- 1Department of Environment, Parks and Water Security, Northern Territory Government, Darwin, NT, Australia
- 2Complex Systems Consulting, Brisbane, QLD, Australia
Climate change is predicted to have devastating impacts on apex predators such as eliminating their required habitats. Crocodilians are no exception as most species require freshwater for nesting, and such freshwater habitats are particularly vulnerable to saltwater inundation (SWI) caused by the sea level rise (SLR) from global warming. Here, we examined the impacts of climate change on saltwater crocodiles Crocodylus porosus in terms of the potential loss of nesting habitat in the Northern Territory, Australia; an area that contains the world’s most extensive nesting habitat for the species. Our spatial model, derived from 730 nest locations and selected environmental features, estimated a total of 32,306.91 km2 of current suitable habitat across the study region. The most important variable was distance to perennial lakes (71.0% contribution, 87.5% permutation importance), which is negatively correlated with nesting habitat suitability. We found that projected changes in temperature and rainfall by 2100 could impact the area of suitable nesting habitat negatively or positively (0.33% decrease under low future emission climate scenario, and 32.30% increase under high emission scenario). Nevertheless, this can be canceled by the strong negative impact of SLR and concomitant SWI on nesting areas. A portion (16.40%) of the modeled suitable habitat for a subsection of our study area, the Kakadu Region, were already subject to > 0.25 m SWI in 2013. The suitable area for nesting in this region is predicted to be further reduced to 1775.70 km2 with 1.1 m SLR predicted for 2100, representing 49.81% loss between 2013 and 2100. Although the estimates of habitat loss do not account for the potential creation of new habitat, nor for the uncertainty in the degree of future SLR, our results suggest that SLR driven by continuing global warming can be the major threat to mound-nest-building crocodilians including C. porosus, rather than direct impacts from changes in temperature and rainfall. The degree of impact on saltwater crocodiles will be determined by the interplay between the loss of nesting habitat, which would appear inevitable under current global warming, and the ability to expand into new areas created by the expansion of the tropics.
Introduction
Apex predators include some of the world’s most imperiled species and climate change is predicted to have devastating impacts on some members of this important trophic group. Perhaps the best publicized example of climate impacts on an apex predator is the polar bear (Ursus maritimus), which undergoes population decline in years with low levels of arctic ice coverage due to reduced reproduction and adult female survivorship (Hunter et al., 2010; Laidre et al., 2020). Projections of continued reductions in arctic ice area indicate that the polar bear will experience severe population declines by 2100 (Hunter et al., 2010; Molnár et al., 2011). Climate change is also predicted to impact tropical apex predators. For example, in the mangrove Sundarbans of southern Bangladesh, sea-level rise is predicted to eliminate all suitable habitat for the Bengal tiger (Panthera tigris tigris) by 2070 (Mukul et al., 2019). Climate change impacts are also likely to extend to reptile apex predators, including the world’s largest lizard, the Komodo dragon (Varanus komodoensis). The Komodo dragon occurs on five islands in Indonesia and climate change under moderate emissions scenarios is predicted to reduce suitable habitat by ∼90% by 2050 (Jones et al., 2020). From these examples, it is apparent that climate change will impact apex predators via several pathways and across multiple biomes.
Crocodilians, with 25 extant species currently recognized, function as a crucial apex predator in semiaquatic ecosystems, and are also anticipated to be negatively impacted by climate change. For example, 37-year observations in Florida showed that Crocodylus acutus hatching shifted to earlier dates by 1.5 days, every 2 years with increased sea surface temperature (Cherkiss et al., 2020). Similar results show that increased temperature led to shorter incubation periods for the same species in Mexico (Charruau et al., 2017). Moreover, the sex ratio is determined, in crocodilian species, by incubation temperature, and climate warming is expected to interfere with this (Maciejewski, 2006; González et al., 2019; Bock et al., 2020). For Alligator mississippiensis in Florida, it is estimated that a temperature rise by 1.1–1.4°C in 2040–2050 may skew the sex ratio initially to 95.6% males and then to 97.8% females with a temperature rise by 1.6–3.2°C in 2090–2100 (Bock et al., 2020).
Global warming also can be a threat to crocodilians by destroying their habitat, in particular freshwater swamps or floodplains, through saltwater inundation (SWI) as a result of the sea level rise (SLR). Most species require freshwater habitat for breeding and nesting and such areas typically lie at a low elevation along coasts or rivers and are, therefore, vulnerable to imminent SWI (Pezeshki et al., 1990; Mulrennan and Woodroffe, 1998; Pettit et al., 2018). Despite the adaptation to the saline environment, as implied by their common name, saltwater crocodiles, C. porosus is one such species and requires constant or regular access to freshwater for breeding.
Here, we examine the impacts of climate change on C. porosus, the largest extant crocodilian species, and quantify the potential loss of nesting habitat through SWI and SLR in the Northern Territory (NT), Australia. This region is of global significance, as it supports the most extensive freshwater wetlands and floodplains and contains the largest population of this apex predator in the world (Webb et al., 2010; Fukuda et al., 2021).
Materials and Methods
Study Area
The study area is the northern coastal region of the NT, Australia, within the latitude range −11.0 and −17.0°, called the Top End (Figure 1). The Top End includes the Kakadu Region, which largely consists of the Kakadu National Park. Four major tidal rivers (East, South, West Alligator Rivers and Wildman River) feed into extensive freshwater floodplains contained within the Kakadu National Park. The climate is tropical and monsoonal with distinct dry (May–October) and wet (November–April) seasons. In the coastal areas of the study region, the daily rainfall can exceed 200 mm, and averages approximately 25 mm daily at the peak wet season (Bureau of Meteorology, 2020). The annual rainfall typically ranges between 1,500 and 2,000 mm. The mean maximum and minimum monthly ambient temperature is approximately 17 and 34°C, respectively.
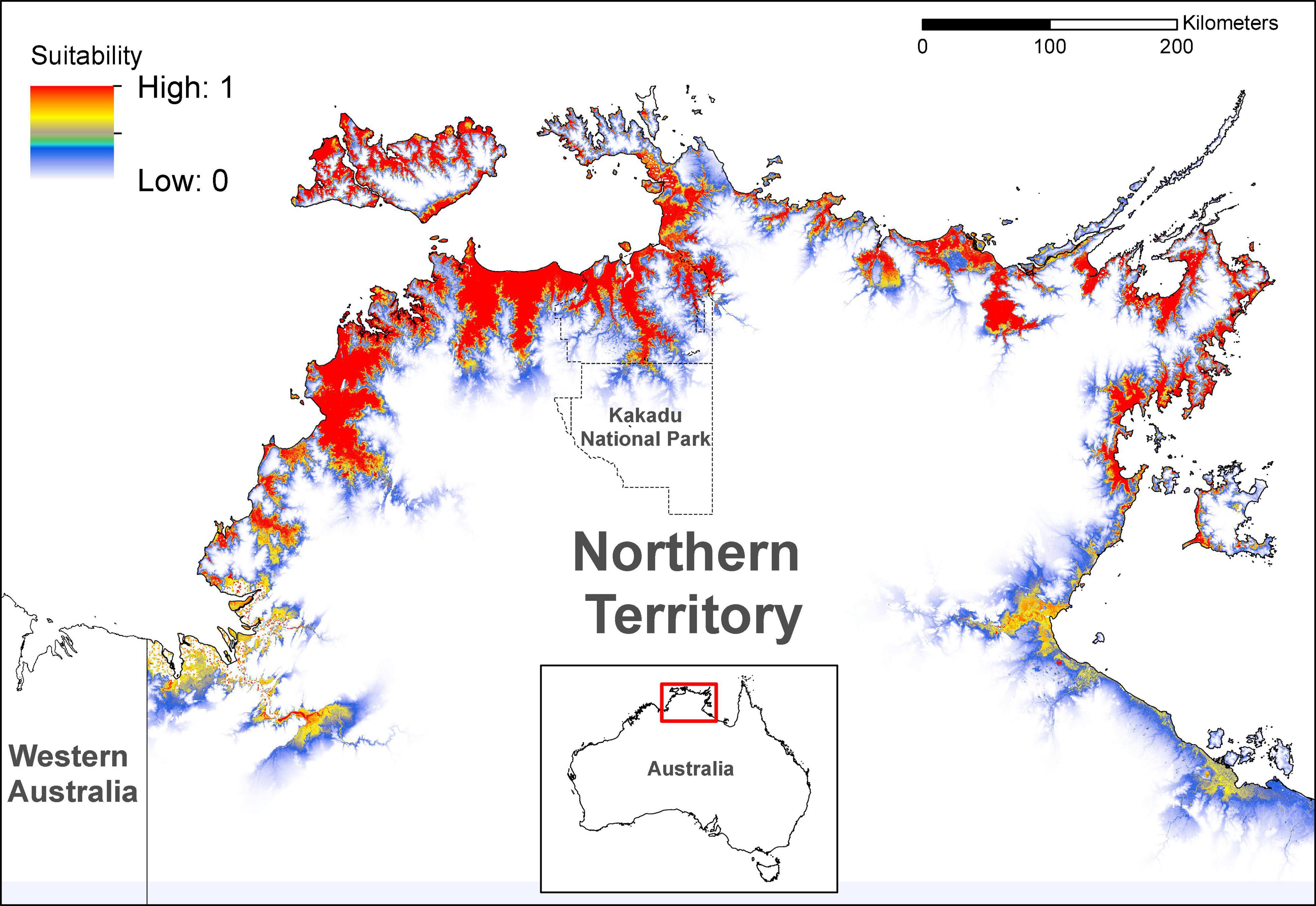
Figure 1. Habitat suitability for nesting of Crocodylus porosus, predicted by the non-thresholded Maxent model incorporating eight environmental variables (Table 1) in the study area of the Top End, the NT, Australia.
Study Species
C. porosus is the largest extant crocodilian species, with the largest individuals exceeding a total length of 6 m and weighing over 1,000 kg (Britton et al., 2012). The species is physiologically adapted to both the freshwater and saline environment (Grigg et al., 1980; Grigg, 1981; Taplin and Grigg, 1981) although they require freshwater for breeding (Webb et al., 1977, 1983). They are found in many different waterbodies including beaches, estuaries, lakes, rivers, and swamps. Some individuals have been reported in the sea far from the shore (Brackhane et al., 2018; Spennemann, 2021). Females build a mound-like nest from vegetation such as tall grasses in the freshwater floodplains or swamps and lay typically 40–55 eggs inside the nest (Webb et al., 1977, 1983; Fukuda and Cuff, 2013). Eggs are incubated by the heat generated by the decomposition of the vegetation material and hatch after approximately 75–95 days (Webb et al., 1983; Richardson et al., 2002). Their breeding is annual and highly seasonal, and is restricted to the wet season.
The species was heavily hunted for commercial use between the 1940s and 1960s, but since legislative protection in 1971, has substantially recovered (Fukuda et al., 2011). They are not considered threatened at any level in the NT and are categorized as Least Concern under the Territory Parks and Wildlife Conservation Act 1976. The Australian population is listed in Appendix II under the Convention on the International Trade in Endangered Species (CITES, IUCN, 2012). As part of the sustainable harvest program implemented in the NT, up to 70,000 eggs are collected annually across the study area for the commercial ranching program (Saalfeld et al., 2015, 2016).
Nesting Habitat Modeling
We used the software package Maxent version 3.4.4 (Phillips et al., 2021) to estimate the current and future areas of suitability for saltwater crocodile nesting in the Top End. Maxent is a presence-only model that minimizes the relative entropy of estimated probability densities between presence points and the background landscape (Elith et al., 2011) and has frequently outperformed other distribution modeling techniques (Hernandez et al., 2006; Wisz et al., 2008).
For the model of suitable nesting habitat at the present time, we collated 730 individual nest locations from across the study area. The crocodile nests were located and harvested by multiple commercial operators during the wet season in 2019 (November–December) and 2020 (January–May) as part of the sustainable harvest program (Saalfeld et al., 2015). We started with an in initial list of 15 environmental variables anticipated to limit saltwater crocodile nesting habitat based on the literature (Table 1). We converted all vector topographic variables to rasters, matching the grain size of a 3 s (∼90 m) digital elevation model (DEM), and masked existing raster layers to the same grain size as the DEM, in ArcGIS version 10.6.1 (esri, 2021). We screened variables for collinearity using the correlation matrix in ArcMap, and excluded one of variables in a pair with a correlation coefficient of ≥ 0.7 (Merow et al., 2013). This left us with the following eight variables: (1) “elevation,” 3 s (∼90 m) digital elevation model; three climate variables from WorldClim downloaded at 30 s resolution including (2) “BIO06,” minimum temperature of coldest month; (3) “BIO15,” precipitation seasonality (coefficient of variation); and (4) “early wet season rainfall,” October–December rainfall (Booth et al., 2014; WorldClim, 2020); and five variables from a Northern Territory 1:250,000 topographic map (Geoscience Australia, 2006), including (5) “floodplain,” distance to land subject to inundation; (6) “perennial lakes,” distance to perennial lakes; (7) “perennial streams,” distance to perennial watercourse; and (8) “freshwater swamp,” categorical value of freshwater swamp (1) vs. other landform type (0) (Table 1). We made this variable categorical because, according to the definitions by Geoscience Australia (2006), the freshwater swamps are small and sparsely distributed in some catchments across the study area. We fitted a nesting habitat suitability model in Maxent with only the linear relationships feature option selected (Merow et al., 2013) and retained 20% of records for validation testing. In addition to the standard cloglog suitability raster output, which we interpreted as relative nesting habitat suitability (Phillips and Dudík, 2008), we also selected a threshold (Maximum training sensitivity plus specificity; Bean et al., 2012) to assign areas of the study area as suitable vs. unsuitable based on the fitted model. To predict suitable nesting habitat in the future, we applied a projection in Maxent using the WorldClim future weather climate projections for the three climate variables. We selected the period 2081–2100 using BCC-CSM2-MR, a medium-resolution global climate model developed by Wu et al. (2019) under two climate scenarios based on low Shared Socio-economic Pathways (SSP 126) and high (SSP 585) future emissions (Hausfather, 2018; WorldClim, 2020).
Sea Level-Rise in Kakadu
Detailed tidally driven, hydrodynamic models of SWI driven by SLR have previously been developed for the Kakadu region (Bayliss et al., 2016), enabling us to make detailed predictions for changes in habitat suitability in this important region. Our thresholded Maxent model produced a raster with binary nesting suitability (0 for unsuitable and 1 for suitable). We overlaid this raster of the thresholded model with the raster datasets of the coastal and river freshwater floodplains simulated by Bayliss et al. (2016), using ArcGIS version 10.6.1. The sea level around the Australian coastlines is expected to rise in a range of 0.75–1.90 m with the mid-range value of 1.1 by 2100 (Short and Woodroffe, 2009). We used the SWI simulation with 1.1 m SLR from Bayliss et al. (2016) to estimate how much of the suitable nesting habitat in the Kakadu Region would be affected by SWI in 2100. Although C. porosus prefers the freshwater environment for nesting, some areas with saline vegetation such as Halosarcia, Tecticornia, and Suaeda are sometimes used because they largely become freshwater in the breeding wet season due to the large input of flushing rainwater during monsoonal events (Fukuda and Cuff, 2013). Thus, we considered that floodplains with less than 0.25 m SWI would remain as habitat suitable for nesting and those areas with SWI of more than 0.25 m are unsuitable. We used the raster predictions from Bayliss et al. (2016) for > 0.25 m SWI to identify areas that will be lost from our thresholded model of current suitable nesting habitat by the year 2100.
Results
Our model of current saltwater crocodile nesting habitat performed well (test AUC of 0.958 ± SD 0.003) and showed suitable areas around the coasts and floodplains of the Top End of the NT (Figure 1). The most important variable was distance to perennial lakes (71.0% contribution, 87.5% permutation importance), with a negative logistic relationship with nesting habitat suitability (Figure 2A). Early wet season rainfall was the next most important variable (12.9% contribution; 0.9% permutation importance), with a positive logistic relationship between Early wet season rainfall and nesting habitat suitability (Figure 2B). The freshwater swamp variable (8.3% contribution; 1.2% permutation importance) showed much higher suitability than other landform types (Figure 2C). Seasonality in rainfall (BIO15) showed 6.6% contribution; 3.0% permutation importance, with a negative logistic relationship to nesting habitat suitability (Figure 2D). All other variables contributed < 5% to the model.
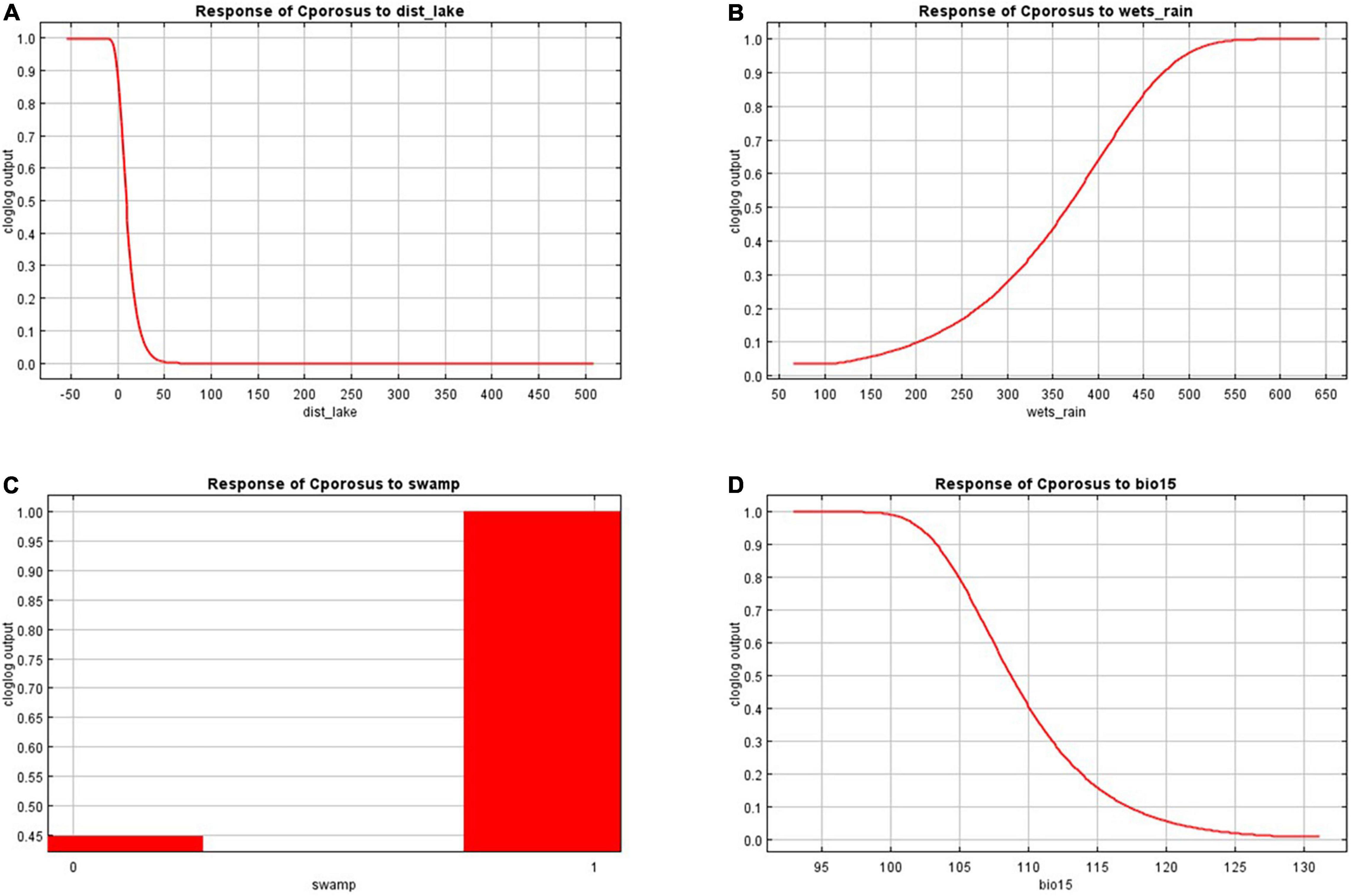
Figure 2. Relationship plots between the nesting suitability and (A) distance to perennial lakes (71.0% contribution, 87.5% permutation importance), (B) early wet season rainfall (12.9% contribution; 0.9% permutation importance), (C) freshwater swamp (8.3% contribution; 1.2% permutation importance), and (D) seasonality in rain (6.6% contribution; 3.0% permutation importance) estimated by Maxent. These plots reflect the dependence of predicted suitability both on the selected variable and on dependencies induced by correlations between the selected variable and other variables (Phillips et al., 2021).
For the whole of the Top End, our thresholded Maxent model predicted a total of 32,306.91 km2 of suitable nesting habitat across the study area for the current time. For the period 2081–2100, using the predicted future temperature and rainfall patterns derived from the BCC-CSM2-MR climate model but retaining the other variables at their present values, our Maxent model predicted an area of suitable habitat of 32,199.32 km2 under the low emissions scenario (SSP 126) and 42,740.78 km2 under the high emissions scenario (SSP 585) across the study area.
Within the Kakadu Region, a total area of 4232.08 km2 was assigned as suitable nesting habitat by our current model (Figure 3A). However, the hydrodynamic models by Bayliss et al. (2016) show that as at 2013 sea level, approximately 16.40% of the suitable habitat was already affected by < 0.25 m SWI, and 3538.10 km2 remains suitable for nesting (Figure 3B). After 1.1 m SLR (that is, sea levels forecast for 2100), only 1775.70 km2 remains suitable (Figure 3C). This represents a 58.04% reduction in predicted suitable nesting habitat over 87 years, between 2013 and 2100).
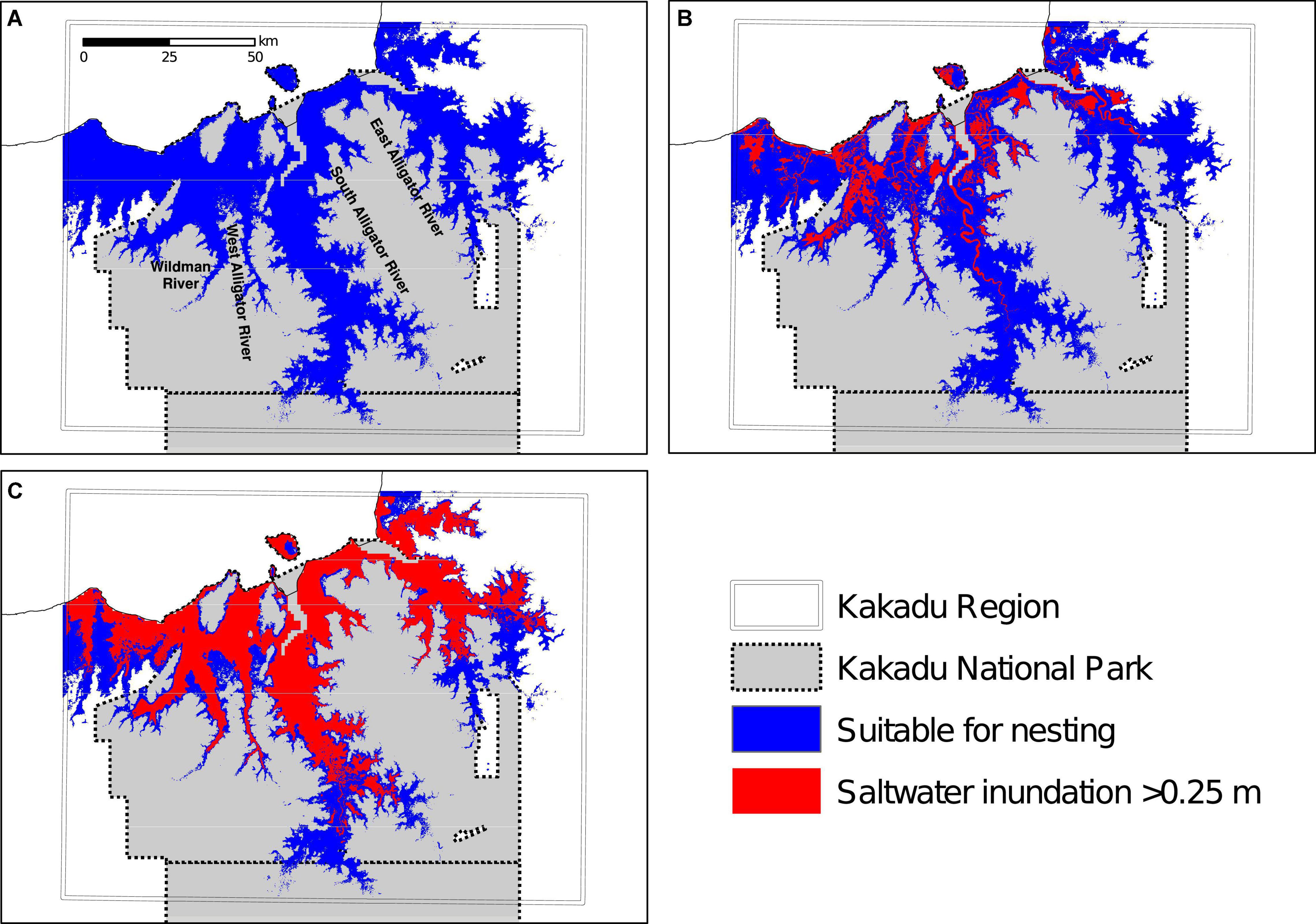
Figure 3. Suitable nesting habitat for Crocodylus porosus in the Kakadu Region of the Northern Territory, predicted by the thresholded Maxent model, with (A) no sea level rise (SLR) and no saltwater inundation (SWI) incorporated, (B) 0 m SLR but excluding areas with > 0.25 m SWI as simulated for 2013 by Bayliss et al. (2016), and (C) 1.1 m SLR and excluding areas with > 0.25 m SWI as simulated for 2100 by Bayliss et al. (2016).
Discussion
Our model of suitable nesting habitat for the saltwater crocodile revealed a dominant importance of abundant, perennial lakes in Australia’s Top End. The estimate of nesting suitability rapidly dropping beyond a 50 m range from perennial lakes (Figure 2A) is consistent with field observations that a vast majority of nests are made around the edges of waterbodies during the breeding season (Webb et al., 1977, 1983; Fukuda and Cuff, 2013). The perennial lakes appear to be important as females guarding their nests during the incubation period, typically 2–3 months, require access to freshwater (Webb et al., 1977; Magnusson, 1980). The model also identified the importance of early rains in the wet season (Figure 2B), supporting previous findings that rains in early wet season (Oct–Dec) trigger the highly seasonal breeding of C. porosus, and higher precipitation during this period results in higher abundance of hatchlings in the following dry season (Fukuda and Saalfeld, 2014).
We found that projected changes in temperature and rainfall under climate change could impact the area of suitable nesting habitat negatively or positively (0.33% decrease under a low emission scenario SSP 126 and 32.30% increase under a high emission scenario SSP 585). It is worth mentioning that, apart from the adverse impact of SLR, the projected nesting habitat would otherwise be increased to some extent under the higher future emission scenario, because of some positive effects on the climate variables such as increased early rains and decreased seasonality. Nevertheless, these effects will be canceled by the much higher, negative impact by SLR.
Most importantly, our analysis for the Kakadu Region showed that almost 50% of the suitable nesting habitat in 2013 would be lost to the 1.1 m SLR by 2100 (Figure 3). While fine-scale SLR data and forecasts are not currently available outside of the Kakadu region, if a similar proportion of the habitat is affected across the larger study area, 16,522 km2 would be lost to SLR across the Top End. This is of significant concern as the majority of the Australian population of C. porosus resides in this area (Fukuda et al., 2007, 2021). This suggests that SLR by climate change may represent the major threat to the species. Other crocodilians that build mound nests in freshwater wetlands or floodplains, such as A. mississippiensis and C. moreletti, could also be at similar risk, as their nests are prone to flooding (Kushlan and Jacobsen, 1990; Platt et al., 2021).
One important caveat to the predicted loss of saltwater crocodile nesting habitat is that the creation of new freshwater habitats in the Kakadu region has not been accounted for. Bayliss et al. (2016) were unable to predict new areas of freshwater habitat created through SLR because the Lidar-derived fine-scale elevation data did not extend beyond the current floodplain. Undoubtedly, SLR will result in the formation of some areas of new habitat suitable for saltwater crocodile nesting which may somewhat offset the areas lost. However, the sandstone escarpments that occur further inland (Northern Territory and Geological Survey, 2006) form a physical barrier to the formation of extensive freshwater floodplains. In addition, while small billabongs or lakes may form on the escarpment, the steep and rocky terrain curtails the dispersal of saltwater crocodiles, and to date, they have never been observed in these areas (Letnic and Connors, 2006). For areas outside of the sandstone escarpments, the potential creation of new floodplain habitat is an important avenue for further research. Additional data such as LiDAR (Dong and Chen, 2017) would facilitate more accurate forecasts of potential future suitable habitat.
Another source of uncertainty is the degree of future SLR. Bayliss et al. (2016) adopted a value of 1.1 m in 2001 based on a range of published projections (Solomon et al., 2007; Jevrejeva et al., 2010). The IPCC recently released their 6th Assessment Report which includes predictions of 0.63–1.01 m by 2100 under the moderate emission scenario and 0.66–1.33 m by 2150 under the very high emissions scenario (Masson-Delmotte et al., 2021). Therefore, the 1.1 m SLR used in our study represents likely SLR between 2100 and 2150. However, several studies document the acceleration of SLR in recent decades (Nerem et al., 2018; Dangendorf et al., 2019), and in addition, the IPCC stated that due to the substantial uncertainty in global ice sheet dynamics, SLR approaching 2 m by 2100 and 5 m by 2150 cannot be ruled out (Masson-Delmotte et al., 2021). The substantial uncertainty in the potential creation of new habitat and the degree of SLR means that estimates of the loss of saltwater crocodile nesting habitat should be recalculated as new data become available.
It should be noted that the change from a freshwater to more saline habitat is not anticipated to be monotonic, and gradual replacement of freshwater plant species with those that are more saline tolerant is the likely scenario. While the floodplain vegetation is determined by fine-scale variation in topography (Finlayson et al., 2006) and the global mean SLR is 4.8 mm annually (Dangendorf et al., 2019), even infrequent SWI can lead to sudden changes in salinity (Finlayson et al., 2006; Bayliss et al., 2016). Freshwater plant taxa have a unique tolerance to salinity and water depth (Cowie, 2003), and most freshwater plant species are unable to tolerate salinity much in excess of 1 ppt (Pettit et al., 2018). Previous work has shown an association between crocodile nesting and Oryza dominated tall tussock and Melaleuca open grassland with an understory comprised of species also present in the Oryza dominated grasslands (Fukuda and Cuff, 2013), and Oryza species are unable to tolerate salinity above 1 ppt (Pettit et al., 2018). In contrast, mangrove species can tolerate salinity to 50 ppt (Ball, 1998), and are not obligate halophiles, that is, they are also able to live in freshwater conditions (Woodroffe, 1988).
Although fossil records and molecular analysis indicate that extant crocodilians were capable of tracking changes in their distribution in response to drastic climate changes in the last 100 million years (Roos et al., 2007; Brochu et al., 2009; Ryberg and Lawing, 2018), whether they can continue to track changes in the spatial distribution and quality of suitable nesting habitat is unknown. Crocodylus porosus is one of the oldest extant species in the world, little changed since dinosaurs roamed the planet, yet their survival during the Anthropocene may now depend on how quickly humanity can transition away from the burning of fossil fuels and thus stabilize global warming.
Data Availability Statement
The original contributions presented in the study are publicly available. This data can be found here: Fukuda and McDonald (2022).
Ethics Statement
Ethical review and approval was not required for the animal study because this study did not involve live animals and only used the location data of crocodile nests that were reported to the Northern Territory Government, Australia.
Author Contributions
YF, PM, and BC contributed to conception and design of the study, and wrote the manuscript. YF organized the data access. YF and PM performed the spatial analysis. All authors contributed to manuscript revision, read, and approved the submitted version.
Funding
Department of Environment, Parks and Water Security provided financial support.
Conflict of Interest
BC was affiliated with Complex Systems Consulting.
The remaining authors declare that the research was conducted in the absence of any commercial or financial relationships that could be construed as a potential conflict of interest.
Publisher’s Note
All claims expressed in this article are solely those of the authors and do not necessarily represent those of their affiliated organizations, or those of the publisher, the editors and the reviewers. Any product that may be evaluated in this article, or claim that may be made by its manufacturer, is not guaranteed or endorsed by the publisher.
Acknowledgments
Crocodile Farmers Association of the Northern Territory provided the location data of the crocodile nests used in the analyses. These location data were collected in accordance to the Code of practice for the humane treatment of wild and farmed Australian crocodiles (Department of the Environment and Energy, 2013) as part of the sustainable harvest program authorized by the Northern Territory Government (Saalfeld et al., 2015). Alaric Fisher, Glenn Edwards, Tim Clancy, and Tony Griffiths supported the study.
References
Ball, M. (1998). Mangrove species richness in relation to salinity and waterlogging: a case study along the Adelaide River floodplain, northern Australia. Glob Ecol. Biogeogr. Lett. 7, 73–82. doi: 10.1111/j.1466-8238.1998.00282.x
Bayliss, P., Saunders, K., Dutra, L. X. C., Melo, L. F. C., Hilton, J., Prakash, M., et al. (2016). Assessing sea level-rise risks to coastal floodplains in the Kakadu Region, northern Australia, using a tidally driven hydrodynamic model. Mar. Freshwater Res. 69, 1064–1078. doi: 10.1071/MF16049
Bean, W. T., Stafford, R., and Brashares, J. S. (2012). The effects of small sample size and sample bias on threshold selection and accuracy assessment of species distribution models. Ecography 35, 250–258. doi: 10.1111/j.1600-0587.2011.06545.x
Bock, S. L., Lowers, R. H., Rainwater, T. R., Stolen, E., Drake, J. M., Wilkinson, P. M., et al. (2020). Spatial and temporal variation in nest temperatures forecasts sex ratio skews in a crocodilian with environmental sex determination. Proc.R. Soc. B.Biol. Sci. 287:20200210. doi: 10.1098/rspb.2020.0210
Booth, T. H., Nix, H. A., Busby, J. R., and Hutchinson, M. F. (2014). bioclim: the first species distribution modelling package, its early applications and relevance to most current MaxEnt studies. Divers. Distrib. 20, 1–9. doi: 10.1111/ddi.12144
Brackhane, S., Webb, G., Xavier, F. M. E., Gusmao, M., and Pechacek, P. (2018). When Conservation Becomes Dangerous: Human-Crocodile Conflict in Timor-Leste. J. Wildl. Manag. 821332–1344. doi: 10.1002/jwmg.21497
Britton, A. R. C., Whitaker, R., and Whitaker, N. (2012). Here be a dragon: exceptional size in a saltwater crocodile (Crocodylus porosus) from the Philippines. Herpetol. Rev. 43, 541–546.
Brochu, C. A., Wagner, J. R., Jouve, S., Sumrall, C. D., and Densmore, L. D. (2009). A Correction Corrected: Consensus Over the Meaning of Crocodylia and Why It Matters. Syst. Biol. 58, 537–543. doi: 10.1093/sysbio/syp053
Bureau of Meteorology. (2020). Climate Data Online. Available online at: http://www.bom.gov.au/climate/data/index.shtml (Accessed on February 20, 2020)
Charruau, P., Cantón, D., and Méndez-de-la-Cruz, F. (2017). Additional details on temperature-dependent sex determination in Crocodylus acutus. Salamandra 53, 304–308.
Cherkiss, M. S., Watling, J. I., Brandt, L. A., Mazzotti, F. J., Lindsay, J., Beauchamp, J. S., et al. (2020). Shifts in hatching date of American crocodile (Crocodylus acutus) in southern Florida. J. Thermal Biol. 88:102521. doi: 10.1016/j.jtherbio.2020.102521
Cowie, I. (2003). Freshwater aquatic plants of Darwin Harbour catchments. in Proceedings of Darwin Harbour Region: Current Knowledge and Future Needs. Public Presentations. (Darwin, AUS: Working Group for the Darwin Harbour Advisory Committee), 160–177.
Dangendorf, S., Hay, C., Calafat, F. M., Marcos, M., Piecuch, C. G., Berk, K., et al. (2019). Persistent acceleration in global sea-level rise since the 1960s. Nat. Clim. Chang. 9, 705–710. doi: 10.1038/s41558-019-0531-8
Department of the Environment and Energy. (2013). Code of Practice for the Humane Treatment of Wild and Farmed Australian Crocodiles. Available online at: http://www.environment.gov.au/resource/code-practice-humane-treatment-wild-and-farmed-australian-crocodiles (Accessed on February 28, 2014)
Elith, J., Phillips, S. J., Hastie, T., Dudík, M., Chee, Y. E., and Yates, C. J. (2011). A statistical explanation of MaxEnt for ecologists. Divers. Distrib. 17, 43–57. doi: 10.1111/j.1472-4642.2010.00725.x
esri. (2021). ArcGIS. Available online at: https://www.esri.com/en-us/home (accessed February 17, 2022).
Finlayson, C. M., Lowry, J., Bellio, M. G., Nou, S., Pidgeon, R., Walden, D., et al. (2006). Biodiversity of the wetlands of the Kakadu Region, northern Australia. Aquat. Sci. 68, 374–399. doi: 10.1007/s00027-006-0852-3
Fukuda, Y., and Cuff, N. (2013). Vegetation communities as nesting habitat for the saltwater crocodiles in the Northern Territory of Australia. Herpetol. Conservat. Biol. 8, 641–651.
Fukuda, Y., and McDonald, P. (2022). Maxent_results_crocodile_nesting_suitability [Dataset]. Figshare. Available online at: https://doi.org/10.6084/m9.figshare.17292191.v1 (accessed February 18, 2022).
Fukuda, Y., and Saalfeld, K. (2014). Abundance of Saltwater Crocodile Hatchlings is Related to Rainfall in the Preceding Wet Season in Northern Australia. herp. 70, 439–448. doi: 10.1655/HERPETOLOGICA-D-13-00096R3
Fukuda, Y., Webb, G., Edwards, G., Saalfeld, K., and Whitehead, P. (2021). Harvesting predators: simulation of population recovery and controlled harvest of saltwater crocodiles Crocodylus porosus. Wildl. Res. 48, 252–263. doi: 10.1071/WR20033
Fukuda, Y., Webb, G., Manolis, C., Delaney, R., Letnic, M., Lindner, G., et al. (2011). Recovery of saltwater crocodiles following unregulated hunting in tidal rivers of the Northern Territory. Aus. J. Wildl. Manag. 75, 1253–1266. doi: 10.1002/jwmg.191
Fukuda, Y., Whitehead, P., and Boggs, G. (2007). Broad-scale environmental influences on the abundance of saltwater crocodiles (Crocodylus porosus) in Australia. Wildl. Res. 34, 167–176. doi: 10.1071/WR06110
González, E. J., Martínez-López, M., Morales-Garduza, M. A., García-Morales, R., Charruau, P., and Gallardo-Cruz, J. A. (2019). The sex-determination pattern in crocodilians: A systematic review of three decades of research. J. Animal Ecol. 88, 1417–1427. doi: 10.1111/1365-2656.13037
Grigg, G. C. (1981). Plasma homeostasis and cloacal urine composition in Crocodylus porosus caught along a salinity gradient. J. Comp. Physiol. B. 144, 261–270. doi: 10.1007/BF00802765
Grigg, G. C., Taplin, L. E., Harlow, P., and Wright, J. (1980). Survival and growth of hatchling Crocodylus porosus in saltwater without access to fresh drinking water. Oecologia 47, 264–266. doi: 10.1007/BF00346830
Hausfather, Z. (2018). Explainer: How ‘Shared Socioeconomic Pathways’ explore future climate change. Carbon Brief. Available online atat: https://www.carbonbrief.org/explainer-how-shared-socioeconomic-pathways-explore-future-climate-change (Accessed on December 13, 2021)
Hernandez, P. A., Graham, C. H., Master, L. L., and Albert, D. L. (2006). The effect of sample size and species characteristics on performance of different species distribution modeling methods. Ecography 29, 773–785. doi: 10.1111/j.0906-7590.2006.04700.x
Hunter, C. M., Caswell, H., Runge, M. C., Regehr, E. V., Amstrup, S. C., and Stirling, I. (2010). Climate change threatens polar bear populations: a stochastic demographic analysis. Ecology 91, 2883–2897. doi: 10.1890/09-1641.1
IUCN (2012). Guidelines for application of IUCN Red List criteria at regional and national levels: version 4.0. (Gland, Switzerland: IUCN Species Survival Commission). Available at: http://www.iucnredlist.org/documents/reg_guidelines_en.pdf(Accessed on January 22, 2014)
Jevrejeva, S., Moore, J. C., and Grinsted, A. (2010). How will sea level respond to changes in natural and 394 anthropogenic forcings by 2100? Geophys. Res. Lett. 37:L07703. doi: 10.1029/2010GL042947
Jones, A. R., Jessop, T. S., Ariefiandy, A., Brook, B. W., Brown, S. C., Ciofi, C., et al. (2020). Identifying island safe havens to prevent the extinction of the World’s largest lizard from global warming. Ecol. Evol. 10, 10492–10507. doi: 10.1002/ece3.6705
Kushlan, J. A., and Jacobsen, T. (1990). Environmental Variability and the Reproductive Success of Everglades Alligators. J. Herpetol. 24, 176–184. doi: 10.2307/1564225
Laidre, K. L., Atkinson, S., Regehr, E. V., Stern, H. L., Born, E. W., Wiig, Ø, et al. (2020). Interrelated ecological impacts of climate change on an apex predator. Ecol. Appl. 30:e02071. doi: 10.1002/eap.2071
Letnic, M., and Connors, G. (2006). Changes in the distribution and abundance of saltwater crocodiles (Crocodylus porosus) in the upstream, freshwater reaches of rivers in the Northern Territory. Aus. Wildl. Res. 33, 529–538.
Maciejewski, K. (2006). Temperature-dependent sex determination in the Nile crocodile, Crocodylus niloticus, in the Okavango River, Botswana, and the effect of global climate change. Available online at: https://scholar.sun.ac.za:443/handle/10019.1/50648 (Accessed on October. 29, 2021).
Magnusson, W. E. (1980). Habitat Required for Nesting by Crocodylus porosus (Reptilia?: Crocodilidae). Wildl. Res. 7, 149–156. doi: 10.1071/wr9800149
Masson-Delmotte, V., Zhai, P., Pirani, A., Connors, S. L., Péan, C., Berger, S., et al. (2021). Climate Change 2021: The Physical Science Basis. Contribution of Working Group I to the Sixth Assessment Report of the Intergovernmental Panel on Climate Change.(Cambridge: Cambridge University Press).
Merow, C., Smith, M. J., and Silander, J. A. Jr. (2013). A practical guide to MaxEnt for modeling species’ distributions: what it does, and why inputs and settings matter. Ecography 36, 1058–1069. doi: 10.1111/j.1600-0587.2013.07872.x
Molnár, P. K., Derocher, A. E., Klanjscek, T., and Lewis, M. A. (2011). Predicting climate change impacts on polar bear litter size. Nat. Commun. 2:186. doi: 10.1038/ncomms1183
Mukul, S. A., Alamgir, M., Sohel, M. S. I., Pert, P. L., Herbohn, J., Turton, S. M., et al. (2019). Combined effects of climate change and sea-level rise project dramatic habitat loss of the globally endangered Bengal tiger in the Bangladesh Sundarbans. Sci. Total Environ. 663, 830–840. doi: 10.1016/j.scitotenv.2019.01.383
Mulrennan, M. E., and Woodroffe, C. D. (1998). Saltwater intrusion into the coastal plains of the Lower Mary River. Northern Territory, Australia. J. Environ. Manag. 54, 169–188. doi: 10.1006/jema.1998.0229
Nerem, R. S., Beckley, B. D., Fasullo, J. T., Hamlington, B. D., Masters, D., and Mitchum, G. T. (2018). Climate-change–driven accelerated sea-level rise detected in the altimeter era. PNAS 115, 2022–2025. doi: 10.1073/pnas.1717312115
Northern Territory, and Geological Survey. (2006). Geological Map of the Northern Territory. Available online at: https://geoscience.nt.gov.au/gemis/ntgsjspui/handle/1/82057 (Accessed on November 2, 2021).
Pettit, N. E., Bayliss, P., and Bartolo, R. (2018). Dynamics of plant communities and the impact of saltwater intrusion on the floodplains of Kakadu National Park. Mar. Freshwater Res. 69, 1124–1133. doi: 10.1071/MF16148
Pezeshki, S. R., Delaune, R. D., and Patrick, W. H. (1990). Flooding and saltwater intrusion: Potential effects on survival and productivity of wetland forests along the U.S. Gulf Coast. Forest Ecol.Manag. 3, 287–301. doi: 10.1016/0378-1127(90)90199-L
Phillips, S. J., and Dudík, M. (2008). Modeling of species distributions with Maxent: new extensions and a comprehensive evaluation. Ecography 31, 161–175. doi: 10.1111/j.0906-7590.2008.5203.x
Phillips, S. J., Dudik, M., and Schapire, R. E. (2021). Maxent software for modeling species niches and distributions. (New York, NY:American Museum of Natural History).
Platt, S. G., Rainwater, T. R., and McMurry, S. T. (2021). Fauna associated with the nests of Crocodylus moreletii and Crocodylus moreletii × acutus in Belize. J. Nat. His. 55, 133–149. doi: 10.1080/00222933.2021.1895350
Richardson, K. C., Webb, G. J. W., and Manolis, S. C. (2002). Crocodiles: Inside Out. A Guide to the Crocodilians and their Functional Morphology. (Sydney, Aus: Surrey Beatty & Sons).
Roos, J., Aggarwal, R. K., and Janke, A. (2007). Extended mitogenomic phylogenetic analyses yield new insight into crocodylian evolution and their survival of the Cretaceous–Tertiary boundary. Mol. Phylogene. Evol. 45, 663–673. doi: 10.1016/j.ympev.2007.06.018
Ryberg, W., and Lawing, M. (2018). “Genetic Consequences and Management Implications of Climate Change for the American Alligator (Alligator mississippiensis),” in American Alligators: Habitats, Behaviors, and Threats, eds S. Henke and C. Eversole 123–153. (Hauppauge, NY: Nova Science Publishers).
Saalfeld, K., Fukuda, Y., Duldig, T., and Fisher, A. (2015). Wildlife Trade Management Plan for the Saltwater Crocodile (Crocodylus porosus) in the Northern Territory of Australia, 2016-2020. (Darwin, AUS: Northern Territory Department of Land Resource Management).
Saalfeld, K., Fukuda, Y., Duldig, T., and Fisher, A. (2016). Management Program for the Saltwater Crocodile in the Northern Territory of Australia, 2016-2020. (Darwin, Australia: Northern Territory Department of Environment and Natural Resources). Available at: https://nt.gov.au/__data/assets/pdf_file/0007/443581/crocodile-management-program.pdf (accessed February 17, 2022).
Short, A., and Woodroffe, C. (2009). The coast of Australia. Available online at: https://ro.uow.edu.au/scipapers/4162 (accessed February 17, 2022).
Solomon, S., and Ipcc, and Intergovernmental Panel on Climate Change. (2007). Climate change 2007: the physical science basis: contribution of Working Group I to the Fourth Assessment Report of the Intergovernmental Panel on Climate Change. (Cambridge?; NY: Cambridge University Press)
Spennemann, D. H. R. (2021). Cruising the currents: Observations of extra-limital saltwater crocodiles (Crocodylus porosus Schneider, 1801) in the Pacific Region. Pacific Science 74, 211–227. doi: 10.2984/74.3.1
Taplin, L. E., and Grigg, G. C. (1981). Salt Glands in the Tongue of the Estuarine Crocodile Crocodylus porosus. Science 212, 1045–1047. doi: 10.1126/science.212.4498.1045
Webb, G. J. W., Manolis, S. C., and Brien, M. L. (2010). “Saltwater Crocodile Crocodylus porosus,” in Crocodiles Status Survey and Conservation Action Plan, eds S. C. Manolis and C. Stevenson (Darwin, AUS: Crocodile Specialist Group), 99–113. Available online at: http://www.iucncsg.org/365_docs/attachments/protarea/18%20–8088e67a.pdf (accessed February 17, 2022).
Webb, G. J. W., Manolis, S. C., Buckworth, R., and Sack, G. C. (1983). An Examination of Crocodylus porosus nests in two northern Australian freshwater swamps, with an analysis of embryo mortality. Wildl. Res. 10, 571–605. doi: 10.1071/wr9830571
Webb, G. J. W., Messel, H., and Magnusson, W. E. (1977). The nesting biology of Crocodylus porosus in Arnhem Land, northern Australia. Copeia 1977, 238–249. doi: 10.2307/1443905
Wisz, M. S., Hijmans, R. J., Li, J., Peterson, A. T., Graham, C. H., Guisan, A., et al. (2008). Effects of sample size on the performance of species distribution models. Divers. Distrib. 14, 763–773. doi: 10.1111/j.1472-4642.2008.00482.x
Woodroffe, C. D. (1988). Relict Mangrove Stand on Last Interglacial Terrace. Christmas Island, Indian Ocean. J. Tropical Ecol. 4, 1–17.
WorldClim. (2020). Bioclimatic variables — WorldClim 1 documentation. Available online at: https://www.worldclim.org/data/bioclim.html (Accessed on October 18, 2021)
Keywords: climate change, sea level rise (SLR), crocodile, habitat suitability analysis, Maxent, saltwater incursion, Kakadu National Park, Australia
Citation: Fukuda Y, McDonald PJ and Crase B (2022) Lost to the Sea: Predicted Climate Change Threats to Saltwater Crocodile Nesting Habitat. Front. Ecol. Evol. 10:839423. doi: 10.3389/fevo.2022.839423
Received: 20 December 2021; Accepted: 10 February 2022;
Published: 18 April 2022.
Edited by:
Jeanine M. Refsnider, The University of Toledo, United StatesReviewed by:
Lin Zhang, Hubei University of Chinese Medicine, ChinaPaula A. White, University of California, Los Angeles, United States
Copyright © 2022 Fukuda, McDonald and Crase. This is an open-access article distributed under the terms of the Creative Commons Attribution License (CC BY). The use, distribution or reproduction in other forums is permitted, provided the original author(s) and the copyright owner(s) are credited and that the original publication in this journal is cited, in accordance with accepted academic practice. No use, distribution or reproduction is permitted which does not comply with these terms.
*Correspondence: Yusuke Fukuda, eXVzdWtlLmZ1a3VkYUBudC5nb3YuYXU=