- 1College of Grassland Science and Technology, China Agricultural University, Beijing, China
- 2Qinghai Provincial Key Laboratory of Adaptive Management on Alpine Grassland, Xining, China
Plants have evolved various defense mechanisms to cope with biotic and abiotic stresses. Cooperation with microorganisms, especially arbuscular mycorrhizal fungi (AMF), strengthens the defense capabilities of host plants. To explore the effect of AMF on the growth of Elymus and the defenses against locust feeding, we designed a two-compartment device to connect or cut the mycelia and roots. We used this to investigate communication cues and pathways between donor and receiver plants. We found that AMF significantly increased the nitrogen content and decreased the carbon to nitrogen (C:N) ratio of donor plants and receiver plants and the carbon content of both. After the establishment of the common mycorrhizal network (CMN) with AMF between the two chambers, inoculations of donor plants challenged by locusts caused enhancement in four defense-related enzymes, namely, lipoxygenase, polyphenol oxidase, phenylalanine ammonia lyase, and β-1,3-glucanase, in the receiver plants. The main components of volatile organic compounds emitted by receiver plants were terpenoids. The findings indicated that AMF could not only improve plant growth but also activate the defense response of plants to insect feeding. Four defense enzymes, volatile organic compounds, and carbon and nitrogen content were involved in the defense response, and the mycelial network could act as a conduit to deliver communication signals.
Introduction
Insects and terrestrial plants have cooperated for millions of years. Plants gain protection and pollination from insect species, and insects gain food from plants (Frew et al., 2017). However, herbivore feeding sometimes threatens the growth of plants and can lead to plant death (Kong et al., 2016); hence, plants have evolved mechanisms to sense herbivorous insects and their natural enemies and rapidly respond to attacks through signaling changes in morphology, physiology, and biochemistry. Challenged by herbivores, plants innovate evolutionarily with induced responses evolving into resistance (Karban, 2020). These relationships are regulated by soil microbes (Wilkinson et al., 2019), and they affect the soil microbial communities in return (Rasmann et al., 2017; Tao et al., 2017; Garzo et al., 2020).
Arbuscular mycorrhizal fungi (AMF) from the phylum Glomeromycota establish symbiotic associations with 80% of terrestrial plants (Smith and Smith, 2011; Martin et al., 2017). This dates to 450 million years ago based on fossil and phylogenetic research (Smith and Read, 2010). AMF assist host plants to obtain more nutrients, such as phosphorus (P) and nitrogen (N), and plants provide carbohydrates to AMF in the form of sugar and lipids via exchange through arbuscules (Hodge and Storer, 2015; Hijikata et al., 2016; van der Heijden et al., 2016). Arbuscules are the primary sites for the exchange of nutrients between host plants and fungus (Caroline and Martin, 2013; Luginbuehl and Oldroyd, 2017). The benefits from AMF facilitate plant growth and strengthen plant resistance by influencing responses to abiotic stresses, such as drought, salinity, and extreme temperatures (Aliasgharzad et al., 2006; Mohamed et al., 2014; Delavaux et al., 2017), and biotic stresses, such as pathogens, herbivorous insects, and parasitic plants (Pineda et al., 2010; Cameron et al., 2013). Moreover, modifications of host plant phenotypes by AM fungi can impact insects affecting the plants (Meier and Hunter, 2018a; Garzo et al., 2020).
A key characteristic of AMF is the formation of common mycorrhizal networks (CMNs) by physically linking the individual roots of con- and heterospecific plants underground (Simard and Durall, 2004; Song et al., 2019). Symbionts of plants and mycorrhizae are capable of acquiring nutrients from other plants through CMNs (Giovannetti et al., 2001). The critical roles that mycorrhizas play in plant physiology, the production of primary or secondary metabolism and hormones, are necessary for individual growth and propagation (Smith and Read, 2010; Johnson and Gilbert, 2015). Research has demonstrated that CMNs deliver nutrients to accelerate the growth of adjacent plants (Smith and Read, 2010; Johnson and Gilbert, 2015), while also acting as a warning system of herbivore attacks and pathogens (Babikova et al., 2013; Song et al., 2019).
Volatile organic compounds (VOCs) as chemical signals are exuded by plants and released into the environment, affecting plant fitness and development indirectly (Unsicker et al., 2009; Bilas et al., 2021). Herbivore-induced VOCs emitted by attacked tissues are specific and stable signals that serve as warning signals to distant parts of the plant or its neighbors (Maffei, 2010; Tomczak et al., 2016; Hill et al., 2018; Meier and Hunter, 2018a,b; Velásquez et al., 2020). Plant defenselessness or resistance to mycorrhiza-induced pathogens and phytophagous insects has been reported (Gange et al., 2002; Barber, 2013; Bernaola et al., 2018). The positive (Pineda et al., 2010; Tomczak et al., 2016; Hill et al., 2018; Meier and Hunter, 2018a; Song et al., 2019) or negative (Vicari et al., 2002; Wang et al., 2015) effects that mycorrhiza induce have been documented. However, the ecological interactions that AMF have on aboveground plant–herbivore interactions in grassland ecosystems remain to be explored.
Locust outbreaks have occurred in many regions, lead to unpredictable agricultural outputs, and threaten human well-being (Cease et al., 2015; Zhang et al., 2019). The Tibetan Plateau, the highest area in the world, extending over 2.5 million km2, has suffered severe locusts damage (Cao et al., 2004). Elymus nutans Griseb. is a perennial, dominant species in the Qinghai-Tibet Plateau, China (Chu et al., 2016). It plays an important role in animal husbandry and in the ecological balance of this region. Despite numerous pieces of evidence indicating AMF-induced resistance against herbivores (Hill et al., 2018; Frew et al., 2020; Garzo et al., 2020), the effects of AMF on triggering or evoking defense against locusts are unclear. The main aim was to investigate how the inoculation of E. nutans with Funneliformis mosseae affected the defense mechanisms of the plant against locusts. We hypothesized that (1) AMF would increase the concentrations of VOCs and defense enzymes, which enhance the host plant defense and induce reactions in neighboring plants, and (2) the CMNs act as the signal pathways.
Materials and Methods
Study Organisms and Growth Conditions
Seeds of E. nutans and Elymus sibiricus Linn. were collected from the Tibetan Autonomous Prefecture of Haibei in Qinghai, China. It has a typical plateau continental climate and clay loam type soil. Precipitation is highest between June and September (Wei et al., 2021). The seeds were surface-sterilized with 10% H2O2 and rinsed three times before germination (Schweiger et al., 2014); then, they were sown and grown in a 3:2 mixture of clay and sand in a climate chamber (20°C, 12/12 h light/dark, 60–70% relative humidity). The soil was excavated (0–15 cm depth) in March 2020. After 2 weeks, 100 individuals (50 of E. nutans and 50 of E. sibiricus) were transferred into square plastic pots (30 cm length × 24 cm width × 12 cm depth) with a 2:1 mixture of soil and sand, and then autoclaved at 121°C for 2 h. They were stored in the dark at 4°C for 3 days and then transferred to a greenhouse (Tomczak et al., 2016). Each pot was watered with the same volume two times a week (1,800 ml/week/pot). The inoculum was mixed with the upper layer (3–4 cm) of the soil–sand mixture. Pots were randomly arranged in the greenhouse and replaced every 10 days during the growing period.
Funneliformis mosseae was purchased from the Beijing Academy of Agriculture and Forestry Sciences (No. BGCYN05), which were propagated with identified fungal spores and white clover (Trifolium repens) for 16 weeks in pots. The inoculant used in this experiment was a rhizosphere soil mixture of spores, extra-rhizome hyphae, and root segments of infected plants (containing 129 spores/g).
Locusts (Locusta migratoria manilensis) were collected from Yushu City, which is in the eastern part of Qinghai Province, China. The locusts were fed on wheat germ (∼16 g/day) and reared indoors at 28–30°C, 18/6 h (day/night), and 30–50% relative humidity (Veenstra et al., 2021). Fifth-instar locusts were used to attack grown plants (Wang et al., 2019).
Experimental Design
A rectangular pot (20 cm × 12 cm × 15 cm) was divided into two sections (I and II) using different nylon meshes to create a 2 cm air gap (Figure 1). Fifty seedlings of E. nutans as donor plants and E. sibiricus as receiver plants were planted in sections I and II, respectively. Six treatments were implemented: (A) I and II separated by 35-μm nylon mesh to prevent plant roots from connecting but CMNs were allowed to pass through (35 μm + AMF); (B) I and II separated by 0.5-μm nylon mesh to prevent CMNs passing through (0.5 μm + AMF); (C) I and II blocked with 35-μm nylon mesh to prevent plant roots from connecting, while CMN could form connections with neighbors. Then, pots were immediately rotated to snap CMNs before insects attack (rotated 35 μm + AMF); (D) I and II with no mesh, allowing donor roots and CMNs to connect with receivers (0 μm + AMF); (E) I and II separated with 35-μm mesh to hinder donor and receiver roots interacting and without AMF inoculation (35 μm + AMF); (F) I and II with no barriers and AMF inoculation (0 μm + AMF). Treatments A, B, C, and D had 20 g F. mosseae added in section I, and E and F were inoculated with 20 g AMF that were autoclaved at 120°C for 2 h. All treatments had three replicates. Twelve weeks after AMF inoculation, the donor plants in section I were treated with fifth-instar locusts. To avoid donor airborne volatile signals communicating with receiver plants, we covered all receivers with polyethylene terephthalate (PET) bags before adult locusts were fed. Fifth-instar locusts were starved for 24 h before they were used in the experiment.
Collection of Plant Volatile Organic Compounds
Volatile organic compounds were collected from all receivers during the day (10:00–16:00) (Huang et al., 2020) using dynamic headspace for 24 h after locusts attacked donor plants. The air was filtered by activated carbon (produced by Beijing Chemical Reagent Company) and humidified with distilled water. A 50 mg sample of Porapak Q (80–100 mesh, Waters Corporation, Ireland) was filled into dried glass narrow tubes (0.5 cm in diameter and 8.0 cm in length), and both ends of the tubes were plugged with a clean glass fiber. The dried tower, PET bags, and Teflon tubes were placed in a constant temperature drying oven to remove odors. The receiver plants were put in PET bags (100 cm × 111 cm, which had low volatile emissions under high temperature and high-intensity light). After the air in the bag was drawn out by a pump, sampling collection started after 30 min, and extraction with a flow rate of 300 ml/min was conducted for 6 h for VOC collection. All treatments were repeated three times. The adsorbent was eluted with 4 ml n-hexane to a 2 ml sample bottle, and the samples were kept in a refrigerator at –20°C for later use (Fontana et al., 2009).
Analysis of Volatile Organic Compounds
Volatile organic compounds were identified with an Agilent 7890B Gas Chromatograph coupled with Agilent 7200 MSD spectrometer and a DB-5MS column (30 m × 0.25 mm × 0.25 μm film, J&W Scientific, Folsom, CA, United States); the carrier gas was helium at 1 ml/min, with splitless injection (injection temperature 220°C, injection volume 1 μl). The working conditions of gas chromatography (GC) were as follows. The carrier gas flow rate was 2.0 ml/min. A two-stage temperature increase method was adopted, with the initial temperature of 40°C for 1 min followed by a heating rate of 5°C/min to 180°C, then at 10°C/min to 270°C for 2 min. The working conditions of mass spectrometry (MS) were as follows: solvent delay 1 min; an electron impact ion source (EI source) was used; electron energy was 70 eV; interface temperature was 250°C; ion source temperature was 220°C; and scanning mass range was 40–600 m/z with the scanning time in 0.2 s (Fontana et al., 2009). Volatile compounds were initially identified by comparing mass spectra with real standards or data system libraries (NIST 08, National Institute of Standards and Technology, Gaithersburg, MD, United States, 2008).
Arbuscular Mycorrhizal Fungi Colonization and Leaf Nitrogen and Carbon Concentration
Plant Traits
Mycorrhizal Colonization Measurement
Mycorrhizal colonization was examined using the method of Phillips and Hayman (1970). We collected the random receiver plant roots from four treatments, washed them in distilled water to remove soil particles, cut them into 1 cm segments of ∼1 g, immersed them in 10% KOH, and heated them in water at 90°C for 60 min. The root segments were watered to eliminate the alkali, and the remaining alkali was neutralized in 2% hydrochloric acid. We added 0.05% trypan blue to stain the root segments, separated them from the acid solution by heating at 90°C for 30 min, and then immersed them in the mixture solution of glycerol/lactic/water acid (1:1:1) to destain for 24 h. The samples were observed under a regular optical microscope at 40× to examine mycorrhizal colonization (Song et al., 2019).
Mycorrhizal colonization (%) = Number of infected root bits/Total number of root bits observed × 100%
Leaf Carbon and Nitrogen Measurement
Leaf tissues were milled with a ball mill (Retsch MM400, Retsch, Haan, Germany); 0.15 g of the sieved plant sample was weighed, filled into a tin cup, and measured using an elemental analyzer (Vario MAX CNS, Elementar, Hanau, Germany).
Enzyme Assays
Polyphenol Oxidase Enzyme Extraction and Activity Assays
Leaf samples were collected after the insects were fed and were held at −20°C until enzyme extraction and activity assays. Fresh leaf tissue (0.1 g) was used for the next preparation step for the crude enzyme extraction. We added 1 ml of extract that was homogenized in an ice bath, it was centrifuged at 8,000 × g at 4°C for 10 min using a FastPrep Instrument high-speed benchtop homogenizer (MP Biomedicals, CA, United States), and we placed the supernatant on ice to determine its activity. Enzymatic activities were evaluated by a microplate reader (SpectraMax iD5, Molecular Devices, San Jose, CA, United States) at 410 nm.
Phenylalanine Ammonia Lyase Enzyme Extraction and Activity Assays
Fresh leaf tissue (0.1 g) was used for the next preparation step of the crude enzyme extraction. We added 1 ml of extract to homogenize it, centrifuged at 10,000 × g at 4°C for 10 min, placed the supernatant on ice to determine its activity, used a plant phenylalanine ammonia lyase (PAL) activity detection kit for activity determination (Solarbio Science and Technology Ltd., Beijing, China), and evaluated enzymatic activities using the microplate reader at 290 nm.
Lipoxygenase Enzyme Extraction and Activity Assays
Fresh leaf tissue (0.1 g) was used for the next preparation step of the crude enzyme extraction. We added 1 ml extraction solution for ice bath homogenization, centrifuged at 16,000 × g at 4°C for 20 min, and placed it on ice to test its activity. We used a plant lipoxygenase (LOX) activity detection kit for activity determination (Solarbio Science and Technology Ltd., Beijing, China) and evaluated enzymatic activities using the microplate reader at 234 nm.
β-1,3-Glucanase Enzyme Extraction and Activity Assays
Fresh leaf tissue (0.1 g) was used for the next preparation step of the crude enzyme extraction. According to the ratio of tissue quality and an extraction solution of 1:5 or 1:10, we weighed 0.1 g of leaf tissue, added 1 ml of extraction solution for ice bath homogenization, centrifuged at 16,000 × g at 4°C for 20 min, and placed the supernatant on ice. We used a plant β-1,3-glucanase activity detection kit (Solarbio Science and Technology Ltd., Beijing, China) and evaluated enzymatic activities with the microplate reader at 410 nm.
Statistical Analysis
Data (means ± SD, n = 3) were analyzed by Analysis of Variance (ANOVA) using R software version 4.1.0. Plant carbon and nitrogen content and enzyme activities were analyzed with a one-way ANOVA in the “agricolae” package of R. The VOC analysis was run using Microsoft Excel 2010, and the figures were produced with R and Origin 2018.
Results
Inoculum Availability and Arbuscular Mycorrhizal Fungi Colonization
Arbuscular mycorrhizal fungi colonization was found in donor plants, with an average colonization rate of 13.34%. In treatment A, AMF colonization in the receiver plant was 5.98% (Figures 2A,B) which was significantly lower than the other three treatments. The mycelial hyphae freely passed through the 35-μm mesh, and the AMF colonization between the donor and the receiver plant was roughly the same in treatment B. With no mesh in treatment C, AMF also colonized in the receiver plants, and the colonization was significantly higher than that of the other three groups (P < 0.05). Treatment D was rotated before the insects were fed, so the donor and receiver plants were connected with the mycelial hyphae. The colonization rate of receiver plants was about 8.96%.
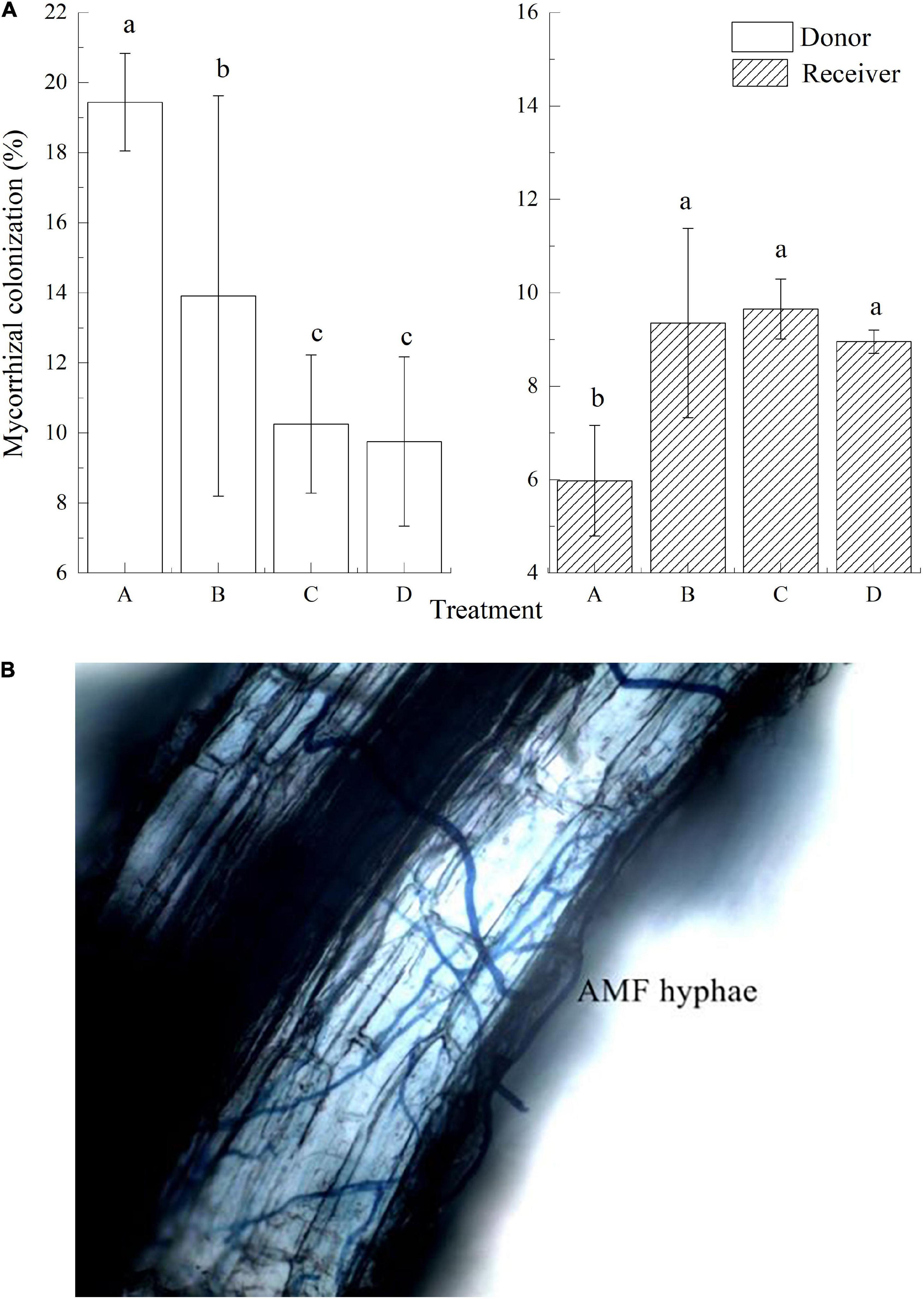
Figure 2. Mycorrhizal colonization and AMF hyphae of donor and received plants in different treatments. Data are mean ± SD (n = 3); different letters indicate significant difference (P < 0.05).
Plant Performance
The carbon content of donor plants inoculated with AMF significantly decreased (Figures 3A,B), while the nitrogen content increased (P < 0.05). Compared with the control (F), the carbon content in treatments A, B, C, and D decreased by 0.91, 0.47, 1.12, and 0.93%, respectively, while the nitrogen content increased by 0.88, 1.64, 1.28, and 1.26%, respectively. With the presence of CMN, the carbon content of receiver plants in treatments B and C was significantly lower than that in treatment A, and the rank among them was A (39.30%) > C (38.90%) > B (38.54%). The nitrogen content in treatment B (1.37%) and treatment C (1.32%) was significantly higher than that in treatment A (0.99%). For non-mycorrhizal plants, the carbon and nitrogen content of receiver plants with roots (F) was higher than that of plants without roots (P < 0.05).
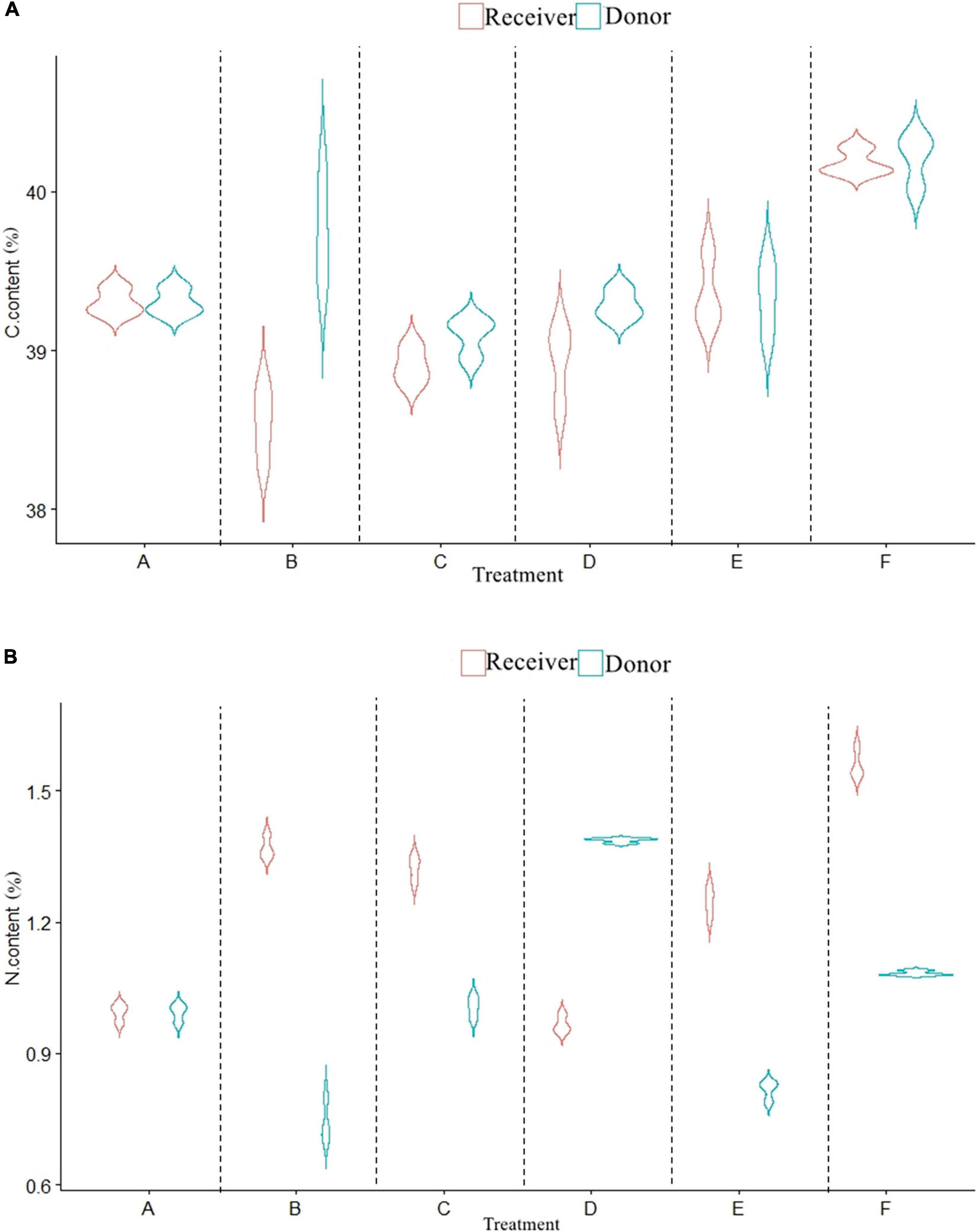
Figure 3. Effects of AMF on C and N content of donor and receiver plants between different treatments. Data are mean ± SD (n = 3).
Compared with non-mycorrhizal donor plants, mycorrhizal plants showed a higher carbon to nitrogen (C:N) ratio (P < 0.05; Figures 4A,B), and the average C:N ratios in mycorrhizal plants and non-mycorrhizal plants were 40.11 and 42.57, respectively. The receiver plants showed the same result (P < 0.05). The C:N ratios in the receiver plants under treatments A and D were significantly higher than those under other treatments, and the maximum difference was 14.66%.
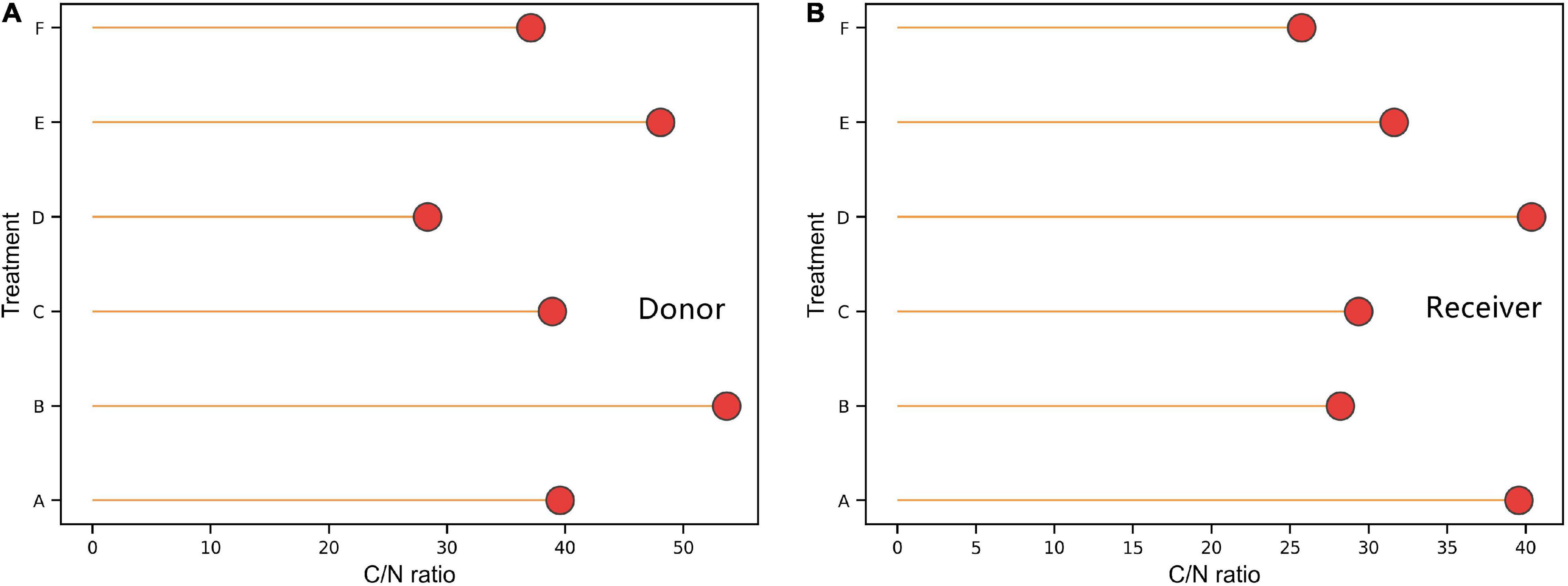
Figure 4. Effects of AMF on the ratio of C and N content of donor and received plants. Data are mean ± SD (n = 3).
Defense Enzymes and Volatile Organic Compounds Affected by Arbuscular Mycorrhizal Fungi
In comparison with non-mycorrhiza (F), four leaf defense-related enzymes, namely, PAL, LOX, polyphenol oxidase (PPO), and β-1,3-glucanase, in the donor plants and receiver plants that were inoculated with AMF were all higher than those in non-mycorrhizal plants, and PPO of both had the most significant differences. Compared with treatments B and C, LOX and PPO in the receiver plants of treatment A (without AMF hyphae) were lower. Without root and hyphae connections in treatments D and E, the activities of the four enzymes in the donor and receiver plants were similar (Figures 5A–D).
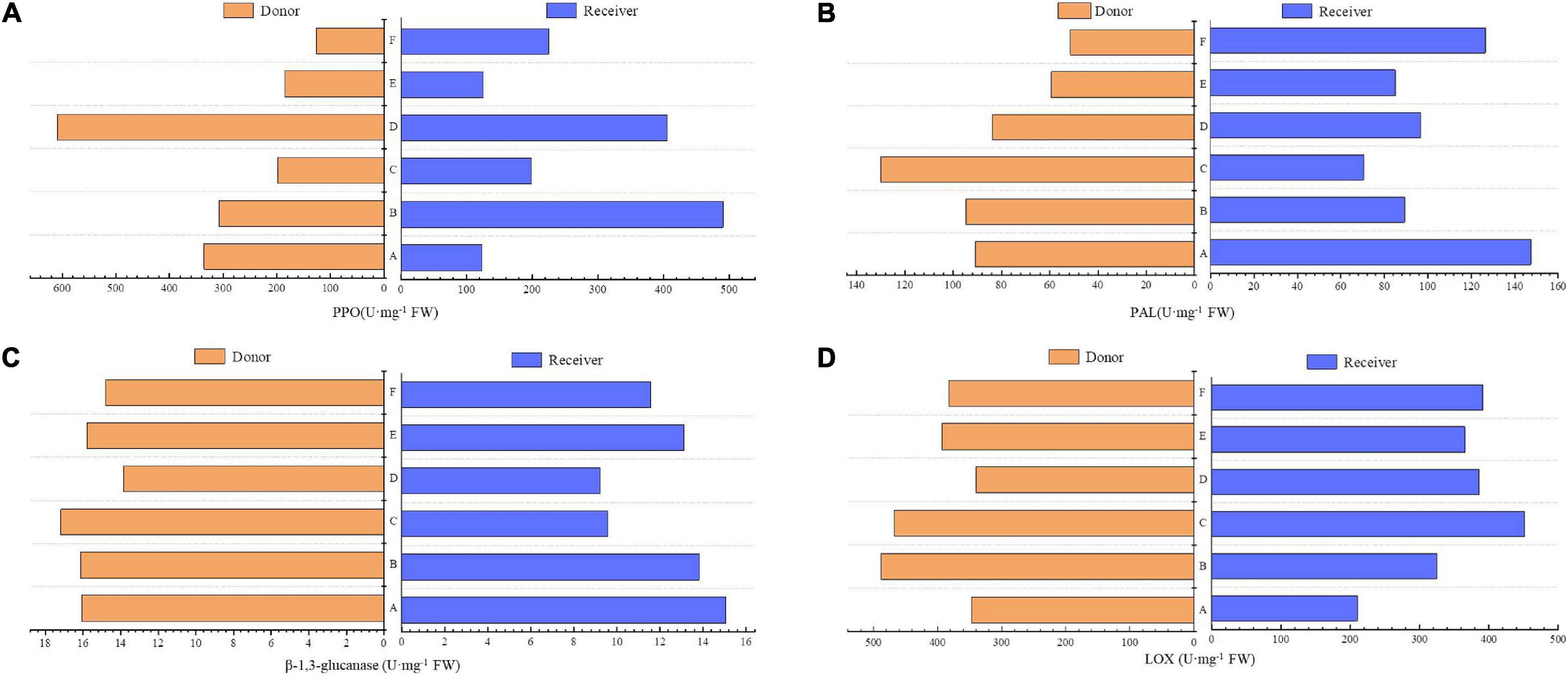
Figure 5. Effects of AMF on enzymes of donor and receiver plants between different treatments. Data are mean ± SD (n = 3).
The main volatile compounds emitted by the receiver plants were terpenoids (Table 1). Treatment E emitted the most kinds of volatile compounds, 13 types, whereas Treatment F emitted the least, eight types. Except for treatment E, the relative content of acetophenone, 2′,4′- dimethyl-, from other treatments was the highest. Other treatments did not emit 1,4-diethylbenzene, p-xylene, and 2- ethyl-, but all had acetophenone, 2′,4′- dimethyl-, 4-ethylbenzaldehyde, β-cymene, and p-cymene. Compared with the non-inoculation treatment, AMF increased acetophenone, 2′,4′- dimethyl-, 4-ethylbenzaldehyde, cumene, phenethyl alcohol, β-cymene, p-cymene, and 2-phenyl-1-propanal. For AMF-mediated plants, treatment B and C connected by hyphae produced eight substances, which were acetophenone, 2′,4′- dimethyl-, 4-ethylbenzaldehyde, β-cymene, phenethyl alcohol, p-cymene, 4-ethyltoluene, and p-propyltoluene. Compared with treatment A, the relative content of 1-methyl-2-phenylcyclopropane, p-cymene, 2-phenyl-1-propanal, 4-ethyltoluene, and p-propyltoluene increased in the other three treatments.
Relationships Between Plant Defense Traits and Arbuscular Mycorrhizal Fungi Colonization
The linear regression showed that VOC blends and C:N ratio varied with AMF colonization (Table 2), but there were no significant interactions between them and AMF colonization. Defense enzymes also varied predictably with AMF colonization, while different from the positive relationships between the other three enzymes and AMF colonization, β-1,3-glucanase decreased with increasing AMF colonization (P = 0.33, F = 1.01), showing the opposite trend. Despite their effects were not very strong, they also indicated that the increase in AMF colonization possibly influences plant defense.
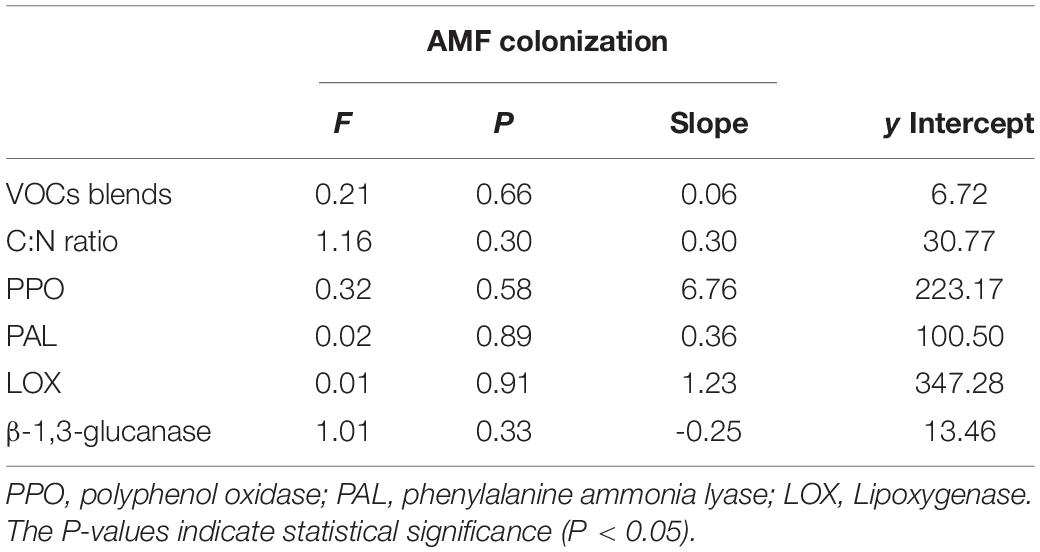
Table 2. Results of linear model analyses of the effects of the availability of arbuscular mycorrhizal fungi (AMF) inoculum on volatile organic compounds (VOCs) blends, C:N ratio, and defense enzymes.
Discussion
Effects of Arbuscular Mycorrhizal Fungi on Plant Nutrition Uptake
Our findings showed that AMF inoculation significantly increased the total nitrogen content and decreased the total carbon in host plants. This can be explained by the fact that nearly 25% of the photosynthetic product of the host plant is supplied to the mycorrhiza (Hobbie, 2006; van der Heijden et al., 2006). Fungal hyphae can explore large volumes of soil, enter soil pores that are inaccessible to roots (Smith and Read, 1997), transfer nitrogen as arginine or urea from the soil to the roots of a host plant, and thereby enhance plant accumulation of nitrogen (Hodge et al., 2010; van der Heijden et al., 2015; Ingraffia et al., 2021). The C:N ratio in plants is an important indicator for studying insect resistance (Těšitel et al., 2021). Healthy and nutrient-rich plants are more resistant to the feeding of herbivores. By contrast, plants with weaker vitality and limited resources may be more vulnerable (Löser et al., 2021). Our findings indicate that the C:N ratios in those receiver plants with AMF were much higher than those plants with no inoculations. This is consistent with another study that showed mycorrhizal symbionts have negative effects on herbivores by changing the C:N ratio (Getman Pickering et al., 2021), as plants with higher C:N ratio may reduce their nutritional value and their attractiveness to herbivores (Bennett et al., 2016; Getman Pickering et al., 2021). The enhancement of defenses results from increased nutrient acquisition (Mhlongo et al., 2018) and changes in defensive trypsin inhibitors (Getman Pickering et al., 2021).
Effects of Arbuscular Mycorrhizal Fungi on Plant Defense to Insects
Mycorrhizae modify plant traits, including chemical resistance (Hartley and Gange, 2009). Two signal pathways mediated by jasmonic acid (JA) and salicylic acid (SA) respond to physical or biological damage (Zhao et al., 2009). Enzymes such as β-1,3-glucanase and PAL are key enzymes involved in the biosynthesis of the signal molecule of SA (Balog et al., 2017). As the final enzymes of the main defense pathway, they directly participate in the synthesis of secondary metabolites related to plant defense and resistance (Selvaraj et al., 2020). Our results found that AMF led to an increase in the enzymatic activities of PPO, β-1,3-glucanase, PAL, and LOX, which has been shown in sweet pepper, tomato, and potato (Constabel and Barbehenn, 2008; Balog et al., 2017). Host plants show higher defenses, demonstrating that inoculation with AMF enhances the resistance of host plants to insects by improving enzymatic activities (Balog et al., 2017; Selvaraj et al., 2020). PPO and PAL increase to catalyze the production of poisonous quinone compounds via cross-linking proteins and carbohydrates, generating oxygen-free radicals (Bagy et al., 2019), and reducing the availability of protein (Selvaraj et al., 2020). The enhancement of melanin through PPOs can also increase the resistance of the cell wall to insects and pathogens (Zhao et al., 2009). LOX and PPO catalyze the initial reaction in the JA biosynthetic pathway, form hydroperoxides through catalytic reactions, and degrade hydroperoxides into reactive aldehydes and ketones through chemical reactions and enzymes (Bruinsma et al., 2009). An increase in PAL can promote the accumulation of phenolic substances and convert them into toxic compounds during oxidation (Bhonwong et al., 2009), and these phenolic substances are generally linked to plant resistances to insects (Frew et al., 2020).
Volatile organic compounds are important chemical signals for communication between phytophagous insects and their host plants (Liu and Brettell, 2019). In the arms race between plants and herbivores, any herbivore that can suppress plant-induced defense signals has increased fitness (Bruinsma et al., 2010; Zu et al., 2020). Plant–plant signals may have a fundamental impact on mycorrhizal plant–herbivore interactions at the community level and exert selective pressure on herbivore insects to evolve antagonistic mechanisms (Gilbert and Johnson, 2015). Plant compounds are complex mixtures of volatile gases that can directly repel herbivores and indirectly attract herbivore natural enemies (Huang et al., 2020). The amount and characteristics of the emitted plant VOCs vary from species to species (Zhao et al., 2009).
Various studies have revealed that AMF affect the interactions between plants and insects. Meier and Hunter (2019) reported that increasing cardenolides (a toxic substance) generated by milkweed inoculated with AMF decreased weights and growth rates of aphids. Velásquez et al. (2020) reported that an increase in (E)-2-hexenal, 3-hexenal, geraniol, benzaldehyde, and methyl salicylate associated with plant defenses has been shown in mycorrhizal plants linked to herbivore attacks. In Plantago lanceolate L., a large increase in volatile terpenoids mediated by AMF can assist in indirect defense against herbivore attacks (Fontana et al., 2009).
Our results showed that receiver plants that were linked to mycorrhizal donor plants released VOC emissions. Acetophenone, 2′,4′- dimethyl-, 4-ethylbenzaldehyde, cumene, phenethyl alcohol, β-cymene, p-cymene, and 2-phenyl-1-propanal were enhanced by AMF, indicating that AMF are capable of affecting the concentration and composition of plant secondary metabolites (Velásquez et al., 2020). As the most abundant and structurally diverse volatiles released by plants, terpenoids can drive interaction among insects, pathogens, and neighboring plants. Besides their role in direct and indirect defense, terpenoids affect defense-related signals released by the injured leaf to undamaged leaves through an internal system or to nearby plants through an external route (Sharma et al., 2017). In our study, mycorrhiza significantly improved and changed the relative content and composition of VOCs, such as cumene, phenethyl alcohol, 4-ethyltoluene, β-cymene, and 4-ethylbenzaldehyde. The majority of VOCs were terpenoids, which are combined with other compounds (green leaf volatiles, esters, aldehydes, and aromatic compounds) to enhance resistances (Kigathi et al., 2013; Kigathi et al., 2019). Among them, cumene affects the feeding of sucking herbivores (Gras et al., 2005) and primes defenses in Brassica nigra to white butterflies (Pashalidou et al., 2020). The specific effects of AMF on VOC emissions may be due to the differences in carbon allocation. Further, VOC emissions are mediated by interactions between plant hormones and secondary defense metabolites (Meier and Hunter, 2019).
The Signal Pathways of Defense Responses That Arbuscular Mycorrhizal Fungi Mediate
Root exudates and the fungal mycelia network mediate belowground plant communication (Guerrieri et al., 2002). Root exudates were excluded by a 2-cm gap in our devices, and our study showed that four defense-related enzymes and VOC emissions were higher in undamaged receiver plants, and the defensive substances induced by hyphal connections performed better compared with the control. This indicated that the defensive substances produced by the donor plants in different treatments were mainly transmitted through the mycelial network, demonstrating the importance of hyphae connection in the response of the receiver plants to the donor plants when harmed by insect pests (Barto et al., 2012). Studies have shown that the mycelial hyphae are a signal conduit (Babikova et al., 2013; Song et al., 2019). They transmit a series of signals and compounds between plants and trigger the response to organisms at various trophic levels (Babikova et al., 2013, 2014a; Alaux et al., 2020). Defense signals induced by herbivores can be transferred by a common mycelial network from injured plants to uninfected neighbors to enhance the resistance of injured individuals to herbivores and warn adjacent plants through CMN to prepare for future attacks without direct contact with herbivores. This early warning system may have a profound impact on trophic webs (Babikova et al., 2014b), especially plant–insect interactions (Barto et al., 2012).
Arbuscular mycorrhizal fungi induced host plant resistance to insects by facilitating the release of PPO, LOX, PAL, and β-1,3-glucanase and by mediating VOCs and C:N ratios. The common mycorrhizal network is an important pathway for transferring defense signals. Our findings showed that CMNs provide a potential approach for triggering defense responses in undamaged neighbors. Further studies are warranted to validate the extent and nature of the defense responses to different herbivores.
Data Availability Statement
The original contributions presented in the study are included in the article/Supplementary Material, further inquiries can be directed to the corresponding author.
Author Contributions
XS and KL conceived the study, supervised the writing, and revised the manuscript. LY led the writing. WZ and YG contributed sections to the manuscript. All authors read and approved the final submission.
Funding
This study was supported by the National Natural Science Foundation of China (grant number 31971746), Major Science and Technology Projects in Qinghai Province (2018-NK-A2), and the Platform of Adaptive Management on Alpine Grassland-livestock System (2020-ZJ-T07).
Conflict of Interest
The authors declare that the research was conducted in the absence of any commercial or financial relationships that could be construed as a potential conflict of interest.
Publisher’s Note
All claims expressed in this article are solely those of the authors and do not necessarily represent those of their affiliated organizations, or those of the publisher, the editors and the reviewers. Any product that may be evaluated in this article, or claim that may be made by its manufacturer, is not guaranteed or endorsed by the publisher.
Supplementary Material
The Supplementary Material for this article can be found online at: https://www.frontiersin.org/articles/10.3389/fevo.2022.833389/full#supplementary-material
Supplementary Figure S1 | The chromatograms of (1-Methylpropyl)benzene.
Supplementary Figure S2 | The chromatograms of 1-Methyl-2-phenylcyclopropane.
Supplementary Figure S3 | The chromatograms of 2,5-Dimethylacetophenone.
Supplementary Figure S4 | The chromatograms of 2,5-dimethylethylbenzene.
Supplementary Figure S5 | The chromatograms of 2-Phenyl-1-propanal.
Supplementary Figure S6 | The chromatograms of 2-Propenal.
Supplementary Figure S7 | The chromatograms of 4-Acetyl-m-xylene.
Supplementary Figure S8 | The chromatograms of 4-Ethyltoluene.
Supplementary Figure S9 | The chromatograms of Cumene, p-ethyl-.
Supplementary Figure S10 | The chromatograms of Cumene.
Supplementary Figure S11 | The chromatograms of Ethyl-benzaldehyde.
Supplementary Figure S12 | The chromatograms of Isobutenylbenzene.
Supplementary Figure S13 | The chromatograms of Isopropyl palmitate.
Supplementary Figure S14 | The chromatograms of p-Cymene.
Supplementary Figure S15 | The chromatograms of Phenethyl alcohol.
References
Alaux, P., Naveau, F., Declerck, S., and Cranenbrouck, S. (2020). Common mycorrhizal network induced JA/ET genes expression in healthy potato plants connected to potato plants infected by phytophthora infestans. Front. Plant Sci. 11:602. doi: 10.3389/fpls.2020.00602
Aliasgharzad, N., Neyshabouri, M. R., and Salimi, G. (2006). Effects of arbuscular mycorrhizal fungi and Bradyrhizobium japonicum on drought stress of soybean. Biologia 6119, S324–S328. doi: 10.2478/s11756-006-0182-x
Babikova, Z., Gilbert, L., Bruce, T., Dewhirst, S. Y., Pickett, J. A., and Johnson, D. (2014a). Arbuscular mycorrhizal fungi and aphids interact by changing host plant quality and volatile emission. Funct. Ecol. 28, 375–385. doi: 10.1111/1365-2435.12181
Babikova, Z., Johnson, D., Bruce, T., Pickett, J., and Gilbert, L. (2014b). Underground allies: how and why do mycelial networks help plants defend themselves? Bioessays 36, 21–26. doi: 10.1002/bies.201300092
Babikova, Z., Gilbert, L., Bruce, T. J. A., Birkett, M., Caulfield, J. C., Woodcock, C. M., et al. (2013). Underground signals carried through common mycelial networks warn neighbouring plants of aphid attack. Ecol. Lett. 16, 835–843. doi: 10.1111/ele.12115
Bagy, H. M. M. K., Hassan, E. A., Nafady, N. A., and Dawood, M. F. A. (2019). Efficacy of arbuscular mycorrhizal fungi and endophytic strain Epicoccum nigrum ASU11 as biocontrol agents against blackleg disease of potato caused by bacterial strain Pectobacterium carotovora subsp. Atrosepticum PHY7. Biol. Control 134, 103–113. doi: 10.1016/j.biocontrol.2019.03.005
Balog, A., Loxdale, H. D., Bálint, J., Benedek, K., Szabó, K., Jánosi-Rancz, K. T., et al. (2017). The arbuscular mycorrhizal fungus Rhizophagus irregularis affects arthropod colonization on sweet pepper in both the field and greenhouse. J. Pest Sci. 90, 935–946. doi: 10.1007/s10340-017-0844-1
Barber, N. A. (2013). Arbuscular mycorrhizal fungi are necessary for the induced response to herbivores by Cucumis sativus. J. Plant Ecol. 6, 171–176. doi: 10.1093/jpe/rts026
Barto, E. K., Weidenhamer, J. D., Cipollini, D., and Rillig, M. C. (2012). Fungal superhighways: do common mycorrhizal networks enhance below ground communication? Trends Plant Sci. 17, 633–637. doi: 10.1016/j.tplants.2012.06.007
Bennett, A. E., Millar, N. S., Gedrovics, E., and Karley, A. J. (2016). Plant and insect microbial symbionts alter the outcome of plant–herbivore–parasitoid interactions: implications for invaded, agricultural and natural systems. J. Ecol. 104, 1734–1744. doi: 10.1111/1365-2745.12620
Bernaola, L., Cosme, M., Schneider, R. W., and Stout, M. (2018). Belowground inoculation with arbuscular mycorrhizal fungi increases local and systemic susceptibility of rice plants to different pest organisms. Front. Plant Sci. 9:747. doi: 10.3389/fpls.2018.00747
Bhonwong, A., Stout, M. J., Attajarusit, J., and Tantasawat, P. (2009). Defensive role of tomato polyphenol oxidases against cotton bollworm (Helicoverpa armigera) and beet armyworm (Spodoptera exigua). J. Chem. Ecol. 35, 28–38. doi: 10.1007/s10886-008-9571-7
Bilas, R. D., Bretman, A., and Bennett, T. (2021). Friends, neighbours and enemies: an overview of the communal and social biology of plants. Plant Cell Environ. 44, 997–1013. doi: 10.1111/pce.13965
Bruinsma, M., Posthumus, M. A., Mumm, R., Mueller, M. J., van Loon, J. J. A., and Dicke, M. (2009). Jasmonic acid-induced volatiles of Brassica oleracea attract parasitoids: effects of time and dose, and comparison with induction by herbivores. J. Exp. Bot. 60, 2575–2587. doi: 10.1093/jxb/erp101
Bruinsma, M., van Broekhoven, S., Poelman, E. H., Posthumus, M. A., Müller, M. J., van Loon, J. J., et al. (2010). Inhibition of lipoxygenase affects induction of both direct and indirect plant defences against herbivorous insects. Oecologia 162, 393–404. doi: 10.1007/s00442-009-1459-x
Cameron, D. D., Neal, A. L., van Wees, S. C. M., and Ton, J. (2013). Mycorrhiza-induced resistance: more than the sum of its parts? Trends Plant Sci. 18, 539–545. doi: 10.1016/j.tplants.2013.06.004
Cao, G., Tang, Y., Mo, W., Wang, Y., Li, Y., and Zhao, X. (2004). Grazing intensity alters soil respiration in an alpine meadow on the Tibetan plateau. Soil Biol. Biochem. 36, 237–243. doi: 10.1016/j.soilbio.2003.09.010
Caroline, G., and Martin, P. (2013). Cell and developmental biology of the arbuscular mycorrhiza symbiosis. Annu. Rev. Cell Dev. Biol. 29, 593–617.
Cease, A. J., Elser, J. J., Fenichel, E. P., Hadrich, J. C., Harrison, J. F., and Robinson, B. E. (2015). Living with locusts: connecting soil nitrogen, locust outbreaks, livelihoods, and livestock markets. BioScience 65, 551–558. doi: 10.1093/biosci/biv048
Chu, X. T., Fu, J. J., Sun, Y. F., Xu, Y. M., Miao, Y. J., Xu, Y. F., et al. (2016). Effect of arbuscular mycorrhizal fungi inoculation on cold stress-induced oxidative damage in leaves of Elymus nutans Griseb. S. Afr. J. Bot. 104, 21–29.
Constabel, C. P., and Barbehenn, R. (2008). “Defensive roles of polyphenol oxidase in plants,” in Induced Plant Resistance to Herbivory, ed. A. Schaller (Dordrecht: Springer Netherlands), 253–270.
Delavaux, C. S., Smith Ramesh, L. M., and Kuebbing, S. E. (2017). Beyond nutrients: a meta-analysis of the diverse effects of arbuscular mycorrhizal fungi on plants and soils. Ecology 98, 2111–2119. doi: 10.1002/ecy.1892
Fontana, A., Reichelt, M., Hempel, S., Gershenzon, J., and Unsicker, S. B. (2009). The effects of arbuscular mycorrhizal fungi on direct and indirect defense metabolites of Plantago lanceolata L. J. Chem. Ecol. 35, 833–843. doi: 10.1007/s10886-009-9654-0
Frew, A., Powell, J. R., Hiltpold, I., Allsopp, P. G., Sallam, N., and Johnson, S. N. (2017). Host plant colonisation by arbuscular mycorrhizal fungi stimulates immune function whereas high root silicon concentrations diminish growth in a soil-dwelling herbivore. Soil Biol. Biochem. 112, 117–126. doi: 10.1016/j.soilbio.2017.05.008
Frew, A., Powell, J. R., and Johnson, S. N. (2020). Aboveground resource allocation in response to root herbivory as affected by the arbuscular mycorrhizal symbiosis. Plant Soil 447, 463–473. doi: 10.1007/s11104-019-04399-x
Gange, A. C., Stagg, P. G., and Ward, L. K. (2002). Arbuscular mycorrhizal fungi affect phytophagous insect specialism. Ecol. Lett. 5, 11–15. doi: 10.1046/j.1461-0248.2002.00299.x
Garzo, E., Rizzo, E., Fereres, A., and Gomez, S. K. (2020). High levels of arbuscular mycorrhizal fungus colonization on Medicago truncatula reduces plant suitability as a host for pea aphids (Acyrthosiphon pisum). Insect Sci. 27, 99–112. doi: 10.1111/1744-7917.12631
Getman Pickering, Z. L., Stack, G. M., and Thaler, J. S. (2021). Fertilizer quantity and type alter mycorrhizae-conferred growth and resistance to herbivores. J. Appl. Ecol. 58, 931–940. doi: 10.1111/1365-2664.13833
Gilbert, L., and Johnson, D. (2015). Plant-mediated ‘apparent effects’ between mycorrhiza and insect herbivores. Curr. Opin. Plant Biol. 26, 100–105. doi: 10.1016/j.pbi.2015.06.008
Giovannetti, M., Fortuna, P., Citernesi, A. S., Morini, S., and Nuti, M. P. (2001). The occurrence of anastomosis formation and nuclear exchange in intact arbuscular mycorrhizal networks. New Phytol. 151, 717–724. doi: 10.1046/j.0028-646x.2001.00216.x
Gras, E. K., Read, J., Mach, C. T., Sanson, G. D., and Clissold, F. J. (2005). Herbivore damage, resource richness and putative defences in juvenile versus adult Eucalyptus leaves. Austral. J. Bot. 53:33. doi: 10.1071/BT04049
Guerrieri, E., Poppy, G. M., Powell, W., Rao, R., and Pennacchio, F. (2002). Plant-to-plant communication mediating in-flight orientation of Aphidius ervi. J. Chem. Ecol. 28, 1703–1715.
Hartley, S. E., and Gange, A. C. (2009). Impacts of plant symbiotic fungi on insect herbivores: mutualism in a multitrophic context. Annu. Rev. Entomol. 54, 323–342.
Hijikata, N., Murase, M., Tani, C., Ohtomo, R., Osaki, M., and Ezawa, T. (2016). Polyphosphate has a central role in the rapid and massive accumulation of phosphorus in extraradical mycelium of an arbuscular mycorrhizal fungus. New Phytol. 2, 285–289.
Hill, E. M., Robinson, L. A., Abdul-Sada, A., Vanbergen, A. J., Hodge, A., and Hartley, S. E. (2018). Arbuscular mycorrhizal fungi and plant chemical defence: effects of colonisation on aboveground and belowground metabolomes. J. Chem. Ecol. 44, 198–208. doi: 10.1007/s10886-017-0921-1
Hobbie, E. A. (2006). Carbon allocation to ectomycorrhizal fungi correlates with belowground allocation in culture studies. Ecology 87, 563–569. doi: 10.1890/05-0755
Hodge, A., Helgason, T., and Fitter, A. H. (2010). Nutritional ecology of arbuscular mycorrhizal fungi. Fungal Ecol. 3, 267–273. doi: 10.1016/j.funeco.2010.02.002
Hodge, A., and Storer, K. (2015). Arbuscular mycorrhiza and nitrogen: implications for individual plants through to ecosystems. Plant Soil 386, 1–19. doi: 10.1007/s11104-014-2162-1
Huang, D., Sun, M., Han, M., Zhang, Z., Miao, Y., Zhang, J., et al. (2020). Volatile organic compounds (VOCs) regulate the spatial distribution of Lepidoptera insects in an orchard ecosystem. Biol. Control 149:104311. doi: 10.1016/j.biocontrol.2020.104311
Ingraffia, R., Saia, S., Giovino, A., Amato, G., Badagliacca, G., Giambalvo, D., et al. (2021). Addition of high C:N crop residues to a P-limited substrate constrains the benefits of arbuscular mycorrhizal symbiosis for wheat P and N nutrition. Mycorrhiza 31, 441–454. doi: 10.1007/s00572-021-01031-8
Johnson, D., and Gilbert, L. (2015). Interplant signalling through hyphal networks. New Phytol. 205, 1448–1453. doi: 10.1111/nph.13115
Karban, R. (2020). The ecology and evolution of induced responses to herbivory and how plants perceive risk. Ecol. Entomol. 45, 1–9. doi: 10.1111/een.12771
Kigathi, R. N., Weisser, W. W., Reichelt, M., Gershenzon, J., and Unsicker, S. B. (2019). Plant volatile emission depends on the species composition of the neighboring plant community. BMC Plant Biol. 19:58. doi: 10.1186/s12870-018-1541-9
Kigathi, R. N., Weisser, W. W., Veit, D., Gershenzon, J., and Unsicker, S. B. (2013). Plants suppress their emission of volatiles when growing with conspecifics. J. Chem. Ecol. 39, 537–545. doi: 10.1007/s10886-013-0275-2
Kong, H. G., Kim, B. K., Song, G. C., Lee, S., and Ryu, C. (2016). Aboveground whitefly infestation-mediated reshaping of the root microbiota. Front. Microbiol. 7:1314. doi: 10.3389/fmicb.2016.01314
Liu, H., and Brettell, L. E. (2019). Plant defense by VOC-induced microbial priming. Trends Plant Sci. 24, 187–189. doi: 10.1016/j.tplants.2019.01.008
Löser, T. B., Mescher, M. C., De Moraes, C. M., Maurhofer, M., and Rudi, K. (2021). Effects of root-colonizing fluorescent Pseudomonas strains on arabidopsis resistance to a pathogen and an herbivore. Appl. Environ. Microbiol. 87:e283120. doi: 10.1128/AEM.02831-20
Luginbuehl, L. H., and Oldroyd, G. E. D. (2017). Understanding the arbuscule at the heart of endomycorrhizal symbioses in plants. Curr. Biol. 17, 952–963.
Maffei, M. E. (2010). Sites of synthesis, biochemistry and functional role of plant volatiles. S. Afr. J. Bot. 76, 612–631. doi: 10.1016/j.sajb.2010.03.003
Martin, F. M., Uroz, S., and Barker, D. G. (2017). Ancestral alliances: plant mutualistic symbioses with fungi and bacteria. Science 6340:d4501.
Meier, A. R., and Hunter, M. D. (2018a). Mycorrhizae alter toxin sequestration and performance of two specialist herbivores. Front. Ecol. Evol. 6:33. doi: 10.3389/fevo.2018.00033
Meier, A. R., and Hunter, M. D. (2018b). Arbuscular mycorrhizal fungi mediate herbivore-induction of plant defenses differently above and belowground. Oikos 127, 1759–1775. doi: 10.1111/oik.05402
Meier, A. R., and Hunter, M. D. (2019). Mycorrhizae alter constitutive and herbivore-induced volatile emissions by milkweeds. J. Chem. Ecol. 45, 610–625. doi: 10.1007/s10886-019-01080-6
Mhlongo, M. I., Piater, L. A., Madala, N. E., Labuschagne, N., and Dubery, I. A. (2018). The chemistry of plant–microbe interactions in the rhizosphere and the potential for metabolomics to reveal signaling related to defense priming and induced systemic resistance. Front. Plant Sci. 9:112. doi: 10.3389/fpls.2018.00112
Mohamed, A. A., Eweda, W. E. E., Heggo, A. M., and Hassan, E. A. (2014). Effect of dual inoculation with arbuscular mycorrhizal fungi and sulphur-oxidising bacteria on onion (Allium cepa L.) and maize (Zea mays L.) grown in sandy soil under greenhouse conditions. Ann. Agric. Sci. 59, 109–118. doi: 10.1016/j.aoas.2014.06.015
Pashalidou, F. G., Eyman, L., Sims, J., Buckley, J., Fatouros, N. E., De Moraes, C. M., et al. (2020). Plant volatiles induced by herbivore eggs prime defences and mediate shifts in the reproductive strategy of receiving plants. Ecol. Lett. 7, 1097–1106.
Phillips, J. M., and Hayman, D. S. (1970). Improved procedures for clearing roots and staining parasitic and vesicular-arbuscular mycorrhizal fungi for rapid assessment of infection. Trans. Br. Mycol. Soc. 55:158. doi: 10.1016/S0007-1536(70)80110-3
Pineda, A., Zheng, S., van Loon, J. J. A., Pieterse, C. M. J., and Dicke, M. (2010). Helping plants to deal with insects: the role of beneficial soil-borne microbes. Trends Plant Sci. 15, 507–514. doi: 10.1016/j.tplants.2010.05.007
Rasmann, S., Bennett, A., Biere, A., Karley, A., and Guerrieri, E. (2017). Root symbionts: powerful drivers of plant above- and belowground indirect defenses. Insect Sci. 24, 947–960. doi: 10.1111/1744-7917.12464
Schweiger, R., Baier, M. C., and Mueller, C. (2014). Arbuscular Mycorrhiza-Induced shifts in foliar metabolism and photosynthesis mirror the developmental stage of the symbiosis and are only partly driven by improved phosphate uptake. Mol. Plant Microbe Interact. 27, 1403–1412. doi: 10.1094/MPMI-05-14-0126-R
Selvaraj, A., Thangavel, K., and Uthandi, S. (2020). Arbuscular mycorrhizal fungi (Glomus intraradices) and diazotrophic bacterium (Rhizobium BMBS) primed defense in blackgram against herbivorous insect (Spodoptera litura) infestation. Microbiol. Res. 231:126355. doi: 10.1016/j.micres.2019.126355
Sharma, E., Anand, G., and Kapoor, R. (2017). Terpenoids in plant and arbuscular mycorrhiza-reinforced defence against herbivorous insects. Ann. Bot. 119:w263. doi: 10.1093/aob/mcw263
Simard, S. W., and Durall, D. M. (2004). Mycorrhizal networks: a review of their extent, function, and importance. Can. J. Bot. 82, 1140–1165. doi: 10.1139/B04-116
Smith, F. A., and Smith, S. E. (2011). What is the significance of the arbuscular mycorrhizal colonisation of many economically important crop plants. Plant Soil 348, 63–79.
Song, Y., Wang, M., Zeng, R., Groten, K., and Baldwin, I. T. (2019). Priming and filtering of antiherbivore defences among Nicotiana attenuata plants connected by mycorrhizal networks. Plant Cell Environ. 42, 2945–2961. doi: 10.1111/pce.13626
Tao, L., Hunter, M. D., and de Roode, J. C. (2017). Microbial root mutualists affect the predators and pathogens of herbivores above ground: mechanisms, magnitudes, and missing links. Front. Ecol. Evol. 5:160. doi: 10.3389/fevo.2017.00160
Těšitel, J., Tahadlová, M., Lepš, J., and Hölzel, N. (2021). Linking insect herbivory with plant traits: phylogenetically structured trait syndromes matter. J. Veg. Sci. 32:e13061. doi: 10.1111/jvs.13061
Tomczak, V. V., Schweiger, R., and Müller, C. (2016). Effects of arbuscular mycorrhiza on plant chemistry and the development and behavior of a generalist herbivore. J. Chem. Ecol. 42, 1247–1258. doi: 10.1007/s10886-016-0785-9
Unsicker, S. B., Kunert, G., and Gershenzon, J. (2009). Protective perfumes: the role of vegetative volatiles in plant defense against herbivores. Curr. Opin. Plant Biol. 12, 479–485.
van der Heijden, M. G., de Bruin, S., Luckerhoff, L., van Logtestijn, R. S., and Schlaeppi, K. (2016). A widespread plant-fungal-bacterial symbiosis promotes plant biodiversity, plant nutrition and seedling recruitment. ISME J. 10, 389–399. doi: 10.1038/ismej.2015.120
van der Heijden, M. G. A., Martin, F. M., Selosse, M., and Sanders, I. R. (2015). Mycorrhizal ecology and evolution: the past, the present, and the future. New Phytol. 205, 1406–1423. doi: 10.1111/nph.13288
van der Heijden, M. G. A., Streitwolf-Engel, R., Riedl, R., Siegrist, S., Neudecker, A., Ineichen, K., et al. (2006). The mycorrhizal contribution to plant productivity, plant nutrition and soil structure in experimental grassland. New Phytol. 172, 739–752. doi: 10.1111/j.1469-8137.2006.01862.x
Veenstra, J. A., Leyria, J., Orchard, I., and Lange, A. B. (2021). Identification of gonadulin and insulin-like growth factor from migratory locusts and their importance in reproduction in Locusta migratoria. Front. Endocrinol. 12: 693068. doi: 10.3389/fendo.2021.693068
Velásquez, A., Vega-Celedón, P., Fiaschi, G., Agnolucci, M., Avio, L., Giovannetti, M., et al. (2020). Responses of Vitis vinifera cv. Cabernet Sauvignon roots to the arbuscular mycorrhizal fungus Funneliformis mosseae and the plant growth-promoting rhizobacterium Ensifer meliloti include changes in volatile organic compounds. Mycorrhiza 30, 161–170. doi: 10.1007/s00572-020-00933-3
Vicari, M., Hatcher, P. E., and Ayres, P. G. (2002). Combined effect of foliar and mycorrhizal endophytes on an insect herbivore. Ecology. 83, 2452–2464. doi: 10.2307/3071806
Wang, M. G., Bezemer, T. M., van der Putten. Wim., H., and Biere Arjen. (2015). Effects of the Timing of Herbivory on Plant Defense Induction and Insect Performance in Ribwort Plantain (Plantago lanceolata L.) Depend on Plant Mycorrhizal Status. J. Chem. Ecol. 41, 1006–17. doi: 10.1007/s10886-015-0644-0
Wang, P., Yin, X., and Zhang, L. (2019). Plant Approach-Avoidance response in locusts driven by plant volatile sensing at different ranges. J. Chem. Ecol. 45, 410–419. doi: 10.1007/s10886-019-01053-9
Wei, X. T., Shi, Y. N., Qin, F. W., Zhou, H. K., and Shao, X. Q. (2021). Effects of experimental warming, precipitation increase and their interaction on AM fungal community in an alpine grassland of the Qinghai-Tibetan Plateau. Eur. J. Soil Biol. 102:103272.
Wilkinson, T. D. J., Miranda, J., Ferrari, J., Hartley, S. E., and Hodge, A. (2019). Aphids influence soil fungal communities in conventional agricultural systems. Front. Plant Sci. 10:895. doi: 10.3389/fpls.2019.00895
Zhang, L., Lecoq, M., Latchininsky, A., and Hunter, D. (2019). Locust and grasshopper management. Annu. Rev. Entomol. 64, 15–34. doi: 10.1146/annurev-ento-011118-112500
Zhao, L. Y., Chen, J. L., Cheng, D. F., Sun, J. R., Liu, Y., Tian, Z., et al. (2009). Biochemical and molecular characterizations of Sitobion avenae-induced wheat defense responses. Crop Protect. 28, 435–442. doi: 10.1016/j.cropro.2009.01.005
Keywords: arbuscular mycorrhizal fungi, plant-insect-microbe interaction, plant evolution, volatile organic compounds, common mycelial network
Citation: Yu L, Zhang W, Geng Y, Liu K and Shao X (2022) Cooperation With Arbuscular Mycorrhizal Fungi Increases Plant Nutrient Uptake and Improves Defenses Against Insects. Front. Ecol. Evol. 10:833389. doi: 10.3389/fevo.2022.833389
Received: 11 December 2021; Accepted: 08 February 2022;
Published: 23 March 2022.
Edited by:
Shiliang Liu, Beijing Normal University, ChinaReviewed by:
Xukun Su, Research Center for Eco-Environmental Sciences (CAS), ChinaShikui Dong, Beijing Forestry University, China
Zhanhuan Shang, Lanzhou University, China
Copyright © 2022 Yu, Zhang, Geng, Liu and Shao. This is an open-access article distributed under the terms of the Creative Commons Attribution License (CC BY). The use, distribution or reproduction in other forums is permitted, provided the original author(s) and the copyright owner(s) are credited and that the original publication in this journal is cited, in accordance with accepted academic practice. No use, distribution or reproduction is permitted which does not comply with these terms.
*Correspondence: Xinqing Shao, c2hhb3hpbnFpbmdAMTYzLmNvbQ==