. (1985). Fruit characters as a basis of fruit choice and seed dispersal in a tropical forest vertebrate community. Oecologia 65, 324–337. doi: 10.1007/BF00378906
Hayes, A. R., and Huntly, N. J. (2005). Effects of wind on the behavior and call transmission of pikas (Ochotona princeps). J. Mammal. 86, 974–981. doi: 10.1644/1545-1542(2005)86[974:eowotb]2.0.co;2
Helsel, D. R. (2004). Nondetects and Data Analysis: Statistics for Censored Environmental Data. Hoboken, NJ: Wiley-Interscience.
Hennessy, G., Harris, C., Eaton, C., Wright, P., Jackson, E., Goulson, D., et al. (2020). Gone with the wind: effects of wind on honey bee visit rate and foraging behavior. Animal Behav. 161, 23–31. doi: 10.1016/j.anbehav.2019.12.018
Herrera, C. M. (1990). Daily patterns of pollinator activity, differential pollinating effectiveness, and floral resource availability, in a summer-flowering mediterranean shrub. Oikos 58, 277–288. doi: 10.2307/3545218
Heydel, F., Cunze, S., Bernhardt-Römermann, M., and Tackenberg, O. (2014). Long-distance seed dispersal by wind: disentangling the effects of species traits, vegetation types, vertical turbulence and wind speed. Ecol. Res. 29, 641–651. doi: 10.1007/s11284-014-1142-5
Hirsch, B. T. (2010). Tradeoff between travel speed and olfactory food detection in ring-tailed coatis (Nasua nasua). Ethology 116, 671–679. doi: 10.1111/j.1439-0310.2010.01783.x
Hughes, G. M., Boston, E. S. M., Finarelli, J. A., Murphy, W. J., Higgins, D. G., and Teeling, E. C. (2018). The birth and death of olfactory receptor gene families in mammalian niche adaptation. Mol. Biol. Evol. 35, 1390–1406. doi: 10.1093/molbev/msy028
Irwin, M. T., Samonds, K. E., Raharison, J.-L., and Wright, P. C. (2004). Lemur latrines: observations of latrine behavior in wild primates and possible ecological significance. J. Mammal. 85, 420–427. doi: 10.1644/1383937
Janmaat, K. R., Polansky, L., Ban, S. D., and Boesch, C. (2014). Wild chimpanzees plan their breakfast time, type, and location. Proc. Natl. Acad. Sci. U S A. 111, 16343–16348. doi: 10.1073/pnas.1407524111
Janson, C. H. (1998). Experimental evidence for spatial memory in foraging wild capuchin monkeys, Cebus apella. Animal Behav. 55, 1229–1243. doi: 10.1006/anbe.1997.0688
Janzen, D. H. (1988). Management of habitat fragments in a tropical dry forest: growth. Annals Missouri Botanical Garden 75, 105–116. doi: 10.2307/2399468
Janzen, D. H., and Hallwachs, W. (2020). Área de Conservación Guanacaste, northwestern Costa Rica: converting a tropical national park to conservation via biodevelopment. Biotropica 52, 1017–1029. doi: 10.1111/btp.12755
Jinn, J., Connor, E. G., and Jacobs, L. F. (2020). How ambient environment influences olfactory orientation in search and rescue dogs. Chem. Senses 45, 625–634. doi: 10.1093/chemse/bjaa060
Johnson, R. P. (1973). Scent marking in mammals. Animal Behav. 21, 521–535. doi: 10.1016/S0003-3472(73)80012-0
Kalacska, M., Sanchez-Azofeifa, G. A., Calvo-Alvarado, J. C., Quesada, M., Rivard, B., and Janzen, D. H. (2004). Species composition, similarity and diversity in three successional stages of a seasonally dry tropical forest. Forest Ecol. Manag. 200, 227–247. doi: 10.1016/j.foreco.2004.07.001
Kats, L. B., and Dill, L. M. (1998). The scent of death: chemosensory assessment of predation risk by prey animals. Écoscience 5, 361–394. doi: 10.1080/11956860.1998.11682468
Kennedy, J. S. (1978). The concepts of olfactory ‘arrestment’ and ‘attraction.’. Physiol. Entomol. 3, 91–98. doi: 10.1111/j.1365-3032.1978.tb00138.x
Klein, M., Garvelmann, J., and Förster, K. (2021). Revisiting forest effects on winter air temperature and wind speed—new open data and transfer functions. Atmosphere 12:710. doi: 10.3390/atmos12060710
Kollikowski, A., Zimmermann, E., and Radespiel, U. (2019). First experimental evidence for olfactory species discrimination in two nocturnal primate species (Microcebus lehilahytsara and M. murinus). Sci. Rep. 9:20386. doi: 10.1038/s41598-019-56893-y
Korine, C., and Kalko, E. K. V. (2005). Fruit detection and discrimination by small fruit-eating bats (Phyllostomidae): echolocation call design and olfaction. Behav. Ecol. Sociobiol. 59, 12–23. doi: 10.1007/s00265-005-0003-1
Kruijt, B., Malhi, Y., Lloyd, J., Norbre, A. D., Miranda, A. C., Pereira, M. G. P., et al. (2000). Turbulence statistics above and within two Amazon rain forest canopies. Boundary-Layer Meteorol. 94, 297–331. doi: 10.1023/A:1002401829007
Kunz, T. H., Gauthreaux, S. A. Jr., Hristov, N. I., Horn, J. W., Jones, G., Kalko, E. K. V., et al. (2008). Aeroecology: probing and modeling the aerosphere. Int. Comp. Biol. 48, 1–11. doi: 10.1093/icb/icn037
Laska, M., Fendt, M., Wieser, A., Endres, T., Hernandez Salazar, L. T., and Apfelbach, R. (2005). Detecting danger—or just another odorant? olfactory sensitivity for the fox odor component 2,4,5-trimethylthiazoline in four species of mammals. Physiol. Behav. 84, 211–215. doi: 10.1016/j.physbeh.2004.11.006
Laundre, J., Hernández, L., and Ripple, W. (2010). The landscape of fear: ecological implications of being afraid. Open Ecol. J. 3, 1–7. doi: 10.2174/1874213001003030001
Lee, L. (2020). Nondetects and Data Analysis for Environmental Data. R Package Version 1.6-1.1. Available online at: https://CRAN.R-project.org/package=NADA (accessed September 1, 2021).
Leiser-Miller, L. B., Kaliszewska, Z. A., Lauterbur, M. E., Mann, B., Riffell, J. A., and Santana, S. E. (2020). A fruitful endeavor: scent cues and echolocation behavior used by Carollia castanea to find fruit. Int. Organismal Biol. 2:obaa007. doi: 10.1093/iob/obaa007
Lewis, S., Phillips, R. A., Burthe, S. J., Wanless, S., and Daunt, F. (2015). Contrasting responses of male and female foraging effort to year-round wind conditions. J. Animal Ecol. 84, 1490–1496. doi: 10.1111/1365-2656.12419
Lomáscolo, S. B., Levey, D. J., Kimball, R. T., Bolker, B. M., and Alborn, H. T. (2010). Dispersers shape fruit diversity in Ficus (Moraceae). Proc. Natl. Acad. Sci. U S A. 107, 14668–14672. doi: 10.1073/pnas.1008773107
Macdonald, D. W. (1980). Patterns of scent marking with urine and faeces amongst carnivore communities. Symposia Zool. Soc. London 45, 107–139.
McCay, M. G. (2003). Winds under the rain forest canopy: the aerodynamic environment of gliding tree frogs. Biotropica 35, 94–102. doi: 10.1111/j.1744-7429.2003.tb00266.x
McInnes, K. L., Erwin, T. A., and Bathols, J. M. (2011). Global climate model projected changes in 10 m wind speed and direction due to anthropogenic climate change. Atmospheric Sci. Lett. 12, 325–333. doi: 10.1002/asl.341
Melin, A. D., Hogan, J. D., Campos, F. A., Wikberg, E., King-Bailey, G., Webb, S., et al. (2020). Primate life history, social dynamics, ecology, and conservation: contributions from long-term research in Área de Conservación Guanacaste, Costa Rica. Biotropica 52, 1041–1064. doi: 10.1111/btp.12867
Monteith, J. L., and Unsworth, M. H. (2013). Principles of Environmental Physics: Plants, Animals, and the Atmosphere, 4th Edn. Amsterdam: Elsevier/Academic Press.
Murlis, J. (1997). “Odor plumes and the signal they provide,” in Insect Pheromone Research: New Directions, eds R. T. Cardé and A. K. Minks (Berlin: Springer), 221–231. doi: 10.1007/978-1-4615-6371-6_21
Murlis, J., Willis, M. A., and Cardé, R. T. (2000). Spatial and temporal structures of pheromone plumes in fields and forests. Physiol. Entomol. 25, 211–222. doi: 10.1046/j.1365-3032.2000.00176.x
Nathan, R., Schurr, F. M., Spiegel, O., Steinitz, O., Trakhtenbrot, A., and Tsoar, A. (2008). Mechanisms of long-distance seed dispersal. Trends Ecol. Evol. 23, 638–647. doi: 10.1016/j.tree.2008.08.003
Nevo, O., and Ayasse, M. (2020). “Fruit scent: biochemistry, ecological function, and evolution,” in Co-Evolution of Secondary Metabolites, eds J.-M. Mérillon and K. G. Ramawat (Berlin: Springer International Publishing), 403–425. doi: 10.1007/978-3-319-96397-6_33
Nevo, O., Razafimandimby, D., Jeffrey, J. A. J., Schulz, S., and Ayasse, M. (2018). Fruit scent as an evolved signal to primate seed dispersal. Sci. Adv. 4:eaat4871. doi: 10.1126/sciadv.aat4871
Nevo, O., Valenta, K., Kleiner, A., Razafimandimby, D., Jeffrey, J. A. J., Chapman, C. A., et al. (2020). The evolution of fruit scent: phylogenetic and developmental constraints. BMC Evol. Biol. 20:138. doi: 10.1186/s12862-020-01708-2
Niimura, Y., Matsui, A., and Touhara, K. (2018). Acceleration of olfactory receptor gene loss in primate evolution: possible link to anatomical change in sensory systems and dietary transition. Mol. Biol. Evol. 35, 1437–1450. doi: 10.1093/molbev/msy042
Oliver, H. R., and Mayhead, G. J. (1974). Wind measurements in a pine forest during a destructive gale. Forestry: Int. J. Forest Res. 47, 185–194. doi: 10.1093/forestry/47.2.185
Pleijel, H., Wallin, G., Karlsson, P. E., and Skärby, L. (1996). Ozone gradients in a spruce forest stand in relation to wind speed and time of the day. Atmospheric Environ. 30, 4077–4084. doi: 10.1016/1352-2310(96)00141-0
Powers, J. S., Becknell, J. M., Irving, J., and Pèrez-Aviles, D. (2009). Diversity and structure of regenerating tropical dry forests in Costa Rica: geographic patterns and environmental drivers. Forest Ecol. Manag. 258, 959–970. doi: 10.1016/j.foreco.2008.10.036
Pulliainen, E. (1981). Scent-marking in the pine marten (Maries martes) in Finnish Forest Lapland in winter. Zeitschrift Säugetierkunde 47, 91–99.
R Core Team (2021). R: A Language and Environment for Statistical Computing. Vienna: R Foundation for Statistical Computing.
Raguso, R. A., Levin, R. A., Foose, S. E., Holmberg, M. W., and McDade, L. A. (2003). Fragrance chemistry, nocturnal rhythms and pollination “syndromes” in Nicotiana. Phytochemistry 63, 265–284. doi: 10.1016/S0031-9422(03)00113-4
Rapp, J., and Silman, M. (2012). Diurnal, seasonal, and altitudinal trends in microclimate across a tropical montane cloud forest. Climate Res. 55, 17–32. doi: 10.3354/cr01127
Raynor, G. S. (1971). Wind and temperature structure in a coniferous forest and a contiguous field. Forest Sci. 17, 351–363.
Ripperger, S. P., Rehse, S., Wacker, S., Kalko, E. K. V., Schulz, S., Rodriguez-Herrera, B., et al. (2019). Nocturnal scent in a ‘bird-fig’: a cue to attract bats as additional dispersers? PLoS One 14:e0220461. doi: 10.1371/journal.pone.0220461
Rodríguez, A., Alquézar, B., and Peña, L. (2013). Fruit aromas in mature fleshy fruits as signals of readiness for predation and seed dispersal. New Phytol. 197, 36–48. doi: 10.1111/j.1469-8137.2012.04382.x
Ruzicka, R. E., and Conover, M. R. (2011). Influence of wind and humidity on foraging behavior of olfactory mesopredators. Canadian Field-Naturalist 125, 132–139. doi: 10.22621/cfn.v125i2.1196
Ruzicka, R. E., and Conover, M. R. (2012). Does weather or site characteristics influence the ability of scavengers to locate food? Ethology 118, 187–196. doi: 10.1111/j.1439-0310.2011.01997.x
Santana, S. E., Kaliszewska, Z. A., Leiser-Miller, L. B., Lauterbur, M. E., Arbour, J. H., Dávalos, L. M., et al. (2021). Fruit odorants mediate co-specialization in a multispecies plant-animal mutualism. Proc. R. Soc. B: Biol. Sci. 288:20210312. doi: 10.1098/rspb.2021.0312
Shukla, J., Nobre, C., and Sellers, P. (1990). Amazon deforestation and climate change. Science 247, 1322–1325. doi: 10.1126/science.247.4948.1322
Sillero-Zubiri, C., and Macdonald, D. W. (1998). Scent-marking and territorial behavior of ethiopian wolves Canis simensis. J. Zool. 245, 351–361. doi: 10.1111/j.1469-7998.1998.tb00110.x
Simmons, L. F. (1990). Time-series decomposition using the sinusoidal model. Int. J. Forecast. 6, 485–495. doi: 10.1016/0169-2070(90)90025-7
Svensson, G. P., Strandh, M., and Löfstedt, C. (2014). “Movements in the olfactory landscape,” in Animal Movements Across Scales, eds L.-A. Hansson and S. Åkesson (Oxford: Oxford University Press).
Therneau, T. (2021). A Package for Survival Analysis in R. R Package Version 3.2-13. Available online at: https://CRAN.R-project.org/package=survival (accessed September 1, 2021).
Thies, W., Kalko, E. K. V., and Schnitzler, H.-U. (1998). The roles of echolocation and olfaction in two neotropical fruit-eating bats, Carollia perspicillata and C. castanea, feeding on Piper. Behav. Ecol. Sociobiol. 42, 397–409. doi: 10.1007/s002650050454
Togunov, R. R., Derocher, A. E., and Lunn, N. J. (2017). Windscapes and olfactory foraging in a large carnivore. Sci. Rep. 7:46332. doi: 10.1038/srep46332
Usbeck, T., Wohlgemuth, T., Pfister, C., Volz, R., Beniston, M., and Dobbertin, M. (2010). Wind speed measurements and forest damage in Canton Zurich (Central Europe) from 1891 to winter 2007. Int. J. Climatol. 30, 347–358. doi: 10.1002/joc.1895
Vaglio, S., Minicozzi, P., Romoli, R., Boscaro, F., Pieraccini, G., Moneti, G., et al. (2016). Sternal gland scent-marking signals sex, age, rank, and group identity in captive mandrills. Chem. Senses 41, 177–186. doi: 10.1093/chemse/bjv077
Valenta, K., and Nevo, O. (2020). The dispersal syndrome hypothesis: how animals shaped fruit traits, and how they did not. Funct. Ecol. 34, 1158–1169. doi: 10.1111/1365-2435.13564
Valenta, K., Burke, R. J., Styler, S. A., Jackson, D. A., Melin, A. D., and Lehman, S. M. (2013). Colour and odor drive fruit selection and seed dispersal by mouse lemurs. Sci. Rep. 3:2424. doi: 10.1038/srep02424
Valenta, K., Kalbitzer, U., Razafimandimby, D., Omeja, P., Ayasse, M., Chapman, C. A., et al. (2018). The evolution of fruit colour: phylogeny, abiotic factors and the role of mutualists. Sci. Rep. 8:14302. doi: 10.1038/s41598-018-32604-x
Vieira, D. L. M., and Scariot, A. (2006). Principles of natural regeneration of tropical dry forests for restoration. Restoration Ecol. 14, 11–20. doi: 10.1111/j.1526-100X.2006.00100.x
Vogel, S. (1996). Life in Moving Fluids: The Physical Biology of Flow. Princeton, NJ: Princeton University Press.
Weldon, P. J. (1990). “Responses by vertebrates to chemicals from predators,” in Chemical Signals in Vertebrates V, eds D. W. MacDonald, D. Müller- Schwarze, and S. E. Natynczuk (New York, NY: Oxford University Press).
Wright, S. J., Trakhtenbrot, A., Bohrer, G., Detto, M., Katul, G. G., Horvitz, N., et al. (2008). Understanding strategies for seed dispersal by wind under contrasting atmospheric conditions. Proc. Natl. Acad. Sci. U S A. 105, 19084–19089. doi: 10.1073/pnas.0802697105
Young, I., Zieger, S., and Babanin, A. (2011). Global trends in wind speed and wave height. Science 332, 451–455. doi: 10.1126/science.1197219
Zhang, H., Henderson-Sellers, A., and McGuffie, K. (1996). Impacts of tropical deforestation. Part II: the role of large-scale dynamics. J. Climate 9, 2498–2521. doi: 10.1175/1520-0442
Keywords: aeroecology, olfactory ecology, tropical dry forest (bosque seco tropical), air speed, sensory landscape, sensory evolution
Citation: DePasquale A, Hogan JD, Guadamuz Araya C, Dominy NJ and Melin AD (2022) Aeroscapes and the Sensory Ecology of Olfaction in a Tropical Dry Forest. Front. Ecol. Evol. 10:849281. doi: 10.3389/fevo.2022.849281
Received: 05 January 2022; Accepted: 28 March 2022;
Published: 29 April 2022.
Edited by:
Felipe M. Gawryszewski, University of Brasilia, BrazilReviewed by:
Ben T. Hirsch, James Cook University, AustraliaRaúl Alberto Laumann, Embrapa Genetic Resources and Biotechnology, Brazil
Copyright © 2022 DePasquale, Hogan, Guadamuz Araya, Dominy and Melin. This is an open-access article distributed under the terms of the Creative Commons Attribution License (CC BY). The use, distribution or reproduction in other forums is permitted, provided the original author(s) and the copyright owner(s) are credited and that the original publication in this journal is cited, in accordance with accepted academic practice. No use, distribution or reproduction is permitted which does not comply with these terms.
*Correspondence: Allegra DePasquale, YWxsZWdyYS5kZXBhc3F1YWxlQHVjYWxnYXJ5LmNh; Amanda D. Melin, YW1hbmRhLm1lbGluQHVjYWxnYXJ5LmNh