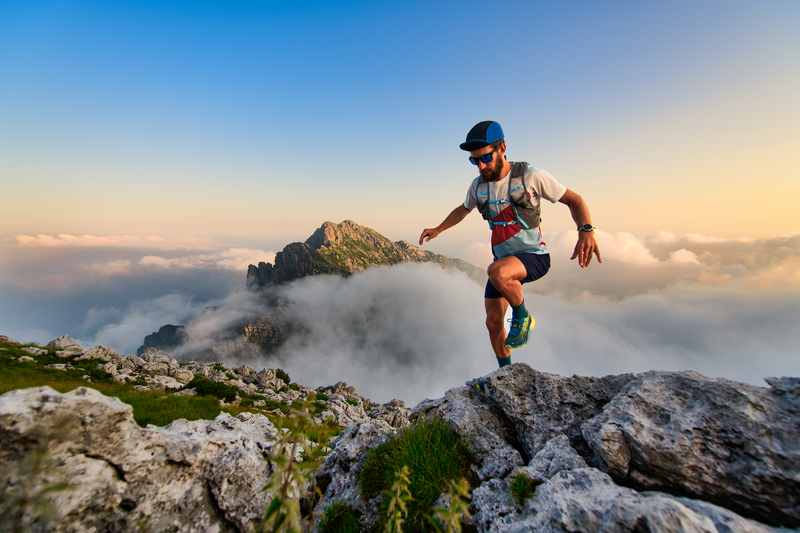
95% of researchers rate our articles as excellent or good
Learn more about the work of our research integrity team to safeguard the quality of each article we publish.
Find out more
ORIGINAL RESEARCH article
Front. Ecol. Evol. , 18 May 2022
Sec. Paleontology
Volume 10 - 2022 | https://doi.org/10.3389/fevo.2022.841784
This article is part of the Research Topic Tetrapod Water-Land Transition: Reconstructing Soft Tissue Anatomy and Function View all 14 articles
While most early limbed vertebrates possessed a fully-roofed dermatocranium in their temporal skull region, temporal fenestrae and excavations evolved independently at least twice in the earliest amniotes, with several different variations in shape and position of the openings. Yet, the specific drivers behind this evolution have been only barely understood. It has been mostly explained by adaptations of the feeding apparatus as a response to new functional demands in the terrestrial realm, including a rearrangement of the jaw musculature as well as changes in strain distribution. Temporal fenestrae have been retained in most extant amniotes but have also been lost again, notably in turtles. However, even turtles do not represent an optimal analog for the condition in the ancestral amniote, highlighting the necessity to examine Paleozoic fossil material. Here, we describe in detail the sutures in the dermatocranium of the Permian reptile Captorhinus aguti (Amniota, Captorhinidae) to illustrate bone integrity in an early non-fenestrated amniote skull. We reconstruct the jaw adductor musculature and discuss its relation to intracranial articulations and bone flexibility within the temporal region. Lastly, we examine whether the reconstructed cranial mechanics in C. aguti could be treated as a model for the ancestor of fenestrated amniotes. We show that C. aguti likely exhibited a reduced loading in the areas at the intersection of jugal, squamosal, and postorbital, as well as at the contact between parietal and postorbital. We argue that these “weak” areas are prone for the development of temporal openings and may be treated as the possible precursors for infratemporal and supratemporal fenestrae in early amniotes. These findings provide a good basis for future studies on other non-fenestrated taxa close to the amniote base, for example diadectomorphs or other non-diapsid reptiles.
Amniota, the clade comprising mammals, turtles, lepidosaurs, crocodylians, birds, and their extinct relatives, emerged no later than the early Pennsylvanian (ca. 319 Ma ago; Ford and Benson, 2020). In contrast to the majority of coeval limbed vertebrates, the earliest amniotes and their closest extinct relatives adopted a predominantly or even exclusively terrestrial lifestyle (Sumida, 1997; Nyakatura et al., 2019; Buchwitz et al., 2021). This ecological shift had been accomplished by innovations in their developmental strategies (e.g., Packard and Seymour, 1997; Werneburg, 2019; Blackburn and Stewart, 2021) and was accompanied by further changes in their general anatomy, involving the appendicular skeleton and vertebral column (Sumida, 1997), as well as the skull (Bramble and Wake, 1985; Iordansky, 1990). Especially, the adaptations in the skull are an essential aspect of amniote terrestrialization, as life outside of the aquatic realm makes specific demands on sensorial abilities, respiration, and feeding (Olson, 1961; Lauder and Gillis, 1997; Laurin, 2010).
One of the most recurrent differences in the skull morphology between early amniotes and most other Paleozoic limbed vertebrates are the reductions of the dermatocranium in the temporal region, leading to the formation of temporal fenestrae or marginal excavations (Werneburg, 2012, 2019; Abel and Werneburg, 2021). Such temporal openings evolved at least twice independently in early amniotes (Ford and Benson, 2020), corroborating the notion that they were a response to new functional demands (e.g., Case, 1924; Fox, 1964; Frazzetta, 1968; for a review see Abel and Werneburg, 2021), likely caused by a greater role of the external jaw adductor musculature and corresponding changes in force distribution (e.g., Versluys, 1919; Case, 1924; Lakjer, 1926; Frazzetta, 1968). Initially, this might have been also bound to amniote terrestrialization and accompanied adaptations like weight reduction, higher mobility of the atlanto-occipital joint, and change from a kinetic-inertial to a static-pressure biting system (Gaupp, 1895; Versluys, 1919; Olson, 1961; Fox, 1964; Kuhn-Schnyder, 1980). Overall, temporal openings have been hypothesized to form especially within “weak” regions of the dermatocranium that could easily have been reduced. Those include generally thin areas (e.g., Case, 1924; Romer and Price, 1940; Fox, 1964), intersections of more than two bones (Frazzetta, 1968; Kuhn-Schnyder, 1980), and bone articulations (Kemp, 1980).
Yet, the ancestral configuration without temporal openings (scutal sensu Abel and Werneburg, 2021) was retained in many Paleozoic amniotes (“Anapsida”; e.g., Carroll and Baird, 1972; Clark and Carroll, 1973), and also secondarily reevolved in groups like the Permian pareiasauromorphs (MacDougall and Reisz, 2014) and turtles (Gaffney, 1990; Jones et al., 2012). However, no ancestrally scutal amniote is known from post-Paleozoic strata and even extant turtles may not be a good analog due to their unique cranial adaptations (e.g., Kilias, 1957; Werneburg, 2011, 2012, 2013a,2015; Ferreira and Werneburg, 2019; Werneburg and Maier, 2019). Consequently, any hypothesis regarding the functional morphology in the ancestral scutal skull and its role in the evolution of temporal openings is dependent upon the assessment of fossil material.
Here, we use the skull of the Permian reptile (sensu Modesto and Anderson, 2004) Captorhinus aguti Cope, 1882 (Amniota, Captorhinidae) as a model for the cranial functional morphology in an early “anapsid.” We chose Captorhinus, because species of this genus represent some of the best documented early scutal reptiles with easily accessible articulated skull material and a wealth of literature on their cranial anatomy (e.g., Branson, 1911; Case, 1911; Sushkin, 1928; Price, 1935; Romer, 1956; Fox and Bowman, 1966; Heaton, 1979; Modesto, 1998; Kissel et al., 2002; Egberts, 2008), including discussions on their jaw musculature, cranial kinesis, and its relevance in understanding the origin of temporal fenestration (Warren, 1961; Fox, 1964; Fox and Bowman, 1966; Bolt, 1974; Heaton, 1979; Jones and Zikmund, 2012; Werneburg and Abel, 2022).
It is worth mentioning that C. aguti postdated the oldest known amniotes by ca. 30 Ma (Woodhead et al., 2010; Ford and Benson, 2020) and already exhibited some adaptations that might be considered derived relative to earlier scutal reptiles, especially in its dentition, feeding mechanics, and skull proportions (Heaton, 1979; Dodick and Modesto, 1995; Hotton et al., 1997; Modesto et al., 2007). Nevertheless, C. aguti appears to be still rather generalized in its overall cranial morphology in relation to some other contemporary taxa with a scutal temporal region (e.g., Labidosaurus Cope, 1895, moradisaurine captorhinids, and probably Mesosaurus Gervais, 1865; Dodick and Modesto, 1995; Modesto, 2006; Modesto et al., 2007) and its temporal morphology is overall similar to other early scutal reptiles (Carroll and Baird, 1972; Clark and Carroll, 1973; Heaton, 1979), among them the oldest known unambiguous amniotes (e.g., Hylonomus lyelli Dawson, 1860; Paleothyris acadiana Carroll, 1969b). Notable differences in its temporal region, especially to non-captorhinid taxa, are the distinctly reduced supratemporals and absent tabulars (Fox and Bowman, 1966; Heaton, 1979; compare to Koyabu et al., 2012).
We provide a detailed description of the suture morphology within the dermatocranium of C. aguti and its adjacent contacts with the viscero- and neurocranium to better understand the general stability and integrity of the hypothetical ancestral amniote skull. We identified possible “weak” regions in the dermatocranium and discuss their implications for strain distribution. Using a conservative reconstruction of the jaw adductor musculature, we further discuss the possibilities of cranial kinesis and elastic bone movements in the temporal region of C. aguti, and how our interpretation compares to previous studies involving this taxon. Lastly, we outline how the cranial mechanics in the ancestral amniote probably differed from other Paleozoic limbed vertebrates and how they might have provided preconditions for the evolution of temporal openings.
For this study, we used an almost complete, three-dimensionally preserved skull of Captorhinus aguti, housed at the Sam Noble Oklahoma Museum of Natural History [OMNH 44816; Figure 1; see also Werneburg and Abel (2022)]. As documented by the OMNH, the specimen derives from an unspecified Cisuralian fissure filling in Comanche County, Oklahoma. It is likely that this refers to the well-documented Richards Spur speleothem, which is Artinskian in age (ca. 289 Ma; Woodhead et al., 2010). OMNH 44816 is missing bones especially in the left half of the skull, namely the left nasal and jugal. The left prefrontal and squamosal, the parabasisphenoid and supraoccipital, as well as the left dentary, angular, and surangular are incomplete. The right septomaxilla is probably present but metallic inclusions precluded a proper segmentation. Completely missing in the skull are the premaxillae, prootics, basioccipital, and exoccipitals. Some bones are broken, but nevertheless completely preserved, these are most notably the right palatine, left postorbital, right maxilla and jugal, and both parietals.
Figure 1. 3D model generated from the scan of OMNH 44816 in left lateral (A), right lateral (B), dorsal (C), ventral (D), anterior (E), and posterior view (F). Abbreviations: an, angular; ar, articular; co, coronoid; de, dentary; ep, epipterygoid; f, frontal; ju, jugal; la, lacrimal; mx, maxilla; na, nasal; op, opisthotic; pa, parietal; pal, palatine; pb, postorbital; pf, postfrontal; pp, postparietal; pra, prearticular; prf, prefrontal; pbs, parabasisphenoid; pt, pterygoid; q, quadrate; qj, quadratojugal; sep, septomaxilla; spl, splenial; sq, squamosal; stp, stapes; sup, supraoccipital; sur, surangular; vo, vomer.
OMNH 44816 was scanned by Matthew Colbert with an NSI scanner at the University of Texas High-Resolution X-ray Computed Tomography Facility (UTCT), Austin, United States, in February 2017. The scanner is powered by a Fein Focus High Power source with 180 kV and 0.15 mA. It uses an aluminum filter and a Perkin Elmer detector. The scan has a voxel size of 33.5 μm and consists of 1897 single slices. Further corrections were performed by Jessica Maisano. MicroCT images are deposited in MorphoSource (morphosource.org/concern/media/000439915).
Each bone was virtually extracted using manual segmentation in Avizo 8.13D renderings of the external surface of the bones and the teeth were generated and converted into meshes. Meshes were saved as PLY-files and were projected as 3D models in MorphoDig 1.5 (Lebrun, 2018; Figures 1–9). Renderings are available in Abel et al. (in press).
Figure 2. Simplified cross section of a skull suture, illustrating the terminology used in this manuscript.
Figure 3. Isolated bones in dorsal, ventral, lateral, medial, anterior and posterior views. Articulation areas with other bones are colored. Left and right angular, left and right articular, left and right coronoid, and left and right dentary are shown. Scale bar = 10 mm. For abbreviations see caption to Figure 1.
Figure 4. Isolated bones in dorsal, ventral, lateral, medial, anterior and posterior views. Articulation areas with other bones are colored. Left and right splenial, left and right surangular, left and right maxilla, and left and right vomer are shown. Scale bar = 10 mm. For abbreviations see caption to Figure 1.
Figure 5. Isolated bones in dorsal, ventral, lateral, medial, anterior and posterior views. Articulation areas with other bones are colored. Left and right prearticular, left and right lacrimal, and right nasal are shown. Scale bar = 10 mm. For abbreviations see caption to Figure 1.
Figure 6. Isolated bones in dorsal, ventral, lateral, medial, anterior and posterior views. Articulation areas with other bones are colored. Left and right epipterygoid, left septomaxilla, left and right frontal, and left and right prefrontal are shown. Scale bar = 10 mm. For abbreviations see caption to Figure 1.
Figure 7. Isolated bones in dorsal, ventral, lateral, medial, anterior and posterior views. Articulation areas with other bones are colored. Left and right pterygoid, left and right palatine, right jugal, and left and right quadratojugal are shown. Scale bar = 10 mm. For abbreviations see caption to Figure 1.
Figure 8. Isolated bones in dorsal, ventral, lateral, medial, anterior and posterior views. Articulation areas with other bones are colored. Left and right postorbital, left and right postfrontal, left and right parietal, and left and right postparietal are shown. Scale bar = 10 mm. For abbreviations see caption to Figure 1.
Figure 9. Isolated bones in dorsal, ventral, lateral, medial, anterior and posterior views. Articulation areas with other bones are colored. Left and right squamosal, Left and right quadrate, Left and right stapes, Left and right opisthotic, parabasisphenoid, and supraoccipital are shown. Scale bar = 10 mm. For abbreviations see caption to Figure 1.
The thickness of the sutures of the dermatocranium was measured using the 2D Length Tool in Avizo 8.1. The measurements were taken in the orientation in which the suture was most complete and visible. Not being homogeneous over the entire suture length, the suture thickness was measured in five different spots. These spots are quite regularly distributed along the whole suture to obtain an accurate and well-representative mean. All measurements and means of the suture thickness are listed in Supplementary Table 2.
For this paper, the term “suture” is generally applied for the contact of two bones, including their non-preserved soft-tissue components. The bone areas that articulate with each other are referred to as “articulation facets,” the externally visible area incorporating the suture is the “external surface” (Figure 2).
We identified and defined eight different suture types in the dermatocranium of Captorhinus aguti (Figure 10). Most used suture type terminology follows Jones et al. (2011). The type “stepped interdigitation” (8 in Figure 10) is a combination of “stepped joint” (7 in Figure 10) and “Type-B interdigitation” (5 in Figure 10) sensu Jones et al. (2011). Sutures can vary in the presence and type of interdigitations as well as in the presence of a bony lamina that extends from one of the suturing bones medially to its respective contralateral neighbor, forming an additional medial contact. Like observed for other taxa (e.g., Clack, 2002; Rayfield, 2005), sutures also can vary in suture type and thickness along the contact of two bones. In some cases, the type of suturing could not be directly observed, because the relevant bones were missing in OMNH 44816 or had been disarticulated. Articulations between dermal bones and those of the neuro- and viscerocranium are briefly described but not categorized after the scheme in Figure 10, because they fall out of our research question and have a very different, enchondral type of ossification (Koyabu et al., 2014) and related suture formation. Likewise, contacts between such non-dermal bones were also not described.
Figure 10. Suture types observed in the dermatocranium of OMNH 44816 and their position in the skull. 1, butt joint; 2, tongue-and-groove joint; 3, slot joint; 4, Type-A interdigitation; 5, Type-B interdigitation; 6, Type-C interdigitation; 7, stepped joint; 8, stepped interdigitation. Terminology mostly after Jones et al. (2011).
The preorbital region of C. aguti consists of the premaxilla, septomaxilla, maxilla, nasal, lacrimal, prefrontal, as well as of the anterior portions of the frontal and jugal. Only some preorbital sutures exhibit a simple structure. Interdigitating contacts appear especially close to the orbits.
The maxilla (Figure 4) forms laterally a facial lamina that fits into a ventral notch of the lacrimal (“tongue-and-groove joint”; 2 in Figure 10). The posterior half of the maxilla is overlapped by the jugal by a “stepped joint” (7 in Figure 10). The palatine contacts the maxilla laterally by what may be referred to as a low “slot joint” (3 in Figure 10). Anteriorly, the maxilla would have overlapped the non-preserved premaxilla (Fox and Bowman, 1966; Heaton, 1979).
The ventral margin of the lacrimal (Figure 5) is concave to house the facial lamina of the maxilla. Overall, the ventral margin forms a medially expanded rim that forms the external surface with the maxilla. On its dorsal margin, it underlies the nasal, the bones interdigitate externally (“stepped interdigitation”; 8 in Figure 10). Posteriorly to the lacrimal-nasal contact, the lacrimal exhibits a similar contact with the prefrontal, the latter underlies the lacrimal. Posteroventrally, it sutures with the jugal by a stepped joint. At its anterior end, the lacrimal is slightly overlapping the septomaxilla laterally. The lacrimal section of the orbital rim medially overlaps a dorsal ridge of the palatine, forming a slot joint.
Excluding its lateral contact with the prefrontal, the nasal (Figure 5) interdigitates with all its preserved neighboring bones. Its anterior tip would have underlain the premaxilla. Posterior to the nasal-lacrimal contact, the nasal forms a “butt joint” with the prefrontal (1 in Figure 10). The remainder of the nasal-prefrontal contact can be described as a stepped interdigitation. A stepped interdigitation can be also observed at the posterior end of the nasal for its contact with the frontal, where the frontal underlies the nasal.
Apart from its already described sutures with the lacrimal and nasal, the prefrontal (Figure 6) medially contacts the frontal by a stepped joint posterior to the prefrontal-nasal contact.
The posterior suture of the frontal (Figure 6) with the parietal is overall similar to the frontal-nasal contact. Posterolaterally, the frontal forms a “Type-A interdigitation” (4 in Figure 10) with the postfrontal. Additionally, a complex inter-frontal suture is present (“Type-C interdigitation”; 6 in Figure 10). Only the posterior end of the frontal that contacts the postfrontal and parietal contributes to the temporal region.
The temporal part of the dermatocranium in C. aguti consists of the posterior portion of the jugal and frontal, as well as the postfrontal, postorbital, squamosal, quadratojugal, parietal, postparietal, and supratemporal. Interdigitating contacts are especially formed on the external surfaces in the “cheeks” (i.e., jugal, squamosal, postorbital, and quadratojugal).
The posterior jugal (Figure 7) dorsally underlies the postorbital, forming a stepped joint (7 in Figure 10). At its posterior end, the jugal also underlies the squamosal; both bones form a stepped interdigitation (8 in Figure 10). The jugal is similarly contacted by the quadratojugal at its posteroventral edge, although the interdigitation sequence is short. A medial contact with the pterygoid has been reported for Captorhinus and other captorhinids (Fox and Bowman, 1966; Heaton, 1979; Dodick and Modesto, 1995; Modesto et al., 2007). However, such a contact is not preserved in OMNH 44816. This is likely due to the loss of the anteromedial process that would have connected the jugal to the pterygoid and a slight displacement of the palate.
The postorbital (Figure 8) anterodorsally underlies the postfrontal (stepped joint). Dorsally, the postorbital is simply contacted by the parietal (butt joint; 1 in Figure 10). Posteriorly, the postorbital is underlain by the squamosal, and like between the squamosal and the jugal, both contacts interdigitate externally (stepped interdigitation).
Next to its sutures with the postorbital and frontal, the postfrontal (Figure 8) likewise contacts the parietal posteriorly by a stepped interdigitation.
The squamosal (Figure 9) underlies the parietal dorsally, forming together another stepped joint. Ventrally, the squamosal itself is underlain by the quadratojugal in a similar manner. Posterodorsally, it would have also sutured to the supratemporal; however, we were not able to reconstruct the latter for OMNH 44816. Similarly, both postparietals are too badly preserved to make any judgment about their contact to the squamosal. Medially, the squamosal is contacted by the quadrate. At the medial margin of its occipital flange, the squamosal forms a short contact with the opisthotic. We are not able to unambiguously confirm a contact with the supraoccipital.
In addition to the contacts with the squamosal and jugal, the quadratojugal (Figure 7) is underlain posteriorly by the quadrate.
Other sutures with the parietal (Figure 8) include the contact with the postparietal, which it overlaps posteriorly (stepped joint). Posterolaterally, it would have contacted the supratemporal. Both parietals suture each other medially by a butt joint. The supraoccipital would have sutured with the parietals posteroventrally.
The left and right postparietal (Figure 8) are sutured to each other medially by a thin Type-C interdigitation (6 in Figure 10). Ventrally, they overlap the supraoccipital.
The dermal palate consists of three bones: the vomer, palatine, and pterygoid. All three bones are rigidly connected by interdigitating sutures.
Both vomers (Figure 4) contact each other medially at their anterior end by a butt joint (1 in Figure 10). Posteriorly, the vomer interdigitates with the palatine by a Type-C interdigitation (6 in Figure 10). A shorter interdigitation with the pterygoid can be observed at its posteromedial edge (“Type-B interdigitation”; 5 in Figure 10). Additionally, the rod-shaped anterior extension of the pterygoid is on much of its lateral margin contacted with the vomer by a butt joint. Anteriorly, the vomer would have contacted the premaxilla.
The palatine (Figure 7) interdigitates further with the pterygoid at its posterior and medial margins (Type-C interdigitation). Anteromedially, the palatine-pterygoid contact develops into simple a butt joint. As described above for the preorbital region, the palatine is laterally contacts the maxilla and lacrimal.
At their anterior extremity, both pterygoids (Figure 7) contact each other medially by a short butt joint. In the anteromedial section of the quadrate process, the pterygoid is overlapped by the epipterygoid. The pterygoid does not contact the parabasisphenoid. Posterolaterally, the quadrate broadly contacts the quadrate process of the pterygoid. Posteromedially, the same process is abutted by the stapes.
The bones of the mandible comprise the dentary, angular, surangular, coronoid, splenial, prearticular, and articular. The mandibular symphysis is restricted to the anterior tip of the mandible. In most cases, the bones of the lower jaw have simple contacts with each other. Interdigitating sutures appear only on the external surface.
Both dentaries (Figure 3) are sutured to each other on their anteromedial end by a butt joint (1 in Figure 10). On its external surface, the dentary interdigitates posteriorly with the angular by a Type-B interdigitation (5 in Figure 10). Medially, the two bones contact each other along the posterior half of the dentary by what may be referred to as a butt joint. A similar arrangement exists between dentary and surangular; the latter borders the dentary posterodorsally. Medially, the splenial also contacts the dentary both dorsally and ventrally to the Meckelian groove. The dorsal contact is similar to the medial contacts with the angular and surangular described above, however, ventrally, the splenial slightly underlies the dentary by a stepped joint (7 in Figure 10). Posterior to the dorsal dentary-splenial suture, the dentary contacts the anterior end of the prearticular by another butt joint. The medial portion of the dentary, dorsally to the other mandibular bones, is mostly covered by the coronoid.
Next to its already described contact with the dentary, the midsection of the coronoid (Figure 3) overlaps the anterior prearticular by a butt joint. Anteriorly, the coronoid tapers between the dentary and splenial. Posteriorly, a large wedge of the surangular protrudes to the coronoid, forming a short interdigitating sequence.
At their anterior ends, both splenials (Figure 4) contact each other medially by a butt joint as part of the mandibular symphysis. Posterodorsally, the prearticular tapers in-between the dentary-splenial contact. Posterolaterally, the angular contacts the splenial by a butt joint; posteroventrally, it also overlies the splenial by a stepped joint.
Like described above, the surangular interdigitates anteriorly with the dentary and coronoid by a Type-B interdigitation. On its external surface, it is ventrally overlapped by the angular by a stepped joint. On their internal surface, both bones exhibit a Type-B interdigitation. The prearticular simply sutures the surangular by a butt joint at its posteroventral extremity. Posteromedially, the surangular is wedged into the articular.
Apart from its sutures mentioned above, the angular (Figure 3) is dorsally overlain by the prearticular medially to the Meckelian groove. The latter widens medially at its posterior end to surround the ventral portion of the articular.
The thickness of bones at their sutures varies greatly in the dermatocranium of OMNH 44816 (Supplementary Table 2 and Figure 11). Overall, the thinnest sutural areas in the skull are the anterior palate (0.52–0.79 mm), the intersection of jugal, squamosal, and postorbital (0.46–1.33 mm), the inter-parietal contact (0.36–1.34 mm) as well as the dorsolateral rostrum (0.78–1.12 mm). The thickest areas are located close to the maxilla (max. 2.93 mm), in the anterior “cheek” (max. 3.56 mm), at the lacrimal-palatine contact (max. 3.55 mm), and between both pterygoids (max. 2.32 mm). Noteworthy trends include a thinning of the jugal-squamosal, jugal-postorbital, and squamosal-postorbital sutures toward the jugal-squamosal-postorbital intersection, as well as a thinning in anterior direction along the sutures between parietal and “cheek.”
Figure 11. Points of measurements for suture thickness in OMNH 44816 as indicated in Supplementary Table 2.
Sutures connect neighboring skull bones by differently arranged viscoelastic fibers (Herring, 2008). In addition to providing sites for bone growth, the main function of sutures is likely the absorption and transmission of strain (e.g., Herring et al., 1996; Herring and Teng, 2000; Herring, 2008; Moazen et al., 2009; Curtis et al., 2013; even though some theoretical approaches showed only little effect of sutures on strain distribution: see Ferreira et al., 2020). Moreover, soft- and hard-tissue suture morphology is dependent on the presence and, probably, also on the type of strain (e.g., Moss, 1957; Herring, 1972, 2008; Herring and Mucci, 1991). However, osteological evidence alone is not suitable to unambiguously determine the main type of strain affecting a suture (Herring and Mucci, 1991; Rayfield, 2005). Overall, suture morphology may be used to roughly infer the distribution of strain within the skull and jaw adductor action even when in vivo observations are not possible (Herring, 1972).
The eight identified suture types can be broadly subdivided based on their complexity and, hence, their assumed robustness (Herring, 2008). Simple butt joints (1 in Figure 10) like they occur especially in the anterior palate, but also at the prefrontal-nasal and postorbital-parietal contacts, as well as between the parietals, could be interpreted as regions little affected by mechanical stimuli (Moss, 1957). Butt joints have been associated with both compressional and tensile strain (Herring and Mucci, 1991; Rayfield, 2005; Porro et al., 2015), making a more detailed interpretation for OMNH 44816 difficult. Compared to other suture morphotypes, butt joints might be least resistant toward stress and strain due to less available attachment area for the fibers (Jones et al., 2011). The slot and tongue-and-groove joints (2 and 3 in Figure 10) only occur in the preorbital region and adjacent palatal contacts. Tongue-and-groove joints had been previously interpreted as an adaptation toward tensile strains (Herring and Mucci, 1991; Porro et al., 2015; Rawson et al., 2021). Based on their horizontally intercalated geometry, we would tentatively argue that slot joints are more resistant to compressive than to tensile strains. The majority of the dermal bones in C. aguti are connected by stepped joints or stepped interdigitations [7 and 8 in Figure 10; see also Jones and Zikmund (2012)]. Other types of interdigitations (4, 5, and 6 in Figure 10) are restricted to the frontal and palatal region. The role of stepped joints appears to be more complex (Rayfield, 2005; Porro et al., 2015; Rawson et al., 2021). They might act as a response to shear (Bolt, 1974), torsion (Busbey, 1995; Clack, 2002), or to a combination of tension and compression (Gans, 1960; Markey et al., 2006). Robustness of stepped joints might have been higher when the respective bones were additionally connected by external interdigitations (8 in Figure 3). Generally, interdigitations have been shown to be an adaptation to compression and overall high strains (e.g., Herring, 1972; Rafferty and Herring, 1999; Rayfield, 2005; Markey et al., 2006).
Additionally, suture robustness may be also controlled by interdigitation type. Bones that are only interdigitated at their articulation facet (5 in Figure 10) may be less resistant to forces acting longitudinally to the suture than bones that are additionally interdigitated at their external surfaces (6 in Figure 10). Comparably, a lack of interdigitations at the articulation facet may also reduce the resistance to vertical forces (4 in Figure 10). Overall, a higher degree of interdigitation leads to more available fiber attachment area and thus to a higher resistance toward strain than simpler suture morphotypes (Herring, 1972). Also considering thickness measurements, potentially weak areas might have been at the jugal-squamosal-postorbital intersection, at the parietal-postorbital suture, between both parietals, at the dorsolateral rostrum, and in the anterior palate.
These assumptions also allow direct comparisons with the suture morphology described for several Paleozoic non-amniotes (e.g., Klembara, 1994; Kathe, 1995, 1999; Berman et al., 2010; Porro et al., 2015; Gruntmejer et al., 2019; Rawson et al., 2021), especially when focusing on the temporal region. Interdigitations can be seen in a wide array of taxa, and often developed for more sutures than found in the skull of OMNH 44816 (Porro et al., 2015; Gruntmejer et al., 2019; Rawson et al., 2021); however, there are also numerous examples with less pronounced or absent interdigitations in this part of the skull. In many cases, morphotypes corresponding to the herein used butt and stepped joints prevail (Klembara, 1994; Kathe, 1995), arguing that compressional strain on the temporal region was comparatively low. This highlights that strain distribution in the temporal region could differ markedly among Paleozoic limbed vertebrates. Considering that the formation of temporal openings is due to specific distributions of strain within the temporal region (Abel and Werneburg, 2021), this might explain why temporal openings occur mainly in amniotes and only in few other clades in which these preconditions are met (see section “Cranial Mechanics and the Evolution of Temporal Openings”).
The main source of strain within the temporal region is likely related to the action of the jaw musculature (Jones et al., 2011). Indeed, it has been demonstrated that attachment of the jaw adductors is directly related to suture morphology (Herring and Mucci, 1991; Herring and Teng, 2000). More precisely, forces that are applied onto the cranium by muscle action cause the bony coverage to respond by a strengthening of the affected bones and sutures (e.g., Case, 1924; Herring and Mucci, 1991; Rayfield, 2005; Jones et al., 2011); with stronger sutures inevitably reducing the intracranial mobility in the respective skull areas (Clack, 2002). Such forces are expected to be applied especially by direct or indirect action of the jaw adductors (Herring and Mucci, 1991; Herring et al., 1996; Rafferty et al., 2000); we, hence, focus on this set of muscles herein. We appreciate, however, that also neck muscles, related to the posture and movement of the head and being relevant for feeding biology when pulling food items, play a role for the biomechanics of the temporal region (sensu Werneburg, 2015; Werneburg et al., 2015). As no radical differences in neck mobility are expected among most early amniotes, we consider that as a stable condition among clades and do not discuss neck musculature any further.
Direct evidence for muscle is usually not preserved in the fossil record. While specific morphological features of bones like processes, ridges, or scars can be used to deduce former muscle attachment sites (e.g., Fox, 1964; Heaton, 1979; Witzmann and Werneburg, 2017), there are also some limitations to a purely osteological approach as muscles may also attach to cartilage or other soft tissue like skin and usually do not leave any trace on the bones (e.g., Romer, 1927; Schumacher, 1973; Werneburg, 2011; Wilken et al., 2019). The development of osteological correlates may be dependent on the type of attaching tissue [fleshy or tendinous; Bryant and Seymour (1990) and references therein; Werneburg, 2011, 2013a,2013b] and may vary within a species or even between both sides of the skull (Poglayen-Neuwall, 1953; Witzmann and Werneburg, 2017). Such uncertainties could be met by taking the known myology of comparable taxa into account.
Comparative anatomical studies show that in tetrapods the jaw adductors generally can be subdivided into an external, internal, and posterior compartment based on their respective position relative to the divisions of the trigeminal nerve (e.g., Luther, 1914; Barghusen, 1973; Holliday and Witmer, 2007; Diogo et al., 2008; Witzmann and Werneburg, 2017; Ferreira and Werneburg, 2019). Within reptiles, the external jaw adductors [“adductor mandibulae externus” (AME)] usually originate from the dermal bones of the skull roof and “cheek,” and sometimes also from the quadrate or neurocranium. The internal adductors [“adductor mandibulae internus” (AMI)] originate mostly from the palate and the lateral braincase wall. The posterior jaw adductor [“adductor mandibulae posterior” (AMP)] arises from the quadrate. All jaw adductors insert onto the posterior portion of the mandible (Holliday and Witmer, 2007; Diogo and Abdala, 2010; Werneburg, 2011, 2013a; Ziermann et al., 2018; Ferreira and Werneburg, 2019).
In reptiles, the external adductor (AME) can be usually further subdivided into a lateral [“adductor mandibulae externus superficialis” (AMES)], a deep [“adductor mandibulae externus profundus” (AMEP)], and a medial muscle portion [“adductor mandibulae externus medialis” (AMEM)]; the internal adductors can be subdivided into the pterygoideus (PT), pseudotemporalis (PST), and constrictor internus dorsalis (CID) muscles (Holliday and Witmer, 2007; Werneburg, 2011; Ferreira and Werneburg, 2019; and references in these works). However, variation to this pattern can be observed widely across various taxa (Holliday and Witmer, 2007; Daza et al., 2011; Werneburg, 2013a). Nevertheless, assuming these subdivisions to represent the plesiomorphic condition of extant reptiles, it may be inferred that it also represented the condition in an early-diverging taxon like C. aguti. The condition found in mammals is highly derived with a masseter muscle first evolving in Cynodontia (Barghusen, 1973; Abdala and Damiani, 2004; Werneburg, 2013b). Homologizations of reptilian to lissamphibian muscles are possible (Diogo and Abdala, 2010). Therefore, we consider the ancestral reptilian condition as also ancestral to Amniota as a whole.
Most extant amniotes possess temporal fenestrae, and at least the external jaw adductors are usually associated with the surrounding temporal bars (e.g., Holliday and Witmer, 2007; Jones et al., 2009; Werneburg, 2019). Consequently, the muscle attachment sites for an extinct taxon with a scutal temporal region like C. aguti may not be fully predictable based on these taxa. Considering the shared trait of a skull without temporal fenestrae, turtles instead might be an intuitively fitting extant analog for C. aguti. Whereas the majority of extant turtles also possess a distinctly reduced dermal armor in the form of a highly emarginated temporal region (Gaffney, 1979; Jones et al., 2012; Werneburg, 2012; Abel and Werneburg, 2021), some groups like sea turtles as well as the earliest known Testudinata exhibit, like C. aguti, a scutal morphology without distinct temporal openings (Gaffney, 1990; Jones et al., 2012). However, most current phylogenetic placements of turtles (e.g., Rieppel and deBraga, 1996; deBraga and Rieppel, 1997; Crawford et al., 2012, 2015; Lyson et al., 2012; Field et al., 2014; Schoch and Sues, 2015; Irisarri et al., 2017; Li et al., 2018; Gemmell et al., 2020; but see also Lichtig and Lucas, 2021) indicate that they are deeply nested within Diapsida and the scutal morphology in early Testudinata likely derived from a fenestrated ancestor due to selective pressures specific to the turtle skull (Zdansky, 1923–1925; Kilias, 1957; Werneburg, 2015), which also involved a comprehensive rearrangement of soft tissue (Werneburg, 2013a,b) and the suturing of the quadrate to the braincase (Werneburg and Maier, 2019). Additionally, the scutal sea turtle skull likely represents a secondary evolution within Testudines and its jaw muscle arrangement is probably different from the one in early Testudinata (Jones et al., 2012; Werneburg, 2013a; Ferreira and Werneburg, 2019; Werneburg et al., 2019). Hence, while extant turtles can help to predict how the jaw adductors would attach in a scutal skull in general, their derived morphology would limit their applicability for the reconstruction of the jaw musculature in an ancestral “anapsid.”
Yet, comparisons with taxa outside of Reptilia might be even less adequate. As mentioned above, mammalian jaw adductors are distinctly derived from the assumed condition in their early synapsid ancestors and cannot be directly homologized with the reptilian condition (Barghusen, 1973; Diogo et al., 2008). Likewise, the specifics of lissamphibian anatomy are largely influenced by metamorphotic developmental events (Haas, 2001, 2003; Iordansky, 2010; Kleinteich and Haas, 2011; Ziermann, 2019), and the nearest extant non-tetrapod relatives, Dipnoi, are argumentatively too distantly related to reptiles to provide a good bracketing taxon (but see Werneburg, 2019). Taking all into account, the jaw adductors of C. aguti may be best inferred from the assumed myology in the last common ancestor of all extant reptiles in the context of a scutal temporal region with respect to the osteological peculiarities of C. aguti.
Reconstructions and suggestions regarding the jaw musculature of C. aguti and other captorhinids have been already provided by previous authors (Adams, 1919; Fox, 1964; Fox and Bowman, 1966; Heaton, 1979; Dodick and Modesto, 1995). Fox (1964) subdivided the external adductors of Captorhinus [“capitimandibularis” in Fox (1964)] into two main sections, the lateral “masseter” (i.e., AMES) and the medial “temporal” (i.e., AMEP) section. Additionally, he discussed also the presence of a third section between the AMES and AMEP, which would likely correspond to the AMEM of diapsids. Fox (1964) argued that the AMEP would have been the largest section and attached to the parietal, postfrontal, postorbital, and squamosal. The AMES would have been sheet-like and originated from the jugal, quadratojugal, and squamosal from where it inserted onto the coronoid process (Fox, 1964; Fox and Bowman, 1966). If the AMEM was present, Fox (1964) argued it would have been sheet-like and extend from the skull roof onto a bony “knob” anterodorsally to the Meckelian fossa, as observed for extant tuatara [however, this is likely more complicated, see Jones et al. (2009), given also the fact that tuatara has a diapsid skull morphotype with specifically derived muscle arrangements]. In regard of the internal jaw adductors [“pterygoideus” in Fox (1964)], he suggested a subdivision into an anterior (i.e., PT) and a posterior section (i.e., AMP). Fox (1964) let the PT occupy large portions of the dorsal pterygoid surface as well as the lateral surface of the pterygoid flange. He argued it would have extended in a posteroventral direction, medially to the AMEP, and inserted medially inside the Meckelian fossa. The AMP would have been of limited size and attached to the quadrate from where it would have inserted onto the mandible between the jaw joint and Meckelian fossa. Lastly, Fox (1964) also discussed the presence of a pseudotemporalis muscle (i.e., PST), but he argued that if it would have been present, the relative position of the pterygoid would prohibit an arrangement as observed for extant taxa.
Fox (1964) and Fox and Bowman (1966) partly provided osteological arguments for their suggested muscle arrangements. However, in some cases they also argued for particular muscle attachment sites without describing a clear reasoning, or despite the lack of osteological evidence like muscle scars. Nevertheless, the latter is per se legitimated as discussed above as the authors indeed highlighted the impact of different attaching tissues on the presence of osteological correlates.
Fox and Bowman (1966) described distinct striae on the medial portion of the parietals close to the suture between these bones, which would likely indicate a tendinous attachment of the AMEP there [however, see Heaton (1979) below for a different interpretation]. Likewise, they interpreted distinct concavities occupying most of the medial parietal surface as likely fleshy attachment sites of the AMEP, because no structures were visible on the internal bone surface. A bony ridge which ran close to the squamosal-parietal suture probably separated the AMEP from the AMES (Fox, 1964; Fox and Bowman, 1966). Apparently, Fox (1964) also assumed the attachment of the AMES onto the jugal and quadratojugal to be fleshy as he highlighted the smooth medial surfaces of these bones. In the mandible, the AMES would insert onto the surangular portion of the coronoid process at a vertical flange of the surangular that bore two concavities (Fox, 1964; Fox and Bowman, 1966).
Fox (1964) further argued for a tendinous attachment of the PT onto the lateral pterygoid flange as evident by its size, which he also compared to the condition in crocodiles. According to him, too, the attachment to the dorsal pterygoid surface was likely fleshy. The PT could have inserted onto the ventral and medial surfaces of the angular. A medial groove on the prearticular, bearing pits and striae, might have been another insertion site of the PT (Fox and Bowman, 1966). These latter authors suggested that the AMP could have inserted onto a medial ridge formed by the articular and prearticular and a small concavity dorsal to that ridge as indicated by probable muscle scars on both structures. Lastly, based on comparison with tuatara, Fox (1964) suggested that an AMEM with uncertain origin on the skull roof (see above) might have inserted onto a “knob” anterior to the Meckelian fossa.
Heaton (1979) discussed the cranial musculature in the related species Captorhinus laticeps [“Eocaptorhinus” in Heaton (1979)] and other material referred to the genus. In fact, some of the material described by Heaton (1979) and used for his illustrations might be actually referable to C. aguti, namely the specimens found at the Dolese Brothers Quarry (Robert Reisz, personal communication, 2021).
Heaton (1979) disagreed with Fox and Bowman (1966) on the interpretation of the medial striae in the parietals of Captorhinus being remnants of a tendinous attachment of the jaw adductors. Instead, he argued they were due to attachment of meninx and taenia marginalis. The bony ridge that runs parallel to the parietosquamosal suture identified by Fox (1964) and Fox and Bowman (1966) as the border between AMES and AMEP was recognized by Heaton (1979) as an attachment site solely for the AMES. Other sections of the AMES might have attached to the mediodorsal portion of the squamosal. Also, the ventral concavities of the parietals already reported by Fox and Bowman (1966) as likely attachment sites for the AMEP are, according to Heaton (1979), muscle scars from the AMEM. He argued that the AMEM was further divided into an “adductor mandibulae externus 2 c (pars media C)” and “adductor mandibulae externus 2 a (pars media A)” muscle head (sensu Lakjer, 1926). The two muscle heads would have been subdivided by the temporal artery, evident by a distinct foramen in the proximity of the mentioned parietal concavity. The “adductor mandibulae externus 2 b (pars media B)” is said to have been attached anteriorly to the concavity, where it left an additional number of muscle scars. A more posterior section of the AMEM would have attached to the quadrate, together with the AMP. Heaton (1979) considered the AMEP to be present; however, he only stated that it would have inserted ventrally to the coronoid like in Fox (1964). For the AMES and AMEM, Heaton (1979) argued that they would have inserted by a joint tendon onto the lateral surface of the surangular.
In addition to the already mentioned AMP, Heaton (1979) identified the PT and PST for the internal jaw adductors. He distinguished between an “anterior” and “posterior” muscle head of the PT [apparently synonymous with “pterygoideus lateralis” and “pterygoideus medius” of Heaton (1979, Figure 24)]. Overall, the PT originated, according to Heaton (1979), from the mediodorsal surface of the pterygoid, including the anterior section of the quadrate process. The PST, subdivided into “pseudotemporalis superficialis” and “pseudotemporalis profundus” after Heaton (1979), originated from the epipterygoid. Heaton (1979) reconstructed a mostly tendinous insertion of the internal jaw adductors onto the mandibular fossa, coronoid, medioposterior prearticular, and onto the medial articular.
The reconstructions of Fox (1964) and Heaton (1979) differ markedly from an earlier proposal of Adams (1919) on the jaw muscles of the captorhinid Labidosaurus. Like Fox (1964), Adams (1919) argued that the AMES would attach to the jugal and squamosal, but also to the quadrate instead of the quadratojugal. The AMEP instead would have not been attached to the skull roof, but only to the pterygoid and epipterygoid [“alisphenoid” in Adams (1919)]. He further reconstructed the AMEM as attaching mostly to the parietal and squamosal, which resembles the reconstruction by Heaton (1979) and effectively may be the attachment sites Fox (1964) suggested for the AMEP in Captorhinus. Adams (1919) suggested that the external adductors would insert onto the suprameckelian fossa, the PT ventrally onto the retroarticular process. The cranial attachment sites proposed by Adams (1919) for the PT are similar to the ones by Fox (1964) and Heaton (1979); i.e., attaching to the pterygoid]. Adams (1919) did not discuss the AMP and PST. None of the cited references discussed the CID.
Based on our observations on bone structure and comparisons with published extant amniote jaw muscle anatomy (e.g., Diogo et al., 2008; Daza et al., 2011; Ziermann et al., 2018), we would modify and expand the models of Fox (1964) and Heaton (1979) as follows: In our model, the external section consists of a medial (AMEP) and lateral muscle portion. In lateral view, the lateral portion occupies most of the “cheek” region (Figure 12A). It attaches to the jugal and quadratojugal, and it extends dorsally to the squamosal until it meets the ventral bony ridge of the parietal that runs roughly parallel to the parietosquamosal suture (Figure 12C).
Figure 12. Rough position of the jaw adductor musculature in the temporal dermatocranium of OMNH 44816 in medial (A) and ventral view (C); as well as of the internal adductor and constrictor musculature in the palate and epipterygoid in dorsal (D) and lateral view (E), and the insertion of all named muscles onto the mandible in medial view (B). AME, adductor mandibulae externus; AMEM, adductor mandibulae externus medialis; AMEP, adductor mandibulae externus profundus; AMES, adductor mandibulae externus superficialis; AMI, adductor mandibulae internus; AMP, adductor mandibulae posterior; CID, constrictor internus dorsalis; PST, pseudotemporalis; PTD, pterygoideus dorsalis; PTV, pterygoideus ventralis.
It is known for marine turtles that the AMES may separate a distinct lateral muscle portion inserting to the broadly armored “cheek” region (Werneburg, 2011; Jones et al., 2012). When present, AMEM is known to attach anterolaterally to the quadrate and medially to the quadratojugal in many turtles (Schumacher, 1973). As highlighted by Werneburg (2011, 2013a), jaw musculature is highly plastic in its anatomy and basically “follows” the arrangements of the temporal skull bones. In this regard, AMEM, which is relatively well-defined in reptiles with temporal fenestration and placed between AMEP and AMES (Holliday and Witmer, 2007; Jones et al., 2009), is considered to exhibit a more fluent nature in non-fenestrated forms. That said, the homology of particular muscle portions and muscle heads among reptiles is debatable (Rieppel, 1987; Werneburg, 2013a). By positional criteria, AMES of turtles might be homologous to AMEM in other reptiles, and the AMEM of turtles might be identical with AMES of other reptiles. However, as musculature develops from a consistent cell mass in early ontogeny and differentiates based on perinatal requirements of the respective animal (Werneburg, 2019), the developmental fate and differentiation of muscle portions might actually be unique to each individual taxon. Medially to the AMES, in our assessment, the AMEP would attach to the postfrontal, postorbital, and parietal (Figure 12C). Both external adductors would fuse at the height of the squamosal and insert onto the coronoid process of the mandible (Figure 12B). The internal adductor section would comprise an anterior (PT) and posterior (AMP) muscle portion, an intercalated pseudotemporalis (PST), and a constrictor internus dorsalis (CID) part. The PT would cover the dorsal face of the dermal palate and reach anteriorly to the palatine as indicated by the smooth transition between palatine and pterygoid (Figures 12D,E). The PST would attach to the lateral face of the posterior process of the pterygoid, to the (mainly cartilaginous) lateral braincase wall, and maybe with a few fibers to the epipterygoid (sensu Heaton, 1979; Figure 12E). The AMP would attach to the anterolateral surface of the quadrate (Figure 12A). All three muscle portions of the internal adductor section would insert onto the posterior part of the mandibular fossa (Figure 12B). The CID is here considered to have been present around the epipterygoid, with an anterior and a posterior part, and attach to the pterygoid dorsally (Figure 12E). It is difficult to reconstruct levator arcus palatini, which would dorsally connect to the skull roof and/or the lateral wall of the braincase and might help to position the palate. Other muscles of the head were not considered for our assessment. Heaton (1979) provided reconstructions of several muscle heads for different muscles, which we think are much too speculative to discern given the rather unspecific osteological correlates in this fossil.
Our assessments on suture morphology and jaw adductors allow further inferences on cranial kinesis and elasticity. Cranial kinesis describes the movement of one or more bones relative to other bones or set of bones along intracranial sutures. Among extant tetrapods, it is present in various squamates, birds, and lissamphibians, but effectively absent in mammals, crocodylians, turtles, and tuatara (e.g., Versluys, 1910; Frazzetta, 1962; Bock, 1964; Iordansky, 1990; Metzger, 2002; Holliday and Witmer, 2008; Jones et al., 2011; Natchev et al., 2016; Werneburg and Maier, 2019; Yaryhin and Werneburg, 2019). The presence and evolution of cranial kinesis has been also discussed for various extinct clades (e.g., Versluys, 1910, 1912; Carroll, 1969a; Bramble and Wake, 1985; Iordansky, 1990; Clack, 2002; Holliday and Witmer, 2008; Cost et al., 2020), even though the inapplicability of in vivo studies represents a considerable limitation. In fact, intracranial mobility observed in prepared specimens does not necessarily correspond to movements actually exhibited by the living animal [Evans, 2008; Holliday and Witmer, 2008; however, see also Iordansky (2011)]. Cranial kinesis proper, which describes active movements of skull parts due to muscle action, should further be distinguished from passive elastic/flexible movements of bone and cartilage due to applied strain (Fracasso, 1983; Rayfield, 2005; Moazen et al., 2009). Indeed, all skulls require at least some degree of elasticity/flexibility to avoid breakage; hence, “passive kinesis” is also present in skulls that might usually be considered akinetic in terms of cranial kinesis proper (Thomson, 1967; Beaumont, 1977; Kathe, 1999; Herring, 2008; Natchev et al., 2016). It is expected that even “stepped joints” and distinctly interdigitated sutures still react elastically to mechanical stimuli, especially when the respective bones are thin in the sutural area (Beaumont, 1977; Clack, 2002; Natchev et al., 2016). The relationship between elasticity at intracranial sutures and the evolution of cranial kinesis proper is uncertain (Moazen et al., 2009).
Using the examples of lepidosaurs and bird-line archosaurs, Holliday and Witmer (2008) provided a list of criteria that need to be fulfilled to allow cranial kinesis proper. In the following, we will discuss these criteria based on our observations on OMNH 44816 and whether previously defined types of cranial kinesis (i.e., at the basicranial joint; otic joint; pleurokinesis; metakinesis; mesokinesis; prokinesis; rhynchokinesis) were present in C. aguti.
For the condylar basicranial joint (i.e., between the palate and neurocranium), we can confirm previous observations in Captorhinus specimens (Warren, 1961; Heaton, 1979; however, see also Olson, 1951; Fox and Bowman, 1966) that the pterygoid does not directly contact the parabasisphenoid. Instead, the joint is formed only between parabasisphenoid and epipterygoid (see Werneburg and Maier, 2019). The basicranial joint is plesiomorphic in tetrapods (Thomson, 1967; Beaumont, 1977; Iordansky, 1990; Porro et al., 2015) and notably immobilized in extant turtles (e.g., Gaffney, 1979; Werneburg and Maier, 2019; Ferreira et al., 2020), further highlighting their more derived state relative to early scutal reptiles. Presence of a synovial basicranial joint is the first criterion defined for cranial kinesis (“basipterygopterygoid joint” in Holliday and Witmer, 2008); however, whether a condylar joint was indeed synovial is hard to determine for fossil specimens (Bailleul and Holliday, 2017) and even if present, it might not necessarily imply any form of cranial kinesis proper (Holliday and Witmer, 2008; Johnston, 2010; Payne et al., 2011). Nevertheless, using a modularity approach of skull network integrity, Werneburg and Abel (2022) reconstructed a clear distinction between the palate and epipterygoid on one side and the braincase on the other side suggesting the presence of ancestral basicranial mobility in C. aguti. The anatomical network approach (AnNA) uded by the authors does not consider suture anatomy but only the presence and absence of bone contact with bones contacting many other bones being more integrated and, hence, less labile or mobile in a functional sense. This methodology might be understood as additional line of evidence to understand intracranial mobility (Werneburg and Abel, 2022).
Iordansky (1990, 2011, 2015) considered pleurokinesis (i.e., mediolateral movement of the quadrate together with other “maxillobuccal” segments relative to the axial parts of the skull) to be present in the earliest amniotes [“reptiles” in Iordansky (1990, 2011, 2015)]. Indeed, the butting contact of the quadrate with the quadrate wing of the pterygoid as well as the probable attachment site of the AMP suggest that some degree of mediolateral movement could have been possible in the quadrate of C. aguti. Yet, this was certainly restricted by the contact of the quadrate with the quadratojugal and squamosal. In fact, a condylar and, therefore, potentially synovial otic joint like it can be observed in many later diverging taxa is not observable in OMNH 44816, C. aguti thereby lacked an important criterion for cranial kinesis sensu Holliday and Witmer (2008). Similar to the basicranial joint, pleurokinesis has been considered to be the plesiomorphic condition in tetrapods (Iordansky, 1990) and proposed for various extant and extinct taxa like lissamphibians (e.g., Iordansky, 2000; Natchev et al., 2016), squamates (e.g., Iordansky, 2004, 2015), or ornithopod dinosaurs (e.g., Norman and Weishampel, 1985); however, unambiguous evidence for active pleurokinesis in any of these taxa is rare at best, if present at all (Evans, 2008; Holliday and Witmer, 2008; Cuthbertson et al., 2012; Heiss and Grell, 2019; but see also Werneburg and Abel, 2022).
A metakinetic joint (i.e., movement of the temporal dermatocranium, together with the snout, relative to the occiput; Frazzetta, 1962) has been repeatedly described for early amniotes (e.g., Versluys, 1912; Carroll, 1969a; Gow, 1972; Bramble and Wake, 1985; Iordansky, 1990), even though its nature in extant taxa has remained barely understood until recently (Mezzasalma et al., 2014; Handschuh et al., 2019), where it is mainly present in squamates (Evans, 2008; Jones et al., 2011), but maybe also in some other tetrapods (Natchev et al., 2016). In OMNH 44816, bony contacts between the temporal dermatocranium and the braincase are only present between the postparietal and supraoccipital, as well as between squamosal and opisthotic. Based on other Captorhinus specimens, the supraoccipital was also dorsally sutured to the parietal by a bony process (Fox and Bowman, 1966; Heaton, 1979) and might have been also sutured to the squamosals (Price, 1935). Even though captorhinids shared with later-diverging taxa the loss of the tabulars (e.g., Modesto et al., 2007), especially the retained contact between postparietals and supraoccipital argues against any major mobility in the metakinetic axis of C. aguti (Evans, 2008). Werneburg and Abel (2022) found the braincase to form a separate functional module in C. aguti, which would argue for some form of metakinesis or for a weaker connection at the metakinetic axis at least that could serve as a precondition to establish metakinesis later in evolution.
Mesokinesis (sensu Frazzetta, 1962; i.e., active movements of the parietals relative to the frontals) can be excluded for C. aguti based on the stepped interdigitation between the respective bones. In fact, mesokinesis can be considered a highly derived condition mostly restricted to squamates and some caudates (Frazzetta, 1962; Bramble and Wake, 1985; Natchev et al., 2016). However, this does not necessarily exclude any form of elasticity in the frontoparietal region (Natchev et al., 2016; Werneburg and Abel, 2022). When the jaw was closed in C. aguti, the AMEP probably exerted a pulling force on the parietals relative to the frontals, distributing force onto the frontoparietal suture, eventually triggering the development of a more complexly stepped interdigitation there. Nevertheless, the comparatively high thickness in the sutures of the frontal region in C. aguti (Supplementary Table 2) as well as the external bony ridges might additionally have decreased the degree of elastic movements. Werneburg and Abel (2022) found a modular distinction between frontals and parietals, but based on the results presented herein, they basically refuted mesokinesis in C. aguti. The distinction between two modules in this area of the skull might hint at functionally differentiated skull parts in C. aguti—that moved against each other by elasticity as assumed herein—or to an ancestral differentiation of the skull inherited from more rootward tetrapods.
Likewise, action of AMEM (of our reconstruction) and AMES onto the “cheek” might have also exerted pulling forces on the jugal, squamosal, and postorbital. Being overall thinner than the parietal, elasticity was probably generally higher in the “cheek” than in the skull roof. Like in the frontoparietal suture, the pulling force on the “cheek” likely selected for a similarly stepped interdigitation between these bones (Figure 13C, sutures II, III, V). Yet, the contact of the parietals with the “cheek” is less complex and might even be considered “weak” (Frazzetta, 1968). However, this area seems to be more stabilized against torsion due to the lamina extruding from the squamosal medially to meet a medioventral ridge of the parietal (Figure 13B). Only the parietal-postorbital contact still appears to be simple (Figure 13A). Nevertheless, the high modular integrity of the postorbital within the dermatocranium and the more complex sutures to its neighboring bones, as well as the parietal-squamosal contact, likely restricted further mobility (Werneburg and Abel, 2022). Yet, the simple nature of the parietal-postorbital suture could indicate that it was less affected by compressional forces than the other sutures in the temporal region (Figure 13C).
Figure 13. Cross section through the temporal region of OMNH 44816 in posterior view highlighting the internal contacts of the dermal bones. (A) Anterior temporal region; (B) posterior temporal region. (C) 3D view on the dermal sutures in the temporal region of OMNH 44816. Dashed lines indicate potentially weak areas that could correspond to temporal openings in other early amniotes.
A joint between the snout and more posterior parts of the skull, as it occurs in most batrachians, snakes, and birds (prokinesis, rhynchokinesis; Bock, 1964; Iordansky, 1990), can be excluded for C. aguti. The frequently stepped interdigitations in the snout likely evolved to withstand the forces generated from the interaction between the tooth-bearing maxilla and food items (Jones and Zikmund, 2012). This is further evident by the relatively thick sutures between the maxilla, lacrimal, and jugal. The force was probably absorbed by the thinner and, hence, more elastic sutures in more dorsal areas of the snout (between lacrimal, nasal, and prefrontal). Analogous to the parietal-postorbital suture, the simple frontal-prefrontal suture might indicate that this skull area was less affected by compressional forces generated during biting (see also Werneburg and Abel, 2022), but again the high integrity of the prefrontal, as well as the thicker suture, argues against any major mobility.
The derived condition and possible mobility of the palate in Captorhinus and other early amniotes has been highlighted by previous authors (Fox, 1964; Carroll, 1969a; Bramble and Wake, 1985; however, see also Heaton, 1979, for an opposing view). At the anterior end of the palate, both vomers as well as both pterygoids were likely only loosely connected to each other. Likewise, the articulation between vomer and premaxilla was likely rather simple (Fox and Bowman, 1966). As described by Fox (1964) and Fox and Bowman (1966), and confirmed by our observations herein, there was a joint between the palatine and maxilla that could, on its own, have allowed some rotational movement of the palate. Yet, we agree with Bolt (1974) that in C. aguti the palatine also sutured to the lacrimal, which would have restricted rotational motion. Further restriction was likely caused by the anterolateral contact of the pterygoid with the jugal. We were not able to confirm a contact between palatine and the ventral process of the prefrontal (see Williston, 1925; Bolt, 1974). While there was no true kinetic joint between the palatine, vomer, and pterygoid, the relatively thin palatines suggest that the middle palate was quite elastic and would have bent dorsally by action of the dorsally attaching PT when the jaw was closed. The epipterygoid is ancestrally not fused to the braincase (as in mammals, turtles, and crocodylians) in C. aguti, suggesting the presence of the epipterygoid-associated CID musculature. Together, they might have permitted a certain pro- and retraction of the palate relative to the rest of the skull. The differentiation of CID into a “protractor pterygoidei,” another criterion for cranial kinesis proper in lepidosaurs and bird-line archosaurs (Holliday and Witmer, 2008), cannot be confirmed for C. aguti.
In summary, none of the traditionally defined types of cranial kinesis can be unambiguously confirmed for C. aguti. While we do not exclude that at least some of the criteria by Holliday and Witmer (2008) could have been fulfilled by C. aguti (i.e., synovial basicranial joint, m. protractor pterygoideus), we conclude that C. aguti, and likely also other early scutal reptiles, were functionally akinetic in terms of cranial kinesis proper. Nevertheless, due to observed differences in suture morphology and thickness, we expect the degree of passive elastic movements to differ depending on the respective skull part and, also, between different parts of the temporal dermatocranium. We wish to highlight that the criteria of Holliday and Witmer (2008) were erected for and nicely apply to extant diapsids, but our discussion might motivate future studies on intracranial mobility beyond traditional perceptions and categorizations.
Taking our morphological considerations, as well as comparisons with other taxa into account, we were able to roughly reconstruct the cranial mechanics in C. aguti that likely applies also to other early scutal reptiles. We argue that this offers new insights into the evolution of the amniote temporal region and allows us to infer how temporal openings might have formed in early amniotes.
It can be debated whether C. aguti actually represents a suitable model for this approach. As stated previously, the presence of several derived traits in the skull of C. aguti as well as its geological age urge to caution in using it as an analog for the condition in the hypothetical ancestral amniote. To complicate things further, interrelationships at the base of Amniota are still widely discussed (e.g., Laurin and Piñeiro, 2017; MacDougall et al., 2018; Klembara et al., 2019; Mann et al., 2019, 2021; Ford and Benson, 2020). Hence, the ancestral morphology of the amniote skull, and especially the point of when as well as how often temporal openings appeared, is difficult to reconstruct (Laurin and Piñeiro, 2017; Ford and Benson, 2020; Abel and Werneburg, 2021). However, due to reasons mentioned in the Introduction section, we think Captorhinus aguti may well be, for now, an acceptable representative to investigate the origins of temporal fenestration in ancestral amniotes (see also Maier, 1993, 1999). We will also further discuss in this chapter differences and similarities between C. aguti and other taxa, notably earlier captorhinids, “protorothyridids”, and some other early potential amniotes.
In case of the “cheek,” the cranial mechanics of C. aguti can be generally described as outlined by Fox (1964). Contraction of the AMES/AMEM (in our reconstruction) would have exerted a ventromedial bending of the bones in the “cheek” region. Additionally, the quadrate would have directed force from posterior onto the squamosal and quadratojugal. This might explain the more stabilized squamosal-quadratojugal contact by the distinctly extruding bony lamina from the quadratojugal medial to the squamosal (Figure 13B). We can confirm the observation of Fox (1964) that there is a thin area at the intersection of jugal, squamosal, and postorbital (Supplementary Table 2), suggesting that it was less affected by muscle forces. Appropriately, the interdigitation that runs dorsoventrally to form most of the anterior contact of the squamosal with jugal and postorbital becomes a less complex suture in the intersection area of the three bones. Fox (1964) reports further that the marginal areas of the “cheek” were strengthened in Captorhinus by medially aligned ridges that might have also served as muscle attachment sites (at least for the stronger tendinous attachments, see above). This is also backed up by our own observations. According to the latter, these would also correspond to the external patterns of bone ornamentation, probably another response to force distribution. Such a “network of lines of stress” (Olson, 1961) on its own might select against ossification in lesser loaded areas of the “cheek,” eventually forming an opening (e.g., Case, 1924; Olson, 1961; Fox, 1964). Intersections between more than two bones like they occur in the “cheek” of C. aguti (Figure 13C) might be especially prone to forming a temporal opening (Frazzetta, 1968; Kuhn-Schnyder, 1980; Werneburg and Abel, 2022).
Indeed, there is more evidence for this scenario in other taxa. Next to their typical infratemporal fenestra between jugal, squamosal, and postorbital, some specimens of the early synapsids Ophiacodon retroversus Cope (1878) and Varanosaurus acutirostris Broili 1904 also exhibit an “accessory temporal fenestra” at their thinned jugal-squamosal-quadratojugal intersection; sometimes even restricted to only one side of the skull (Romer and Price, 1940; Frazzetta, 1968; Berman et al., 1995; Ford, 2018). In the usually non-fenestrated parareptile Procolophon trigoniceps Owen 1876, small infratemporal fenestrae can appear in-between different sets of three to four bones (Cisneros, 2008). Moving more rootward, Jaekel (1902) reported for the possible amniote-line tetrapod Gephyrostegus bohemicus Jaekel, 1902 thin regions in the “cheek” and parietal area, presumably fitting to the position of temporal openings in later amniotes. These traits have not been mentioned in a recent reassessment of the species (Klembara et al., 2014) and until now, we were not able to study the fossils in person to confirm or refute Jaekel’s (1902) observation. Conclusively, it might be nevertheless rather easy to form small temporal openings within thin bone intersections, with their occasional presence representing no major disadvantage for the animal (Romer and Price, 1940; Cisneros, 2008).
While we currently do not possess data on relative suture thickness in other early scutal tetrapods, a jugal-squamosal-postorbital intersection like observed in C. aguti is widespread among scutal taxa close to the amniote base (e.g., Clark and Carroll, 1973; Berman et al., 1988; Boy and Martens, 1991; Modesto et al., 2007; Kissel, 2010). Like infratemporal fenestrae (e.g., Abel and Werneburg, 2021), these intersections vary in their relative position in the “cheek,” probably related to differences in muscle force distribution. In fact, the latter is thought to play a significant role for the formation of temporal openings during early ontogeny (Werneburg, 2019). Such variation in jaw adductor arrangement might also explain why in many parareptiles the infratemporal fenestra is located within the jugal-squamosal-quadratojugal intersection instead (MacDougall and Reisz, 2014; 3 in Figure 14), whereas in many non-sphenacodontian synapsids as well as in some early diapsids, jugal, squamosal, postorbital, and quadratojugal form the margin of the infratemporal fenestra (Romer and Price, 1940; Ford and Benson, 2020). In regard of the condition in non-sphenacodontian synapsids and early diapsids, the formation of the fenestra may be explained by a larger weak area in the “cheek” incorporating both discussed three-bone-intersections. Alternatively, the formation of “accessory temporal fenestrae” like observed for some ophiacodontids as mentioned above could have led to a later fusion of two separate temporal openings (see also Werneburg and Abel, 2022).
Figure 14. Drawing of the skull of Captorhinus aguti after Fox and Bowman (1966) with possible origin sites for temporal openings in the hypothetical ancestral amniote. 1, squamosal-postorbital-parietal intersection; 2, jugal-squamosal-postorbital intersection; 3, jugal-squamosal-quadratojugal intersection. Sites 1 and 2 are the most likely origin sites for a taxon similar to Captorhinus. Site 3 occurs especially in Parareptilia. Temporal openings in early Synapsida and Diapsida formed probably by fusion of two of such origin sites (see also Werneburg and Abel, 2022).
In fact, this is congruent with our own observations on Protorothyris archeri Price (1937) (MCZ 1532, 2148; Museum of Comparative Zoology, Harvard, United States), a close relative of Diapsida, which still possessed a scutal morphology (e.g., Müller and Reisz, 2006; MacDougall et al., 2018; Ford and Benson, 2020). P. archeri is similar to C. aguti in regards of the jugal interdigitating with the squamosal posteriorly. Likewise, the parietal overlaps the squamosal, and there also seems to be a butt joint between parietal and postorbital. However, it differs from C. aguti by the lack of interdigitation in the postorbital-squamosal suture and the simpler contact between jugal and quadratojugal. These might be suitable preconditions for the formation of two pairs of temporal fenestrae in the diapsid ancestor. Yet, as we currently have no information on relative suture thickness in P. archeri, segmentation of a μCT-scan like herein applied to OMNH 44816 would be needed to further elaborate on this hypothesis.
There are more reasons to assume that as a model captorhinids alone might not be able to explain the whole morphological diversity of the temporal region in early amniotes. An infratemporal fenestra corresponding to the weak area in C. aguti (i.e., within the jugal-squamosal-postorbital intersection; 2 in Figure 14) occurs mostly, likely as a derived trait, in taxa with a reduced quadratojugal (e.g., Romer and Price, 1940; Modesto and Reisz, 1990), sometimes by also involving the parietal (Modesto, 1995; Lucas et al., 2018). In fact, while especially moradisaurine captorhinids likely evolved larger external jaw adductors in context of their derived herbivorous lifestyle, neither they or any other known captorhinid evolved an infratemporal fenestra like it can be observed in coeval herbivorous synapsids, but enlarged their adductor chamber instead (Dodick and Modesto, 1995; Sues and Reisz, 1998). This might be due to other constraints like skull flattening during ontogeny, especially in more deeply nested captorhinids (Heaton, 1979). Indeed, skull doming and accompanying changes in jaw adductor orientation has been repeatedly argued to be necessary for the initial evolution of temporal openings (Olson, 1961; Frazzetta, 1968; Tarsitano et al., 2001; Abel and Werneburg, 2021), even though it might have been the opposite in lissamphibians (Schoch, 2014). There might be also a phylogenetic signal due to herbivorous synapsids retaining their fenestrae from their fenestrated non-herbivorous ancestors. Considering this and other peculiarities of the captorhinid temporal region, notably the loss of the tabular and the distinctly reduced supratemporal (e.g., Fox and Bowman, 1966; Dodick and Modesto, 1995), it emphasizes that even early scutal radiations like captorhinids might not offer a “perfect” analog for the ancestral amniote.
Lastly, the probably weak connection between the parietal and “cheek” (i.e., postorbital and, to a lesser degree, squamosal) could have been related to what Kemp (1980) called the “crossopterygian hinge line,” a mobile joint presumably inherited from tetrapodomorph fishes and retained as a loose contact between squamosal and supratemporal in probable amniote-line tetrapods and other early limbed vertebrates (Panchen, 1964; Thomson, 1967; Frazzetta, 1968; Kemp, 1980; Klembara et al., 2014).
In this regard, Kemp (1980) highlighted that the temporal region of early synapsids plesiomorphically bears large supratemporals and tabulars; thus, they differ from C. aguti and other early non-diapsid reptiles. The author suggested that the synapsid fenestra originated between postorbital, squamosal, and supratemporal by attaching the jaw adductors around the former “crossopterygian hinge line.” The postorbital would have later extended posteriorly along the lateroventral margin of the supratemporal. The argumentation of Kemp (1980) implies that the synapsid fenestra would have subsequently expanded to also incorporate the jugal and quadratojugal into its border. The possible role of the “crossopterygian hinge line” has been also discussed by others (Frazzetta, 1968; Gow, 1972; Werneburg and Abel, 2022). In regard of the parareptile Milleretta rubidgei Broom 1938, which possessed a small opening between the jugal, squamosal, and postorbital that was presumably closed in adult specimens, Gow (1972) argued that a firm suture between squamosal and skull roof was present. This would have led to the formation of a “line of weakness” between the squamosal, jugal, and quadratojugal in M. rubidgei at whose dorsal end the opening formed. A similar case might have been also present in the embolomere Anthracosaurus russelli Huxley 1863, which differs from other embolomeres by interdigitation of the suture between skull roof and “cheek” as well as the formation of an infratemporal fenestra between jugal and squamosal, adjacent to the jugal-squamosal-quadratojugal intersection (Panchen, 1977; Clack, 1987a).
It is worth noting that there are several other interpretations of this presumed “hinge line,” which either has been argued to represent a taphonomic phenomenon (Reisz and Heaton, 1980), a misinterpretation of the sutures (Berman et al., 2010) or, if present, to be of little functional relevance (Clack, 1987b). Also, given that the jugal seems to be always involved in the infratemporal fenestra of early synapsids, we consider it more parsimonious that the fenestra would form within one of the three-bone intersections discussed above. This would also apply to other taxa like diapsids or especially to those in which the postorbital does not contribute to the infratemporal fenestra like in A. russelli or many parareptiles. Moreover, an infratemporal fenestra bordered by the jugal might still develop if there is a weaker contact between the skull roof and “cheek” (Frazzetta, 1968). Nonetheless, a contact as observed for C. aguti and probably other scutal non-diapsid reptiles might have implications for the formation of the diapsid supratemporal fenestra (Werneburg and Abel, 2022).
When the jaw was closed in C. aguti, contraction of the AMEP would have exerted a pulling force with the parietals bending in ventral direction, concentrating the main forces onto the anterior and posterior ends of the parietals, while their lateral contacts to the “cheeks” would have been less affected. Like discussed in the previous section, this could have maintained the plesiomorphically interdigitated suture found between parietals and frontals. Indeed, such an interdigitated frontoparietal suture can be also observed in many other species close to the amniote base, including other captorhinids even though with varying degree of complexity (e.g., Clark and Carroll, 1973; Gaffney and McKenna, 1979; Heaton, 1979; Dodick and Modesto, 1995; Kissel et al., 2002; Müller and Reisz, 2005; Modesto et al., 2007; Kissel, 2010).
The simpler contacts of the parietal to the squamosals and postorbitals fit their interpretation as less affected areas. Analogous to the formation of the infratemporal fenestra, the less loaded parietal-postorbital and parietal-squamosal contacts could be selected to form a temporal opening. Especially the simple parietal-postorbital contact would be affected by this. In moradisaurine captorhinids with their enlarged adductor chamber, the sutures between skull roof and “cheek” appear to be more strengthened than in C. aguti (Dodick and Modesto, 1995), whereas in other early non-diapsid reptiles, including the ones closest to diapsids (e.g., Protorothyris Price, 1937), the parietal simply overlapped the postorbital and squamosal (Carroll, 1969b; Carroll and Baird, 1972; Clark and Carroll, 1973), indicating that the ancestor of diapsids still possessed a comparatively weak suture there and, hence, the suggested precondition to form a supratemporal fenestra (1 in Figure 14). The question remains why an amniote supratemporal fenestra has only evolved in diapsids. Observation of a weaker contact between skull roof and “cheek” in some early synapsids (Frazzetta, 1968) might suggest that the potential to evolve a more dorsal fenestra was also present in synapsids. Indeed, expansion of the infratemporal fenestra toward the parietals in early therapsids and other sphenacomorph synapsids (Boonstra, 1936; Romer and Price, 1940; Modesto, 1995; Kammerer, 2011) could have been enabled by a still rather weak connection in that region of the skull.
There might be other factors involved for the lack of temporal openings in most other early limbed vertebrates. These include a less mobile head-neck joint (Panchen, 1964; Kuhn-Schnyder, 1980), differing jaw mechanics (Olson, 1961; Clack, 1987b) with a, hence, different role of the external jaw adductors, eventually affected by differences in ontogenetic strategies (Werneburg, 2019). Studies on fenestrated lissamphibians (e.g., Paluh et al., 2020) could also provide more insights into morphological patterns underlying tetrapod temporal openings. However, more detailed data on the suture morphology of lissamphibians and other non-amniotes is needed to comprehend the biomechanical differences between fenestrated and non-fenestrated taxa.
Our reconstruction of the likely arrangement of jaw adductors and cranial mechanics in Captorhinus aguti highlighted the biomechanical differences between the non-fenestrated skull of an early reptile with equally non-fenestrated skulls in extant turtles and possible extinct amniote-line tetrapods. We confirm previous observations of a comparatively thin area in the jugal-squamosal-postorbital intersection (2 in Figure 14) but also report another thin area for the parietal-postorbital contact (1 in Figure 14). These correspond to the position of temporal fenestrae in other early amniotes, corroborating the hypothesis that such openings formed due to the reduction of less loaded areas. Yet, consideration of captorhinid evolution also emphasizes that an increasing role of the external jaw adductors does not necessarily lead to the formation of temporal openings. Hence, even a generalist captorhinid like C. aguti might not be the best analog for the ancestor of fenestrated amniotes. Future studies on taxa closer to early fenestrated amniotes (e.g., “protorothyridids”) or of different early diverging taxa (e.g., embolomeres, Gephyrostegus, seymouriamorphs, “lepospondyls”, diadectomorphs), as well as quantitative approaches, might further deepen our understanding of the early evolution of temporal openings.
The original contributions presented in the study are included in the article/Supplementary Material, further inquiries can be directed to the corresponding author/s.
PA and IW: manuscript writing and conceptualization. PA, YP, and IW: analyses and illustrations. DF: material providing. IW and DK: fund raising. All authors critically revised the manuscript.
This study was funded by DFG grant WE 5440/6-1 (to IW).
The authors declare that the research was conducted in the absence of any commercial or financial relationships that could be construed as a potential conflict of interest.
All claims expressed in this article are solely those of the authors and do not necessarily represent those of their affiliated organizations, or those of the publisher, the editors and the reviewers. Any product that may be evaluated in this article, or claim that may be made by its manufacturer, is not guaranteed or endorsed by the publisher.
Gabriel Ferreira (University of Tübingen) supported the segmentation process in Avizo. Richard Cifelli (Sam Noble Oklahoma Museum of Natural History) and Nicholas Czaplewski (Sam Noble Oklahoma Museum of Natural History) kindly provided access to OMNH 44816. The scan was created by Matthew Colbert (University of Texas at Austin) and prepared by Jessica Maisano (University of Texas at Austin). We thank JF and DM for their valuable comments that enhanced our manuscript. Lastly, we thank Robert Reisz, Sean Modesto, and two reviewers for comments/support with a former version of this manuscript.
The Supplementary Material for this article can be found online at: https://www.frontiersin.org/articles/10.3389/fevo.2022.841784/full#supplementary-material
Abdala, F., and Damiani, R. (2004). Early development of the mammalian superficial masseter muscle in cynodonts. Palaeontologica Africana 40, 23–29.
Abel, P., Pommery, Y., Ford, D. P., Koyabu, D., Werneburg, I., et al. (in press). 3D models related to the publication: Skull sutures and cranial mechanics in the Permian reptile Captorhinus aguti and the evolution of the temporal region in early amniotes. MorphoMuseuM. doi: 10.18563/m3.167
Abel, P., and Werneburg, I. (2021). Morphology of the temporal skull region in tetrapods: research history, functional explanations, and a new comprehensive classification scheme. Biolog. Rev. 96, 2229–2257. doi: 10.1111/brv.12751
Adams, L. A. (1919). A memoir on the phylogeny of the jaw muscles in recent and fossil vertebrates. Ann. N Y Acad. Sci. 28, 51–166. doi: 10.1111/j.1749-6632.1918.tb55350.x
Bailleul, A. M., and Holliday, C. M. (2017). Joint histology in Alligator mississippiensis challenges the identification of synovial joints in fossil archosaurs and inferences of cranial kinesis. Proc. R. Soc. B 284:20170038. doi: 10.1098/rspb.2017.0038
Barghusen, H. R. (1973). The adductor jaw musculature of Dimetrodon (Reptilia. Pelycosauria). J. Paleontol. 47, 823–834.
Beaumont, E. H. (1977). Cranial morphology of the Loxommatidae (Amphibia: Labyrinthodontia). Philosoph. Trans. R. Soc. Lond. 280, 29–101. doi: 10.1098/rstb.1977.0099
Berman, D. S., Eberth, D. A., and Brinkman, D. B. (1988). Stegotretus agyrus a new genus and species of microsaur (amphibian) from the Permo-Pennsylvanian of New Mexico. Ann. Carn. Mus. 57, 293–323.
Berman, D. S., Reisz, R. R., Bolt, J. R., and Scott, D. (1995). The cranial anatomy and relationships of the synapsid Varanosaurus (Eupelycosauria: Ophiacodontidae) from the Early Permian of Texas and Oklahoma. Ann. Carn. Mus. 64, 99–133.
Berman, D. S., Reisz, R. R., and Scott, D. (2010). Redescription of the skull of Limmoscelis paludis Williston (Diadectomorpha: Limnoscelidae) from the Pennsylvanian of Cañon del Cobre, Northern New Mexico. New Mex. Mus. Nat. Hist. Sci. Bull. 49, 185–210.
Blackburn, D. G., and Stewart, J. R. (2021). Morphological research on amniote eggs and embryos: an introduction and historical retrospective. J. Morphol. 282, 1–23. doi: 10.1002/jmor.21320
Bock, W. J. (1964). Kinetics of the avian skull. J. Morphol. 114, 1–42. doi: 10.1002/jmor.1051140102
Bolt, J. R. (1974). Evolution and functional interpretation of some suture patterns in Paleozoic labyrinthodont amphibians and other lower tetrapods. J. Paleontol. 48, 434–458.
Boonstra, L. D. (1936). On some features of the cranial morphology of the tapinocephalid deinocephalians. Bull. Am. Mus. Nat. Hist. 72, 75–98.
Boy, J. A., and Martens, T. (1991). Ein neues captorhinomorphes Reptil aus dem thüringischen Rotliegend (Unter-Perm; Ost-Deutschland). Paläontol. Zeitschrift 65, 363–389. doi: 10.1007/BF02989852
Bramble, D. M., and Wake, D. B. (1985). “Feeding Mechanisms of Lower Tetrapods,” in Functional Vertebrate Morphology, eds M. Hildebrand, D. Bramble, K. Liem, and D. Wake (Cambridge, MA: Harvard University Press), 230–261. doi: 10.4159/harvard.9780674184404.c13
Branson, E. B. (1911). Notes on the osteology of the skull of Pariotichus. J. Geol. 19, 135–139. doi: 10.1086/621823
Bryant, H. N., and Seymour, K. L. (1990). Observations and comments on the reliability of muscle reconstruction in fossil vertebrates. J. Morphol. 206, 109–117. doi: 10.1002/jmor.1052060111
Buchwitz, M., Jansen, M., Renaudie, J., Marchetti, L., and Voigt, S. (2021). Evolutionary change in locomotion close to the origin of amniotes inferred from trackway data in an ancestral state reconstruction approach. Front. Ecol. Evol. 9, 1–24. doi: 10.3389/fevo.2021.674779
Busbey, A. B. (1995). “The structural consequences of skull flattening in crocodiles,” in Functional Morphology in Vertebrate Paleontology, ed. J. J. Thomason (Cambridge, MA: Cambridge University Press), 173–192.
Carroll, R. L. (1969a). Problems of the origins of reptiles. Biolog. Rev. 44, 393–432. doi: 10.1111/j.1469-185X.1969.tb01218.x
Carroll, R. L. (1969b). A Middle Pennsylvanian captorhinomorph, and the interrelationships of primitive reptiles. J. Paleontol. 43, 151–170.
Carroll, R. L., and Baird, D. (1972). Carboniferous stem-reptiles of the family Romeriidae. Bull. Mus. Comp. Zool. 143, 321–363.
Case, E. C. (1911). A Revision of the Cotylosauria of North America. Washington, D. C.: Carnegie Institution of Washington.
Case, E. C. (1924). A possible explanation of fenestration in the primitive reptilian skull, with notes on the temporal region of the genus Dimetrodon. Contrib. Mus. Geol. Univ. 2, 1–12. doi: 10.15625/2615-9023/15888
Cisneros, J. C. (2008). Taxonomic status of the reptile genus Procolophon from the Gondwanan Triassic. Palaeontol. Afr. 43, 7–17.
Clack, J. A. (1987a). Two new specimens of Anthracosaurus (Amphibia: Anthracosauria) from the Northumberland Coal Measures. Palaeontology 30, 15–26.
Clack, J. A. (1987b). Pholiderpeton scutigerum Huxley, an amphibian from the Yorkshire coal measures. Philosoph. Trans. R. Soc. Lond. 318, 1–107. doi: 10.1098/rstb.1987.0082
Clack, J. A. (2002). The dermal skull roof of Acanthostega gunnari, an early tetrapod from the Late Devonian. Trans. R. So. Edinb. 93, 17–33. doi: 10.1017/S0263593300000304
Clark, J., and Carroll, R. L. (1973). Romeriid reptiles from the Lower Permian. Bull. Mus. Comp. Zool. 144, 353–407.
Cope, E. D. (1878). Descriptions of extinct Batrachia and Reptilia from the Permian formation of Texas. Proc. Am. Philos. Soc. 17, 505–530.
Cope, E. D. (1882). Third contribution to the history of the Vertebrata of the Permian formation of Texas. Proc. Am. Philosoph. Soc. 20, 447–461.
Cost, I. N., Middleton, K. M., Sellers, K. C., Echols, M. S., Witmer, L. M., Davis, J. L., et al. (2020). Palatal biomechanics and its significance for cranial kinesis in Tyrannosaurus rex. Anat. Rec. 303, 999–1017. doi: 10.1002/ar.24219
Crawford, N. G., Faircloth, B. C., McCormack, J. E., Brumfield, R. T., Winker, K., and Glenn, T. C. (2012). More than 1000 ultraconserved elements provide evidence that turtles are the sister group of archosaurs. Biol. Lett. 8, 783–786. doi: 10.1098/rsbl.2012.0331
Crawford, N. G., Parham, J. F., Sellas, A. B., Faircloth, B. C., Glenn, T. C., Papenfuss, T. J., et al. (2015). A phylogenomic analysis of turtles. Mole. Phylogenet. Evol. 83, 250–257. doi: 10.1016/j.ympev.2014.10.021
Curtis, N., Jones, M. E. H., Evans, S. E., O′Higgins, P., and Fagan, M. (2013). Cranial sutures work collectively to distribute strain throughout the reptile skull. J. R. Soc. Interf. 10, 1–8. doi: 10.1098/rsif.2013.0442
Cuthbertson, R. S., Tirabasso, A., Rybczynski, N., and Holmes, R. B. (2012). Kinetic limitations of intracranial joints in Brachylophosaurus canadensis and Edmontosaurus regalis (Dinosauria: Hadrosauridae), and their implications for the chewing mechanics of hadrosaurids. Anat. Rec. 295, 968–979. doi: 10.1002/ar.22458
Dawson, J. W. (1860). On a terrestrial mollusk, a chilognathous myriapod, and some new species of reptiles, from the Coal-Formation of Nova Scotia. Q. J. Geol. Soc. Lond. 16, 268–277. doi: 10.1144/GSL.JGS.1860.016.01-02.37
Daza, J. D., Diogo, R., Johnston, P., and Abdala, V. (2011). Jaw adductor muscles across lepidosaurs: a reappraisal. Anat. Rec. 294, 1765–1782. doi: 10.1002/ar.21467
deBraga, M., and Rieppel, O. (1997). Reptile phylogeny and the interrelationships of turtles. Zool. J. Linn. Soc. 120, 281–354. doi: 10.1111/j.1096-3642.1997.tb01280.x
Diogo, R., and Abdala, F. (2010). “The Head Muscles of Dipnoans – a Review on the Homologies and Evolution of these Muscles within Vertebrates,” in Biology of Lungfishes, eds J. Joss and J. M. Jorgensen (Boca Raton: CRC Press), 169–218.
Diogo, R., Abdala, V., Lonergan, N., and Wood, B. A. (2008). From fish to modern humans – comparative anatomy, homologies and evolution of the head and neck musculature. J. Anat. 213, 391–424. doi: 10.1111/j.1469-7580.2008.00953.x
Dodick, J. T., and Modesto, S. P. (1995). The cranial anatomy of the captorhinid reptile Labidosaurikos meachami from the Lower Permian of Oklahoma. Palaeontology 38, 687–711.
Egberts, S. (2008). A morphological study of Captorhinus aguti using X-ray computed tomography: new insights on a well-studied species. J. Paleont. 28:74A.
Evans, S. E. (2008). “The skull of lizards and tuatara,” in Biology of the Reptilia, eds C. Gans, A. S. Gaunt, and K. Adler (New York, NY: Society for the Study of Amphibians and Reptiles), 1–347.
Ferreira, G. S., Lautenschlager, S., Evers, S. W., Pfaff, C., Kriwet, J., Raselli, I., et al. (2020). Feeding biomechanics suggests progressive correlation of skull architecture and neck evolution in turtles. Sci. Rep. 10, 1–11. doi: 10.1038/s41598-020-62179-5
Ferreira, G. S., and Werneburg, I. (2019). “Evolution, Diversity, and Development of the Craniocervical System in Turtles with Special Reference to Jaw Musculature,” in Heads, Jaws, and Muscles - Anatomical, Functional, and Developmental Diversity in Chordate Evolution, eds J. M. Ziermann, R. E. Diaz, and Jr. R. Diogo (New York, NY: Springer), 171–206. doi: 10.1007/978-3-319-93560-7_8
Field, D. J., Gauthier, J. A., King, B. L., Pisani, D., Lyson, T. R., and Peterson, K. J. (2014). Toward consilience in reptile phylogeny: miRNAs support an archosaur, not lepidosaur, affinity for turtles. Evol. Dev. 16, 189–196. doi: 10.1111/ede.12081
Ford, D. P. (2018). The Evolution and Phylogeny of Early Amniotes. Dissertation. Oxford: University of Oxford.
Ford, D. P., and Benson, R. B. J. (2020). The phylogeny of early amniotes and the affinities of Parareptilia and Varanopidae. Nat. Ecol. Evol. 4, 57–65. doi: 10.1038/s41559-019-1047-3
Fox, R. C. (1964). The adductor muscles of the jaw in some primitive reptiles. Univ. Kan. Publ. 12, 657–680.
Fox, R. C., and Bowman, M. C. (1966). Osteology and relationships of Captorhinus aguti (Cope) (Reptilia: Captorhinomorpha). Univ. Kans. Paleont. Contrib. Vert. 11, 1–79.
Fracasso, M. A. (1983). Cranial osteology, functional morphology, systematics and paleoenvironment of Limnoscelis paludis Williston. Dissertation. New Haven: Yale University.
Frazzetta, T. H. (1962). A functional consideration of cranial kinesis in lizards. J. Morphol. 111, 287–319. doi: 10.1002/jmor.1051110306
Frazzetta, T. H. (1968). Adaptive problems and possibilities in the temporal fenestration of tetrapod skulls. J. Morphol. 125, 145–157. doi: 10.1002/jmor.1051250203
Gaffney, E. S. (1979). Comparative cranial morphology of recent and fossil turtles. Bull. Am. Mus. Hist. 164, 65–376. doi: 10.7717/peerj.5964
Gaffney, E. S. (1990). The comparative osteology of the Triassic turtle Proganochelys. Bull. Am. Mus. Nat. Hist. 194, 1–263. doi: 10.1127/0077-7749/2007/0246-0001
Gaffney, E. S., and McKenna, M. C. (1979). A Late Permian captorhinid from Rhodesia. Am. Mus. Novit. 2688, 1–15.
Gans, C. (1960). Studies on amphisbaenids (Amphisbaenia, Reptilia) – 1. A taxonomic revision of the Trogonophinae, and a functional interpretation of the amphisbaenid adaptive pattern. Bull. Am. Mus. Nat. Hist. 119, 133–204.
Gaupp, E. (1895). Ueber die Jochbogen-Bildungen am Schädel der Wirbelthiere. Jahres-Bericht der Schlesischen Gesellschaft für vaterländische Cultur 72, 56–63.
Gemmell, N. J., Rutherford, K., Prost, S., Tollis, M., and Winter, D. (2020). The tuatara genome reveals ancient features of amniote evolution. Nature 584, 403–409. doi: 10.1038/s41586-020-2561-9
Gervais, P. (1865). Description du Mesosaurus tenuidens, reptile fossile de l’Afrique australe. Académie des Sciences et Lettres de Montpellier, Mémoires de la Section des Sciences 6, 169–175.
Gow, C. E. (1972). The osteology and relationships of the Millerettidae (Reptilia: Cotylosauria). J. Zool. 167, 219–264. doi: 10.1111/j.1469-7998.1972.tb01731.x
Gruntmejer, K., Konietzko-Meier, D., Marcé-Nogué, J., Bodzioch, A., and Fortuny, J. (2019). Cranial suture biomechanics in Metoposaurus krasiejowensis (Temnospondyli, Stereospondyli) from the Upper Triassic of Poland. J. Morph. 280, 1850–1864. doi: 10.1002/jmor.21070
Haas, A. (2001). Mandibular arch musculature of anuran tadpoles; with comments on homologies of amphibian jaw muscles. J. Morph. 247, 1–33. doi: 10.1002/1097-4687(200101)247:1<1::AID-JMOR1000<3.0.CO;2-3
Haas, A. (2003). Phylogeny of frogs as inferred from primarily larval characters (Amphibia: Anura). Cladistics 19, 23–89. doi: 10.1111/j.1096-0031.2003.tb00405.x
Handschuh, S., Natchev, N., Kummer, S., Beisser, C. J., Lemell, P., Herrel, A., et al. (2019). Cranial kinesis in the miniaturised lizard Ablepharus kitaibelii (Squamata: Scincidae). J. Exp. Biol. 222:jeb198291. doi: 10.1242/jeb.198291
Heaton, M. J. (1979). Cranial anatomy of primitive captorhinid reptiles from the Late Pennsylvanian and Early Permian Oklahoma and Texas. Oklah. Geolog. Surv. 127, 1–84. doi: 10.1130/spe152-p1
Heiss, E., and Grell, J. (2019). Same but different: aquatic prey capture in paedomorphic and metamorphic Alpine newts. Zoolog. Lett. 5, 1–12. doi: 10.1186/s40851-019-0140-4
Herring, S. W. (1972). Sutures – a tool in functional cranial analysis. Acta Anatom. 83, 222–247. doi: 10.1159/000143860
Herring, S. W. (2008). “Mechanical Influences on Suture Development and Patency,” in Craniofacial Sutures. Development, Disease and Treatment, ed. D. P. Rice (Basel: Karger), 41–56. doi: 10.1159/000115031
Herring, S. W., and Mucci, R. J. (1991). In vivo strain in cranial sutures: the zygomatic arch. J. Morph. 207, 225–239. doi: 10.1002/jmor.1052070302
Herring, S. W., and Teng, S. (2000). Strain in the braincase and its sutures during function. Am. J. Phys. Anthrop. 112, 575–593. doi: 10.1002/1096-8644(200008)112:4<575::AID-AJPA10<3.0.CO;2-0
Herring, S. W., Teng, S., Huang, X., Mucci, R. J., and Freeman, J. (1996). Patterns of bone strain in the zygomatic arch. Anat. Rec. 246, 446–457. doi: 10.1002/(SICI)1097-0185(199612)246:4<446::AID-AR4<3.0.CO;2-T
Holliday, C. M., and Witmer, L. M. (2007). Archosaur adductor evolution: integration of musculoskeletal and topological criteria in jaw muscle homology. J. Morphol. 268, 457–484. doi: 10.1002/jmor.10524
Holliday, C. M., and Witmer, L. M. (2008). Cranial kinesis in dinosaurs: intracranial joints, protractor muscles, and their significance for cranial evolution and function in diapsids. J. Verteb. Paleont. 28, 1073–1088. doi: 10.1671/0272-4634-28.4.1073
Hotton, N., Olson, E. C., and Beerbower, R. (1997). “The Amniote Transition and the Discovery of Herbivory,” in Amniote Origins – Completing the Transition to Land, eds S. Sumida and K. L. M. Martin (San Diego: Academic Press), 207–264. doi: 10.1016/b978-012676460-4/50008-1
Iordansky, N. N. (1990). Evolution of cranial kinesis in lower tetrapods. Netherl. J. Zool. 40, 32–54. doi: 10.1163/156854289x00174
Iordansky, N. N. (2000). Cranial kinesis in the Amphibia: a review. Zhurnal Obshchei Biologii 61, 102–118.
Iordansky, N. N. (2004). The jaw apparatus of Lialis (Lacertilia, Pygopodidae): patterns of intensification of cranial kinesis and the problem of the origin of snakes. Entomol. Rev. 84, (Suppl. 1), S99–S109.
Iordansky, N. N. (2010). Pterygoideus muscles and other jaw adductors in amphibians and reptiles. Biol. Bull. 37, 905–914. doi: 10.1134/S1062359010090050
Iordansky, N. N. (2011). Cranial kinesis in lizards (Lacertilia): origin, biomechanics, and evolution. Biol. Bull. 38, 868–877. doi: 10.1134/S1062359011090032
Iordansky, N. N. (2015). Streptostyly and biological coordinations in the jaw apparatus of lizards (Lacertilia). Biol. Bull. 42, 784–792. doi: 10.1134/S1062359015090046
Irisarri, I., Baurain, D., Brinkmann, H., Delsuc, F., Sire, J.-Y., Kupfer, A., et al. (2017). Phylotranscriptomic consolidation of the jawed vertebrate timetree. Nat. Ecol. Evol. 1, 1370–1378. doi: 10.1038/s41559-017-0240-5
Johnston, P. (2010). The constrictor dorsalis musculature and basipterygoid articulation in Sphenodon. J. Morph. 271, 280–292. doi: 10.1002/jmor.10797
Jones, M. E. H., Curtis, N., Fagan, M. J., O′Higgins, P., and Evans, S. E. (2011). Hard tissue anatomy of the cranial joints in Sphenodon (Rhynchocephalia): sutures, kinesis, and skull mechanics. Palaeontol. Electr. 14, 1–92.
Jones, M. E. H., Curtis, N., O′Higgins, P., Fagan, M., and Evans, S. E. (2009). The head and neck muscles associated with feeding in Sphenodon (Reptilia: Lepidosauria: Rhynchocephalia). Palaeontol. Electr. 12, 1–56.
Jones, M. E. H., Werneburg, I., Curtis, N., Penrose, R., O′Higgins, P., Fagan, M. J., et al. (2012). The head and neck anatomy of sea turtles (Cryptodira: Chelonioidea) and skull shape in Testudines. PLoS One 7:e47852. doi: 10.1371/journal.pone.0047852
Jones, M. E. H., and Zikmund, T. (2012). A functional interpretation of the cranial suture morphology in Captorhinus aguti (Reptilia). J. Paleont. 32:118. doi: 10.1080/02724634.2012.10635175
Kammerer, C. F. (2011). Systematics of the Anteosauria (Therapsida: Dinocephalia). J. Syst. Palaeont. 9, 261–304. doi: 10.1080/14772019.2010.492645
Kathe, W. (1995). Morphology and function of the sutures in the dermal skull roof of Discosauriscus austriacus Makowsky, 1876 (Seymouriamorpha; Lower Permian of Moravia) and Onchiodon labyrinthicus Geinitz, 1861 (Temnospondyli; Lower Permian of Germany). Geobios 19, 255–261. doi: 10.1016/S0016-6995(95)80123-5
Kathe, W. (1999). Comparative morphology and functional interpretation of the sutures in the dermal skull roof of temnospondyl amphibians. Zool. J. Lin. Soc. 126, 1–39. doi: 10.1111/j.1096-3642.1999.tb00605.x
Kilias, R. (1957). Die funktionell-anatomische und systematische Bedeutung der Schläfenreduktionen bei Schildkröten. Mitteilungen aus dem Museum für Naturkunde in Berlin 33, 307–354. doi: 10.1002/mmnz.19570330203
Kissel, R. A. (2010). Morphology, Phylogeny, and Evolution of Diadectidae (Cotylosauria: Diadectomorpha). Dissertation. Toronto: University of Toronto.
Kissel, R. A., Dilkes, D. W., and Reisz, R. R. (2002). Captorhinus magnus, a new captorhinid (Amniota: Eureptilia) from the Lower Permian of Oklahoma, with new evidence on the homology of the astragalus. Can. J. Earth Sci. 39, 1363–1372. doi: 10.1139/e02-040
Kleinteich, T., and Haas, A. (2011). The hyal and ventral branchial muscles in caecilian and salamander larvae: Homologies and evolution. J. Morphol. 272, 598–613. doi: 10.1002/jmor.10940
Klembara, J. (1994). The sutural pattern of skull-roof bones in Lower Permian Discosauriscus austriacus from Moravia. Lethaia 27, 85–95. doi: 10.1111/j.1502-3931.1994.tb01560.x
Klembara, J., Clack, J. A., Milner, A. R., and Ruta, M. (2014). Cranial anatomy, ontogeny, and relationships of the Late Carboniferous tetrapod Gephyrostegus bohemicus Jaekel, 1902. J. Verteb. Paleont. 34, 774–792. doi: 10.1080/02724634.2014.837055
Klembara, J., Hain, M., Ruta, M., Berman, D. S., Pierce, S. E., and Henrici, A. C. (2019). Inner ear morphology of diadectomorphs and seymouriamorphs (Tetrapoda) uncovered by high-resolution X-ray microcomputed tomography, and the origin of the amniote crown group. Palaeontology 63, 131–154. doi: 10.1111/pala.12448
Koyabu, D., Maier, W., and Sánchez-Villagra, M. R. (2012). Paleontological and developmental evidence resolve the homology and dual embryonic origin of a mammalian skull bone, the interparietal. Proc. Natl. Acad. Sci. U S A 109, 14075–14080. doi: 10.1073/pnas.1208693109
Koyabu, D., Werneburg, I., Morimoto, N., Zollikofer, C. P. E., Forasiepi, A. M., Endo, H., et al. (2014). Mammalian skull heterochrony reveals modular evolution and a link between cranial development and brain size. Nat. Comm. 5, 1–9. doi: 10.1038/ncomms4625
Kuhn-Schnyder, E. (1980). “Observations on Temporal Openings of Reptilian Skulls and the Classification of Reptiles,” in Aspects of Vertebrate History, Essays in Honor of Edwin Harris Colbert, ed. L. L. Jacobs (Flagstaff: Museum of Northern Arizona), 153–175.
Lakjer, T. (1926). Studien über die Trigeminus-versorgte Kaumuskulatur der Sauropsiden. Kopenhagen: CA Reitzel, Buchandlung.
Lauder, G. V., and Gillis, G. B. (1997). “Origin of the amniote feeding mechanism: experimental analyses of outgroup clades,” in Amniote Origins - Completing the Transition to Land, eds S. Sumida and K. L. M. Martin (San Diego: Academic Press), 169–206. doi: 10.1016/b978-012676460-4/50007-x
Laurin, M. (2010). How Vertebrates Left the Water. Berkeley: University of California Press, doi: 10.1525/9780520947986
Laurin, M., and Piñeiro, G. H. (2017). A reassessment of the taxonomic position of mesosaurs, and a surprising phylogeny of early amniotes. Front. Earth Sci. 5, 1–13. doi: 10.3389/feart.2017.00088
Lebrun, R. (2018). “MorphoDig, an open-source 3D freeware dedicated to biology,” in The 5th International Palaeontological Congress, abstract volume, (Paris).
Li, C., Fraser, N. C., Rieppel, O., and Wu, X.-C. (2018). A Triassic stem turtle with an edentulous beak. Nature 560, 476–479. doi: 10.1038/s41586-018-0419-1
Lichtig, A. J., and Lucas, S. G. (2021). Chinlechelys from the Upper Triassic of New Mexico, USA, and the origin of turtles. Palaeont. Electr. 24, 1–49. doi: 10.26879/886
Lucas, S. G., Rinehart, L. F., and Celeskey, M. D. (2018). The oldest specialized tetrapod herbivore: a new eupelycosaur from the Permian of New Mexico, USA. Palaeontol. Electr. 21, 1–42. doi: 10.26879/899
Luther, A. (1914). Über die vom N. trigeminus versorgte Muskulatur der Amphibien, mit einem vergleichenden Ausblick über den Adductor mandibulae der Gnathostomen, und einem Beitrag zum Verständnis der Organisation der Anurenlarven. Acta Societ. Sci. Fennicae 7, 1–151.
Lyson, T. R., Sperling, E. A., Heimberg, A. M., Gauthier, J. A., King, B. L., and Peterson, K. J. (2012). MicroRNAs support a turtle + lizard clade. Biol. Lett. 8, 104–107. doi: 10.1098/rsbl.2011.0477
MacDougall, M. J., Modesto, S. P., Brocklehurst, N., Verrière, A., Reisz, R. R., and Fröbisch, J. (2018). Commentary: a reassessment of the taxonomic position of mesosaurs, and a surprising phylogeny of early amniotes. Front. Earth Sci. 6, 1–5. doi: 10.3389/feart.2018.00099
MacDougall, M. J., and Reisz, R. R. (2014). The first record of a nyctiphruretid parareptile from the Early Permian of North America, with a discussion of parareptilian temporal fenestration. Zoolog. J. Linn. So. 172, 616–630. doi: 10.1111/zoj.12180
Maier, W. (1993). “Cranial Morphology of the Therian Common Ancestor, as Suggested by the Adaptation of Neonate Marsupials,” in Mesozoic Differentiation, Multituberculates, Monotremes and Early Marsupials, eds F. S. Szalay, M. J. Novacek, and M. C. McKenna (New York, NY: Springer Verlag), 165–181. doi: 10.1007/978-1-4613-9249-1_12
Maier, W. (1999). On the evolutionary biology of early mammals – with methodological remarks on the interaction between ontogenetic adaptation and phylogenetic transformation. Zoologischer Anzeiger 238, 55–74.
Mann, A., Calthorpe, A. S., and Maddin, H. C. (2021). Joermungandr bolti, an exceptionally preserved ‘microsaur’ from the Mazon Creek Lagerstätte reveals patterns of integumentary evolution in Recumbirostra. R. Soc. Open Sci. 8:210319. doi: 10.1098/rsos.210319
Mann, A., Pardo, J. D., and Maddin, H. C. (2019). Infernovenator steenae, a new serpentine recumbirostran from the ‘Mazon Creek’ Lagerstätte further clarifies lysorophian origins. Zoolog. J. Linn. Soc. 187, 506–517. doi: 10.1093/zoolinnean/zlz130
Markey, M. J., Main, R. P., and Marshall, C. D. (2006). In vivo cranial suture function and suture morphology in the extant fish Polypterus: implications for inferring skull function in living and fossil fish. J. Exp. Biol. 209, 2085–2102. doi: 10.1242/jeb.02266
Metzger, K. (2002). “Cranial Kinesis in Lepidosaurs: Skulls in Motion,” in Topics in Functional and Ecological Vertebrate Morphology, eds P. Aerts, K. D′Août, A. Herrel, and R. Van Damme (Düren: Shaker Publishing), 15–46.
Mezzasalma, M., Maio, N., and Guarino, F. M. (2014). To move or not to move: cranial joints in European gekkotans and lacertids, an osteological and histological perspective. Anat. Rec. 297, 463–472. doi: 10.1002/ar.22827
Moazen, M., Curtis, N., O′Higgins, P., Jones, M. E. H., Evans, S. E., and Fagan, M. (2009). Assessment of the role of sutures in a lizard skull: a computer modelling study. Proc. R. Soc. B 276, 39–46. doi: 10.1098/rspb.2008.0863
Modesto, S. P. (1995). The skull of the herbivorous synapsid Edaphosaurus boanerges from the Lower Permian of Texas. Palaeontology 38, 213–239.
Modesto, S. P. (1998). New information on the skull of the Early Permian reptile Captorhinus aguti. Paleobios 18, 21–35.
Modesto, S. P. (2006). The cranial skeleton of the Early Permian aquatic reptile Mesosaurus tenuidens: implications for relationships and palaeobiology. Zool. J. Linn. Soc. 146, 345–368. doi: 10.1111/j.1096-3642.2006.00205.x
Modesto, S. P., and Anderson, J. S. (2004). The phylogenetic defintion of Reptilia. System. Biol. 53, 815–821. doi: 10.1080/10635150490503026
Modesto, S. P., and Reisz, R. R. (1990). A new skeleton of Ianthasaurus hardestii, a primitive edaphosaur (Synapsida: Pelycosauria) from the Upper Pennsylvanian of Kansas. Can. J. Earth Sci. 27, 834–844. doi: 10.1139/e90-086
Modesto, S. P., Scott, D. M., Berman, D. S., Müller, J., and Reisz, R. R. (2007). The skull and palaeoecological significance of Labidosaurus hamatus, a captorhinid reptile from the Lower Permian of Texas. Zoolog. J. Linn. Soc. 149, 237–262. doi: 10.1111/j.1096-3642.2007.00242.x
Moss, M. L. (1957). Experimental alteration of sutural area morphology. Anat. Rec. 127, 569–589. doi: 10.1002/ar.1091270307
Müller, J., and Reisz, R. R. (2005). An early captorhinid reptile (Amniota, Eureptilia) from the Upper Carboniferous of Hamilton, Kansas. J. Verteb. Paleont. 25, 561–568. doi: 10.1671/0272-46342005025[0561:AECRAE]2.0.CO;2
Müller, J., and Reisz, R. R. (2006). The phylogeny of early eureptiles: comparing parsimony and Bayesian approaches in the investigation of a basal fossil clade. Syst. Biol. 55, 503–511. doi: 10.1080/10635150600755396
Natchev, N., Handschuh, S., Lukanov, S., Tzankov, N., Naumov, B., and Werneburg, I. (2016). Contributions to the functional morphology of caudate skulls: kinetic and akinetic forms. PeerJ 4, 1–15. doi: 10.7717/peerj.2392
Norman, D. B., and Weishampel, D. B. (1985). Ornithopod feeding mechanisms: their bearing on the evolution of herbivory. Am. Natur. 126, 154–164. doi: 10.1086/284406
Nyakatura, J. A., Melo, K., Horvat, T., Karakasiliotis, K., Allen, V. R., Andikfar, A., et al. (2019). Reverse-engineering the locomotion of a stem amniote. Nature 565, 351–355. doi: 10.1038/s41586-018-0851-2
Olson, E. C. (1961). Jaw mechanisms: rhipidistians, amphibians, reptiles. Am. Zool. 1, 205–215. doi: 10.1093/icb/1.2.205
Packard, M. J., and Seymour, R. S. (1997). “Evolution of the amniote egg,” in Amniote Origins - Completing the Transition to Land, eds S. S. Sumida and K. L. M. Martin (San Diego: Academic Press), 265–290. doi: 10.1016/b978-012676460-4/50009-3
Paluh, D. J., Stanley, E. L., and Blackburn, D. C. (2020). Evolution of hyperossification expands skull diversity in frogs. Proc. Natl. Acad. Sci. U S A 117, 8554–8562. doi: 10.1073/pnas.2000872117
Panchen, A. L. (1964). The cranial anatomy of two Coal Measure anthracosaurs. Philosoph. Trans. R. Soc. 247, 593–637. doi: 10.1098/rstb.1964.0006
Panchen, A. L. (1977). On Anthracosaurus russelli Huxley (Amphibia: Labyrinthodontia) and the family Anthracosauridae. Philosoph. Trans. R. Soc. Lond. 279, 447–512. doi: 10.1098/rstb.1977.0096
Payne, S. L., Holliday, C. M., and Vickaryous, M. K. (2011). An osteological and histological investigation of cranial joints in geckos. Anat. Rec. 294, 399–405. doi: 10.1002/ar.21329
Poglayen-Neuwall, I. (1953). Untersuchungen der Kiefermuskulatur und deren Innervation bei Schildkröten. Acta Zool. 34, 241–292. doi: 10.1111/j.1463-6395.1953.tb00472.x
Porro, L. B., Rayfield, E. J., and Clack, J. A. (2015). Descriptive anatomy and three-dimensional reconstruction of the skull of the early tetrapod Acanthostega gunnari Jarvik, 1952. PLoS One 10:e0118882. doi: 10.1371/journal.pone.0118882
Price, L. I. (1935). Notes on the brain case of Captorhinus. Proc. Bost. Soc. Natur. Hist. 40, 377–386.
Rafferty, K. L., and Herring, S. W. (1999). Craniofacial sutures: morphology, growth, and in vivo masticatory strains. J. Morphol. 242, 167–179. doi: 10.1002/(SICI)1097-4687(199911)242:2<167::AID-JMOR8<3.0.CO;2-1
Rafferty, K. L., Herring, S. W., and Artese, F. (2000). Three-dimensional loading and growth of the zygomatic arch. J. Exp. Biol. 203, 2093–3004. doi: 10.1242/jeb.203.14.2093
Rawson, J. R. G., Porro, L. B., Martin-Silverstone, E., and Rayfield, E. J. (2021). Osteology and digital reconstruction of the skull of the early tetrapod Whatcheeria deltae. J. Vert. Paleontol. 2021, 1–16. doi: 10.1080/02724634.2021.1927749
Rayfield, E. J. (2005). Using finite-element analysis to investigate suture morphology: a case study using large carnivorous dinosaurs. Anat. Rec. 283, 349–365. doi: 10.1002/ar.a.20168
Reisz, R. R., and Heaton, M. J. (1980). Matters arising: origin of mammal-like reptiles. Nature 288:193. doi: 10.1038/288193b0
Rieppel, O. (1987). The development of the trigeminal jaw adductor musculature and associated skull elements in the lizard Podarcis sicula. J. Zool. 212, 131–150. doi: 10.1111/j.1469-7998.1987.tb05120.x
Rieppel, O., and deBraga, M. (1996). Turtles as diapsid reptiles. Nature 384, 453–455. doi: 10.1038/384453a0
Romer, A. S. (1927). The pelvic musculature of ornithischian dinosaurs. Acta Zool. 8, 225–275. doi: 10.1111/j.1463-6395.1927.tb00653.x
Romer, A. S. (1956). A Typical Reptilian Skull Pattern – the Captorhinomorph Skull in Osteology of the Reptiles. Malabar: Krieger Publishing Company, 69–73.
Romer, A. S., and Price, L. I. (1940). Review of the Pelycosauria. Special Paper Geol. Soc. Am. 28, 1–538. doi: 10.1130/spe28-p1
Schoch, R. R. (2014). Amphibian skull evolution: The developmental and functional context of simplification, bone loss and heterotopy. Journal of Experimental Zoology (Molecular and Dev. Evol. 322B, 619–630. doi: 10.1002/jez.b.22599
Schoch, R. R., and Sues, H.-D. (2015). A Middle Triassic stem-turtle and the evolution of the turtle body plan. Nature 523, 584–587. doi: 10.1038/nature14472
Schumacher, G. (1973). “The Head Muscles and Hyolaryngeal Skeleton of Turtles and Crocodilians,” in Biology of the Reptilia, eds C. Gans and T. S. Parsons (New York, NY: Academic Press), 101–199.
Sues, H.-D., and Reisz, R. R. (1998). Origins and early evolution of herbivory in tetrapods. Trends Ecol. Evol. 13, 141–145. doi: 10.1016/S0169-5347(97)01257-3
Sumida, S. S. (1997). “Locomotor features of taxa spanning the origin of amniotes,” in Amniote Origins - Completing the Transition to Land, eds S. Sumida and K. L. M. Martin (San Diego: Academic Press), 353–398. doi: 10.1016/b978-012676460-4/50012-3
Sushkin, P. P. (1928). Contributions to the cranial morphology of Captorhinus Cope (Reptilia, Cotylosauria, Captorhinidae). Palaeobiologica 1, 263–280.
Tarsitano, S. F., Oelofsen, B., Frey, E., and Riess, J. (2001). The origin of temporal fenestrae. South Afr. J. Sci. 97, 334–336.
Thomson, K. S. (1967). Mechanisms of intracranial kinetics in fossil rhipidistian fishes (Crossopterygii) and their relatives. Zool. J. Linn. Soc. 46, 223–253. doi: 10.1111/j.1096-3642.1967.tb00505.x
Versluys, J. (1910). Streptostylie bei Dinosauriern, nebst Bemerkungen über die Verwandtschaft der Vögel und Dinosaurier. Zoologische Jahrbücher, Abteilung für Anatomie und Ontogenie der Tiere 30, 177–260.
Versluys, J. (1912). Das Streptostylie-Problem und die Bewegungen im Schädel der Sauropsiden. Zoologische Jahrbücher 15(Suppl. pt. 2), 545–702.
Versluys, J. (1919). Über die Phylogenie der Schläfengruben und Jochbogen bei den Reptilia. Sitzungsberichte der Heidelberger Akademie der Wissenschaften, Stiftung Heinrich Lanz, Mathematisch-naturwissenschaftliche Klasse Abteilung B. Biologische Wissenschaften 13, 3–29.
Warren, J. W. (1961). The basicranial articulation of the Early Permian cotylosaur, Captorhinus. J. Paleont. 35, 561–563.
Werneburg, I. (2012). Temporal bone arrangements in turtles: an overview. J. Exp. Zool. 318, 235–249. doi: 10.1002/jez.b.22450
Werneburg, I. (2013a). Jaw musculature during the dawn of turtle evolution. Org. Div. Evol. 13, 225–254. doi: 10.1007/s13127-012-0103-5
Werneburg, I. (2013b). The tendinous framework in the temporal skull region of turtles and considerations about its morphological implications in amniotes: a review. Zool. Sci. 30, 141–153. doi: 10.2108/zsj.30.141
Werneburg, I. (2015). Neck motion in turtles and its relation to the shape of the temporal skull region. Comptes Rendus Palevol 14, 527–548. doi: 10.1016/j.crpv.2015.01.007
Werneburg, I. (2019). Morphofunctional categories and ontogenetic origin of temporal skull openings in amniotes. Front. Earth Sci. 7, 1–7. doi: 10.3389/feart.2019.00013
Werneburg, I., and Abel, P. (2022). Modeling skull network integrity at the dawn of amniote diversification with considerations on functional morphology and fossil jaw muscle reconstructions. Front. Ecol. Evol. 9:799637. doi: 10.3389/fevo.2021.799637
Werneburg, I., Esteve-Altava, B., Bruno, J., Torres Ladeira, M., and Diogo, R. (2019). Unique skull network complexity of Tyrannosaurus rex among land vertebrates. Scient. Rep. 9:1520. doi: 10.1038/s41598-018-37976-8
Werneburg, I., and Maier, W. (2019). Diverging development of akinetic skulls in cryptodire and pleurodire turtles: an ontogenetic and phylogenetic study. Verteb. Zool. 69, 113–143. doi: 10.26049/VZ69-2-2019-01
Werneburg, I., Wilson, L. A. B., Parr, W. C. H., and Joyce, W. G. (2015). Evolution of neck vertebral shape and neck retraction at the transition to modern turtles: an integrated geometric morphometric approach. Syst. Biol. 64, 187–204. doi: 10.1093/sysbio/syu072
Wilken, A. T., Middleton, K. M., Sellers, K. C., Cost, I. N., and Holliday, C. M. (2019). The roles of joint tissues and jaw muscles in palatal biomechanics of the savannah monitor (Varanus exanthematicus) and their significance for cranial kinesis. J. Exp. Biol. 222:jeb201459. doi: 10.1242/jeb.201459
Witzmann, F., and Werneburg, I. (2017). The palatal interpterygoid vacuities of temnospondyls and the implications for the associated eye-and jaw musculature. Anat. Rec. 300, 1240–1269. doi: 10.1002/ar.23582
Woodhead, J., Reisz, R. R., Fox, D., Drysdale, R., Hellstrom, J., Maas, R., et al. (2010). Speleothem climate records from deep time? Exploring the potential with an example from the Permian. Geology 38, 455–458. doi: 10.1130/G30354.1
Yaryhin, O., and Werneburg, I. (2019). The origin of orbitotemporal diversity in lepidosaurs: insights from tuatara chondrocranial anatomy. Verteb. Zool. 69, 169–181. doi: 10.26049/VZ69-2-2019-04
Zdansky, O. (1923–1925). Über die Temporalregion des Schildkrötenschädels. Bull. Geolog. Instit. Univ. Upasala 19, 89–114.
Ziermann, J. M. (2019). “Diversity of Heads, Jaws, and Cephalic Muscles in Amphibians,” in Heads, Jaws, and Muscles - Anatomical, Functional, and Developmental Diversity in Chordate Evolution, eds J. M. Ziermann, R. E. Diaz, and Jr. R. Diogo (New York, NY: Springer), 143–170. doi: 10.1007/978-3-319-93560-7_7
Keywords: Amniota, Captorhinidae, biomechanics, functional morphology, cranial osteology, temporal openings
Citation: Abel P, Pommery Y, Ford DP, Koyabu D and Werneburg I (2022) Skull Sutures and Cranial Mechanics in the Permian Reptile Captorhinus aguti and the Evolution of the Temporal Region in Early Amniotes. Front. Ecol. Evol. 10:841784. doi: 10.3389/fevo.2022.841784
Received: 22 December 2021; Accepted: 13 April 2022;
Published: 18 May 2022.
Edited by:
Haijun Song, China University of Geosciences Wuhan, ChinaReviewed by:
David Marjanović, Museum of Natural History Berlin (MfN), GermanyCopyright © 2022 Abel, Pommery, Ford, Koyabu and Werneburg. This is an open-access article distributed under the terms of the Creative Commons Attribution License (CC BY). The use, distribution or reproduction in other forums is permitted, provided the original author(s) and the copyright owner(s) are credited and that the original publication in this journal is cited, in accordance with accepted academic practice. No use, distribution or reproduction is permitted which does not comply with these terms.
*Correspondence: Ingmar Werneburg, aW5nbWFyLndlcm5lYnVyZ0BzZW5ja2VuYmVyZy5kZQ==
Disclaimer: All claims expressed in this article are solely those of the authors and do not necessarily represent those of their affiliated organizations, or those of the publisher, the editors and the reviewers. Any product that may be evaluated in this article or claim that may be made by its manufacturer is not guaranteed or endorsed by the publisher.
Research integrity at Frontiers
Learn more about the work of our research integrity team to safeguard the quality of each article we publish.