- 1Chengdu Institute of Biology, Chinese Academy of Sciences, Chengdu, China
- 2College of Life Science, Sichuan Agricultural University, Ya’an, China
Whether and how poikilothermic animals change their thermal performance to cope with global warming are crucial questions to predict the future of biodiversity. Intraspecific comparison among populations that occur in different climatic zones can provide insight into how poikilotherms may alter their thermal performance under a particular climatic event. We compared populations of the Asiatic toad (Bufo gargarizans) from two altitudinal zones (3239 and 926 m above sea level) to explore variations of post-exercise hypothermia, which can lead to lower temperature preference than normal conditions. Common garden experiment was also employed to test plasticity of hypothermic performance in adult toads. As results, exhaustive exercise induced measurable reduction in body temperature for both populations. Furthermore, high-altitude population experienced larger reduction in body temperature than low-altitude conspecifics in both original habitat and common garden conditions. Therefore, low-altitude toads may to enhance their hypothermic reaction if they shift their ranges to higher altitudes to survive warming climate; However, the relatively limited plasticity of hypothermic performance may constraint their adaptative process.
Introduction
Upward range shift has been recorded in numerous organisms as a response to climatic change in recent decades since cooler environments at higher altitudes may relieve them from overheating (Parmesan and Yohe, 2003; Molina-Martínez et al., 2016; Freeman et al., 2018). During this process, high-altitude environments may impose other severe physiological stressors to species, which may represent new challenges for their survival and reproduction, including hypoxia and intense UV radiation (Bouverot, 1985; Storz et al., 2010; Qiu et al., 2012). Knowledge on how low-altitude poikilothermic organisms cope with high-altitude environments, however, remains limited, which may impede our understanding of the processes and mechanisms of how species react and evolve under climatic change (Pinsky et al., 2019; Sunday et al., 2019; Feldmeier et al., 2020; Jacobsen, 2020).
Body temperature of poikilothermic animals is largely dependent on environmental temperature (Gracey et al., 2004; Harwood, 2007). Meanwhile, body temperature is a key parameter for poikilothermic animals, and has a significant influence on animal physiological processes including energy metabolism, respiration, acid-base balance, and enzyme activity, of which are all closely correlated (Withers, 1978; Vitt and Caldwell, 2014; Abram et al., 2017; Gangloff et al., 2019; Taylor et al., 2021). Consequently, each poikilothermic species has its own optimal temperature range, and they typically reach their ranges by physiological or behavioral thermoregulation (Vitt and Caldwell, 2014; Rozen-Rechels et al., 2019). Because environmental temperature changes along with altitudinal gradient, poikilothermic animals that live in high-altitudes have adapted to the cold environments by regulating their thermal regimes (Bouverot, 1985; Muir et al., 2014; Domínguez-Godoy et al., 2020). Therefore, understanding the variations of preferred temperature and thermoregulation behavior among high- and low-altitude populations is crucial for apprehending the effects of climatic change on range shift of poikilothermic animals (Trochet et al., 2018).
Hypothermia results in a low temperature preference and can be achieved through thermoregulation behavior in poikilothermic vertebrates, including amphibians, reptiles, and fish (Wood and Gonzales, 1996; Moretti et al., 2018; Jones et al., 2019; Duran et al., 2020; Skandalis et al., 2020). Hypothermia can be induced by exhaustive exercise (Tattersall and Boutilier, 1999), hypoxia (Branco et al., 2014; Skandalis et al., 2020), or other stimulations (Moretti et al., 2018; Duran et al., 2020; Skandalis et al., 2020), and is known as lower body temperature than normal state (Romanovsky et al., 2005). Several physiological mediators of low body temperature in poikilotherms have been uncovered, including acidosis and lactate (Wagner et al., 1999; Nedrow et al., 2001). How animals benefit from hypothermia, however, remains controversial. Decreasing body temperature can shift the oxygen dissociation curve leftward, which increases oxygen loading in lungs, reduces energetic cost of ventilatory and cardiac hyperactivity, and restores acid-base balance in blood and tissue (Withers, 1978; Wang et al., 1998; Petersen et al., 2003). Thus, low body temperature in hypoxia likely represents an adaptive response to protect vital organs and reduce energy expenditure (Wood and Gonzales, 1996; Petersen et al., 2003). Furthermore, hypothermia may be a protective mechanism in hypoxic condition, such as at high-altitudes, which can increase survival rate (Bicego et al., 2007; Branco et al., 2014).
Upward range shift in poikilothermic animals has been a key research topic in recent decades (Gangloff et al., 2019; Domínguez-Godoy et al., 2020). Hence, studies on variations of natural thermal performance and tolerance, and the mechanisms behind the formation of variation are highly desirable, especially thermal biology on variations among individuals and populations within species (Taylor et al., 2021). High-altitude environmental stressor, particularly hypoxia and cold, impose significant constraints on animal physiology. Hypoxia typically has an negative impact on life activities, such as reproduction and development (Souchet et al., 2020). How animals living at different altitudes respond to their environments, and how they may change their thermal physiological reaction norms are fascinating questions. Therefore, intraspecific comparison between high- and low-altitudinal populations of poikilotherms represents an ideal paradigm to explore the variations of thermal biology and enhance understanding of how poikilothermic animals cope with climatic change.
Anurans are typical poikilothermic vertebrates, and are sensitive to rapidly elevating environmental temperature, which makes them excellent models for studying impacts of changing environmental temperature (Bodensteiner et al., 2021; Taylor et al., 2021). The Asiatic Toad (Bufo gargarizans) is a common anuran species that has a wide distribution in east Asia, and a wide altitudinal range from sea level to over 4,000 m above sea level (a.s.l.; AmphibiaChina, 2020). Therefore, we choose Asiatic toads that live in different altitudes to test intraspecific variations of hypothermic performance.
In this study, we used an exhaustive-exercise induced hypothermia and compared hypothermic performance between high- and low-altitudinal populations of Asiatic toads, both at their original habitat and in a common garden environment. We hypothesized that the high-altitude toads would have a stronger hypothermic reaction (lower body temperature) to exhaustive exercise than the low-altitude toads do, because cold temperature and hypoxia at high-altitudes may interactively affect their thermal physiology and high-altitudes toads may have adapted or acclimated to their environments. Besides, modifications of their thermal physiology could be fixed (evolutionary adaptation) or plastic (phenotypic plasticity).
Materials and Methods
Animal Sampling and Experimental Design
Adult Asiatic toads were sampled from a high-altitude site (Kangding, 3239 m a.s.l.) and a low-altitude site (Shimian, 926 m a.s.l.; Figure 1A) in Sichuan province of western China during breeding season of 2020. Four males and four females were collected from each site. Because of the delayed phenology at high altitudes, low-altitudinal adults (origin-L) were sampled in January, while high-altitudinal adults (origin-H) were sampled in April (Table 1 and Figure 1B).
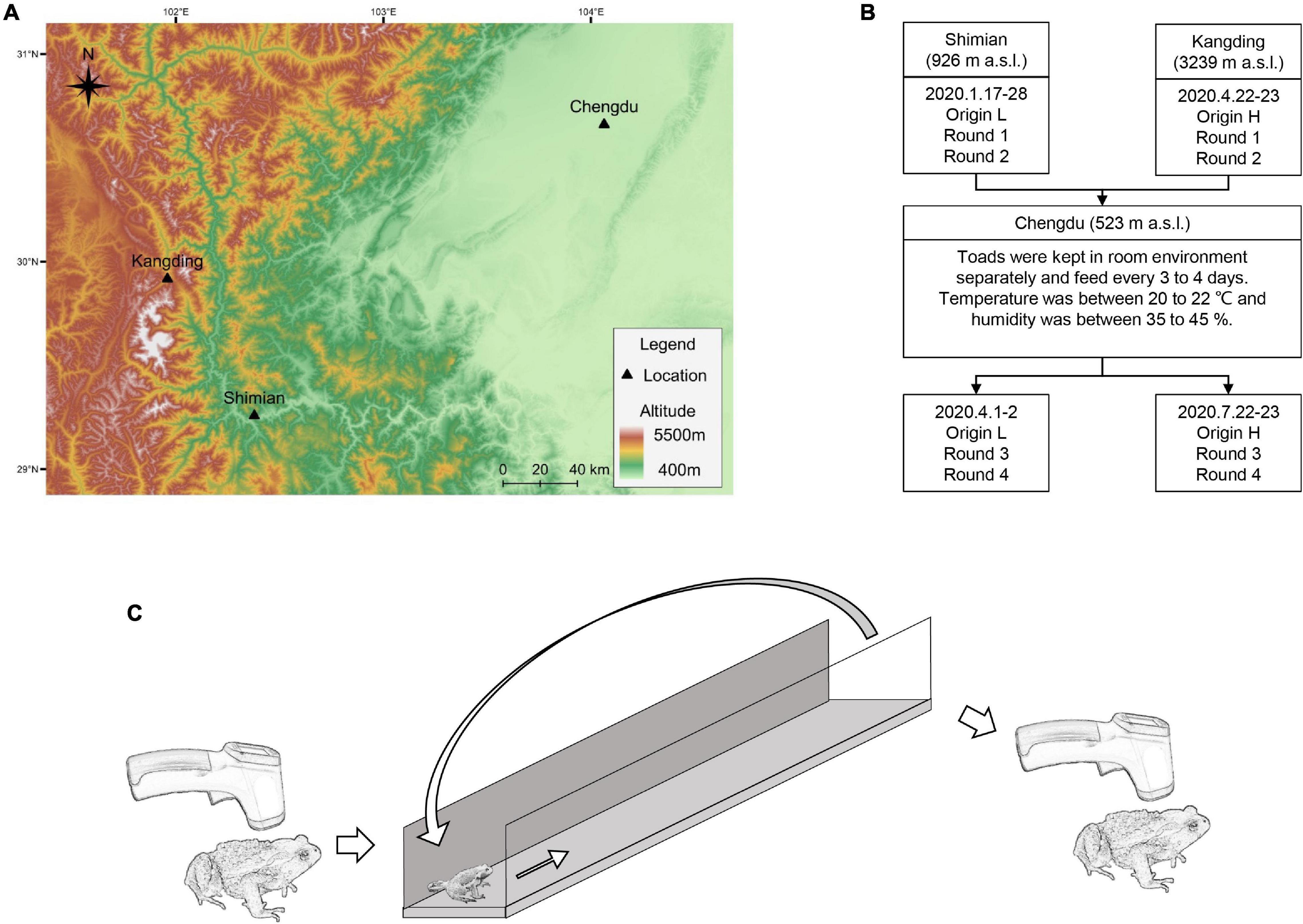
Figure 1. Experimental protocols. (A) Sampling sites and common garden site. (B) Experimental schedule. (C) Setup of racetrack trial.
We used racetrack trials as the exhaustive exercise, which could lead to post-exercise hypothermia. Racetrack trial was a common method in testing animal locomotor performance (Llewelyn et al., 2010; Hudson et al., 2020; Niu et al., 2021; Wölfer et al., 2021) and was also reliable in testing anurans (Zamora-Camacho, 2018; Rebelo and Measey, 2019; Hudson et al., 2020). Two rounds (round 1 and round 2) of trials were conducted near the sampling sites approximately 48 h after capture. The trials were conducted in January 17 to 18 near Shimian, and in April 22 to 23 near Kangding (Table 1 and Figure 1B). The toads were then moved to the laboratory at the Chengdu Institute of Biology (Chengdu, Sichuan, 523 m a.s.l.) and acclimatized in a common garden environment for at least 2 months. Two rounds of trials (round 3 and round 4) were conducted after the acclimation period in April 1 and 2 with the origin-L individuals, and in July 22 and 23 with the origin-H individuals (Table 1 and Figure 1B).
Common Garden Husbandry
The toads were kept individually in boxes with a size of 35 cm × 24 cm ×14.5 cm (length × width × height), and were fed with mealworms (Tenebrio molitor). Mealworms were replenished every three to four days, and at the same time the boxes were cleaned. Calcium powder and vitamin powder were added with the mealworms every half month. Refuge and moist sponge mat were also provided to each toad, and the sponge was rehydration every three or four days. Dark and light rhythm was kept at 12:12 h. Meanwhile, the temperature of husbandry room was between 20 to 22°C, and humidity was between 35 % to 45 %.
Exhaustive Exercise and Racetrack Trial
The racetrack was made of a wood board floor with a length of 120 cm and a width of 15 cm, and two acrylic side walls with a height of 30 cm (Figure 1C). The racetrack trials were conducted at night between 10 p.m. to 4 a.m. During the trials, toads were released at one end (start point) of the racetrack and allowed to move along racetrack to the other end (end point). A blunt-pointed pen was used to stimulate at the caudal vertebra area of the toads when they stopped (Hudson et al., 2020). Toads were manually moved (with thick gloves to prevent heat conduction) back to the start point to continue the exercise once they arrived at the end point. Exhaustive exercise was terminated after 10 continued pokes without movement, and the toad was considered as exhausted or unwilling to move (Hudson et al., 2020). From round 1 to round 4, a total of 64 trials were completed with 16 individuals. Each animal ran the trial once in each round with randomized orders. Moved distance was recorded for each subject after each trial. Surface body temperature was measured at the center of upper back immediately before and after each trial using an infrared thermometer (FR830, JIACOM) to the nearest 0.1°C. Environmental air temperature and humidity during racetrack trial were controlled at between 20–22°C and 35–55% and recorded (Table 1). The temperature of the runway floor was also recorded. The reduction values of surface body temperature (ΔTS) were estimated as the after-exercise temperature (TS2) minus the before-exercise temperature (TS1) of each trial. To control for personnel effects, all trials was conducted by the same investigator (KH).
To test if surface body temperature could reflect core body temperature well, another two rounds (round 5 and round 6) of trials were conducted in September 20 and 21 (Table 1). Ten males of each population were chosen, of which five males had been tested before and the others were novice. In round 5, ten individuals were tested and four of them were poked (between 1 to 2 min) but not allowed to run as negative control. In round 6, 20 individuals were tested and eight of them were negative controls (between 1 to 5 min). A thermometer (UT321, UNI-T) was used to measure cloacal temperature to the nearest 0.1°C (Withers, 1978; Wagner et al., 1999; Duran et al., 2020) before and after each trial, in addition to surface temperature. The reduction of core body temperature (ΔTC) was calculated in the same way as ΔTS.
Before each trial, toads were weighted using a digital balance (I-2000, MAXN) to the nearest 0.1 g. A photo with scale was taken for each toad from the back view with a camera (HDR PJ680, Sony) and then the photo was used to measure the snout-vent-length (SVL) to the nearest 0.001 cm using ImageJ 1.53 g (Abramoff et al., 2004).
Statistical Analysis
All continuous variables, including body-mass, SVL, distance, ΔTS, and ΔTC, were scaled before statistical analysis. To test how well ΔTS variation reflect ΔTC variation, general linear models (GLM) were constructed using package lme4 (Bates et al., 2014). ΔTC was the dependent variable and ΔTS was the independent variable. Model assumptions, including homoscedasticity and normality of residuals, were tested with plots. The outliers that violate model assumptions were removed and models were reconstructed (Supplementary Tables 1, 2).
To test the correlation between moved distance and temperature reduction, a polynomial regression was conducted using package lme4, in which ΔTS was the dependent variable (Supplementary Table 1). Distance was the independent variable, which was transformed to binomial expression. There was no violation on model assumptions.
To test effects of all the considered factors on ΔTS and their interactions, GLMs were constructed. ΔTS was set as the dependent variable, while the independent variables including distance, origin (population), round, location (places of racetrack trials), sex, body-mass, and SVL (Supplementary Table 1). A second model was constructed without body-mass, SVL, and sex, as they were not the target factors and had no significant effect on ΔTS (Supplementary Table 1). Model comparison was conducted with the method of Analysis of Variance (ANOVA), and it indicated that there was no significant difference between the two models (Supplementary Table 3). Therefore, model without body-mass, SVL, and sex was selected. Because of the potential interactive effects among the independent variables, interactive effects were introduced into the models (Supplementary Table 1). Six combinations of interactive predictors were included:
1) Location × Round
2) Origin × Location × Round
3) Distance × Origin
4) Distance × Origin + Location × Round
5) Distance × Origin × Location
6) Distance × Origin × Location × Round
Model comparison showed that the fourth combination had the lowest AIC score (Supplementary Table 4), and therefore, the final model had two interactive effects. Model assumptions were also checked and there was no violation.
We also tested differences of surface body temperature between origin-H and origin-L toads before and after exercise in each trial from round 1 to round 4, as supplementary to model selection. When the scaled temperature data passed tests of normal distribution and homogeneity of variance, an independent-sample t-test was conducted. When the data did not pass the tests, an unpaired two-sample Wilcoxon test was conducted.
To test the correlation between moved distance and origin, location, and rounds, GLMs were constructed. The distance was the dependent variable, and origin, location, rounds were set as the independent variables (Supplementary Table 1). Model assumptions were also checked and there was no violation.
All analysis was performed using R 4.1.2 (R Core Team, 2020). For visualization, linear models and non-linear models were plotted with package ggplot2 (Wickham, 2016, 2) and model predictions were extracted using package ggeffects (Lüdecke, 2018). The package interactions (Long, 2019) was used to plot interactive effects of models.
Results
The average moved distance of the origin-L population in rounds 1 and 2 (total trial n = 16) was 44.87 ± 37.73 m (mean ± sd), and in rounds 3 and 4 (total trial n = 16) was 61.33 ± 33.40 m. The same measurements of the origin-H population were 14.35 ± 10.51 m and 17.08 ± 10.06 m for rounds 1 and 2 and for rounds 3 and 4, respectively. The ΔTS of the origin L population in round 1 and round 2 was –1.41 ± 0.76°C, and in round 3 and round 4 was –3.01 ± 1.05. The same measurements of the origin H population were 0.11 ± 0.93°C and –3.05 ± 0.71°C for rounds 1 and 2 and for rounds 3 and 4, respectively.
In rounds 5 and 6, the ΔTS was –1.61 ± 0.61°C in the experimental group, and was 0.06 ± 0.31°C in the control group. The ΔTC in the two groups were –1.17 ± 0.58°C and 0.08 ± 0.17°C, respectively. The control group were not allowed to move in trials, so the moved distance was zero, and the distance of the experimental group was 24.65 ± 28.52 m.
Furthermore, the adjusted R2 from the GLM between ΔTS and ΔTC was 0.90. After removing three outliers, the adjusted R2 increased to 0.93 (Supplementary Table 2). Therefore, variations between the two measurements were within an acceptable level (estimate ± se = 1.363 ± 0.074; p < 0.01; Table 2 and Figure 2A), and ΔTS was a valid substitute for ΔTC.
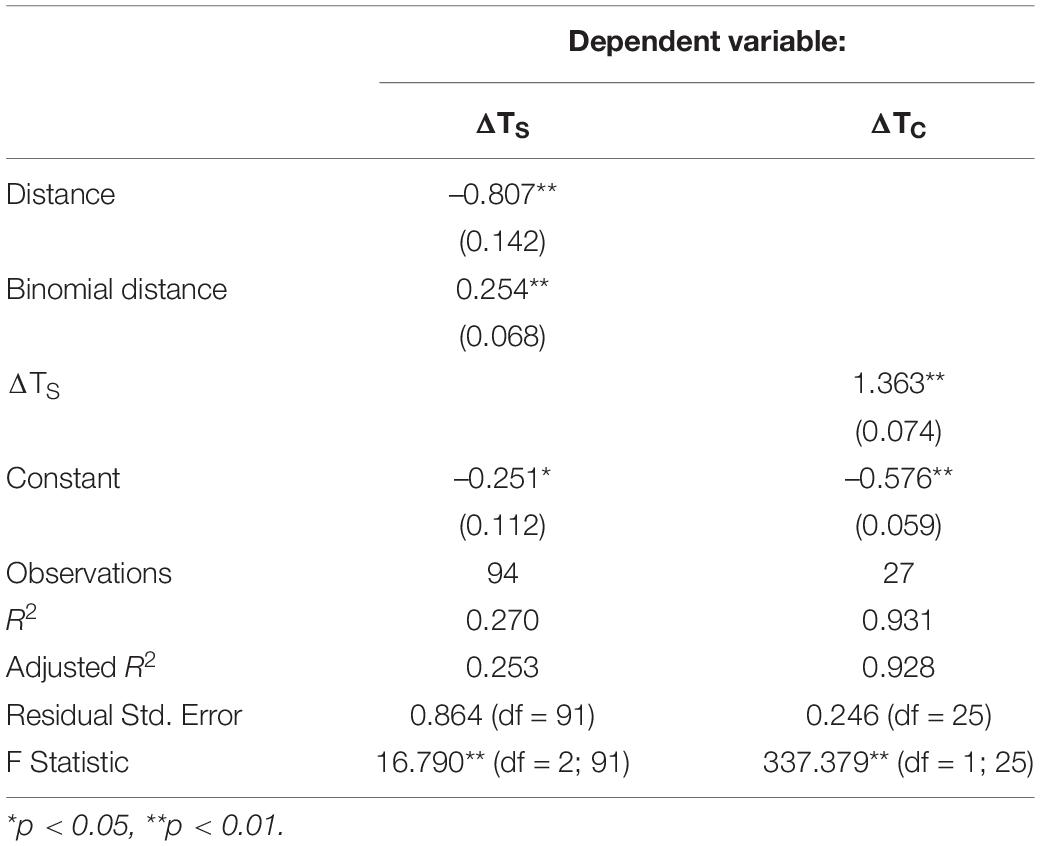
Table 2. Results of polynomial regression between distance and ΔTS; and linear regression between ΔTS and ΔTC.
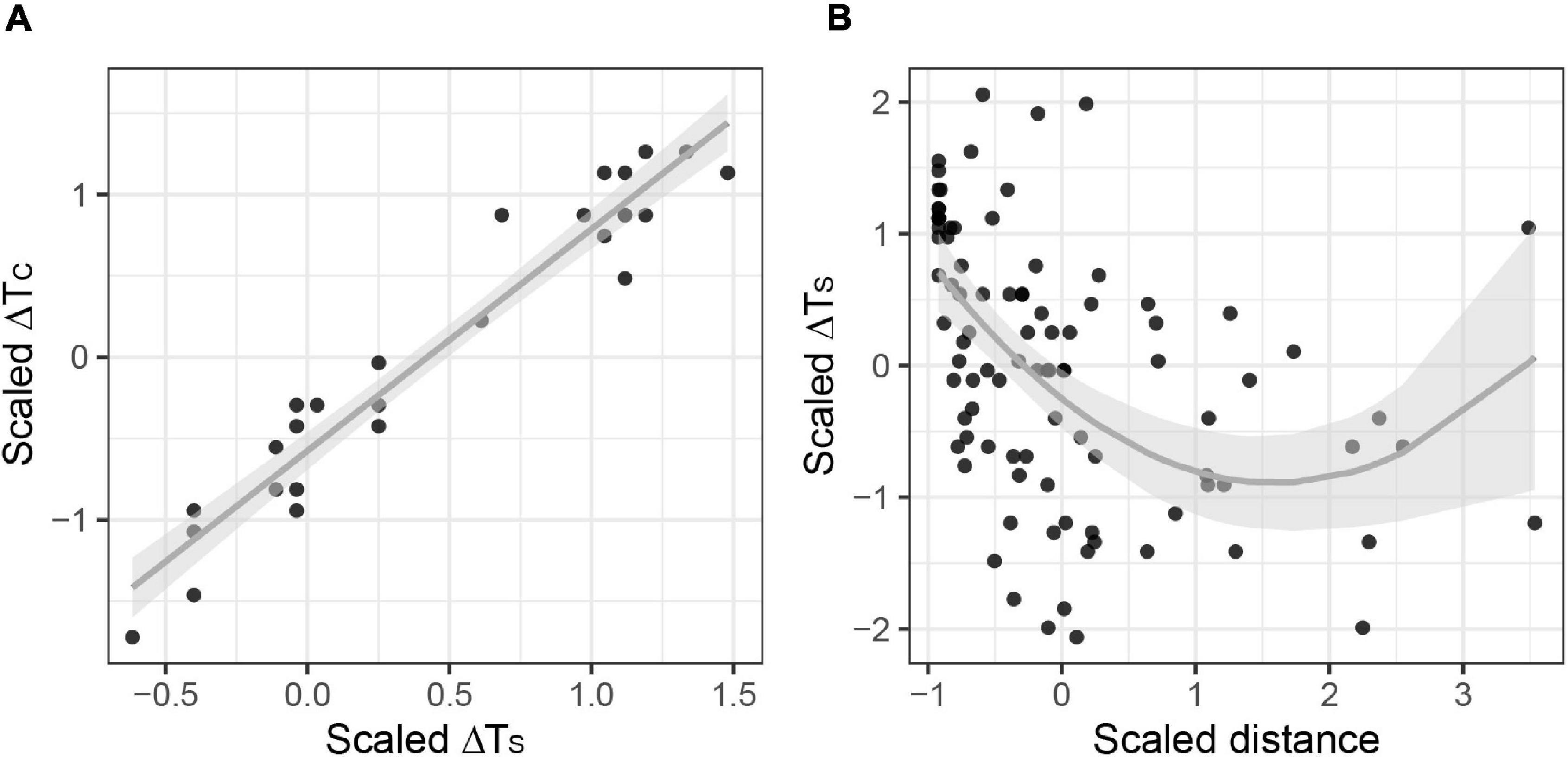
Figure 2. (A) Linear relationship between ΔTS and ΔTC. (B) Binomial regressions between moved distance and ΔTS.
Model analysis indicated a non-linear correlation between quadratic moved distance and ΔTS (0.254 ± 0.068, p < 0.01; –0.807 ± 0.142, p < 0.01; Table 2 and Figure 2B). The results clearly demonstrated that exhaustive exercise caused hypothermia. Body temperature reduction increased with prolonged moved distance, but the slope of changing temperature gradually became flat along with the distance.
The constructed linear model, which contained two interactive effects, supported a significant effect of all independent variables on ΔTS. The total moved distance was negatively correlated with ΔTS (–0.731 ± 0.299, p < 0.01; Table 3 and Figure 3A), which was concordant with the polynomial regression. Round was positively correlated with ΔTS (0.377 ± 0.187, p < 0.05; Table 3 and Figure 3B), which meant the ΔTS was significantly increased in round 2 and round 4, compared to round 1 and round 3, except at the Kangding location (Figure 3B). When compared to the origin-H population, the origin-L population had a positive relationship with ΔTS (0.579 ± 0.223, p < 0.05; Table 3 and Figure 3A). When compared to the Chengdu location, the Kangding location had a significantly positive correlation with ΔTS (4.804 ± 0.787, p < 0.01; Table 3 and Figure 3B), but the Shimian location was not (0.853 ± 0.784, p < 0.05; Table 3).
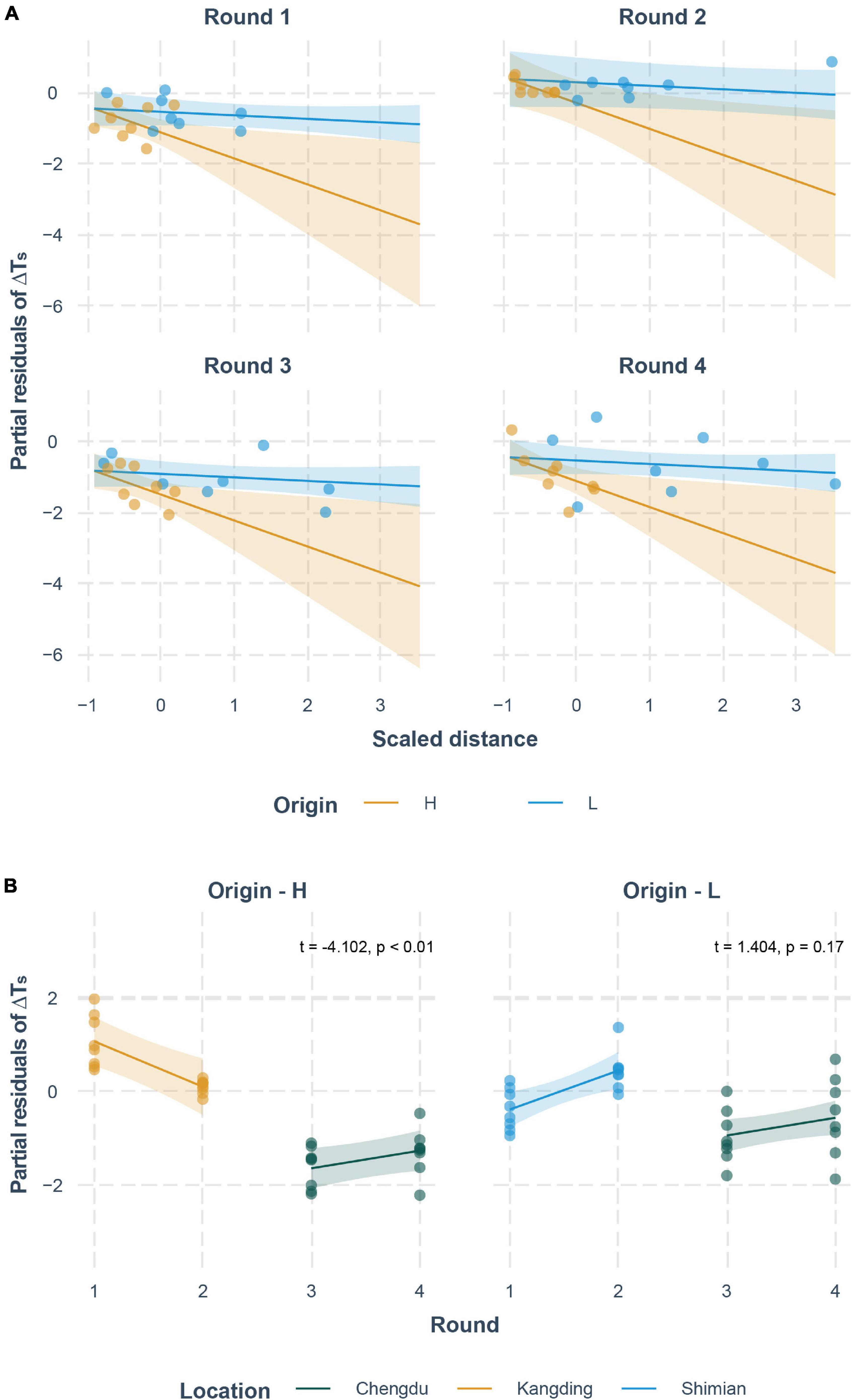
Figure 3. Correlation between interactive effects and ΔTS. (A) Interactive effect of distance and origin on ΔTS. (B) Interactive effect of round and location on ΔTS. The partial residuals of ΔTS are plotted after controlling for effects of all controlled variables.
A better way to understand the results was through the interactive effects. Interactive effect of distance and origin was significantly correlated with ΔTS (distance × Shimian: 0.632 ± 0.312, p < 0.05; Table 3), which meant when moving the same distance, a toad from the origin-L group had a larger ΔTS than a toad from the origin-H group (Figure 3A). Another set of significantly interactive variables was round and location (round × Kangding: –1.344 ± 0.328, p < 0.01; Table 3), which predicted a negative relationship between round and ΔTS when the racetrack trials were conducted in Kangding, and the slope was neither similar to slope in Shimian nor similar to slope in Chengdu (Figure 3B).
The origin-H toads had lower body temperature before exercise than origin-L toads did in round 1 and round 2 (round 1: W = 0, p < 0.001; round 2: W = 11, p < 0.05; Supplementary Table 5 and Supplementary Figure 1A). However, origin-H toads had higher body temperature than origin-L toads did in rounds 3 and 4 (round 3: T = 2.147, p < 0.05; round 4: W = 56, p < 0.05; Supplementary Table 5 and Supplementary Figure 1A). As for the post-exercise body temperature, there was no significant difference between the two population (Supplementary Table 5 and Supplementary Figure 1B).
Furthermore, origin had significant correlation with moved distance from the linear model (origin-L: 1.289 ± 0.287, p < 0.01; Supplementary Table 6 and Supplementary Figure 2).
Discussion
Our results showed that high-altitude population of Asiatic toads had a stronger hypothermic reaction to exhaustive exercise than low-altitude conspecific toads did. The high-altitude population had lower body temperature at round 2 than round 1 at its original habitat, which was different from toads of low-altitude. When the effect of moved distance was controlled, the high-altitude population had larger body temperature reduction than low-altitude population at both original habitats and common garden location. In other words, high-altitude population did not change the extent of hypothermic reaction to exhaustive exercise after acclimation.
Our results confirm the exhaustive-exercise induced hypothermia in the Asiatic Toad, a phenomenon that has previously been reported for a few other poikilothermic animals (Petersen et al., 2003). Behavioral hypothermia has been proposed as a protective strategy to reduce metabolic demands during hypoxia or after exercise (Wagner et al., 1999; Hicks and Wang, 2004; Branco et al., 2006). Poikilothermic animals in hypothermic state may reduce oxygen consumption, increase affinity of hemoglobin for oxygen, and reduce energetically costly responses (Steiner and Branco, 2002). In turtles (Chrysemys picta), for instance, the oxygen-hemoglobin dissociation curve shifts rightward at high temperatures, meaning that oxygen saturation changes to a lower level at the same PO2 at high temperature than at low temperature (da Silva et al., 2013). Besides, glucose metabolism is positively correlated with oxygen availability (Gullino et al., 1968; Storz and McClelland, 2017), and experiment on vitro muscle of the American Bullfrog (Lithobates catesbeianus) suggests that net rate of glucose metabolism at low temperature is reduced compared to that at high temperature (Petersen and Gleeson, 2009). Therefore, hypothermia may increase survival rate of poikilothermic animals under various metabolism interruptions such as hypoxia or exhaustion (Wood and Gonzales, 1996; Petersen et al., 2003; Morris, 2004).
An interesting difference between high- and low-altitude toads is the predicted slope between moved distance and body temperature reduction, which indicates a lower body temperature of high-altitude toads than that of low-altitude conspecifics when moving the same distance. We postulate that this variation may represent an adaptative shift of hypothermic performance in high-altitude environments. For poikilothermic animals living at high altitudes, the cost of allocating energy to thermoregulation to achieve a high body temperature as a response to stress (e.g., infection) may offset the benefits of the high body temperature in cold and hypoxic environments (Duran et al., 2020). Thus, these poikilothermic animals tend to lower their body temperature to save energy for other more important life activities (Duran et al., 2020). On the other hand, oxygen uptake at resting state changes along with body temperature in most animals (Deluen et al., 2022), and animals in a hypoxic environment may suffer from hyperthermia (Wood and Gonzales, 1996). In turn, hyperthermia favors higher metabolism and promotes body recovery but also demands higher oxygen uptake. Amphibians like Bufo marinus have a low temperature preference during hypoxic exposure, and this behavioral hypothermia increases arterial saturation and reduces oxygen uptake (Wood and Malvin, 1991). For poikilothermic vertebrates, low temperature also eases ventilatory responses in hypoxic conditions (da Silva et al., 2013). Another possible explanation is the metabolic cold adaptation (MCA) hypothesis, which suggests poikilotherms in cold environments would have a high standard metabolic rate to ensure life activity at low temperatures (Gaston et al., 2009; Deluen et al., 2022). High-altitude populations of the Pyrenean Brook Newts have a higher baseline of the relationship between standard metabolic rate and body temperature compared to low-altitude populations, which supports the MCA (Deluen et al., 2022). Thus, the high-altitude toads may have a metabolic level at a low body temperature similar to that of low-altitude toads at a high body temperature. Hence, more exploration on the physiological significance of hypothermic performance in high-altitudinal poikilotherms is needed.
The observed variations of hypothermic reaction may predict a potential changing direction for low-altitude populations when facing warming climate. Warming temperature affects important physiological processes such as metabolism, thus increased temperature may push poikilothermic organisms to higher altitudes or latitudes for cooler environments (da Silva et al., 2013; Gangloff et al., 2019; Feldmeier et al., 2020; Jacobsen, 2020). Low-altitude toads may change their hypothermic performance to match their high-altitude conspecifics when they shift to high-altitude environments.
Another key challenge to poikilotherms is whether thermal trait modifications can keep pace with the warming climate (Bodensteiner et al., 2021). Poikilotherms have a relatively low plasticity in thermal tolerance, which means physiological adjustments of thermoregulation may not be sufficient for poikilotherms when facing rapid climate warming (Gunderson and Stillman, 2015; Gangloff et al., 2019). According to the common garden comparison of toad populations, both high- and low-altitude toads retain their own extents of hypothermic reaction. Thus, coping with climate change relying on plasticity of hypothermic performance may not be optimistic for the Asiatic Toad.
Conclusion
Exhausting-exercise induces a hypothermic response in both high- and low-altitude toads, and this reaction leads to measurable reduction in body temperature. Besides, high-altitude toads have lower body temperature than low-altitude conspecifics do when moving the same distance. However, the common garden comparison suggests minimum capacity on plasticity of hypothermic performance for both high- and low-altitude toad populations.
Data Availability Statement
The original contributions presented in the study are included in the article/Supplementary Material, further inquiries can be directed to the corresponding author/s.
Ethics Statement
The animal study was reviewed and approved by the Animal Care and Use Committee at the Chengdu Institute of Biology, Chinese Academy of Sciences.
Author Contributions
KH: conceptualization and data curation. ZY and KH: methodology. ZY: software, formal analysis, writing – original draft, and visualization. YQ: investigation, supervision, and project administration. ZY and YQ: writing – review and editing. All authors read, edited, and approved the final submitted version of the manuscript.
Funding
This project was supported by a National Natural Science Foundation of China (NSFC) grant (31729003).
Conflict of Interest
The authors declare that the research was conducted in the absence of any commercial or financial relationships that could be construed as a potential conflict of interest.
Publisher’s Note
All claims expressed in this article are solely those of the authors and do not necessarily represent those of their affiliated organizations, or those of the publisher, the editors and the reviewers. Any product that may be evaluated in this article, or claim that may be made by its manufacturer, is not guaranteed or endorsed by the publisher.
Acknowledgments
We would like to thank X. Zhu and Q. Yang for their assistant in animal husbandry. X. Qiu provided helpful suggestions statistical analysis. We also thank S. Tan for suggestions in experiment design. W. Yang and J. Fu kindly edited several versions of our manuscript.
Supplementary Material
The Supplementary Material for this article can be found online at: https://www.frontiersin.org/articles/10.3389/fevo.2022.846663/full#supplementary-material
References
Abram, P. K., Boivin, G., Moiroux, J., and Brodeur, J. (2017). Behavioural effects of temperature on ectothermic animals: unifying thermal physiology and behavioural plasticity. Biol. Rev. 92, 1859–1876. doi: 10.1111/brv.12312
Abramoff, M. D., Magalhães, P. J., and Ram, S. J. (2004). Image processing with imageJ. Biophotonics Int. 11, 36–42.
AmphibiaChina (2020). The Database of Chinese Amphibians. Kunming, China.: Kunming Institute of Zoology (CAS). Available online at: http://www.amphibiachina.org/ (accessed November 13, 2019).
Bates, D., Mächler, M., Bolker, B., and Walker, S. (2014). Fitting linear mixed-effects models using lme4. arXiv [preprint]. arXiv:1406.5823,Google Scholar
Bicego, K. C., Barros, R. C. H., and Branco, L. G. S. (2007). Physiology of temperature regulation: comparative aspects. Comp. Biochem. Physiol. A Mol. Integr. Physiol. 147, 616–639. doi: 10.1016/j.cbpa.2006.06.032
Bodensteiner, B. L., Agudelo-Cantero, G. A., Arietta, A. Z. A., Gunderson, A. R., Muñoz, M. M., Refsnider, J. M., et al. (2021). Thermal adaptation revisited: how conserved are thermal traits of reptiles and amphibians? J. Exp. Zool. Part A Ecol. Integr. Physiol. 335, 173–194. doi: 10.1002/jez.2414
Branco, L. G. S., Gargaglioni, L. H., and Barros, R. C. H. (2006). Anapyrexia during hypoxia. J. Therm. Biol. 31, 82–89. doi: 10.1016/j.jtherbio.2005.11.020
Branco, L. G. S., Soriano, R. N., and Steiner, A. A. (2014). Gaseous mediators in temperature regulation. Compr. Physiol. 4, 1301–1338. doi: 10.1002/cphy.c130053
da Silva, G. S. F., Glass, M. L., and Branco, L. G. S. (2013). Temperature and respiratory function in ectothermic vertebrates. J. Therm. Biol. 38, 55–63. doi: 10.1016/j.jtherbio.2012.11.001
Deluen, M., Blanchet, S., Aubret, F., Trochet, A., Gangloff, E. J., Guillaume, O., et al. (2022). Impacts of temperature on O2 consumption of the Pyrenean brook newt (Calotriton asper) from populations along an elevational gradient. J. Therm. Biol. 103:103166. doi: 10.1016/j.jtherbio.2021.103166
Domínguez-Godoy, M. A., Hudson, R., Pérez-Mendoza, H. A., Ancona, S., and Díaz de la Vega-Pérez, A. H. (2020). Living on the edge: lower thermal quality but greater survival probability at a high altitude mountain for the mesquite lizard (Sceloporus grammicus). J. Therm. Biol. 94:102757. doi: 10.1016/j.jtherbio.2020.102757
Duran, F., Boretto, J. M., and Ibargüengoytía, N. R. (2020). Decrease in preferred temperature in response to an immune challenge in lizards from cold environments in Patagonia, Argentina. J. Therm. Biol. 93:102706. doi: 10.1016/j.jtherbio.2020.102706
Feldmeier, S., Schmidt, B. R., Zimmermann, N. E., Veith, M., Ficetola, G. F., and Lötters, S. (2020). Shifting aspect or elevation? The climate change response of ectotherms in a complex mountain topography. Divers. Distrib. 26, 1483–1495. doi: 10.1111/ddi.13146
Freeman, B. G., Lee-Yaw, J. A., Sunday, J. M., and Hargreaves, A. L. (2018). Expanding, shifting and shrinking: the impact of global warming on species’ elevational distributions. Glob. Ecol. Biogeogr. 27, 1268–1276. doi: 10.1111/geb.12774
Gangloff, E. J., Sorlin, M., Cordero, G. A., Souchet, J., and Aubret, F. (2019). Lizards at the peak: physiological plasticity does not maintain performance in lizards transplanted to high altitude. Physiol. Biochem. Zool. 92, 189–200. doi: 10.1086/701793
Gaston, K. J., Chown, S. L., Calosi, P., Bernardo, J., Bilton, D. T., Clarke, A., et al. (2009). Macrophysiology: a conceptual reunification. Am. Nat. 174, 595–612. doi: 10.1086/605982
Gracey, A. Y., Fraser, E. J., Li, W., Fang, Y., Taylor, R. R., Rogers, J., et al. (2004). Coping with cold: an integrative, multitissue analysis of the transcriptome of a poikilothermic vertebrate. Proc. Natl. Acad. Sci. U.S.A. 101, 16970–16975. doi: 10.1073/pnas.0403627101
Gullino, P. M., Grantham, F. H., Courtney, A. H., and Losonczy, I. (1968). Relationship between oxygen and glucose consumption by transplanted tumors in vivo. Transplantation 6:140.
Gunderson, A. R., and Stillman, J. H. (2015). Plasticity in thermal tolerance has limited potential to buffer ectotherms from global warming. Proc. R. Soc. B 282:20150401. doi: 10.1098/rspb.2015.0401
Harwood, J. L. (2007). Temperature stress: reacting and adapting: lessons from Poikilotherms. Ann. N. Y. Acad. Sci. 1113, 52–57. doi: 10.1196/annals.1391.025
Hicks, J. W., and Wang, T. (2004). Hypometabolism in reptiles: behavioural and physiological mechanisms that reduce aerobic demands. Respir. Physiol. Neurobiol. 141, 261–271. doi: 10.1016/j.resp.2004.03.012
Hudson, C. M., Vidal-García, M., Murray, T. G., and Shine, R. (2020). The accelerating anuran: evolution of locomotor performance in cane toads (Rhinella marina, Bufonidae) at an invasion front. Proc. R. Soc. B 287:20201964. doi: 10.1098/rspb.2020.1964
Jacobsen, D. (2020). The dilemma of altitudinal shifts: caught between high temperature and low oxygen. Front. Ecol. Environ. 18:211–218. doi: 10.1002/fee.2161
Jones, N. A. R., Mendo, T., Broell, F., and Webster, M. M. (2019). No experimental evidence of stress-induced hyperthermia in zebrafish (Danio rerio). J. Exp. Biol. 222:jeb192971. doi: 10.1242/jeb.192971
Llewelyn, J., Phillips, B. L., Alford, R. A., Schwarzkopf, L., and Shine, R. (2010). Locomotor performance in an invasive species: cane toads from the invasion front have greater endurance, but not speed, compared to conspecifics from a long-colonised area. Oecologia 162, 343–348. doi: 10.1007/s00442-009-1471-1
Long, J. A. (2019). interactions: Comprehensive, User-Friendly Toolkit for Probing Interactions. R Package Version 1.1.0. Available online at: https://cran.r-project.org/package=interactions (accessed December 16, 2022).
Lüdecke, D. (2018). ggeffects: tidy data frames of marginal effects from regression models. J. Open Source Softw. 3:772. doi: 10.21105/joss.00772
Molina-Martínez, A., León-Cortés, J. L., Regan, H. M., Lewis, O. T., Navarrete, D., Caballero, U., et al. (2016). Changes in butterfly distributions and species assemblages on a Neotropical mountain range in response to global warming and anthropogenic land use. Divers. Distrib. 22, 1085–1098. doi: 10.1111/ddi.12473
Moretti, E. H., Ortega Chinchilla, J. E., Marques, F. S., Fernandes, P. A. C. M., and Gomes, F. R. (2018). Behavioral fever decreases metabolic response to lipopolysaccharide in yellow Cururu toads (Rhinella icterica). Physiol. Behav. 191, 73–81. doi: 10.1016/j.physbeh.2018.04.008
Morris, S. (2004). HIF and anapyrexia; a case for crabs. Int. Congress Ser. 1275, 79–88. doi: 10.1016/j.ics.2004.08.056
Muir, A. P., Biek, R., Thomas, R., and Mable, B. K. (2014). Local adaptation with high gene flow: temperature parameters drive adaptation to altitude in the common frog (Rana temporaria). Mol. Ecol. 23, 561–574. doi: 10.1111/mec.12624
Nedrow, J., Scholnick, D., and Gleeson, T. (2001). Roles of lactate and catecholamines in the energetics of brief locomotion in an ectothermic vertebrate. J. Comp. Physiol. B 171, 237–245. doi: 10.1007/s003600000168
Niu, Z., Li, M., Pu, P., Wang, H., Zhang, T., Tang, X., et al. (2021). Effects of temperature on the locomotor performance and contraction properties of skeletal muscle from two Phrynocephalus lizards at high and low altitude. J. Comp. Physiol. B 191, 907–916. doi: 10.1007/s00360-021-01391-9
Parmesan, C., and Yohe, G. (2003). A globally coherent fingerprint of climate change impacts across natural systems. Nature 421, 37–42. doi: 10.1038/nature01286
Petersen, A. M., and Gleeson, T. T. (2009). Skeletal muscle substrate utilization is altered by acute and acclimatory temperature in the American bullfrog (Lithobates catesbeiana). J. Exp. Biol. 212, 2378–2385. doi: 10.1242/jeb.023408
Petersen, A. M., Gleeson, T. T., and Scholnick, D. A. (2003). The effect of oxygen and adenosine on lizard thermoregulation. Physiol. Biochem. Zool. 76, 339–347. doi: 10.1086/375429
Pinsky, M. L., Eikeset, A. M., McCauley, D. J., Payne, J. L., and Sunday, J. M. (2019). Greater vulnerability to warming of marine versus terrestrial ectotherms. Nature 569, 108–111. doi: 10.1038/s41586-019-1132-4
Qiu, Q., Zhang, G., Ma, T., Qian, W., Wang, J., Ye, Z., et al. (2012). The yak genome and adaptation to life at high altitude. Nat. Genet. 44, 946–949. doi: 10.1038/ng.2343
R Core Team (2020). R: A Language and Environment for Statistical Computing. Vienna: R Foundation for Statistical Computing.
Rebelo, A. D., and Measey, J. (2019). Locomotor performance constrained by morphology and habitat in a diverse clade of African frogs (Anura: Pyxicephalidae). Biol. J. Linn. Soc. 127, 310–323. doi: 10.1093/biolinnean/blz007
Romanovsky, A. A., Almeida, M. C., Aronoff, D. M., Ivanov, A. I., Konsman, J. P., Steiner, A. A., et al. (2005). Fever and hypothermia in systemic inflammation: recent discoveries and revisions. Front. Biosci. 10:2193–2216. doi: 10.2741/1690
Rozen-Rechels, D., Dupoué, A., Lourdais, O., Chamaillé-Jammes, S., Meylan, S., Clobert, J., et al. (2019). When water interacts with temperature: ecological and evolutionary implications of thermo-hydroregulation in terrestrial ectotherms. Ecol. Evol. 9, 10029–10043. doi: 10.1002/ece3.5440
Skandalis, D. A., Dobell, C. D., Shaw, J. C., and Tattersall, G. J. (2020). Hydrogen sulfide exposure reduces thermal set point in zebrafish. R. Soc. Open Sci. 7:200416. doi: 10.1098/rsos.200416
Souchet, J., Gangloff, E. J., Micheli, G., Bossu, C., Trochet, A., Bertrand, R., et al. (2020). High-elevation hypoxia impacts perinatal physiology and performance in a potential montane colonizer. Integr. Zool. 15, 544–557. doi: 10.1111/1749-4877.12468
Steiner, A. A., and Branco, L. G. S. (2002). Hypoxia-induced Anapyrexia: implications and putative mediators. Annu. Rev. Physiol. 64, 263–288. doi: 10.1146/annurev.physiol.64.081501.155856
Storz, J. F., and McClelland, G. B. (2017). Rewiring metabolism under oxygen deprivation. Science 356, 248–249. doi: 10.1126/science.aan1505
Storz, J. F., Scott, G. R., and Cheviron, Z. A. (2010). Phenotypic plasticity and genetic adaptation to high-altitude hypoxia in vertebrates. J. Exp. Biol. 213, 4125–4136. doi: 10.1242/jeb.048181
Sunday, J., Bennett, J. M., Calosi, P., Clusella-Trullas, S., Gravel, S., Hargreaves, A. L., et al. (2019). Thermal tolerance patterns across latitude and elevation. Philos. Trans. R. Soc. B 374:20190036. doi: 10.1098/rstb.2019.0036
Tattersall, G. J., and Boutilier, R. G. (1999). Does behavioural hypothermia promote post-exercise recovery in cold-submerged frogs? J. Exp. Biol. 202, 609–622. doi: 10.1242/jeb.202.5.609
Taylor, E. N., Diele-Viegas, L. M., Gangloff, E. J., Hall, J. M., Halpern, B., Massey, M. D., et al. (2021). The thermal ecology and physiology of reptiles and amphibians: a user’s guide. J. Exp. Zool. A Ecol. Integr. Physiol. 335, 13–44. doi: 10.1002/jez.2396
Trochet, A., Dupoué, A., Souchet, J., Bertrand, R., Deluen, M., Murarasu, S., et al. (2018). Variation of preferred body temperatures along an altitudinal gradient: a multi-species study. J. Therm. Biol. 77, 38–44. doi: 10.1016/j.jtherbio.2018.08.002
Vitt, L. J., and Caldwell, J. P. (2014). Herpetology: An Introductory Biology of Amphibians and Reptiles, 4th Edn. San Diego, CA: Academic Press.
Wagner, E. L., Scholnick, D. A., and Gleeson, T. T. (1999). The roles of acidosis and lactate in the behavioral hypothermia of exhausted lizards. J. Exp. Biol. 202, 325–331. doi: 10.1242/jeb.202.3.325
Wang, T., Abe, A. S., and Glass, M. L. (1998). Effects of temperature on lung and blood gases in the South American rattlesnake Crotalus durissus terrificus. Comp. Biochem. Physiol. A Mol. Integr. Physiol. 121, 7–11. doi: 10.1016/S1095-6433(98)10102-2
Withers, P. C. (1978). Acid-base regulation as a function of body temperature in ectothermic toads, a heliothermic lizard, and a heterothermic mammal. J. Therm. Biol. 3, 163–171. doi: 10.1016/0306-4565(78)90013-X
Wölfer, J., Aschenbach, T., Michel, J., and Nyakatura, J. A. (2021). Mechanics of arboreal locomotion in Swinhoe’s striped squirrels: a potential model for early Euarchontoglires. Front. Ecol. Evol. 9:636039. doi: 10.3389/fevo.2021.636039
Wood, S. C., and Gonzales, R. (1996). Hypothermia in hypoxic animals: mechanisms, mediators, and functional significance. Comp. Biochem. Physiol. B Biochem. Mol. Biol. 113, 37–43. doi: 10.1016/0305-0491(95)02045-4
Wood, S. C., and Malvin, G. M. (1991). Physiological significance of behavioral hypothermia in hypoxic toads (Bufo marinus). J. Exp. Biol. 159, 203–215. doi: 10.1242/jeb.159.1.203
Keywords: hypothermia, exhaustive exercise, high-altitude, common garden, Bufo gargarizans
Citation: Yao Z, Huang K and Qi Y (2022) Post-exercise Hypothermia Varies Between High- and Low-Altitude Populations in the Asiatic Toad (Bufo gargarizans). Front. Ecol. Evol. 10:846663. doi: 10.3389/fevo.2022.846663
Received: 31 December 2021; Accepted: 16 February 2022;
Published: 17 March 2022.
Edited by:
Bao-jun Sun, Institute of Zoology (CAS), ChinaCopyright © 2022 Yao, Huang and Qi. This is an open-access article distributed under the terms of the Creative Commons Attribution License (CC BY). The use, distribution or reproduction in other forums is permitted, provided the original author(s) and the copyright owner(s) are credited and that the original publication in this journal is cited, in accordance with accepted academic practice. No use, distribution or reproduction is permitted which does not comply with these terms.
*Correspondence: Zhongyi Yao, eWFvenlAY2liLmFjLmNu