- 1W.K. Kellogg Biological Station, Michigan State University, Hickory Corners, MI, United States
- 2Ecology, Evolution, and Behavior Program, Michigan State University, East Lansing, MI, United States
- 3Environmental Science and Policy Program, Michigan State University, East Lansing, MI, United States
- 4United States Department of Agriculture-Agricultural Research Service (USDA–ARS) Center for Medical, Agricultural and Veterinary Entomology, Gainesville, FL, United States
- 5Department of Biology, University of Puerto Rico, Arecibo, PR, United States
- 6College of Natural and Computational Sciences, Hawai’i Pacific University, Kaneohe, HI, United States
Agricultural landscapes can be managed to protect biodiversity and maintain ecosystem services. One approach to achieve this is to restore native perennial vegetation within croplands. Where rowcrops have displaced prairie, as in the US Midwest, restoration of native perennial vegetation can align with crops in so called “prairie strips.” We tested the effect of prairie strips in addition to other management practices on a variety of taxa and on a suite of ecosystem services. To do so, we worked within a 33-year-old experiment that included treatments that varied methods of agricultural management across a gradient of land use intensity. In the two lowest intensity crop management treatments, we introduced prairie strips that occupied 5% of crop area. We addressed three questions: (1) What are the effects of newly established prairie strips on the spillover of biodiversity and ecosystem services into cropland? (2) How does time since prairie strip establishment affect biodiversity and ecosystem services? (3) What are the tradeoffs and synergies among biodiversity conservation, non-provisioning ecosystem services, and provisioning ecosystem services (crop yield) across a land use intensity gradient (which includes prairie strips)? Within prairie strip treatments, where sampling effort occurred within and at increasing distance from strips, dung beetle abundance, spider abundance and richness, active carbon, decomposition, and pollination decreased with distance from prairie strips, and this effect increased between the first and second year. Across the entire land use intensity gradient, treatments with prairie strips and reduced chemical inputs had higher butterfly abundance, spider abundance, and pollination services. In addition, soil organic carbon, butterfly richness, and spider richness increased with a decrease in land use intensity. Crop yield in one treatment with prairie strips was equal to that of the highest intensity management, even while including the area taken out of production. We found no effects of strips on ant biodiversity and greenhouse gas emissions (N2O and CH4). Our results show that, even in early establishment, prairie strips and lower land use intensity can contribute to the conservation of biodiversity and ecosystem services without a disproportionate loss of crop yield.
Introduction
Two global challenges of our time are supporting a growing human population and preventing the loss of biodiversity and ecosystem services (Kremen and Merenlender, 2018; Intergovernmental Platform on Biodiversity and Ecosystem Services [IPBES], 2019). The capacity to address these challenges depends largely on management of agricultural lands that dominate the landscape globally (Campbell et al., 2017; Raven and Wagner, 2021). In the US Midwest, for example, 38% of the landscape is planted in principal row crops (USDA National Agricultural Statistics Service, 2019). These agroecosystems were designed to maximize the production of food, fuel, and fiber, and they contribute to greenhouse gas emissions, pollution, and the loss of natural ecosystems and biodiversity (Tilman and Clark, 2015). To prevent further ecological harm and to sustain food, fuel, and fiber production for future generations, agricultural landscapes must be managed for multifunctionality and biodiversity (Asbjornsen et al., 2013; Intergovernmental Platform on Biodiversity and Ecosystem Services [IPBES], 2019; Mitchell et al., 2021). Yet, there are few assessments of the tradeoffs and synergies of biodiversity and ecosystem service responses across crop management and conservation practices required to address the two grand challenges (Wittwer et al., 2021).
Diversifying agricultural landscapes can promote biodiversity and non-provisioning ecosystem services without compromising crop yield (Tamburini et al., 2020; Tscharntke et al., 2021). Landscape diversification, an approach to land management rooted in indigenous knowledge, has been experimentally studied by the scientific community for applications in input-intensive cropping systems (Intergovernmental Platform on Biodiversity and Ecosystem Services [IPBES], 2016; Nkuba et al., 2020). One method of diversifying agricultural landscapes, as is done in the US Midwest, is to establish prairie strips on row crop farms. This conservation practice consists of retiring areas of farmland and actively restoring them by seeding native perennial vegetation. Supported by the United States Department of Agriculture (USDA) Conservation Reserve Program (CRP), prairie strips are one of many “edge of field” practices–including riparian buffers, hedgerows, and wildflower strips–aimed at incorporating native and diverse habitat into agricultural landscapes (The Nature Conservancy [TNC], 2021).
Once established, prairie strips in contoured farm landscapes can reduce soil erosion, improve water quality, and support biodiversity. This can provide benefits to the farm and farmer at disproportionately higher levels than the amount of farmland removed from production (Schulte et al., 2017). Prairie strips also have the potential to provide resources and habitat for beneficial insects and increase their spillover into the farm, where they can provide ecosystem services such as biocontrol and pollination (Blitzer et al., 2012; Morandin and Kremen, 2013; Kordbacheh et al., 2020). While prairie strips are known to benefit biodiversity and ecosystem services once they have been established for multiple years, these impacts have not been studied during their initial years of establishment. Measuring prairie strips’ conservation potential during their early establishment period can address this gap and increase the precision of farmers’ expectations of prairie strips.
The conservation potential of prairie strips relative to other agricultural conservation practices such as no tillage is also unknown. Biodiversity and ecosystem services in agroecosystems are driven not only by the presence of natural habitat on or near farms, but also by the agricultural management practices used in row crop areas. Crop rotations and cover crops generally increase biodiversity and enhance nutrient availability (Rusch et al., 2013; de Pedro et al., 2020; Bowers et al., 2021), whereas the use of tillage, pesticides, and fertilizer generally decrease biodiversity and increase greenhouse gas emissions (Syswerda and Robertson, 2014; Bowles et al., 2016; Raven and Wagner, 2021). But when implemented jointly, landscape diversification and crop management practices can interact to produce unique impacts on on-farm biodiversity and ecosystem services (Schmidt et al., 2005; Tscharntke et al., 2005; Landis, 2017). There is some evidence that conventionally managed farm fields with prairie strips increase ecosystem services compared to conventionally managed farm fields without strips, and research has focused on the reduction of nutrient runoff and erosion in contoured agricultural landscapes (Schulte et al., 2017). By directly comparing responses in fields with prairie strips with responses in fields managed with other practices, we can better identify combinations of prairie strips and crop management that may optimize crop yield, biodiversity, and ecosystem services.
We address how targeted conservation can promote multifunctionality including biodiversity, agricultural production, and other ecosystem services. Working in a 33-year-old experiment, we tested the effects of prairie strips and a gradient of crop management strategies across a suite of invertebrate biodiversity metrics and ecosystem services during the first 2 years of prairie strip establishment. First, we asked: what are the effects of newly established prairie strips on the spillover of biodiversity and ecosystem services into cropland? To test this, we measured how services changed with distance from a prairie strip. Second, we asked: how does time since prairie strip establishment affect biodiversity and ecosystem services in agricultural plots? Third, we asked: what are the tradeoffs and synergies among biodiversity conservation, non-provisioning ecosystem services, and crop yield across a gradient of land use intensity? To answer our third question, we examined all services across a land use gradient (including treatments with prairie strips) in relation to yield changes. Our study includes biodiversity measurements of ants, butterflies, dung beetles, and spiders, all of which can provide ecosystem services to farms. Our study also includes ecosystem service measurements of microbial activity, decomposition, greenhouse gas emissions, pollination, soil carbon, and crop yield.
Materials and Methods
Study Sites and Sampling Locations
We conducted our study at the Kellogg Biological Station Long-Term Ecological Research (KBS LTER) site in Hickory Corners, Michigan, United States (occupied Anishinaabe land) that was established in 1987. The KBS LTER is located in a temperate climate with a mean temperature of 10.1°C and mean annual precipitation of 100.5 cm (1981–2011 means) with increasing trends in temperature over the past few decades (Robertson and Hamilton, 2015; Liang and Robertson, 2021). Surface soils are 17% clay/43% sand Alfisol loams developed on glacial till and outwash (Robertson and Hamilton, 2015).
This study occurred in 2019–2020, the first 2 years after prairie strip planting. We worked in five treatments of KBS-LTER’s Main Cropping System Experiment (MCSE): conventionally managed row crops, no till row crops, reduced input row crops, biologically based (organic) row crops, and conservation land [details of crop management treatments are compared in Figure 1 and in Robertson and Hamilton (2015)]. The experiment consisted of six replicated plots of each treatment (six experimental blocks; Supplementary Figure 1a), with each plot having an area of one hectare (87 m × 105 m; Supplementary Figure 1b). Conventional and no till treatments received levels of chemical inputs that follow Generally Accepted Agricultural and Management Practices (GAAMP) in Michigan, United States and are typical for the US Midwest (details on the dates and quantities of fertilizer application, pesticide application, weed management, and soil preparation can be found at https://aglog.kbs.msu.edu). The conventional treatment was tilled with a chisel plow, and the no till treatment was managed as the conventional treatment but was left unplowed. The reduced input treatment received lower levels of inputs (nitrogen at planting and pesticides) than conventional and no till and had a legume cover crop in the winter. The biologically based treatment did not receive any chemical inputs, compost, or manure, and it had a legume cover crop and was rotary hoed five times after planting in 2020 to control weeds. Conservation land (referred to as early successional in site maps and earlier publications from this experiment) was unmanaged other than yearly burning in the spring to suppress woody vegetation. This treatment was a grassland with the dominant bloom period in the fall when goldenrods and asters flower. All treatments except conservation land were on a 3-year maize (Zea mays L.)—winter wheat (Triticum aestivum L.)—soybean (Glycine max L.) rotation. In the years of our study, wheat was planted in 2019 and maize in 2020.
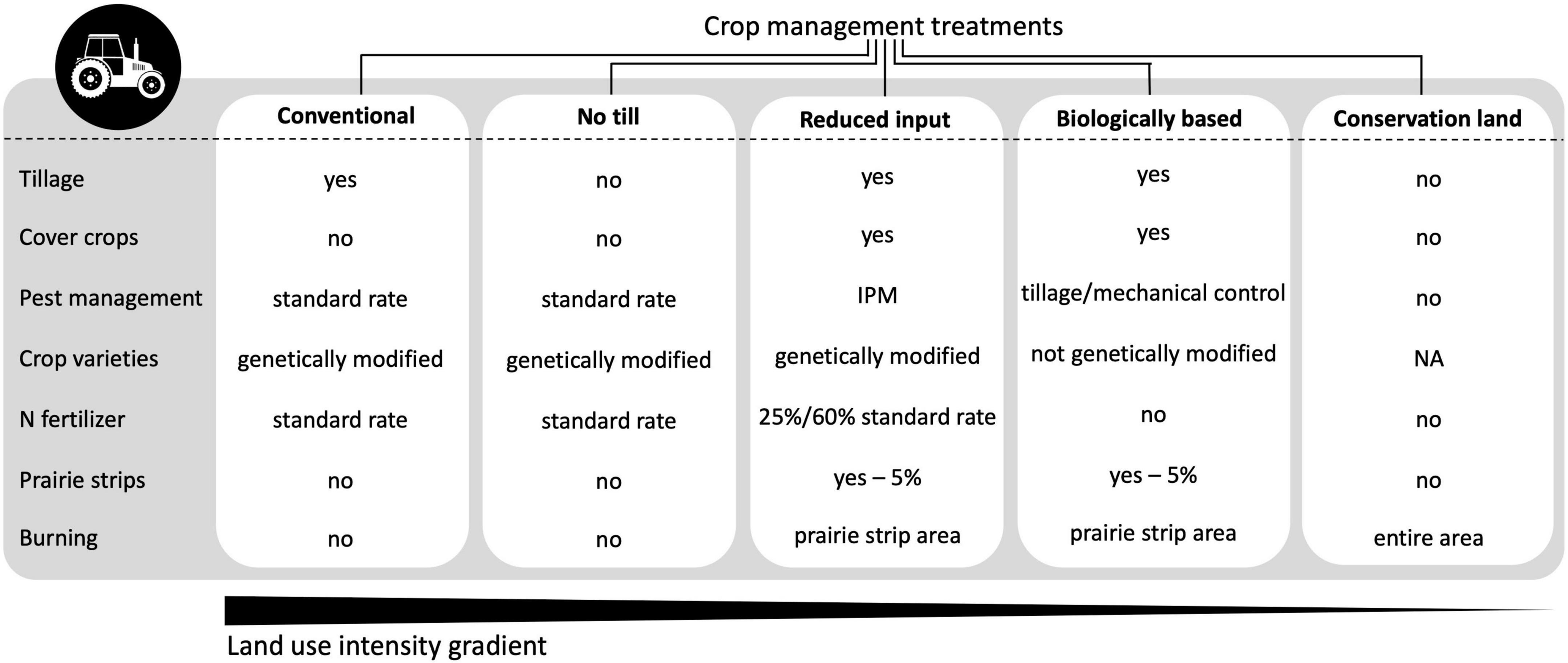
Figure 1. Characteristics of experimental crop management treatments. Standard rate refers to Generally Accepted Agricultural and Management Practices. IPM (Integrated Pest Management) in the reduced input treatment refers to a combination of extra tillage (maize years), narrow row spacing (soy years), and reduced herbicide use, mostly related to the number of residual herbicides. Fungicides and pesticides are applied as problems arise and severity is assessed. Also regarding reduced input, N fertilizer is applied at 25% of the standard rate for maize, and at 60% of the standard rate for wheat.
Prairie strips were introduced in the reduced input and biologically based treatments in April 2019. In five percent of each plot, configured as a strip parallel to row crops down the middle of each plot, we sowed a native prairie plants species mix. The mix consisted of 4 grass species and 18 forb species (Supplementary Table 1) purchased from Native Connections, Kalamazoo, Michigan, United States. The mix was chosen to have species bloom throughout the growing season (Isaacs et al., 2009). The mixes sown in each plot contained the same weight and proportion of each species. The first year’s plant community was dominated by agricultural weeds, but with some seeded species flowering. The second year’s plant community contained more seeded species, and we expect more seeded species to establish as the prairie strips mature. Prairie strips were mowed three times during the 2019 season to reduce weeds and support establishment of native seeds.
We compared a variety of biodiversity and ecosystem service measures by sampling three sets of sampling locations within the plots (Supplementary Figure 1b). First, to compare among plot-level treatments, each plot had five sampling locations distributed throughout, all located outside of prairie strips. These sampling locations are referred to as Standard Sampling Stations. Second, also to compare among plot-level treatments, each plot had six sampling stations at the northeast corner for destructive sampling that could not occur at the Standard Sampling Stations. These sampling locations are referred to as Subplot Sampling Stations. Third, reduced input and biologically based treatments had an additional three transects perpendicular to the prairie strips with sampling locations at distances of 0, 1, 5, and 20 m from the prairie strip (the station at 0 m was located within the prairie strip). These sampling stations were used to measure biodiversity and ecosystem services at different distances from the prairie strips and are referred to as Strip Sampling Stations.
Because prairie strips were implemented in all replicates of the reduced input and biologically based treatments, we do not have a fully factorial experiment. Therefore, we cannot isolate the effect of prairie strips from effects of crop management and year on measures of biodiversity and ecosystem services across all crop management treatments. However, we can (i) make conclusion about how treatments with prairie strips, in the context of their associated management strategies (including historical data on these treatments), compare to other crop management strategies without prairie strips, (ii) examine trends of prairie strips with time since establishment, where increases from year to year would suggest prairie strips play a role for processes that stabilized with the background management treatments (though are confounded by crop rotation), and (iii) attribute significant effects of distance from prairie strips on response variables to the presence of the strip, though benefits of prairie strips are not isolated to spillover effects.
Dung Beetles
Soil dwelling macroarthropods contribute to decomposition in agricultural landscapes by fragmenting litter, altering soil structure, and feeding on other soil dwelling fauna. Dung beetles break apart manure, mobilize nutrients in the soil (Coleman et al., 2018a), and suppress human and livestock pathogens (Nichols et al., 2008; Jones et al., 2016; Sands and Wall, 2017). Dung beetle communities are vulnerable to the effects of crop management and are negatively affected by agricultural intensification (Barbero et al., 1999; Hutton and Giller, 2003).
Across Management Treatments
To compare dung beetle diversity (as well as ants and spiders which are described next) across crop management treatments, we installed pitfall traps at Subplot Sampling Stations within each plot. Pitfall traps consisted of plastic containers (5.1 cm diameter, 120 mL) buried so the container’s rim was flush with the soil surface. We partially filled containers with 95% ethanol mixed with a few drops of detergent to break surface tension. To protect the traps from rain and flooding, we mounted clear Plexiglass rain covers (15 × 15 cm) 10 cm above the ground over each trap. We baited traps with approximately 10 g of cow manure per trap that was collected from the Kellogg Pasture Dairy Farm (located approximately 0.4 km from experimental sites) and homogenized by stirring in a bucket. Cows were treated with an ingested larvicide for fly control (unpublished data shows this had no effect on abundance and richness of dung beetles collected or manure decomposition). We deployed pitfall traps at all Subplot Sampling Stations three times during the growing season in 2019, once each in June, July, and August. For each sampling event, traps were collected after 48 h in the field and the samples collected from the traps were stored in ethanol at −20°C. Dung beetles (Coleoptera: Scarabaeidae of the subfamily Scarabaeinae) were identified to species using a regional guide (Nemes and Price, 2015).
Distances From Prairie Strips
To measure dung beetle richness at distances from prairie strips, baited pitfall traps were deployed at Strip Sampling Stations for each of three sampling rounds each year occurring in June, July, and August of 2019 and 2020.
Ants
Ants comprise half of global insect biomass and perform many ecosystem services (Hölldobler and Wilson, 1990; Folgarait, 1998; Wills and Landis, 2018). Ants are the major predators of agricultural pests at our study site and elsewhere in the US Midwest (Grieshop et al., 2012; Wills et al., 2019; Helms et al., 2020). They also disperse plant material, seeds, and nitrifying bacteria and pool nutrients in the soil (Mueller et al., 2005; Hölldobler and Wilson, 2009; Benckiser, 2010). Ants are sensitive to harvesting and management practices that can reduce ant activity (Peck et al., 1998; Agosti et al., 2000; Wodika et al., 2014; Helms et al., 2021; Hussain et al., 2021).
Across Management Treatments
We collected ants across crop management treatments using baited pitfall traps at Subplot Sampling Stations as described for dung beetles. Captured ants were identified using regional guides (Coovert, 2005; Ellison et al., 2012) and vouchers were stored in the senior author’s reference collection.
To test effects of treatments on species richness, we first combined species occurrences from all repeated pitfall traps (maximum of 3 traps per each of 6 sampling stations, 16–18 total pitfall traps per plot). The occurrence of workers of a given species at least once at any of the 6 sampling stations was conservatively treated as indicating the presence of a single colony of that species (abundance = 1) within a plot during the study year, regardless of how many or how frequently workers were captured (Ellison et al., 2007; Gotelli et al., 2011). In this way, we derived one species list for each of the 30 plots (6 plots per each of 5 treatments).
Distances From Prairie Strips
To measure ant richness at different distances from prairie strips, ants were collected with non-baited pitfall traps at the Strip Sampling Stations (reduced input and biologically based treatments). Non-baited traps were sampled on a rolling weekly basis (3 weeks on, one week off during which baited traps were deployed) from May to September with a total of five sampling rounds per station in 2019 and four in 2020. 2019 prairie strip ant data are modified from those used in Helms et al. (2021).
Spiders
Spiders are generalist predators that can contribute to pest control in agricultural landscapes. Spider communities generally respond positively to agricultural conservation practices, such as cover crops and reduced tillage (Sunderland and Samu, 2000; de Pedro et al., 2020). Increased natural habitat in an agricultural landscape can increase spider abundance and richness, but there is little evidence of spillover of spiders from natural habitat into cropland (Sunderland and Samu, 2000; Schmidt et al., 2005, but see Hussain et al., 2021).
Across Management Treatments
We collected spiders across crop management treatments as described for ants. Spiders were identified to family with a key to spiders of North America (Ubick et al., 2017).
Distances From Prairie Strips
Pitfall traps were used to collect spiders at distances from the prairie strips as described for ants.
Butterflies
Butterflies are diverse pollinators, herbivores, and indicators of insect response to habitat change, and they hold cultural value (Ghazanfar et al., 2016). Butterflies are declining in abundance at a rate of 2% per year in the US Midwest with agriculture as a main reason for this decline due to habitat loss, pesticides, and fertilizers, which are sources of direct mortality and destroy host plants and food resources (Wepprich et al., 2019; van Klink et al., 2020). Reducing the use of pesticides and fertilizer and restoring habitat on farms helps mitigate the loss of butterfly biodiversity in agricultural landscapes (Reeder et al., 2005; Rundlöf et al., 2008).
Across Management Treatments
Unlike samples for all the other species and services, we sampled butterfly species richness and abundance using transect counts, modified from Pollard (1977). We conducted surveys along a 12-min one-way walking transect through each plot. Observers recorded butterflies within 5 m on both sides and above the transect in front of the observer. Transects were surveyed between 10:00 a.m. and 4:00 p.m. weekly from June 2019 to September 2019 and May 2020 to September 2020. Butterflies were identified to species using a regional guide (Nielsen, 1999) and supplementary sources as needed.
Active Carbon
Biologically available soil carbon, also termed “active carbon,” reflects a fraction of total soil carbon that is readily mineralized by soil microorganisms and serves as an early indicator of longer-term soil carbon accrual (Culman et al., 2012; Coleman et al., 2018b). Conversion of agricultural fields to perennial vegetation has been shown to increase soil active carbon compared to conventionally managed agricultural soils by increasing the production of fine root biomass (Sprunger et al., 2017; Sprunger and Robertson, 2018). We expect prairie strips to increase levels of active carbon and for active carbon to spill over from prairie strips into cropland at short distances from prairie strips if roots from perennials extend into cropland, if nitrogen from farming doesn’t reach to exactly the edge of the prairie strip, or if litter from prairie strips spills over into cropland.
Across Management Treatments
Active carbon was determined via a 24-h assay based on Franzluebbers et al. (2000) that measures CO2 respired from soils rewetted to a common water holding capacity. We collected soil cores at the Standard Sampling Stations in June, July, and August of 2019. Samples were analyzed individually for active carbon, then data were pooled across June, July and September to form a single dataset for each year in each treatment. We collected field soil with a soil push probe at 0–10 cm depth then sieved to 2 mm. Soil water holding capacity (WHC) and gravimetric soil moisture were determined from fresh sieved soil. We added 5 g of air-dried soil and sterile ultrapure water to a 125 mL Wheaton serum bottle to achieve 70% WHC. Bottles were sealed and incubated at room temperature for 24 h. We collected gas samples from bottle headspace at two time points following the incubation period (0 and 24 h). CO2 samples were collected in overpressurized 6 mL glass vials (Exetainers, Labco Ltd., Lampeter, Wales) flushed with N2. We analyzed samples with a gas chromatograph (Agilent 7890A) coupled to an autosampler (Gerstel MPS2XL) as described in Shcherbak and Robertson (2019).
We calculated short-term mineralizable C as the difference between 0 and 24-h CO2 measurements. We report active carbon in micrograms (μg) of CO2 per day per g of dry soil.
Distances From Prairie Strips
Soil cores were collected at Strip Sampling Stations and processed as described above in both 2019 and 2020.
Decomposition
Decomposition is essential for suppressing pathogens, cycling nutrients, and creating soil organic matter (Barrios, 2007; Coleman et al., 2018c). Diversified landscapes can increase decomposition by increasing the abundance and richness of beneficial soil fauna (Landis et al., 2000; Karp et al., 2016; Jones et al., 2019). To quantify decomposition services, we measured mass loss of manure over time.
Across Management Treatments
We placed one patty of fresh cow manure (20 g) at each of the Subplot Sampling Stations (Jones et al., 2019). Manure (fresh) was weighed in the lab and separated into individual packets prior to deployment. We left manure under a rain cover (same rain cover as described for ants) for 7 days immediately following pitfall trap collection of dung beetles for all sampling rounds. We then collected manure in an envelope, placed the envelope in a drying oven until moisture evaporated, and then weighed it. The dry weight after deployment was divided by the dry weight of the manure (20 g of fresh manure was equivalent to 6 g dried manure). We defined decomposition as the proportion of manure removed.
Distances From Prairie Strips
We placed sentinel cow manure patties (20 g) at the Strip Sampling Stations and processed samples as described above.
Global Warming Impact
Agriculture produces 10–14% of global anthropogenic greenhouse gas emissions (Barker et al., 2007; Smith et al., 2007). Prairie strips, no till management, and cover crops are among management practices that have the potential to sequester carbon in cropping systems (Robertson et al., 2000; Gelfand and Robertson, 2015). We use 100-year global warming impact (GWI) as a measure to convert greenhouse gas emissions (N2O and CH4) to units of CO2 equivalent emissions.
Across Management Treatments
We sampled greenhouse gas fluxes per Kahmark et al. (2020) approximately biweekly May–September and monthly October–April in both 2019 and 2020 using a stainless-steel gas chamber (14.3 cm radius, 22.8 cm height) with a plastic lid. After placing the lid on the chamber, a needle was inserted into the chamber lid septum to relieve any induced pressure changes. We inserted another individual needle into the septum of a 5.9-mL exetainer sample vial to act as a vent. Then we mixed the chamber headspace three times with a 10-mL sampling syringe. After mixing, we withdrew 10-mL and injected the air into the sample vial with the vent needle in place. After flushing the vial three times, we removed the vent needle, drew a 10-mL sample from the chamber, and injected it into the flushed sample vial (so that it was overpressurized). We collected a sample of ambient air at the same time in each sampling round and also a duplicate chamber sample using the same gas sampling procedure described above. We also recorded soil temperature and moisture next to the gas chamber during the sampling period. We collected four gas samples at 15-min intervals over each sampling period. Post gas sampling and flux calculations were conducted following the protocol of Holland et al. (1999).
Pollination
Pollinators are necessary for the function of natural and managed ecosystems. Pollinators have experienced a steep decline in abundance and richness, and prairie strips could restore pollinators and their services to agricultural landscapes (Intergovernmental Platform on Biodiversity and Ecosystem Services [IPBES], 2016; Wepprich et al., 2019; van Klink et al., 2020; Kordbacheh et al., 2020).
Across Management Treatments
Pollination was measured with sentinel plants placed at the Standard Sampling Stations in the conventional, no till, and conservation land treatments, as well as at the Strip Sampling Stations in the reduced input and biologically based treatments. We used Black-eyed Susans (Rudbeckia hirta) as our sentinel plants, as it is native to southwest Michigan and was also included in the prairie strip seed mix. We propagated plants from seed (purchased from the same location as the prairie strip seed mix) in a greenhouse. Seedlings were transplanted into 16.5 cm pots with a low dose of 12-12-12 N-P-K controlled release organic fertilizer. To avoid pollination prior to receiving experimental treatment, we marked and covered two flower heads on each plant with pollinator exclusion bags just before they started producing pollen. The following day, we deployed plants with exclusion bags on both flowers into the field. Upon placement in the field, we removed one bag to be exposed to pollinators (called open flowers). One bag remained over the flower through the duration in the field (called closed flowers). Closed flowers acted as a measure of potential self-pollination. We deployed plants in experimental treatments for 14 days during each of the three sampling rounds starting on June 18, 2019, August 7, 2019, and July 8, 2020. We bagged all experimental flower heads prior to removal from experimental stations. For analysis of pollination across treatments, we used all plants from Standard Sampling Stations in conventional, no till, and conservation land, and we randomly selected five plants from the Strip Sampling Stations in the reduced input and biologically based treatments to compare consistently among all treatments.
Following experimental deployment, we returned plants to a greenhouse where they senesced and set seed. Seeds were then harvested and stored in a refrigerator from September to January each year. We randomly selected 30 seeds from each flower head for a germination trial. We placed these seeds in petri dishes in a greenhouse, watered them regularly over a 14-day period, and counted the number of individuals that germinated. We calculated seedset as the ratio of not-germinated:germinated seeds for each seed head (two measures per plant). We measured pollination services as the difference between seedset of the open flower and seedset of the closed flower for each plant (one measure per plant).
Distances From Prairie Strips
The sentinel plants from the Strip Sampling locations were used to measure pollination services at distances from the prairie strips.
Soil Organic Carbon
Soil organic carbon (SOC) is a measure of total carbon in soil organic matter. Agricultural management practices influence SOC accrual and loss. SOC accrual can be stimulated by the addition of high-quality organic inputs like cover crops (Syswerda et al., 2011), as well as the establishment of perennial vegetation (Kravchenko et al., 2019). On the other hand, practices that involve physical soil disturbance, such as tillage, generally reduce SOC by disrupting soil aggregates and releasing organic matter for decomposition (Paul et al., 2015). Whereas active carbon responds quickly to land management changes, SOC generally responds on the order of years to decades, as it is a measure of total carbon across both labile and recalcitrant soil organic matter pools (Culman et al., 2012).
Across Management Treatments
We collected one soil core with a soil push probe at each Standard Sampling Station in April 2019 and May 2020 at a depth of 0–25 cm. Soil cores within each plot (5 stations per plot) were combined into a pooled sample. We air dried soil samples and then pulverized them to a powder using a Shatterbox grinding mill. We then weighed soil samples (15–20 mg) and packed them into tins. Samples were analyzed for total carbon in triplicate (three soil tins for each sample) on a Costech Elemental Combustion System 4010. Because these soils did not contain carbonates, we express these data as percent SOC.
Crop Yield
Across Management Treatments
Crops were harvested from the entire crop area of each plot across all agronomic treatments. Prairie strips were not harvested, but we area-scaled yields in the reduced input and biologically based treatments by reducing yields 5% to account for area in strips. Wheat was harvested from conventional and no till plots on July 24, 2019 and from reduced input and biologically based plots on July 25, 2019. Maize was harvested from all treatments and plots on October 29, 2020. Crops were harvested with a harvest combine, and yield for the entire crop area of each plot was measured with a weigh wagon. We report yield as kg/ha at crop harvest at standard moisture content (13% for wheat, 15.5% for maize). We did not compare yield with the conservation land treatment, although perennial grasslands have potential to be harvested for bioenergy (Robertson et al., 2017).
In addition to measuring crop yields for 2019 and 2020, we separately compared historical crop yields in the same plots prior to the sowing of prairie strips. We used yield measurements from 2013 to 2018—two cycles of the crop rotation prior to prairie strips.
Statistical Analyses
Across Management Treatments
We aggregated the individuals of our measures of invertebrate biodiversity (ants, butterflies, dung beetles, and spiders) surveyed over each year within each plot of each treatment (six plots for each of five treatments) by summing. For all measures of biodiversity and ecosystem services, we calculated effect sizes of no till, reduced input, biologically based, and conservation land treatments relative to the conventional treatment. We measured the Hedge’s g effect size and 95% confidence intervals using the “compute.es” package in R (Del Re, 2013). The conventional treatment served as the baseline, which does not include a confidence interval.
To determine the differences in arthropod richness (ants, butterflies, dung beetles, and spiders) across treatments, we used generalized linear mixed effects models with normal distributions. All model assumptions were met. Richness was used as the response variable; main effects were treatment and year (except for ants which were sampled in only one year), and the random effect was experimental block. We calculated the estimated species richness of butterflies within each replicate of each treatment per year using the R package “iNext” with Chao1 abundance-based rarefaction (Chao et al., 2014; Hsieh et al., 2016). We used measures of raw richness for ants, dung beetles, and spiders; we recognize that abundance affects richness for these measures, but because our abundances were low, rarefaction was not possible.
To determine the differences in arthropod abundances across treatments, we used generalized linear mixed effects models constructed similarly but with negative binomial distributions (except for ants for which we used a normal distribution to meet model assumptions). R package “lme4” was used to construct the models (Bates et al., 2015). An ANOVA followed by a Tukey test was used for post hoc analyses for all models using R packages “car” (Fox and Weisberg, 2019) and “multcomp,” respectively (Hothorn et al., 2008). The same method was used for all measured ecosystem services including crop yield (except GWI), but with the measure of the service as the response variable and sampling round included as a fixed effect when multiple sampling rounds occurred within a year (decomposition and pollination). We also modeled crop yield independently for each year using the same method to measure relative yields among treatments for each crop. For GWI, we constructed a generalized linear mixed effects model with log transformed CO2 as the response variable, treatment, year, sampling round, and temperature as fixed effects, and experimental block as the random effect.
Distances From Prairie Strips
We aggregated the individuals of our measures of invertebrate biodiversity (ants, dung beetles, and spiders) collected over each year within each distance of each plot of each treatment (four distances for each of six plots for each of two treatments) by summing. To determine the effect of distance from prairie strip and year on measures of arthropod richness (ants, dung beetles, and spiders), we constructed a generalized linear mixed effects model with a normal distribution. Richness was the response variable, crop management treatment, distance from prairie strip, and year were fixed effects with an interaction between distance and year, and experimental block was a random effect. We followed this with an ANOVA. The same method was used for measures of abundance, but with a negative binominal distribution (except ants for which we used a normal distribution). We constructed similar models with normal distributions for measures of ecosystem services but included sampling round as a fixed effect when relevant (decomposition and pollination). Distance was treated as a continuous variable. To test if trends were occurring across distances from the prairie strips, or if they were driven solely by high values within the prairie strips, we also ran analyses for all measures with datapoints at 0 m removed.
Results
Dung Beetles
Across Management Treatments
We collected a total of 553 dung beetles in Standard Sampling Plots (Supplementary Table 2). The effect sizes of dung beetle richness across all treatments did not differ from baseline (Figure 2). Species richness did not differ among treatments, however, dung beetle abundance was 128–992% higher in conservation land than in all other treatments (Figure 3 and Table 1).
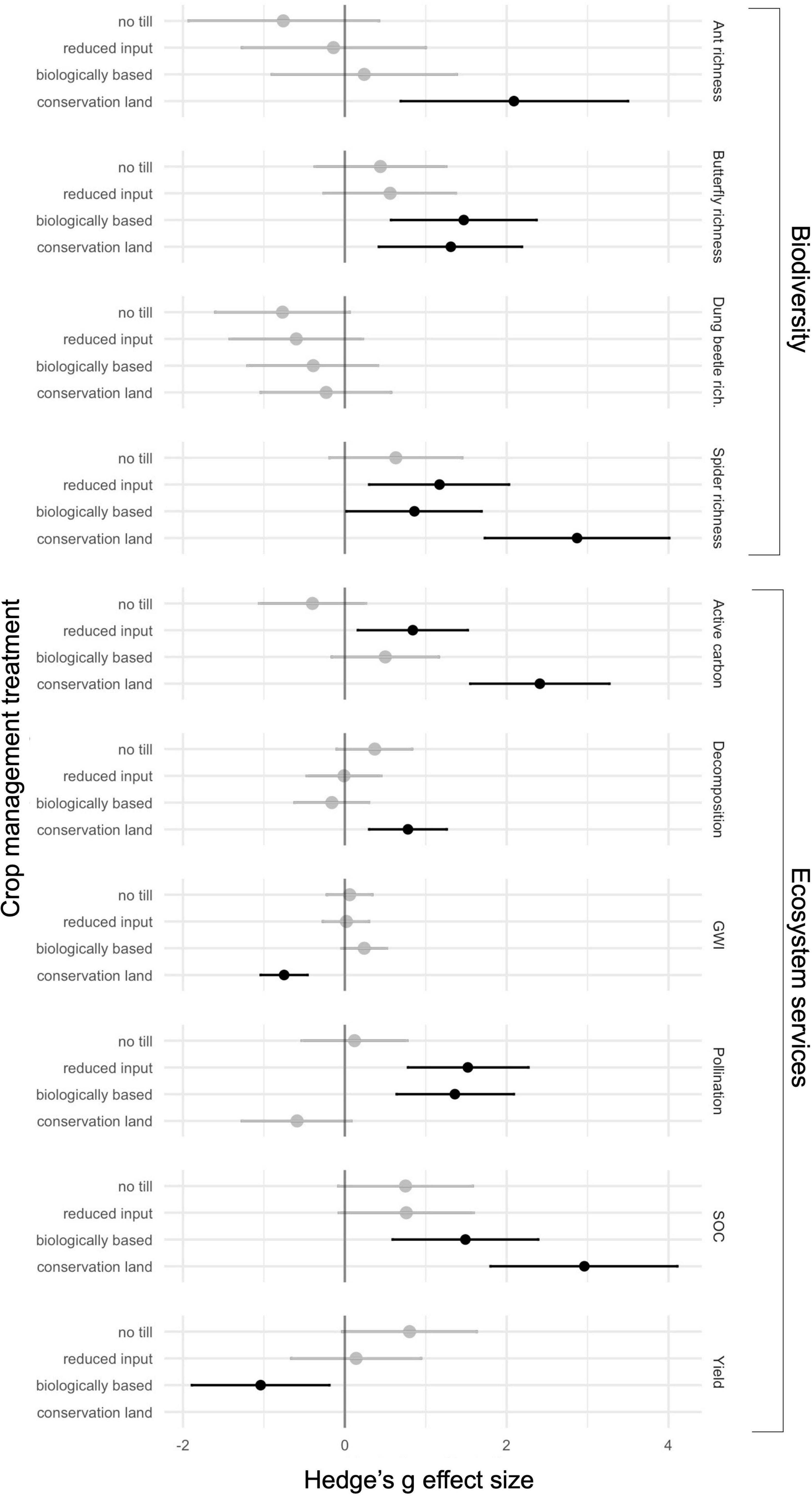
Figure 2. Hedge’s g effect size (black and gray dots) with 95% confidence interval. The baseline is the conventional management treatment. Negative values are effect sizes lower than that of the baseline, and positive values are effect sizes higher than the baseline. Values that cross zero are shaded gray. Note that lower levels of global warming impact (GWI) would be a more positive ecosystem service. Conservation land was not harvested, therefore crop yield is not applicable for that treatment.
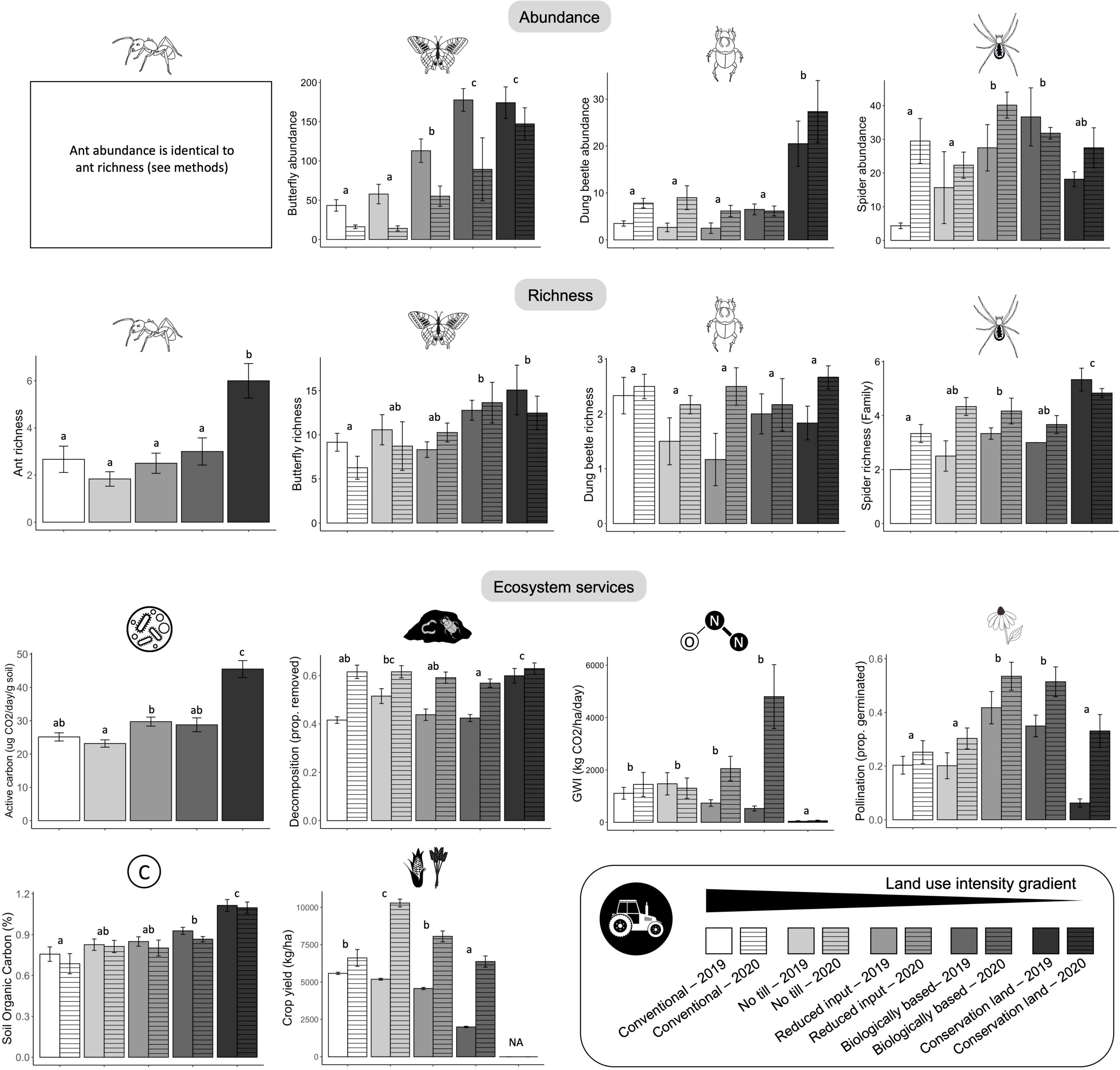
Figure 3. Effects of crop management treatment on measures of biodiversity and ecosystem services between years 2019 and 2020. For measures of biodiversity, bars depict means over an entire year; for measures of ecosystem services, bars depict means for each sampling round. Letters denote statistical differences among treatments. Error bars show standard errors. Ants and active carbon were only measured in 2019.
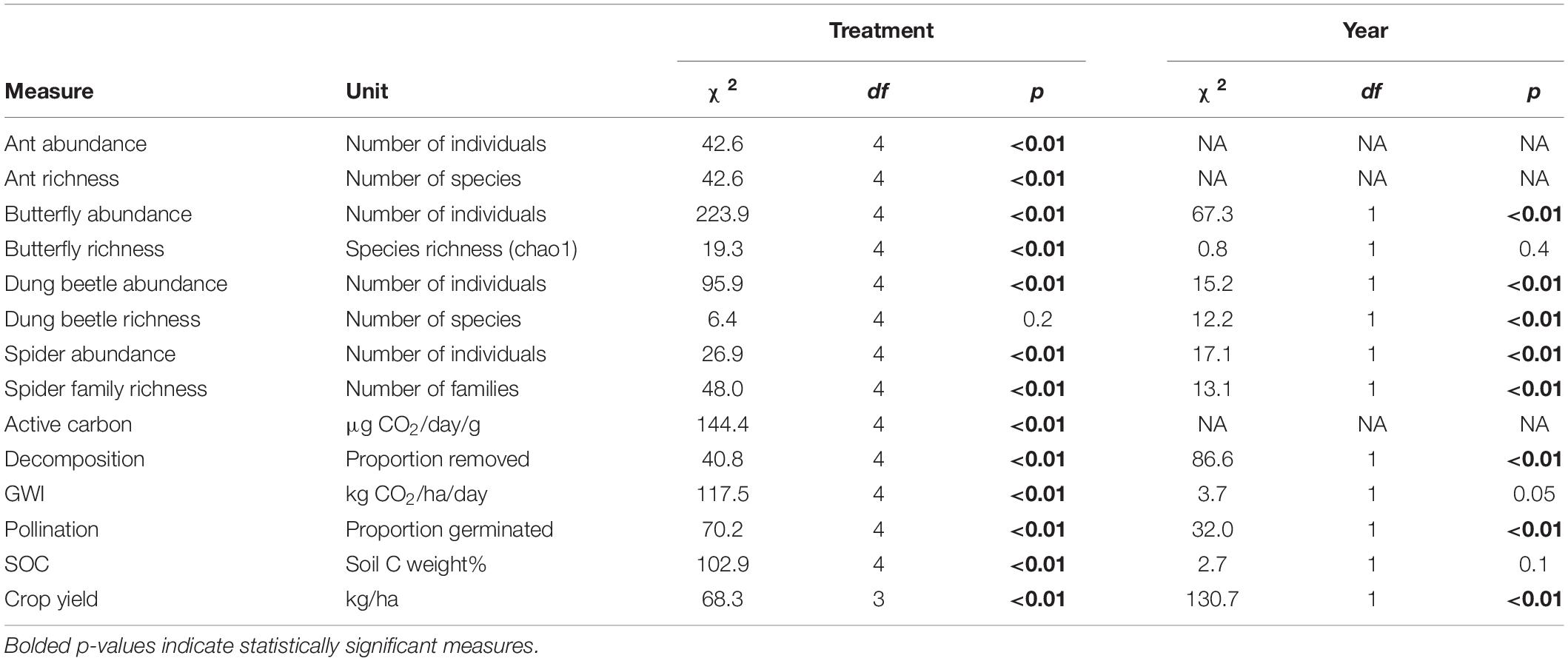
Table 1. Effects of crop management treatments on measures of biodiversity and ecosystem services treatments.
Distances From Prairie Strips
We collected a total of 284 dung beetles at Strip Sampling Stations (Supplementary Table 2). There was no relationship between distance and dung beetle richness, but dung beetle richness was higher in 2020 than in 2019 (Figure 4 and Table 2). There was an interaction between year and distance from prairie strip for dung beetle abundance, with 2019 having no relationship with distance from strip and 2020 abundance decreasing with distance from strip (Figure 4 and Table 2). When 0 m samples were removed, there was no effect of distance or year on dung beetle abundance (distance: χ2 = 0.6, df = 1, p = 0.4; year: χ2 = 0.5, df = 1, p = 0.8) or richness (distance: χ2 = 0.4, df = 1, p = 0.53; year: χ2 = 1.7, df = 1, p = 0.2), meaning the linear trend of abundance was driven by high dung beetle abundance in the prairie strips.
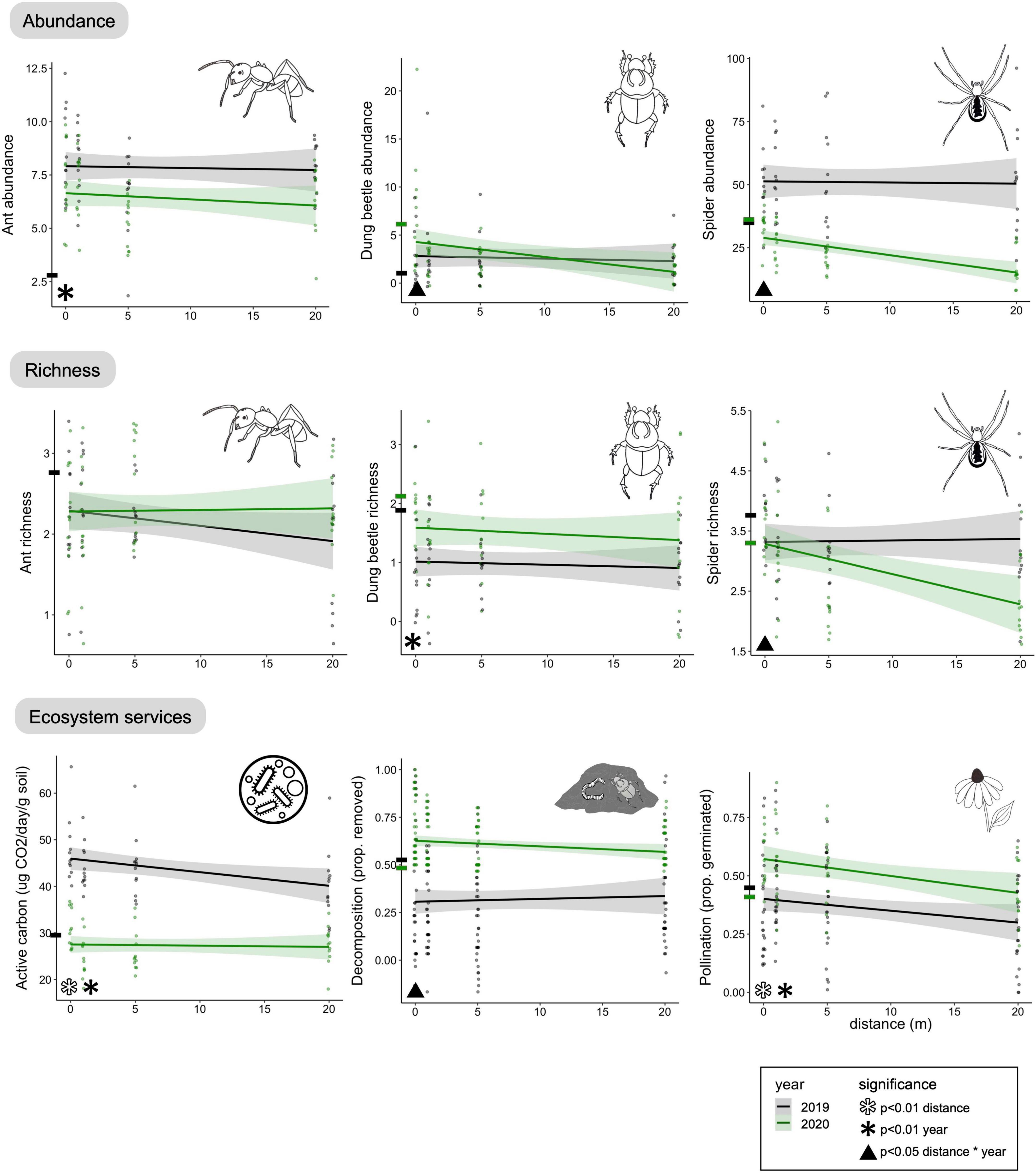
Figure 4. Effects of distance from prairie strips on measures of biodiversity and ecosystem services in both reduced input and biologically based treatments. We considered linear effects of distance from prairie strips. The interaction with year is presented, with 2019 being the first year of prairie strip implementation and a wheat year, and 2020 being the second year and a maize year. Distance 0 m is within the prairie strip. Black dashes on the y-axis represent the mean of the plot level values for reduced input and biologically based for that measure in 2019; green dashes represent 2020. Ant data from 2019 is modified from Helms et al. (2021).
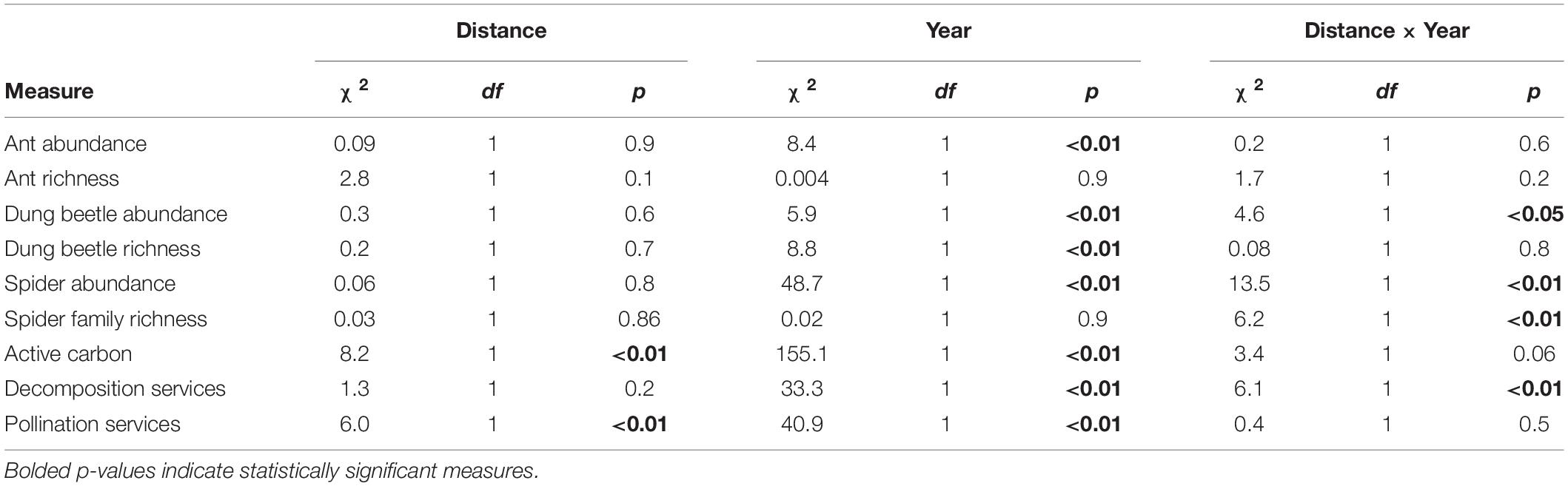
Table 2. Effects of crop management treatments on measures of biodiversity and ecosystem services treatments.
Ants
Across Management Treatments
We collected a total of 1821 worker ants from the Subplot Sampling Stations (Supplementary Table 3). The Hedge’s g effect sizes of ant richness in conservation land were higher than the baseline (Figure 2). Species richness was higher in conservation land treatments than in all row crop treatments (Figure 3 and Table 1). Ant abundance was the same as ant richness because abundance of any particular species could only be 0 or 1 at a single sampling station, and sampling stations were summed per plot per year (see methods).
Distances From Prairie Strips
We collected a total of 3218 ants from the Strip Sampling Stations (Supplementary Table 3). Ant species richness did not vary by year nor with distance from the prairie strip (Figure 4 and Table 2). Ant abundance did not differ by distance from prairie strip, but differed by year, decreasing from 2019 to 2020 (Figure 4 and Table 2).
Spiders
Across Management Treatments
We collected a total of 1522 spiders from Subplot Sampling Stations (Supplementary Table 4). The effect size of spider richness for treatments with prairie strips and the conservation land treatment were higher than baseline; no till did not differ from the baseline (Figure 2). Spider richness increased from conventional to no till and biologically based, to reduced input, to conservation land (Figure 3 and Table 1). Spider abundance was highest in the treatments with prairie strips followed by conservation land and no till, and lowest in conventional (Figure 3 and Table 1).
Distances From Prairie Strips
We collected a total of 3626 spiders from Strips Sampling Stations (Supplementary Table 4). There was an interaction between distance from prairie strip and year to explain spider richness and abundance, with 2019 having no change in richness and abundance with increasing distance and 2020 having a decrease in richness with increasing distance (Figure 4 and Table 2).
Butterflies
Across Management Treatments
We visually identified 5329 butterflies during transect counts (Supplementary Table 5). The effect sizes of butterfly richness were higher than the baseline of conventional in the biologically based and conservation land treatments; no till and reduced input did not differ from baseline (Figure 2). Butterfly richness was the highest in the conservation land and biologically based treatments followed by reduced input and no till treatments, and lowest in conventional (Figure 3 and Table 1). Giant Swallowtails, Checkered Skippers, and Red Spotted Purples were species identified in treatments with prairie strips that were never observed in conventional or no till treatments. Butterfly abundance was 134–349% higher in treatments with prairie strips than crop treatments without prairie strips (conventional and no till; Figure 3).
Active Carbon
Across Management Treatments
The effect sizes of active carbon in the reduced input and the conservation land treatments were higher than the baseline; no till and biologically based did not differ from the baseline (Figure 2). Active carbon was highest in the conservation land treatment, lowest in the no till treatment, and intermediate in the reduced input, biologically based, and conventional (Figure 3 and Table 1).
Distances From Prairie Strips
Distance from prairie strip and year had significant effects on active carbon, with 2019 having higher active carbon than 2020, and 2019 having a decrease in active carbon with distance from prairie strip (Figure 4 and Table 2). In the crop area alone (0 m datapoints removed from analyses), there was a significant interaction between distance and year with a decrease in active carbon with increasing distance from prairie strips in 2019 and no change with distance from prairie strip in 2020 (χ2 = 4.9, df = 1, p < 0.05).
Decomposition
Across Management Treatments
The effect size of decomposition in conservation land was higher than the baseline; no other treatment differed from the baseline (Figure 2). Conservation land and no till treatments had the highest rates of decomposition, followed by conventional and reduced input, and then by biologically based (Figure 3 and Table 1).
Distances From Prairie Strips
There was an interaction between year and distance from prairie strip, with 2019 having a lower rate of decomposition and no change with distance from prairie strip and 2020 having a higher rate of decomposition with a decreasing trend with increasing distance from prairie strip (Figure 4 and Table 2).
Global Warming Impact
Across Management Treatments
The effect size of GWI in conservation land was lower than the baseline; no other treatments differed from the baseline (Figure 2). GWI in the conservation land treatment was at least 25× lower than all other treatments (Figure 3 and Table 1). Methane tended to be consumed in the soils rather than emitted to the atmosphere across all land use types. Fluxes in conservation land across 2019 and 2020 were lowest at −2.68 ± 2.46 (mean ± SD) g CH4-C ha–1 day–1, while those in the biologically based treatment were highest at −0.79 ± 1.78 g CH4-C ha–1 day–1. Similarly, N2O fluxes were lowest in conservation land and highest in the biologically based treatment at 0.34 ± 0.51 and 7.27 ± 17.13 g N2O-N ha–1 day–1, respectively.
Pollination
Across Management Treatments
The effect size of pollination was higher in reduced input and biologically based treatments than the baseline (Figure 2). Pollination rates were 72–222% higher in the treatments with prairie strips than all other treatments (Figure 3 and Table 1).
Distances From Prairie Strips
Pollination services decreased with distance from prairie strip, and pollination services increased from 2019 to 2020 (Figure 4 and Table 2).
Soil Organic Carbon
Across Management Treatments
Soil organic carbon was higher than baseline in biologically based and conservation land treatments (Figure 2). SOC was lowest in conventional, intermediate in the no till, reduced input, and biologically based treatments, and highest in conservation land treatment (Figure 3 and Table 1).
Crop Yield
Across Management Treatments
The effect size was lower than baseline in the biologically based treatment but did not differ from the baseline for no till and reduced input treatments (Figure 2). When including both wheat and maize in analyses, crop yield was highest in the no till treatment, intermediate in the conventional and reduced input treatments, and lowest in the biologically based treatment (Figure 3 and Table 1). Crop yield was scaled to include the land area of prairie strips in analyses (kg/ha measurements include area of the prairie strips). These relative crop yields across treatments were consistent with the previous two crop rotations where, across all the whole crop rotation, crop yields were highest in no till, intermediate in conventional and reduced input (although conventional and no till were not significantly different), and lowest in the biologically based treatment.
When considering the wheat and maize years independently, the pattern among treatments changed from the previous two crop rotations. When measuring just the wheat year (2019), crop yield was lowest in the biologically based, followed by the reduced input treatment, then no till, then conventional (χ2 = 1921.2, df = 3, p ≤ 0.01). Historically (2013–2017), however, wheat yield was lowest in biologically based but did not differ among other crop treatments. When measuring just the maize year (2020), crop yield was lowest in the biologically based and conventional, intermediate in the reduced input, and highest in no till (χ2 = 70.3, df = 3, p < 0.01). This also differs from the previous two crop rotations, where maize yield was lowest in biologically based, intermediate in reduced input and conventional, and highest in no till. Therefore, maize yields in the conventional treatment in 2020 were low compared to the previous two crop rotations. The third crop in the rotation, soy, historically was lowest in conventional and biologically based and highest in no till and reduced input.
Discussion
We show that prairie strips, even early in their establishment, combined with lower land use intensity can promote biodiversity and ecosystem services without compromising crop yield. Within reduced input and biologically based treatments, where sampling effort occurred within prairie strips and at increasing distance from strips, biodiversity and ecosystem services spilled over into agronomic areas for five out of six measures. Among all treatments, using data from the entire plot area (not sampling within prairie strips for treatments with strips), pollination services and the abundance of butterflies and spiders were higher in plots with prairie strips. In addition, soil organic carbon, butterfly richness, and spider richness increased with a decrease in land use intensity. Crop yield in the reduced input treatment was equal to that of conventional management, even while including the area taken out of production. These effects were evident early in strip establishment, during which prairie strip plant communities changed from mostly weeds to a diversity of planted species. We expect the effects of strips to grow over time as native plants establish and become more abundant, and as lagged effects of historic agronomic disturbances abate.
The benefits of prairie strips decreased with distance into cropland for spider abundance and richness, dung beetle abundance, active carbon, decomposition, and pollination (Figure 4). Each of these responses decreased with distance from the prairie strip, but did so by different mechanisms. For spiders, the spillover into cropland was delayed one year after the prairie strips were sown. Prairie strips provided new habitat and sources of prey for spiders (Hussain et al., 2021), such that spiders could move into cropland to capture additional prey. The impact on dung beetles and decomposition are likely due to the prairie strips harboring dung beetles in the year after restoration, which in turn caused a higher rate of manure removal near the prairie strips and a decrease in dung beetle abundance and manure removal with increasing distance from the strips (Manning and Cutler, 2018). For pollination, prairie strips increased floral resources which attracted pollinators and then exported them into the surrounding habitat (Garibaldi et al., 2011). We were surprised that prairie strips increased pollination as our plot sizes are a fraction of pollinator foraging range (Ricketts et al., 2008), and we suspect that pollinators were attracted to the high concentration of resources that contrasted strongly with crops. For active carbon, the effect of distance from prairie strip may have been driven by high prairie litter inputs. Mowing prairie strips in 2019 may have caused a spillover of prairie litter inputs into cropland that did not occur in 2020, leading to higher levels of active carbon in crop soils immediately adjacent to prairie strips. While the increase of active carbon in nearby sites was subtle, it still highlights the biogeochemical benefits of adjacent prairies for agricultural lands, which are generally underappreciated (Pérez-Suárez et al., 2014).
Our study shows that spillover effects from prairie strips extend across measures of biodiversity and ecosystem services. Pollinators and pollination services have been studied at distances from restorations and habitat edges, often with higher numbers of pollinators and rates of pollination near non-crop habitat (Ricketts, 2004; Carvalheiro et al., 2010; Kordbacheh et al., 2020); by also demonstrating this phenomenon in dung beetles, spiders, active carbon, and decomposition, we show that these spatial effects apply to a broader array of organisms and ecosystem services. These results, with detailed attention to mechanism in our controlled experiment, strengthen evidence that suggests that strategic placement and amount of natural habitat in agricultural landscapes can add both conservation value and ecosystem services to an agricultural landscape (Basso et al., 2019; Mitchell et al., 2021). Our study focused on ecosystem services, and we did not measure potential ecosystem disservices from prairie strips that could impact yield, such as herbivory, however, such disservices could be addressed in future studies.
Prairie strips require several years after planting to resemble a restored prairie community, over which time diversity and ecosystem services have been shown to accrue (Kurtz, 2013; Griffin et al., 2017). Supporting this, we found that year since prairie strip establishment affected all responses that we measured at distances from prairie strips except ant richness (Figure 4). Lack of response of ant species richness is consistent with other grassland restoration projects where it takes several years for ant communities to turnover (Dauber and Wolters, 2005; Menke et al., 2015; Hussain et al., 2021; Scharnhorst et al., 2021). With the exception of active carbon, variables displayed a general progression of the first year having small to no effect of distance from prairie strip, to the second year showing a stronger negative effect of distance. It is important to note that year differences in our experiment are confounded by crop type (wheat or maize). These effects will become easier to separate from effects of prairie strips as measurements are repeated over the next 4 years (after two full crop rotations) and then in seven years (one full rotation after strip maturation). We expect that as more plant species establish, prairie strips will increase biodiversity even further.
Prairie strips are also likely to continue to increase the provision of soil services; for example, we found higher levels of active carbon and SOC in the prairie strips in this study, with potential for these benefits to extend into the cropland at short distances. Over time, prairie strips could thus be a significant carbon sink, which could provide benefits to agricultural landscapes and may come with economic reward with future carbon pricing. Quantifying the aggregated potential of this sequestration should be a priority, and continued measurement of these variables in our study after the early establishment phase of prairie strips will provide insight to their long-term potential for conservation and impact on crop yield, and more comprehensive opportunities for synergies.
Across all crop management treatments, there was a variable effect of land use intensity on measures of biodiversity (Figure 3). Conservation land consistently had the highest level of richness, and often had the highest level of ecosystem services. We found that lower land use intensity treatments with prairie strips increased butterfly abundance and pollination services compared to other crop management schemes. This may be the consequence of pollinators and butterflies being mobile agents with large ranges. They can therefore find and utilize the resources of prairie strips early in strip establishment (Cant et al., 2005; Pasquet et al., 2008). In addition to the prairie strips, reduced input and biologically based treatments have reduced pesticides which likely contributes to increased butterfly richness and pollination services; but the decreasing pollination services with distance from the prairie strips suggests that pollinators are attracted to the strips. Butterflies were most diverse in the conservation land treatment due to the increased floral and habitat diversity throughout the plot (Menéndez et al., 2007). We attribute the low level of pollination services in the conservation land treatment, especially in 2019, to the forager dilution effect, in which pollination services are diluted in an area of mass flowering (Holzschuh et al., 2011). While butterflies and pollination services do not improve the yield of wheat or maize crops, they may improve yield in soy crops (Cunningham-Minnick et al., 2019) or other crops in the landscapes that benefit from pollinators, and the potential of these services remain among the most important to surveyed farmers (Arbuckle, 2019; Hevia et al., 2021).
Our hypotheses of increasing biodiversity with a decrease in land use intensity were not supported uniformly. For ground dwelling arthropods, conventional management had surprisingly high species richness. This finding is not without precedent; despite previous findings that organic farms support more biodiversity than conventional farms (Bengtsson et al., 2005; Tuck et al., 2014), ants and dung beetles show mixed results (Hutton and Giller, 2003; Jones et al., 2019; Piccini et al., 2019; Helms et al., 2021). In addition, while diversifying farms generally increases spider diversity (Schmidt et al., 2005), prairie strips in our study have not increased spider diversity at the plot level, but have increased spider abundance.
Soil-related ecosystem services (active carbon, decomposition, GWI, and SOC) across the land use intensity gradient were highest in conservation lands, with variable differences among cropping treatments. Decomposition was highest in untilled treatments (no till and conservation land), possibly due to the higher microbial activity and soil moisture maintained by soil aggregates with greater physical protection (Paul et al., 2015), or due to mesofaunal differences that we did not measure, such as earthworm abundance (Smith et al., 2008). As strips develop, we expect decomposition to increase with dung beetle abundance and diversity in prairie strip treatments (Hosler et al., 2021). Conservation land had increased active carbon likely due to the fine root production of diverse perennial vegetation, which we also expect to increase in and near prairie strips as they mature (Sprunger et al., 2017; Sprunger and Robertson, 2018). The increased SOC along the land use intensity gradient was likely due to carbon from perennial plants (Syswerda et al., 2011; Mosier et al., 2021). GWI was almost entirely driven by N2O in our study. The reduced GWI in the conservation land treatment was likely due to reduced fertilizer inputs. Although there was no difference in overall GWI across row crop management treatments, as Gelfand et al. (2016) has also found for N2O emissions, there was higher GWI in the wheat year, compared to maize. This was likely due to several days of tillage early in the growing season for biologically based maize, management that is different from other treatments that receive alternative methods of weed management. We note that GWI only represents soil emissions and is not a full life cycle analysis.
The potential for prairie strips to enhance biodiversity and ecosystem services at large scales will be most powerful if they do not sacrifice agricultural yield. Historically in our experiment, the no till treatment has the highest yield, followed by the conventional and reduced input treatments with intermediate yields, and the biologically based treatment with the lowest yield. We show that converting 5% of crop area to prairie strips (and using yield measures that include the area taken out of production) does not change differences in yield across treatments beyond differences already induced by existing management. Reduced input management with prairie strips maintained a high yield, equivalent to conventional, while having high levels of pollination, spider abundance, and butterfly richness; biologically based crop management with prairie strips similarly maximized these services, but at the cost of a large cut in yields. This reduction was likely due to limitation of inorganic nitrogen (Robertson et al., 2015), unrelated to prairie strips. While no single method of crop management performed highest or lowest across all measures (e.g., no till treatments had the highest yield but did not have the highest levels of biodiversity or other ecosystem services), there was a synergy among crop yield, biodiversity, and non-provisioning ecosystem services in the reduced input treatment. Prairie strips are an effective conservation practice that can be combined with other techniques—reduced fertilizer and pesticides—to conserve biodiversity and ecosystem services without compromising crop yield.
When considering both 2019 and 2020 yields together, yield in reduced input treatments may have remained equivalent to yield in conventional treatments for at least three reasons. First, converting 5% of cropland to prairie was not enough area to result in significant changes among treatments. Second, ecosystem services generated by strips could increase yield in the remainder of the plot. Several of the responses we measured were higher at the plot scale outside of prairie strips. For example, relative to conventional treatments, active carbon was higher in reduced input treatments, and SOC trended toward higher (Figure 2). Third, climate or other environmental conditions during the time of our study could have had stronger negative impact in conventional treatments. As the strips mature, and with results through multiple rotations, the mechanism of yield response will become clearer.
Prairie strips were implemented on land that had previously been in crop production in this experiment, but prairie strips could also be implemented on the landscape in a way that does not reduce farm profitability. For instance, prairie strips can be strategically placed on marginal land—land that has consistent low yields relative to nutrient and greenhouse gas inputs. Marginal land occupies 26% of annual cropland land in the US Midwest, resulting in excessive pollution and wasted monetary and nutrient resources (Basso et al., 2019). Converting marginal cropland to prairie strips would reduce inputs without sacrificing crop yield. In addition, prairie strips could be harvested as perennial biofuel for added profitability, and we suggest future studies address how this would impact biodiversity and ecosystem services. Future studies might also examine how the optimal benefits of prairie strips could be achieved with strategic location, particularly cropland that is consistently underperforming and therefore not as profitable, on slopes to prevent soil erosion, or in locations that could increase habitat connectivity in the broader landscape (Basso, 2021).
We expect that as prairie strips mature their effects on biodiversity and ecosystem services will grow. We converted just 5% of cropland to prairie, however, optimal benefit may be achieved by even larger strips, such as the 10% conversion of cropland to prairie as recommended by Schulte et al. (2017). Our study supports that prairie strips are an effective strategy for conserving biodiversity, and can in some cases be created without impacting crop yield in the US Midwest. More broadly, diversifying agricultural landscapes can help mitigate the loss of biodiversity and ecosystem services while supporting the growing human population.
Data Availability Statement
The data supporting the conclusions of this article are available at: doi: 10.5281/zenodo.6497814. Any further requests should be directed to the corresponding author.
Author Contributions
LK, NH, SE, JH, and CR designed research. LK, CR, JH, JS, and EC-O conducted research and collected data in addition to other researchers. LK, CR, JH, CV, and JVC analyzed data and results. LK led the synthesis and writing. All authors contributed to manuscript drafts, contributed to the article, and approved the submitted version.
Funding
Support for this research was provided by the NSF Long-Term Ecological Research Program (DEB 1832042) at the Kellogg Biological Station and by Michigan State University AgBioResearch. Support for this research was also provided by the Great Lakes Bioenergy Research Center, United States Department of Energy, Office of Science, Office of Biological and Environmental Research (Award DE-SC0018409). This research was also partially funded by the Kellogg Biological Station, the Michigan State University Graduate School, and the Michigan State University Ecology, Evolution, and Behavior graduate program.
Conflict of Interest
The authors declare that the research was conducted in the absence of any commercial or financial relationships that could be construed as a potential conflict of interest.
Publisher’s Note
All claims expressed in this article are solely those of the authors and do not necessarily represent those of their affiliated organizations, or those of the publisher, the editors and the reviewers. Any product that may be evaluated in this article, or claim that may be made by its manufacturer, is not guaranteed or endorsed by the publisher.
Acknowledgments
We thank Stacey VanderWulp and Mark Hammond for project coordination, Sven Bohm and Hsun-yi Hsieh for data management, and Kevin Kahmark and Joe Simmons for fieldwork management. We thank Doug Landis for efforts to create prairie strip treatments. We thank Alexandra Brown, Michaela Rose, Oren Lerner, and other field technicians for assistance with data collection and processing. We thank Phil Robertson, Will Wetzel, and Sarah Fitzpatrick for constructive review of these experiments and this manuscript. We also appreciate the insightful feedback from two reviewers. We acknowledge the W.K. Kellogg Biological Station’s LTER Site, where this work was conducted, occupies the ancestral land of the Peoria Tribe and the ancestral, traditional, and contemporary Lands of the Anishinaabeg–Three Fires Confederacy of Ojibwe, Odawa, and Potawatomi peoples. We honor with gratitude the land itself and the people who have stewarded it through the generations. This is Kellogg Biological Station Contribution no. 2313.
Supplementary Material
The Supplementary Material for this article can be found online at: https://www.frontiersin.org/articles/10.3389/fevo.2022.833170/full#supplementary-material
References
Agosti, D., Majer, J. D., Alonso, L. E., and Schultz, T. R. (2000). Ants: Standard Methods for Measuring and Monitoring Biodiversity. Washington, D.C: Smithsonian Institution Press.
Arbuckle, J. G. (2019). STRIPS Cooperator Follow-On Survey: 2018 Results. Sociology Technical Report No. 1057. Ames, IA: Iowa State University Extension Sociology.
Asbjornsen, H., Hernandez-Santana, V., Liebman, M., Bayala, J., Chen, J., Helmers, M., et al. (2013). Targeting perennial vegetation in agricultural landscapes for enhancing ecosystem services. Renew. Agric. Food Syst. 29, 101–125. doi: 10.1017/S1742170512000385
Barbero, E., Palestrini, C., and Rolando, A. (1999). Dung beetle conservation: effects of habitat and resource selection (Coleoptera: Scarabaeoidea). J. Insect Conserv. 3, 75–84.
Barker, T., Bashmakov, I., Bernstein, L., Bogner, J. E., Bosch, P. R., Dave, R., et al. (2007). “Technical summary,” in Climate Change 2007: Mitigation. Contribution of Working Group III to the Fourth Assessment Report of the Intergovernmental Panel on Climate Change, eds B. Metz, O. R. Davidson, P. R. Bosch, R. Dave, and L. A. Meyer (New York, NY: Cambridge University Press), 25–93.
Barrios, E. (2007). Soil biota, ecosystem services and land productivity. Ecol. Econ. 64, 269–285. doi: 10.1016/j.ecolecon.2007.03.004
Basso, B. (2021). Precision conservation for a changing climate. Nat. Food 2, 322–323. doi: 10.1038/s43016-021-00283-z
Basso, B., Shuai, G., Zhang, J., and Robertson, G. P. (2019). Yield stability analysis reveals sources of large-scale nitrogen loss from the US Midwest. Sci. Rep. 9:5774. doi: 10.1038/s41598-019-42271-1
Bates, D., Mächler, M., Bolker, B., and Walker, S. (2015). Fitting linear mixed-effects models using lme4. J. Stat. Softw. 67, 1–48. doi: 10.18637/jss.v067.i01
Benckiser, G. (2010). Ants and sustainable agriculture. A review. Agron. Sustain. Dev. 30, 191–199. doi: 10.1051/agro/2009026
Bengtsson, J., Ahnström, J., and Weibull, A. C. (2005). The effects of organic agriculture on biodiversity and abundance: a meta-analysis: organic agriculture, biodiversity and abundance. J. Appl. Ecol. 42, 261–269. doi: 10.1111/j.1365-2664.2005.01005.x
Blitzer, E. J., Dormann, C. F., Holzschuh, A., Klein, A.-M., Rand, T. A., and Tscharntke, T. (2012). Spillover of functionally important organisms between managed and natural habitats. Agric. Ecosyst. Environ. 146, 34–43. doi: 10.1016/j.agee.2011.09.005
Bowers, C., Toews, M. D., and Schmidt, J. M. (2021). Winter cover crops shape early-season predator communities and trophic interactions. Ecosphere 12:e03635. doi: 10.1002/ecs2.3635
Bowles, T. M., Jackson, L. E., Loeher, M., and Cavagnaro, T. R. (2016). Ecological intensification and arbuscular mycorrhizas: a meta-analysis of tillage and cover crop effects. J. Appl. Ecol. 54, 1785–1793. doi: 10.1111/1365-2664.12815
Campbell, B. M., Beare, D. J., Bennett, E. M., Hall-Spencer, J. M., Ingram, J. S. I., Jaramillo, F., et al. (2017). Agriculture production as a major driver of the Earth system exceeding planetary boundaries. Ecol. Soc. 22:8. doi: 10.5751/ES-09595-220408
Cant, E. T., Smith, A. D., Reynolds, D. R., and Osborne, J. L. (2005). Tracking butterfly flight paths across the landscape with harmonic radar. Proc. R. Soc. B Biol. Sci. 272, 785–790. doi: 10.1098/rspb.2004.3002
Carvalheiro, L. G., Seymour, C. L., Veldtman, R., and Nicolson, S. W. (2010). Pollination services decline with distance from natural habitat even in biodiversity-rich areas: sub-tropics crop pollination limitation. J. Appl. Ecol. 47, 810–820. doi: 10.1111/j.1365-2664.2010.01829.x
Chao, A., Gotelli, N. J., Hsieh, T. C., Sander, E. L., Ma, K. H., Colwell, R. K., et al. (2014). Rarefaction and extrapolation with Hill numbers: a framework for sampling and estimation in species diversity studies. Ecol. Monogr. 84, 45–67. doi: 10.1890/13-0133.1
Coleman, D. C., Callaham, M. A. Jr., and Crossley, D. A. Jr. (eds). (2018a). “Chapter 4 – Secondary production: activities of heterotrophic organisms—the soil fauna,” in Fundamentals of Soil Ecology, 3rd Edn, (Cambridge, MA: Academic press), 77–171. doi: 10.1016/B978-0-12-805251-8.00003-X
Coleman, D. C., Callaham, M. A. Jr., and Crossley, D. A. Jr. (eds). (2018b). “Chapter 3 – Secondary production: activities of heterotrophic organisms—microbes,” in Fundamentals of Soil Ecology, 3rd Edn, (Cambridge, MA: Academic press), 47–76.
Coleman, D. C., Callaham, M. A. Jr., and Crossley, D. A. Jr. (eds). (2018c). “Chapter 1 – Introduction to soil: historical overview, soil science basics, and the fitness of the soil environment,” in Fundamentals of Soil Ecology, 3rd Edn, (Cambridge, MA: Academic press), 1–20. doi: 10.1016/B978-0-12-805251-8.00001-6
Culman, S., Snapp, S. S., Freeman, M. A., Schipanski, M. E., Beniston, J., Lal, R., et al. (2012). Permanganate oxidizable carbon reflects a processed soil fraction that is sensitive to management. Soil Sci. Soc. Am. J. 76, 494–504. doi: 10.2136/sssaj2011.0286
Cunningham-Minnick, M. J., Peters, V. E., and Crist, T. O. (2019). Nesting habitat enhancement for wild bees within soybean fields increases crop production. Apidologie 50, 833–844. doi: 10.1007/s13592-019-00691-y
Dauber, J., and Wolters, V. (2005). Colonization of temperate grassland by ants. Basic Appl. Ecol. 6, 83–91. doi: 10.1016/j.baae.2004.09.011
de Pedro, L., Perera-Fernández, L. G., López-Gallego, E., Pérez-Marcos, M., and Sanchez, J. A. (2020). The effect of cover crops on the biodiversity and abundance of ground-dwelling arthropods in a Mediterranean pear orchard. Agronomy 10:580. doi: 10.3390/agronomy10040580
Del Re, A. C. (2013). compute.es: Compute Effect Sizes. Available online at: https://cran.r-project.org/web/packages/compute.es/index.html (accessed August 1, 2021).
Ellison, A. M., Gotelli, N. J., Farnsworth, E. J., and Alpert, G. D. (2012). A Field Guide to the Ants of New England. New Haven: Yale University Press.
Ellison, A. M., Record, S., Arguello, A., and Gotelli, N. J. (2007). Rapid inventory of the ant assemblage in a temperate hardwood forest: species composition and assessment of sampling methods. Environ. Entomol. 36, 766–75. doi: 10.1603/0046-225X(2007)36[766:RIOTAA]2.0.CO;2
Folgarait, P. J. (1998). Ant biodiversity and its relationship to ecosystem functioning: a review. Biodivers. Conserv. 7, 1221–1244. doi: 10.1023/A:1008891901953
Fox, J., and Weisberg, S. (2019). An R Companion to Applied Regression, 3rd Edn. Thousand Oaks, CA: Sage.
Franzluebbers, A. J., Haney, R. L., Honeycutt, C. W., Schomberg, H. H., and Hons, F. M. (2000). Flush of carbon dioxide following rewetting of dried soil relates to active organic pools. Soil Sci. Soc. Am. J. 64, 613–623. doi: 10.2136/sssaj2000.642613x
Garibaldi, L. A., Steffan-Dewenter, I., Kremen, C., Morales, J. M., Bommarco, R., Cunningham, S. A., et al. (2011). Stability of pollination services decreases with isolation from natural areas despite honey bee visits: habitat isolation and pollination stability. Ecol. Lett. 14, 1062–1072. doi: 10.1111/j.1461-0248.2011.01669.x
Gelfand, I., and Robertson, G. P. (2015). “Mitigation of greenhouse gas emissions in agricultural ecosystems,” in The Ecology of Agricultural Landscapes: Long-Term Research on the Path to Sustainability, eds S. K. Hamilton, J. E. Doll, and G. P. Robertson (New York, NY: Oxford University Press), 310–339.
Gelfand, I., Shcherbak, I., Millar, N., Kravchenko, A. N., and Robertson, G. P. (2016). Long-term nitrous oxide fluxes in annual and perennial agricultural and unmanaged ecosystems in the upper Midwest USA. Glob. Chang. Biol. 22, 3594–3607. doi: 10.1111/gcb.13426
Ghazanfar, M., Malik, M. F., Hussain, M., Iqbal, R., and Younas, M. (2016). Butterflies and their contribution in ecosystem: a review. J. Entomol. Zool. Stud. 115, 115–118.
Gotelli, N. J., Ellison, A. M., Dunn, D. R., and Sanders, N. J. (2011). Counting ants (Hymenoptera: Formicidae): biodiversity sampling and statistical analysis for myrmecologists. Myrmecol. News 15, 13–19.
Grieshop, M. J., Werling, B., Buehrer, K., Perrone, J., Isaacs, R., and Landis, D. (2012). Big brother is watching: studying insect predation in the age of digital surveillance. Am. Entomol. 58, 172–182. doi: 10.1093/ae/58.3.172
Griffin, S. R., Bruninga-Socolar, B., Kerr, M. A., Gibbs, J., and Winfree, R. (2017). Wild bee community change over a 26-year chronosequence of restored tallgrass prairie: bee communities of restored tallgrass prairie. Restor. Ecol. 25, 650–660. doi: 10.1111/rec.12481
Helms, J. A. IV., Ijelu, S. E., Wills, B. D., Landis, D. A., and Haddad, N. M. (2020). Ant biodiversity and ecosystem services in bioenergy landscapes. Agric. Ecosyst. Environ. 290:106780. doi: 10.1016/j.agee.2019.106780
Helms, J. A. IV., Smith, J., Clark, S., Knupp, K., and Haddad, N. M. (2021). Ant communities and ecosystem services in organic versus conventional agriculture in the U.S. Corn Belt. Environ. Entomol. 50, 1276–1285. doi: 10.1093/ee/nvab105
Hevia, V., García-Llorente, M., Martínez-Sastre, R., Palomo, S., García, D., Miñarro, M., et al. (2021). Do farmers care about pollinators? A cross-site comparison of farmers’ perceptions, knowledge, and management practices for pollinator-dependent crops. Int. J. Agric. Sustain. 19, 1–15. doi: 10.1080/14735903.2020.1807892
Holland, E. A., Robertson, G. P., Greenberg, J., Groffman, P. M., Booner, R. D., and Gosz, J. R. (1999). “Soil CO2, N2O, and CH4 exchange,” in Standard Soil Methods for Long-term Ecological Research, eds G. P. Robertson, D. C. Coleman, C. S. Bledsoe, and P. Sollins (New York, NY: Oxford University Press), 185–201.
Hölldobler, B., and Wilson, E. O. (2009). The Superorganism. New York, NY: W.W. Norton & Company, Inc, 522.
Holzschuh, A., Dormann, C. F., Tscharntke, T., and Steffan-Dewenter, I. (2011). Expansion of mass-flowering crops leads to transient pollinator dilution and reduced wild plant pollination. Proc. R. Soc. B Biol. Sci. 278, 3444–3451. doi: 10.1098/rspb.2011.0268
Hosler, S. C., Jones, H. P., Nelson, M., and Barber, N. A. (2021). Management actions shape dung beetle community structure and functional traits in restored tallgrass prairie. Ecol. Entomol. 46, 175–186. doi: 10.1111/een.12950
Hothorn, T., Bretz, F., and Westfall, P. (2008). Simultaneous inference in general parametric models. Biometr. J. 50, 346–363. doi: 10.1002/bimj.200810425
Hsieh, T. C., Ma, K. H., and Chao, A. (2016). iNEXT: an R package for rarefaction and extrapolation of species diversity (Hill numbers). Methods Ecol. Evol. 7, 1451–1456. doi: 10.1111/2041-210X.12613
Hussain, R. I., Brandl, M., Maas, B., Rabl, D., Walcher, R., Krautzer, B., et al. (2021). Re-established grasslands on farmland promote pollinators more than predators. Agric. Ecosyst. Environ. 319:107543. doi: 10.1016/j.agee.2021.107543
Hutton, S. A., and Giller, P. S. (2003). The effects of the intensification of agriculture on northern temperate dung beetle communities. J. Appl. Ecol. 40, 994–1007. doi: 10.1111/j.1365-2664.2003.00863.x
Intergovernmental Platform on Biodiversity and Ecosystem Services [IPBES] (2016). “Summary for policymakers of the assessment report of the Intergovernmental science-policy platform on biodiversity and ecosystem services on pollinators, pollination and food production,” in Secretariat of the Intergovernmental Science-Policy Platform on Biodiversity and Ecosystem Services, eds S. G. Potts, V. L. Imperatriz-Fonseca, H. T. Ngo, J. C. Biesmeijer, T. D. Breeze, L. V. Dicks, et al. (Bonn: IPBES secretariat), 36.
Intergovernmental Platform on Biodiversity and Ecosystem Services [IPBES] (2019). “Summary for policymakers of the global assessment report on biodiversity and ecosystem services of the Intergovernmental science-policy platform on biodiversity and ecosystem services,” in Secretariat of the Intergovernmental Science-Policy Platform on Biodiversity and Ecosystem Services, eds S. Díaz, J. Settele, E. S. Brondízio, H. T. Ngo, M. Guèze, J. Agard, et al. (Bonn: IPBES secretariat), 56. doi: 10.5281/zenodo.3553579
Isaacs, R., Tuell, J., Fiedler, A., Gardiner, M., and Landis, D. (2009). Maximizing arthropod-mediated ecosystem services in agricultural landscapes: the role of native plants. Front. Ecol. Environ. 7, 196–203. doi: 10.1890/080035
Jones, M. S., Fu, Z., Reganold, J. P., Karp, D. S., Besser, T. E., Tylianakis, J. M., et al. (2019). Organic farming promotes biotic resistance to foodborne human pathogens. J. Appl. Ecol. 56, 1117–1127. doi: 10.1111/1365-2664.13365
Jones, M. S., Halteman, W. A., and Drummond, F. A. (2016). Predator- and scavenger-mediated ecosystem services determined by distance to field-forest interface in the maine lowbush blueberry agroecosystem. Environ. Entomol. 45, 1131–1140. doi: 10.1093/ee/nvw082
Kahmark, K., Millar, N., and Robertson, G. P. (2020). Static Chamber Method for Measuring Soil Greenhouse Gas Fluxes. Geneva: Zenodo. doi: 10.5281/ZENODO.4630396
Karp, D. S., Moses, R., Gennet, S., Jones, M. S., Joseph, S., M’Gonigle, L. K., et al. (2016). Agricultural practices for food safety threaten pest control services for fresh produce. J. Appl. Ecol. 53, 1402–1412. doi: 10.1111/1365-2664.12707
Kordbacheh, F., Liebman, M., and Harris, M. (2020). Strips of prairie vegetation placed within row crops can sustain native bee communities. PLoS One 15:e0240354. doi: 10.1371/journal.pone.0240354
Kravchenko, A. N., Guber, A. K., Razavi, B. S., Koestel, J., Quigley, M. Y., Robertson, G. P., et al. (2019). Microbial spatial footprint as a driver of soil carbon stabilization. Nat. Commun. 10:3121. doi: 10.1038/s41467-019-11057-4
Kremen, C., and Merenlender, A. M. (2018). Landscapes that work for biodiversity and people. Science 362:eaau6020. doi: 10.1126/science.aau6020
Kurtz, C. (2013). A Practical Guide to Prairie Reconstruction, 2nd Edn. Iowa City, IA: University of Iowa Press.
Landis, D. A. (2017). Designing agricultural landscapes for biodiversity-based ecosystem services. Basic Appl. Ecol. 18, 1–12. doi: 10.1016/j.baae.2016.07.005
Landis, D. A., Wratten, S. D., and Gurr, G. M. (2000). Habitat management to conserve natural enemies of arthropod pests in agriculture. Annu. Rev. Entomol. 45, 175–201. doi: 10.1146/annurev.ento.45.1.175
Liang, D., and Robertson, G. P. (2021). Nitrification is a minor source of nitrous oxide (N2O) in an agricultural landscape and declines with increasing management intensity. Glob. Chang. Biol. 27, 5599–5613. doi: 10.1111/gcb.15833
Manning, P., and Cutler, G. C. (2018). Ecosystem functioning is more strongly impaired by reducing dung beetle abundance than by reducing species richness. Agric. Ecosyst. Environ. 264, 9–14. doi: 10.1016/j.agee.2018.05.002
Menéndez, R., González-Megías, A., Collingham, Y., Fox, R., Roy, D. B., Ohlemüller, R., et al. (2007). Direct and indirect effects of climate and habitat factors on butterfly diversity. Ecology 88, 605–611. doi: 10.1890/06-0539
Menke, S. B., Gaulke, E., Hamel, A., and Vachter, N. (2015). The effects of restoration age and prescribed burns on grassland ant community structure. Environ. Entomol. 44, 1336–1347. doi: 10.1093/ee/nvv110
Mitchell, M. G. E., Schuster, R., Jacob, A. L., Hanna, D. E. L., Dallaire, C. O., Raudsepp-Hearne, C., et al. (2021). Identifying key ecosystem service providing areas to inform national-scale conservation planning. Environ. Res. Lett. 16:014038. doi: 10.1088/1748-9326/abc121
Morandin, L. A., and Kremen, C. (2013). Hedgerow restoration promotes pollinator populations and exports native bees to adjacent fields. Ecol. Appl. 23, 829–839. doi: 10.1890/12-1051.1
Mosier, S., Córdova, S. C., and Robertson, G. P. (2021). Restoring soil fertility on degraded lands to meet food, fuel, and climate security needs via perennialization. Front. Sustain. Food Syst. 5:706142. doi: 10.3389/fsufs.2021.706142
Mueller, U. G., Gerardo, N. M., Aanen, D. K., Six, D. L., and Schultz, T. R. (2005). The evolution of agriculture in insects. Annu. Rev. Ecol. Evol. Syst. 36, 563–595. doi: 10.1146/annurev.ecolsys.36.102003.152626
Nemes, S. N., and Price, D. L. (2015). Illustrated Keys to the Scarabaeinae (Coleoptera: Scarabaeidae) of Maryland. Northeast. Nat. 22, 318–344. doi: 10.1656/045.022.0208
Nichols, E., Spector, S., Louzada, J., Larsen, T., Amezquita, S., and Favila, M. E. (2008). Ecological functions and ecosystem services provided by Scarabaeinae dung beetles. Biol. Conserv. 141, 1461–1474. doi: 10.1016/j.biocon.2008.04.011
Nielsen, M. C. (1999). Michigan Butterflies and Skippers: A Field Guide and Reference. East Lansing, MI: Michigan State University Extension.
Nkuba, M. R., Chanda, R., Mmopelwa, G., Kato, E., Mangheni, M. N., and Lesolle, D. (2020). Influence of indigenous knowledge and scientific climate forecasts on arable farmers’ climate adaptation methods in the Rwenzori region, Western Uganda. Environ. Manage. 65, 500–516. doi: 10.1007/s00267-020-01264-x
Pasquet, R. S., Peltier, A., Hufford, M. B., Oudin, E., Saulnier, J., Paul, L., et al. (2008). Long-distance pollen flow assessment through evaluation of pollinator foraging range suggests transgene escape distances. Proc. Natl. Acad. Sci. 105, 13456–13461. doi: 10.1073/pnas.0806040105
Paul, E. A., Kravchenko, A., Grandy, A. S., and Morris, S. (2015). “Soil organic matter dynamics: controls and management for sustainable ecosystem functioning,” in The Ecology of Agricultural Landscapes: Long-Term Research on the Path to Sustainability, eds S. K. Hamilton, J. E. Doll, and G. P. Robertson (New York, NY: Oxford University Press), 104–134.
Peck, S. L., McQuaid, B., and Campbell, C. L. (1998). Using ant species (Hymenoptera: Formicidae) as a biological indicator of Agroecosystem condition. Environ. Entomol. 27, 1102–1110.
Pérez-Suárez, M., Castellano, M. J., Kolka, R., Asbjornsen, H., and Helmers, M. (2014). Nitrogen and carbon dynamics in prairie vegetation strips across topographical gradients in mixed Central Iowa agroecosystems. Agric. Ecosyst. Environ. 188, 1–11. doi: 10.1016/j.agee.2014.01.023
Piccini, I., Palestrini, C., Rolando, A., and Roslin, T. (2019). Local management actions override farming systems in determining dung beetle species richness, abundance and biomass and associated ecosystem services. Basic Appl. Ecol. 41, 13–21. doi: 10.1016/j.baae.2019.09.001
Pollard, E. (1977). A method for assessing changes in the abundance of butterflies. Biol. Conserv. 12, 115–134. doi: 10.1016/0006-3207(77)90065-9
Raven, P. H., and Wagner, D. L. (2021). Agricultural intensification and climate change are rapidly decreasing insect biodiversity. Proc. Natl. Acad. Sci. 118:e2002548117. doi: 10.1073/pnas.2002548117
Reeder, K. F., Debinski, D. M., and Danielson, B. J. (2005). Factors affecting butterfly use of filter strips in Midwestern USA. Agric. Ecosyst. Environ. 109, 40–47. doi: 10.1016/j.agee.2005.02.016
Ricketts, T. H. (2004). Tropical forest fragments enhance pollinator activity in nearby coffee crops. Conserv. Biol. 18, 1262–1271. doi: 10.1111/j.1523-1739.2004.00227.x
Ricketts, T. H., Regetz, J., Steffan-Dewenter, I., Cunningham, S. A., Kremen, C., Bogdanski, A., et al. (2008). Landscape effects on crop pollination services: are there general patterns? Ecol. Lett. 11, 499–515. doi: 10.1111/j.1461-0248.2008.01157.x
Robertson, G. P., and Hamilton, S. K. (2015). “Long-term ecological research in agricultural landscapes at the Kellogg Biological Station LTER site: conceptual and experimental framework,” in The Ecology of Agricultural Landscapes: Long-Term Research on the Path to Sustainability, eds S. K. Hamilton, J. E. Doll, and G. P. Robertson (New York, NY: Oxford University Press), 1–32.
Robertson, G. P., Gross, K. L., Hamilton, S. K., Landis, D. A., Schmidt, T. M., Snapp, S. S., et al. (2015). “Farming for ecosystem services: an ecological approach to production agriculture,” in The Ecology of Agricultural Landscapes: Long-Term Research on the Path to Sustainability, eds S. K. Hamilton, J. E. Doll, and G. P. Robertson (New York, NY: Oxford University Press), 33–53. doi: 10.1093/biosci/biu037
Robertson, G. P., Hamilton, S. K., Barham, B. L., Dale, B. E., Izaurralde, R. C., Jackson, R. D., et al. (2017). Cellulosic biofuel contributions to a sustainable energy future: choices and outcomes. Science 356:eaal2324. doi: 10.1126/science.aal2324
Robertson, G. P., Paul, E. A., and Harwood, R. R. (2000). Greenhouse gases in intensive agriculture: contributions of individual gases to the radiative forcing of the atmosphere. Science 289, 1922–1925. doi: 10.1126/science.289.5486.1922
Rundlöf, M., Bengtsson, J., and Smith, H. G. (2008). Local and landscape effects of organic farming on butterfly species richness and abundance: scale-dependent effects of organic farming. J. Appl. Ecol. 45, 813–820. doi: 10.1111/j.1365-2664.2007.01448.x
Rusch, A., Bommarco, R., Jonsson, M., Smith, H. G., and Ekbom, B. (2013). Flow and stability of natural pest control services depend on complexity and crop rotation at the landscape scale. J. Appl. Ecol. 50, 345–354. doi: 10.1111/1365-2664.12055
Sands, B., and Wall, R. (2017). Dung beetles reduce livestock gastrointestinal parasite availability on pasture. J. Appl. Ecol. 54, 1180–1189. doi: 10.1111/1365-2664.12821
Scharnhorst, V. S., Fiedler, K., Frank, T., Moser, D., Rabl, D., Brandl, M., et al. (2021). Ant community composition and functional traits in new grassland strips within agricultural landscapes. Ecol. Evol. 11, 8319–8331. doi: 10.1002/ece3.7662
Schmidt, M. H., Roschewitz, I., Thies, C., and Tscharntke, T. (2005). Differential effects of landscape and management on diversity and density of ground-dwelling farmland spiders: landscape vs. management effects on spiders. J. Appl. Ecol. 42, 281–287. doi: 10.1111/j.1365-2664.2005.01014.x
Schulte, L. A., Niemi, J., Helmers, M. J., Liebman, M., Arbuckle, J. G., James, D. E., et al. (2017). Prairie strips improve biodiversity and the delivery of multiple ecosystem services from corn–soybean croplands. Proc. Natl. Acad. Sci. 114, 11247–11252. doi: 10.1073/pnas.1620229114
Shcherbak, I., and Robertson, G. P. (2019). Nitrous Oxide (N2O) emissions from subsurface soils of agricultural ecosystems. Ecosystems 22, 1650–1663. doi: 10.1007/s10021-019-00363-z
Smith, P., Martino, D., Cai, Z., Gwary, D., Janzen, H., Kumar, P., et al. (2007). “Agriculture,” in Climate Change 2007: Mitigation. Contribution of Working Group III to the Fourth Assessment Report of the Intergovernmental Panel on Climate Change, eds B. Metz, O. R. Davidson, P. R. Bosch, R. Dave, and L. A. Meyer (Cambridge: Cambridge University Press), 498–540.
Smith, R., Mcswiney, C., Grandy, A., Suwanwaree, P., Snider, R., and Robertson, G. P. (2008). Diversity and abundance of earthworms across an agricultural land-use intensity gradient. Soil Tillage Res. 100, 83–88. doi: 10.1016/j.still.2008.04.009
Sprunger, C. D., and Robertson, G. P. (2018). Early accumulation of active fraction soil carbon in newly established cellulosic biofuel systems. Geoderma 318, 42–51. doi: 10.1016/j.geoderma.2017.11.040
Sprunger, C. D., Oates, L. G., Jackson, R. D., and Robertson, G. P. (2017). Plant community composition influences fine root production and biomass allocation in perennial bioenergy cropping systems of the upper Midwest, USA. Biomass Bioenergy 105, 248–258. doi: 10.1016/j.biombioe.2017.07.007
Sunderland, K., and Samu, F. (2000). Effects of agricultural diversification on the abundance, distribution, and pest control potential of spiders: a review. Entomol. Exp. Appl. 95, 1–13. doi: 10.1046/j.1570-7458.2000.00635.x
Syswerda, S. P., and Robertson, G. P. (2014). Ecosystem services along a management gradient in Michigan (USA) cropping systems. Agric. Ecosyst. Environ. 189, 28–35. doi: 10.1016/j.agee.2014.03.006
Syswerda, S., Corbin, A., Mokma, D., Kravchenko, A., and Robertson, G. (2011). Agricultural management and soil carbon storage in surface vs. deep layers. Soil Sci. Soc. Am. J. 75, 92–101. doi: 10.2136/sssaj2009.0414
Tamburini, G., Bommarco, R., Wanger, T. C., Kremen, C., van der Heijden, M. G. A., Liebman, M., et al. (2020). Agricultural diversification promotes multiple ecosystem services without compromising yield. Sci. Adv. 6:eaba1715. doi: 10.1126/sciadv.aba1715
The Nature Conservancy [TNC] (2021). Leading at the Edge: A Roadmap to Advance Edge of Field Practices in Agriculture. Available online at: https://www.nature.org/content/dam/tnc/nature/en/documents/EOF_Report_LORES_SPREADS.pdf (accessed September 15, 2021).
Tilman, D., and Clark, M. (2015). Food, agriculture & the environment: can we feed the world & save the earth? Daedalus 144, 8–23. doi: 10.1162/DAED_a_00350
Tscharntke, T., Grass, I., Wanger, T. C., Westphal, C., and Batáry, P. (2021). Beyond organic farming – harnessing biodiversity-friendly landscapes. Trends Ecol. Evol. 36, 919–930. doi: 10.1016/j.tree.2021.06.010
Tscharntke, T., Klein, A. M., Kruess, A., Steffan-Dewenter, I., and Thies, C. (2005). Landscape perspectives on agricultural intensification and biodiversity – ecosystem service management. Ecol. Lett. 8, 857–874. doi: 10.1111/j.1461-0248.2005.00782.x
Tuck, S. L., Winqvist, C., Mota, F., Ahnström, J., Turnbull, L. A., and Bengtsson, J. (2014). Land-use intensity and the effects of organic farming on biodiversity: a hierarchical meta-analysis. J. Appl. Ecol. 51, 746–755. doi: 10.1111/1365-2664.12219
Ubick, D., Paquin, P., Cushing, P. E., and Roth, V. (2017). Spiders of North America: an Identification Manual, 2nd Edn. Keene, NH: American Arachnological Society.
USDA National Agricultural Statistics Service (2019). Crop Production 2019 Summary. Washington, DC: NASS.
van Klink, R., Bowler, D. E., Gongalsky, K. B., Swengel, A. B., Gentile, A., and Chase, J. M. (2020). Meta-analysis reveals declines in terrestrial but increases in freshwater insect abundances. Science 368, 417–420. doi: 10.1126/science.aax9931
Wepprich, T., Adrion, J. R., Ries, L., Wiedmann, J., and Haddad, N. M. (2019). Butterfly abundance declines over 20 years of systematic monitoring in Ohio, USA. PLoS One 14:e0216270. doi: 10.1371/journal.pone.0216270
Wills, B. D., and Landis, D. A. (2018). The role of ants in north temperate grasslands: a review. Oecologia 186, 323–338. doi: 10.1007/s00442-017-4007-0
Wills, B. D., Kim, T. N., Fox, A. F., Gratton, C., and Landis, D. A. (2019). Reducing native ant abundance decreases predation rates in Midwestern Grasslands. Environ. Entomol. 48, 1360–1368. doi: 10.1093/ee/nvz127
Wittwer, R. A., Bender, S. F., Hartman, K., Hydbom, S., Lima, R. A. A., Loaiza, V., et al. (2021). Organic and conservation agriculture promote ecosystem multifunctionality. Sci. Adv. 7:eabg6995. doi: 10.1126/sciadv.abg6995
Keywords: prairie strips, conservation, US Midwest, agriculture, spillover, soil, arthropod
Citation: Kemmerling LR, Rutkoski CE, Evans SE, Helms JA IV, Cordova-Ortiz ES, Smith JD, Vázquez Custodio JA, Vizza C and Haddad NM (2022) Prairie Strips and Lower Land Use Intensity Increase Biodiversity and Ecosystem Services. Front. Ecol. Evol. 10:833170. doi: 10.3389/fevo.2022.833170
Received: 10 December 2021; Accepted: 30 March 2022;
Published: 10 May 2022.
Edited by:
James Moran, Galway-Mayo Institute of Technology, IrelandReviewed by:
Gabor L. Lovei, Aarhus University, DenmarkMarco Ferrante, University of the Azores, Portugal
Copyright © 2022 Kemmerling, Rutkoski, Evans, Helms, Cordova-Ortiz, Smith, Vázquez Custodio, Vizza and Haddad. This is an open-access article distributed under the terms of the Creative Commons Attribution License (CC BY). The use, distribution or reproduction in other forums is permitted, provided the original author(s) and the copyright owner(s) are credited and that the original publication in this journal is cited, in accordance with accepted academic practice. No use, distribution or reproduction is permitted which does not comply with these terms.
*Correspondence: Lindsey R. Kemmerling, a2VtbWVyTDRAbXN1LmVkdQ==