- Department of Entomology and Plant Pathology, North Carolina State University, Raleigh, NC, United States
Aposematism and mimicry are complex phenomena which have been studied extensively; however, much of our knowledge comes from just a few focal groups, especially butterflies. Aposematic species combine a warning signal with a secondary defense that reduces their profitability as prey. Aculeate hymenopterans are an extremely diverse lineage defined by the modification of the ovipositor into a stinger which represents a potent defense against predators. Aculeates are often brightly colored and broadly mimicked by members of other arthropod groups including Diptera, Lepidoptera, Coleoptera, and Araneae. However, aculeates are surprisingly understudied as aposematic and mimetic model organisms. Recent studies have described novel pigments contributing to warning coloration in insects and identified changes in cis-regulatory elements as potential drivers of color pattern evolution. Many biotic and abiotic factors contribute to the evolution and maintenance of conspicuous color patterns. Predator distribution and diversity seem to influence the phenotypic diversity of aposematic velvet ants while studies on bumble bees underscore the importance of intermediate mimetic phenotypes in transition zones between putative mimicry rings. Aculeate hymenopterans are attractive models for studying sex-based intraspecific mimicry as male aculeates lack the defense conferred by the females’ stinger. In some species, evolution of male and female color patterns appears to be decoupled. Future studies on aposematic aculeates and their associated mimics hold great promise for unraveling outstanding questions about the evolution of conspicuous color patterns and the factors which determine the composition and distribution of mimetic communities.
Introduction
Aposematism has been a phenomenon of evolutionary and ecological interest since Alfred Russell Wallace and Charles Darwin first discussed warning coloration in caterpillars over 150 years ago (Wallace, 1867). Originally, the term aposematism was primarily associated with visual cues, such as the bright colors of many caterpillars, as an explanation for how conspicuousness could be favorable in life stages that do not experience sexual selection. We now know that warning signals are not limited to visual cues; many examples of non-visual aposematic signals, such as auditory and odor-based cues, are known, including the ultrasonic clicks of moths and non-repellent odors released by some plants and animals (Eisner and Grant, 1981; Dunning and Kruger, 1995; Hristov and Conner, 2005; Low et al., 2021). Many species present multimodal aposematic signals incorporating different types of cues which may strengthen or generalize the signal to a broader range of predators (Rowe and Halpin, 2013). As a result, aposematism is now generally defined as the pairing of a warning signal with a secondary defense that renders the prey unprofitable to attack by predators (Rojas et al., 2015). According to this definition, aposematism is a form of honest signaling which can benefit the prey and also potential predators by allowing them to make informed decisions about prey profitability.
The current body of literature on aposematism and mimicry, an intrinsically linked topic, is vast, a large proportion of studies have focused on poison dart frogs (Dendrobatidae) and Nymphalid butterflies, particularly the genus Heliconius. Heliconius butterflies display striking aposematic wing patterns and have served as a primary model group for studying mimicry for over 150 years (Bates, 1862). Hypotheses on the evolution, maintenance, and spatial distribution of mimetic patterns have been tested in Heliconius, and the genetic basis of mimicry is particularly well characterized in this group compared to other model systems (Mallet and Barton, 1989; Mallet and Gilbert, 1995; Jiggins et al., 2001; Kapan et al., 2006; Baxter et al., 2008; Kronforst and Papa, 2015). Extending these studies to different taxonomic groups would allow us to test whether other mimetic communities follow similar trends and explore questions such as: What are the chemical and genetic bases of conspicuous color patterns and color pattern variation? Which ecological factors and selective pressures are important in determining the phenotypic variability and geographic distribution of conspicuous color patterns? What factors facilitate the evolution of intraspecific sex-based color pattern variation?
The Aculeata (ants, bees, and stinging wasps) are a promising clade for addressing these questions and other related topics as they include some of the most widely mimicked aposematic species, and the female stinger represents an innate difference in the defenses of males and females. Surprisingly, aculeates have received little attention as model aposematic and mimicry systems despite the diversity of conspicuous color patterns found in the group. Ants (Formicidae) are well known as an incredibly diverse and ecologically important group mimicked by a wide array of arthropods; however, outside the recently described golden mimicry complex in Australia, most ant mimics imitate their body shape or behavior rather than conspicuous coloration (McIver and Stonedahl, 1993; Pekár et al., 2017). This review will focus on aposematic aculeates which do display conspicuous warning color patterns and their associated mimicry rings. Two groups that have been studied in this context are bumble bees (Bombus), whose coloration has a long history of study, and velvet ants (Mutillidae) which have emerged as another promising system in the past decade (Stiles, 1979; Plowright and Owen, 1980; Wilson et al., 2012; Ezray et al., 2019). The findings of these studies will be discussed following a brief overview of our current understanding of aposematism and mimicry systems.
Background
In some cases, it may be difficult to tell whether a species meets both criteria required to be considered aposematic: a warning signal and a secondary defense. Some cryptic species have secondary defenses, and many undefended species mimic aposematic species by exhibiting dishonest conspicuous warning signals. Organisms falling into either of these categories can be readily distinguished from aposematic species with sufficient knowledge about the defensive strategies they employ and how conspicuous they are to co-occurring predators. Since humans are often not the target audience of warning signals, we may not recognize or perceive certain signals in the same way as an organism’s natural predators. Human perception of color patterns may be similar to that of visual predators such as birds; however, predators may also pick up on ultraviolet or infrared signals exhibited by prey that are outside the spectrum of light visible to us (Cuthill and Bennett, 1993; Dittrigh et al., 1993; Penney et al., 2012). Some conspicuous color patterns are important for mate recognition, thermoregulation and other functions, without also conferring increased predator avoidance (Stuart-Fox et al., 2017; Rojas et al., 2018). On one hand, we may misdiagnose some signals which do not actually play a role in predator avoidance as aposematic, and on the other, overlook genuine aposematic signals due to sensory biases.
If we lack sufficient information to definitively identify a given conspicuous color pattern as aposematic, more conservative terminology should be used. The term “conspicuous color pattern” is appropriate for situations in which the function of a given color phenotype is unknown. Conspicuous color patterns can be labeled as warning signals if they are known to confer increased predator avoidance, whether or not they are paired with defenses. For example, in guppies, strong sexual selection for conspicuousness may reduce survival due to increased predation, therefore these conspicuous color patterns should not be referred to as warning signals or as aposematic (Endler, 1980). Care must also be taken in distinguishing aposematic species from undefended mimics as only honest warning signals should be referred to as aposematic.
Aposematism and mimicry are closely connected and both phenomena have traditionally been viewed as frequency-dependent predator avoidance mechanisms, such that changes in the relative abundance of aposematic models, undefended mimics, and alternative prey can influence the fitness benefits of being aposematic or a mimic (Marples and Mappes, 2011). The two most prevalent forms of mimicry are Batesian and Müllerian, although several others have been described (Figure 1). Batesian mimicry involves an undefended species mimicking a defended model to gain a parasitic benefit at the cost of the model species (Bates, 1862). The dishonest signals of Batesian mimics are thought to reduce the relative fitness of the model by diluting the model’s honest signal in a frequency-dependent manner. Müllerian mimicry, on the other hand, is characterized by the convergence of two or more defended species upon similar aposematic signals, which strengthens the overall signal and spreads out the burden of educating naive predators to the mutual benefit of all involved mimics (Müller, 1878). In some situations, two or more defended species that differ in the degree to which they are defended may mimic each other. When the less defended species gains a parasitic benefit, this is referred to as quasi-Batesian mimicry (Speed, 1990; Rowland et al., 2010). Batesian, quasi-Batesian, and Müllerian mimicry are all types of interspecific mimicry; two types of intraspecific mimicry will also be discussed in this review. The first is Browerian mimicry, or automimicry, which occurs when there is variation in the degree to which individuals in a population are defended (Brower et al., 1967). Automimicry can be thought of as intraspecific Batesian mimicry. The second type is dual sex-limited mimicry (DSLM) which occurs when there is sex-based intraspecific variation in conspicuous color patterns. In species exhibiting DSLM, males and females are often members of different mimicry rings (Evans, 1968).
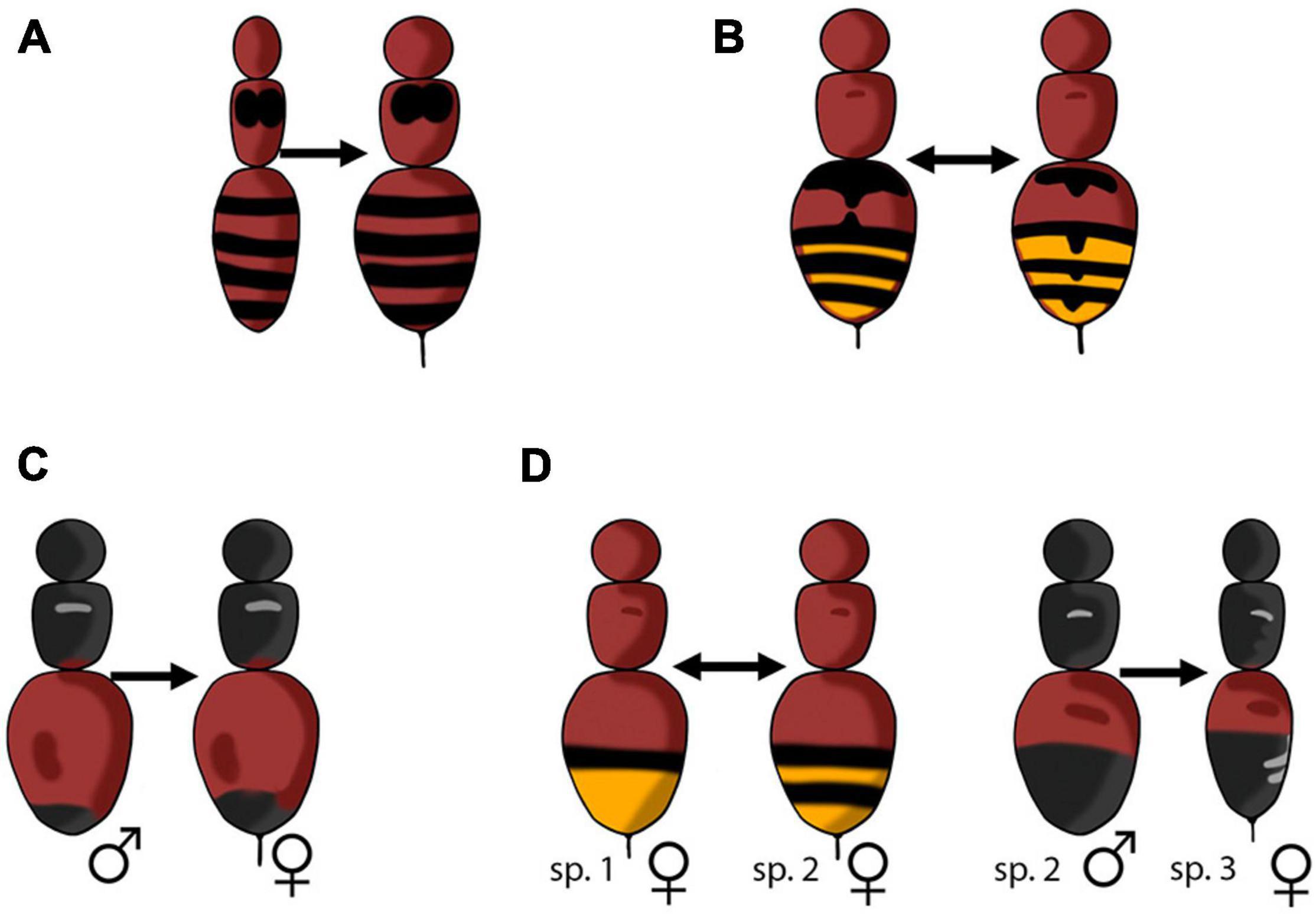
Figure 1. Diagram of the types of mimicry discussed in this review. Arrows represent the direction of selection and point from the mimic to the model, defended model species are indicated by a stinger. (A) Batesian mimicry, an undefended species mimicking a defended model species. (B) Müllerian mimicry, two different defended model species mimic each other. (C) Automimicry, an undefended member of species mimics a defended conspecific model. (D) Dual sex-limited mimicry, a defended female of species 2 is a Müllerian mimic of species 1, an undefended male of species 2 is a Batesian mimic of species 3. Illustration created by Jen Schlauch.
The fitness benefits of being aposematic may vary both spatially and temporally (Ruxton et al., 2018). Skelhorn et al. (2016) and Ruxton et al. (2018) both give excellent summaries of the topic, particularly the subtleties of correctly identifying aposematism. The most salient points are outlined below. Spatial variation in relative fitness is evident due to the existence of mimicry rings and transition zones between regions with different characteristic patterns (Mallet and Barton, 1989; Mallet and Gilbert, 1995; Wilson et al., 2012). Transition zones are of particular interest and utility in identifying how dispersal barriers, differences in predation pressure, and other factors interact to drive different color patterns to local dominance in a spatial mosaic. Additionally, the conspicuousness of warning signals can be context dependent. Aposematism and crypsis are often thought of as opposite, mutually exclusive strategies, but some apparently conspicuous patterns may be cryptic against certain backgrounds, or when viewed from certain distances (Tullberg et al., 2005). Temporal variation in the fitness benefits conferred by aposematism is perhaps less obvious but also common. The relative fitness of aposematic individuals can fluctuate based on a variety of factors including variation in the relative abundance of naive predators and the availability of alternative prey (Skelhorn et al., 2016; Ruxton et al., 2018). A study of caterpillars in Finland found a phenological match between the emergence time of aposematic caterpillars and the points in the season when the relative fitness of aposematism is highest compared to crypsis (Mappes et al., 2014). Crypsis was favored when young naive avian predators were abundant and learning to associate aposematic signals with unprofitable prey (Mappes et al., 2014). Variation in predator state, such as hunger level or toxin load, can influence the decision making of experienced predators and cause them to knowingly attack aposematic prey they would usually avoid (Skelhorn et al., 2016). Predators and predator communities also vary in their susceptibility to specific toxins and their ability to detect prey or learn to associate specific patterns with unprofitability (Endler and Mappes, 2004).
Hymenopteran Color Pattern Diversity
There are currently over 150,000 described hymenopteran species within ∼90 extant families, many of which contain conspicuously colored species (Figure 2; Branstetter et al., 2017; Peters et al., 2017). Hymenopterans are divided into two groups. Symphyta is a paraphyletic assemblage composed of early diverging lineages whose members have a broadly connected thorax and abdomen and are commonly known as sawflies (Malm and Nyman, 2015). Apocrita is a monophyletic suborder containing the vast majority of hymenopteran species distinguished by a narrow “waist” between the first two abdominal segments (Peters et al., 2017). All stinging hymenopterans (ants, bees and stinging wasps) belong to the monophyletic infraorder Aculeata which is nested within Apocrita (Branstetter et al., 2017). Aculeata includes over 70,000 currently described species grouped into nearly 40 families, bees (Anthophila) and ants represent distinct monophyletic lineages within Aculeata containing over 20,000 and 14,000 species, respectively (Branstetter et al., 2017; Sann et al., 2018). The stinger has been secondarily lost in some lineages, including a few families of bees and several subfamilies of ants. A huge variety of aposematic patterns are displayed by adult aculeates, and a number of sawflies and non-aculeate Apocrita also display warning coloration, although they lack a stinger. The larvae of some sawfly species also exhibit conspicuous color patterns. Accurately quantifying the number of hymenopteran species displaying warning colors, let alone distinguishing between aposematic species and undefended mimics, is an immense undertaking due to the sheer diversity of the order. A study which focused on a single color pattern, black-orange-black, highlights the magnitude of the task. This pattern was found to occur in over 200 species in at least 23 hymenopteran families, and although the authors examined over 1.2 million specimens, they describe their survey as preliminary with respect to this single pattern (Mora and Hanson, 2019).
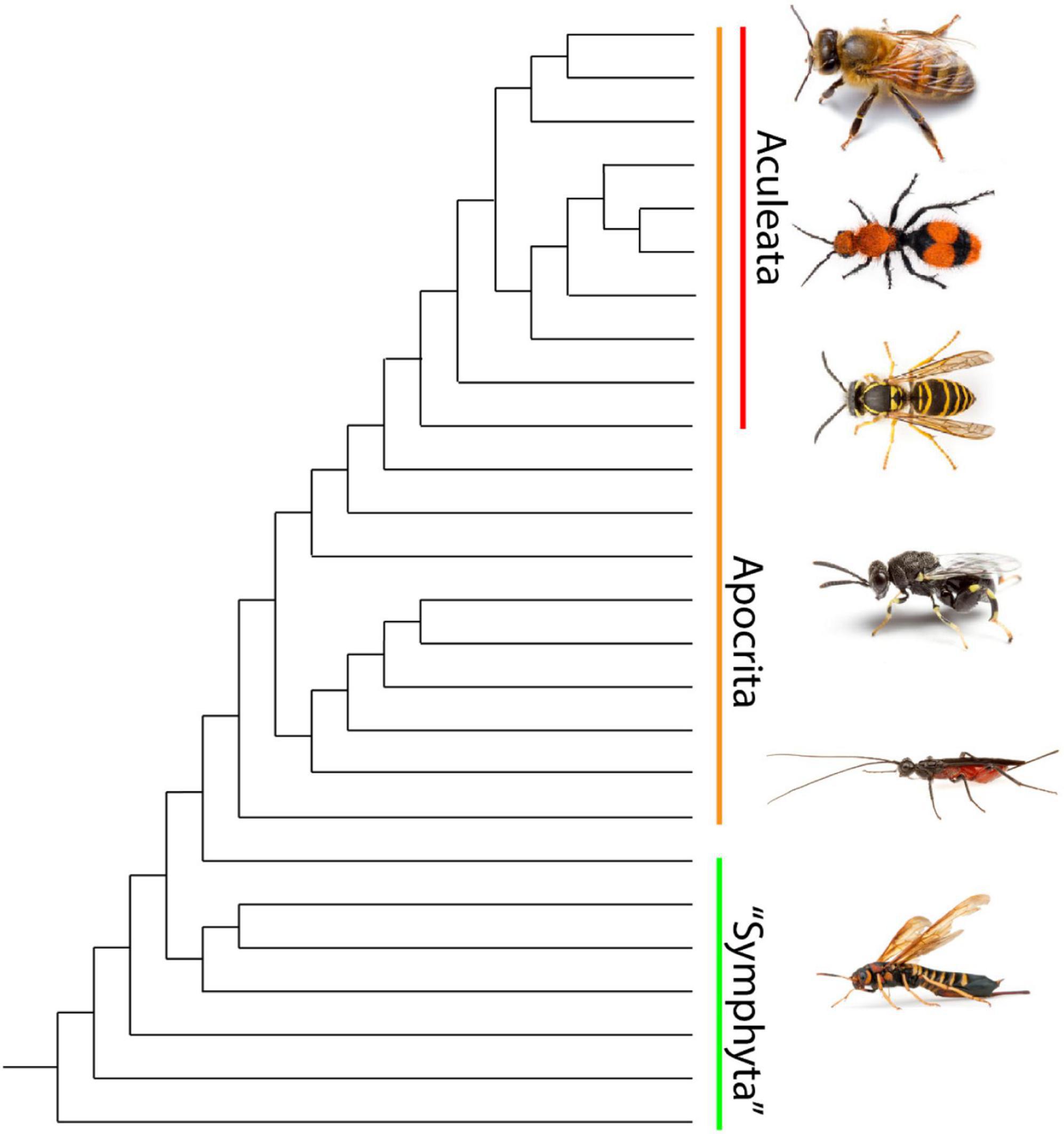
Figure 2. Phylogeny of hymenopteran superfamily relationships after (Branstetter et al., 2017). Symphyta is a paraphyletic grade consisting of the earliest diverging hymenopteran lineages. The monophyletic suborder Apocrita contains all remaining lineages and the bulk of Hymenopteran diversity including the Aculeata. Apidae and Chalcididae images are ©Matt Bertone, used with permission. All other images are ©Alex Wild, used with permission.
A diverse array of hymenopteran defensive mechanisms and other warning signals are known in addition to conspicuous color patterns. Velvet ants in particular exhibit many other adaptations. These include a hard slippery exoskeleton, a potent stinger in females, the ability to stridulate in response to the presence of predators, and the ability to release unpleasant odors which may function as both a defense and a warning signal (Manley, 2000). Other common strategies employed by hymenopterans include spines, aggregation of immatures by several sawfly species, communal attack by some social species, and the ability to spray noxious chemicals in some stingless ant lineages.
Chemical and Genetic Basis of Insect Warning Coloration
Understanding the chemical and genetic underpinnings of color in insects is important as it allows researchers to test why some groups exhibit greater phenotypic variation than others and whether similar patterns are produced by common mechanisms and pathways at different taxonomic levels. Insects produce an exceptional variety of colors, most of which can be classified as either structural or pigment-based. This review will focus primarily on pigment-based color, as the majority of literature on insect structural color comes from study of Coleoptera and Lepidoptera although some research has been conducted on structural color in hymenopterans (Nemésio, 2005; Kroiss et al., 2009; Pekár et al., 2017). Insect pigments have been studied in several orders while studies of the genetic basis of conspicuous coloration in insects have focused predominantly on Heliconius, a single genus within Lepidoptera. Increasing the taxonomic breadth of these studies could elucidate whether their findings are lineage-specific or consistent across other groups.
Pigments have many functions in insects including predator avoidance and thermoregulation as well as resistance to desiccation, ultraviolet radiation and infection by parasites (Shamim et al., 2014). The chemical basis of aposematism and color pattern polymorphism is relatively understudied in Hymenoptera but has recently been explored in bumble bees and velvet ants (Hines et al., 2017; Tian et al., 2019). Melanins were identified as the primary pigment underlying aposematic color patterns in these groups and a novel yellow pigment similar to those found in butterfly wings was discovered (Hines, 2008a,b; Hines et al., 2017). Both black eumelanins, which were thought to be responsible for all insect melanization, and red pheomelanins, previously unknown in insects, are important components of color patterns in these two aculeate groups (Hines et al., 2017). These findings suggest that further analysis of the chemistry underlying conspicuous aculeate color patterns will likely continue to yield new discoveries.
Our knowledge of the genetic basis of insect color patterns is primarily limited to Heliconius and swallowtail butterflies (Papilionidae: Papilio) (Joron et al., 2011; Heliconius Genome Consortium., 2012; Kunte et al., 2014; McMillan et al., 2020). Wing pattern variation in swallowtails is controlled by a small number of large-effect loci (Kunte et al., 2014; Nishikawa et al., 2015). Extensive crossing experiments have shown that wing pattern variation in Heliconius is also mostly controlled by a few large-effect Mendelian loci with many quantitative loci contributing to a lesser extent (Sheppard et al., 1985; Kronforst and Papa, 2015). In Heliconius, these large effect loci include WntA which controls melanic coloration, optix associated with red coloration, and the P locus which is conserved across the genus and named for a supergene that controls the entire wing pattern in one species, H. numata (Joron et al., 2006; Kronforst and Papa, 2015). The protein sequences of WntA and optix are also well conserved within Heliconius suggesting that changes in cis-regulatory elements may drive the evolution of wing patterns (Martin et al., 2012). Cis-regulatory elements are also known to be important drivers of the divergence and subsequent adaptive radiation of color patterns in East African cichlids (Urban et al., 2021).
Now that the fundamental genetic basis of conspicuous wing patterns is known in Heliconius, researchers have started looking for similar genetic structure in other butterfly groups to test whether this function arose in this genus or is evolutionarily conserved. For example, in Limenitis, another Nymphalid genus, WntA was found to control a similar melanic wing pattern and this phenotype was perfectly associated with a 9-kb retrotransposon in the first intron of WntA (Gallant et al., 2014). In Heliconius, a 1.8-kb indel was found to be perfectly associated with a specific wing pattern phenotype. Alignment of Limenitis and Heliconius WntA sequences showed that the respective position of the retrotransposon and indel overlap, suggesting independent origins of similar wing pattern elements through cis-regulatory mutations in the same region (Gallant et al., 2014).
New genomic tools can facilitate study of the pathways driving color pattern expression in non-model systems which do not have data from decades of crosses to utilize. Genes driving color polymorphism observed in the bumble bee Bombus melanopygus were identified using comparative functional analysis of genomes (Tian et al., 2019). A cis-regulatory element between the Hox genes abd-A and Abd-B determines whether the abdomen is black or red, corresponding to either the Pacific coastal or Rocky Mountain mimicry ring, respectively (Tian et al., 2019). Comparative studies, such as this and the previously mentioned work on WntA in Limenitis, seem to be a particularly promising approach for identifying loci controlling color pattern expression. Expanding studies to other hymenopteran taxa and comparing more distantly related groups could help establish whether the genetic mechanisms underlying similar conspicuous color patterns are phylogenetically conserved or arose from repeated independent mutations at specific loci within diverse clades such as vespid wasps, Aculeata, or Hymenoptera as a whole.
Ecological Factors and Selective Pressures
Early models of the evolution and maintenance of aposematic signals and Müllerian and Batesian mimicry treated the fitness benefits of conspicuous color patterns as unifunctional and strictly frequency dependent and their predictions do not match the local variation seen in nature. Conspicuous color phenotypes are now known to be influenced by other biotic and abiotic factors in addition to functioning as warning signals to predators (Ojala et al., 2007). Briolat et al. (2018) provide an excellent overview and discussion of diversity in warning coloration in their recent review. Numerous hypotheses have been put forward to explain variation in conspicuous coloration both within and between species and communities. For example, Kikuchi and Pfennig (2013) reviewed hypotheses regarding imperfect mimicry and grouped them into four categories: factors which temporarily prevent a response to selection for perfect mimicry, relaxed selection for perfect mimicry, local trade-offs which cause imperfect mimicry to be favored by selection, and imperfect mimicry being an artifact of human perception. The purpose of this section is not to provide a comprehensive summary of all such hypotheses on imperfect mimicry and other phenomena, but to highlight studies which focus on identifying ecological factors and selective pressures that could drive the phenotypic diversity of aculeate mimicry systems and to suggest specific hypotheses which may be well suited to future testing within Aculeata.
Velvet ant mimicry rings are an excellent example of multiple selective pressures driving extant variation in aposematic color patterns. Females are apterous, mostly diurnal, and spend much of their time actively searching for hosts, mainly large ground nesting hymenopterans, for their offspring to parasitize (Schmidt and Blum, 1977). These factors and behavior may increase their susceptibility to discovery and attack by predators, reducing the benefits of crypsis and driving the evolution of conspicuous patterns and potent defenses within the clade. Lizards are thought to be the primary predators of velvet ants and have been shown to either innately avoid aposematic species or rapidly learn avoidance (Vitt and Cooper, 1988; Pan et al., 2017). Eight distinct mimicry rings were identified in a study which included all 21 diurnal North American velvet ant genera and 84% of North American species (Wilson et al., 2015). The distribution of conspicuous phenotypes within the phylogeny suggests that convergent evolution of co-occurring species due to Müllerian mimicry was responsible for the observed mimicry complex rather than common ancestry (Wilson et al., 2012).
Interestingly, African velvet ant mimicry rings are less phenotypically diverse, with only 4 distinct rings identified based on examination of a comparable number of species, even though velvet ants are much more taxonomically rich in Africa than in North America (Wilson et al., 2018). This disparity could be explained by differences in the diversity and distribution of predators and ecoregions between the two continents as the richness of velvet ant mimicry rings appears to correlate with the richness of local lizard predators (Wilson et al., 2013, 2018). North America harbors a greater diversity of insectivorous lizards than Africa does at the family, genus, and species levels and most North American lizard species are concentrated in a few ecoregions clustered in a relatively narrow geographic range (Pianka, 1967; Wilson et al., 2018). The more even distribution of lizard species across a larger landmass in Africa could explain the relatively low phenotypic diversity of velvet ant mimicry rings compared to those found in North America (Wilson et al., 2018).
Another study on velvet ants highlights the need for caution in ascribing function to color patterns without direct evidence. Three Dasymutilla species within the North American desert mimicry ring have separately evolved long white hairs and were previously thought to mimic the fruits of creosote bushes. However, the three species in question were found to share similar reflectance spectra, distinct from that of creosote fruit, and ancestral state reconstructions place the origin of this white color pattern some 4 million years prior to the introduction of creosote bushes (Wilson et al., 2020). Temperature assays found that white members of the desert mimicry ring remain cooler than those which are orange. Combined, these results point to this phenotype initially evolving as a thermoregulatory adaptation to the desert climate rather than being driven by predation pressure (Wilson et al., 2020). The authors suggest that Müllerian mimicry could have then facilitated diversification of light-colored setal phenotypes within the desert mimicry ring. This study underscores the need to evaluate the actual fitness benefits conferred by a given color pattern before making assumptions about the selective factors driving initial evolution of the phenotype.
The richness and distribution of predator communities seems to be an important factor driving the phenotypic diversity of velvet ant mimicry rings. Comparing the responses of naive and educated predators to aposematic species from mimicry rings which overlap with the distribution of the predator, and those from other non-overlapping mimicry rings would test two questions. First, is predator avoidance of velvet ants an innate or learned behavior? Second, how do predators respond to new conspicuous phenotypes? Additionally, comparing responses to different conspicuous phenotypes within a given mimicry ring would tell us to what extent predators generalize color patterns within putative mimicry rings. If both naive and educated predators avoid unfamiliar aposematic phenotypes, or educated predators broadly generalize avoidance, this could indicate that some of the other warning signals exhibited by velvet ants are more important for predator avoidance. Manipulating the expression of these signals, such as odors and stridulations, and testing whether predator responses change based on the suite of characters presented, would provide insight into how individual components impact predator learning and avoidance and interact to form multimodal signals. Pekár et al. (2017) found that visually- and non-visually orienting predators show similar degrees of avoidance of members of the golden mimicry complex suggesting that displaying multimodal signals is important for avoiding a predator community consisting of multiple distinct guilds. This was an interesting finding, and it illustrates the difficulty of quantifying predator generalization of warning signals when mimics exhibit multimodal signals.
Color patterns in bumble bees are perhaps the best characterized of all hymenopterans thanks to a long history of research (Dalla Torre, 1880; Vogt, 1909; Stiles, 1979; Plowright and Owen, 1980). In a landmark paper published in 2007, Paul Williams examined all 219 social bumble bee species described at that time for the presence of repeated and co-occurring color patterns, and to determine whether any patterns are associated with specific habitats (Williams, 2007). Several color pattern groups were identified, and the most species-rich groups were found to significantly cluster in specific geographic regions based on available collection data (Williams, 2007).
A subsequent study used a similar dataset and unsupervised machine learning based on human visual perception to cluster color patterns (Ezray et al., 2019). The authors used an extensive geographic dataset for preserved specimens found in the United States to describe the “average pattern” in 167 × 88 km cells. Both the average color pattern of all species within a grid cell (perceptual mimicry optimum), and the average counting each distinct morphotype once independent of frequency (average color pattern) were calculated. When viewed at a coarse scale, bumble bee color patterns appear to fall into four mimicry complexes which are distinct both visually and geographically as predicted by traditional mimicry theory. However, the authors found that at a finer scale, bumble bee color patterns form a perceptual continuum along a geographic gradient. Imperfect or intermediate mimicry may even be favored in transition zones and persist due to generalization by predators or by being the best representation of the average pattern in these regions (Ezray et al., 2019). Similar color patterns were found to have independent origins in different bumble bee clades, again supporting convergent evolution rather than common ancestry (Ezray et al., 2019). This study provided a useful, broadly applicable method for quantifying color patterns and laid the groundwork for future studies on the evolution of mimicry patterns in transition zones.
Comparing the responses of predators in transition zones to the phenotypes of imperfect mimics and distinct phenotypes from adjacent regions would help tease apart whether intermediate phenotypes persist due to generalization or are actually selectively favored. Testing the relative thermal tolerance, desiccation resistance, and parasite load of distinct, co-occurring species and morphs could identify additional selective pressures, outside of predation, driving the phenotypic variability and spatial turnover observed in transition zones. Applying this method to other widespread aposematic groups which form multiple mimicry rings would be useful in determining whether most groups occur along continua or cluster into discrete groups with hard spatial boundaries. The identification of areas in which the perceptual mimicry optimum and average color pattern differ significantly may be of special interest.
Velvet ants and bumble bees represent speciose clades containing many important aposematic models and a diverse array of conspicuous color patterns. Within each group, similar color patterns were found to have arisen through convergent evolution as opposed to shared phylogenetic history. Combined with the comparative study on color patterns in Bombus melanopygus, this lends some support to the hypothesis that mutations at cis-regulatory elements controlling conserved loci play an important role in bumble bee color pattern evolution as in Heliconius. In velvet ants, mimicry ring diversity appears to be correlated with predator and ecoregion diversity in line with the expected benefits of Müllerian mimicry. Bumble bee color patterns were found to form geographic and perceptual continua with significant transition zones between seemingly discrete mimicry rings. These studies have paved the way for future work in the many other aculeate groups known to exhibit a variety of conspicuous color patterns.
Sex-Based Intraspecific Mimicry
Brower et al. (1967) first described automimicry in monarch butterflies when they found that adults reared on more toxic milkweed species are chemically defended while adults reared on less toxic milkweeds are palatable and mimic noxious individuals. In aculeates, the characteristic female stinger represents an innate sex-based morphological difference in defenses rather than a diet-based difference. In many aculeate species, males display color patterns identical or very similar to those of conspecific females even though the males lack a stinger. DSLM was first described by Howard Evans based on several spider wasp (Pompilidae) species (Evans, 1968). In these species, females are Müllerian mimics, while males are Batesian mimics, but of other aposematic species; males and females are thus members of different mimicry rings. DSLM is now also known to occur in other insect groups in addition to spider wasps, including the closely related velvet ants and some butterfly species, although in butterflies both sexes are often Batesian mimics (Kunte, 2008; Wilson et al., 2015).
The evolutionary dynamics of sexual automimicry and DSLM in aculeates are intriguing. The evolution and persistence of these phenomena could potentially be explained by a wide variety of factors which may alter the benefit gained by male automimics and cost incurred by aposematic females. These include biased sex ratios, the presence of other distinct patterns for males to mimic, and the presence of shared, or potentially male specific, defenses. Differences in life history traits such as lifespan, emergence time, foraging behavior, and mate finding strategies could also drive the evolution of sexual dichromatism by causing males and females to effectively reside in different mimetic communities or experience predation pressure from somewhat different predator communities. The presence of highly noxious, non-conspecific models may facilitate this process if predators avoid even low fidelity mimics due to the high cost of accidentally attacking a potently defended model species.
The effects automimics have on predator foraging decisions were evaluated in a study which used chicks as predators and crumbs as prey (Skelhorn and Rowe, 2007). Prey included defended and undefended conspicuous red crumbs, representing automodels and automimics, respectively, alongside undefended green crumbs which matched the background representing alternative cryptic prey. Crumbs representing automodels were sprayed with a distasteful quinine solution while those representing automimics or alternative cryptic prey were sprayed with water. The authors found that the presence of automimics did not affect predation rates when undefended automimics comprised less than 25% of the population (Skelhorn and Rowe, 2007). This provides some support for mechanisms involving reduced male presence, such as biased sex ratios, as explanations for the maintenance of automimicry in populations where sex-based differences in defenses exist.
To date, most studies on automimicry have been theoretical or lab-based with relatively few being conducted in natural systems. Using sex-based automimicry model systems like aculeates, rather than groups in which automimicry is diet-based would alleviate the need to monitor and track the feeding habits of individuals, making field studies more feasible. DSLM in general is poorly understood, likely at least in part because associating males with their conspecific females can be difficult morphologically, but genetic identification methods should help alleviate this initial problem. Future studies on the genetic underpinnings of DSLM could seek to explain how sex-based polymorphism of color phenotypes is produced and in what order new phenotypes evolved from the ancestral pattern. Comparative studies focusing on the life history of populations with automimics or DSLM and their closest relatives not exhibiting these phenomena could identify specific selective pressures driving these types of mimicry.
Discussion
Traditionally, the study of aposematism and mimicry has been restricted to a few focal taxonomic groups; however, recently developed methods and tools are giving researchers new ways to view and measure color patterns in other taxonomic groups. Sequencing costs have declined to the point where genome scale datasets can be generated fairly affordably and comparative genomic studies have emerged as a powerful method for identifying the genes and common genetic architecture underlying color pattern polymorphism and diversification (Gallant et al., 2014; Tian et al., 2019). Identification of common aposematic patterns within and between groups will be aided by ongoing efforts to digitize specimens and accompanying advances in analysis of digitized material. Multispectral imaging of specimens will facilitate identification of patterns conspicuous to predators but invisible to humans. Image analysis with machine learning tools may maintain the continuous nature of patterning, which is lost in matrix-based approaches, while being less sensitive to minor differences in color pattern location which may not be biologically relevant (Wham et al., 2019).
Recent studies leveraged some of these tools to establish velvet ants and bumble bees as the first aculeate models for studying color pattern variation and mimicry. As in Heliconius and cichlids, cis-regulatory elements were identified as drivers of color pattern evolution in a species of bumble bee. Continued study of the pigment chemistry and genetic basis of conspicuous color patterns will further our understanding of how these striking phenotypes are produced and allow us to test whether cis-regulatory elements are important drivers of color pattern variation across taxa or if other mechanisms are also common. The evolution of aposematic color patterns in velvet ants and bumble bees has been driven by convergent evolution which underscores the importance of studying these patterns in an ecological and phylogenetic context. Aculeates represent a valuable group for testing hypotheses about the evolution and maintenance of conspicuous color patterns in natural systems as they display such a wide variety of conspicuous patterns, many of which are shared with more distantly related hymenopteran taxa.
The papers discussed in this review on velvet ants and bumble bees primarily focus on these groups in the context of within-group Müllerian mimicry. Future studies could instead focus on other Müllerian or Batesian mimics of these taxa. Batesian mimics of velvet ants include beetles, spiders and antlion larvae, while at least five fly families contain species which mimic bumble bees (Gabritschevsky, 1926; Wilson et al., 2012). Comparing the abundance, diversity, and mimetic fidelity of Batesian mimics within a community to their aposematic models could further our understanding of the impact of dishonest mimics on model fitness. It would be especially interesting to compare the abundance and relative fitness of Müllerian and Batesian mimics in communities in which there is seasonal variation in the presence of naive predators, or in the availability and relative profitability of alternative prey.
Aculeate hymenopterans represent an evolutionarily diverse set of models and mimics, they are mimicked by a wide variety of insects and even other arthropods and some mimicry rings based on aculeate models are taxonomically rich at or above the ordinal level. These super diverse mimicry rings are well-suited for studying imperfect mimicry, especially for exploring what factors set the spatial boundaries of a given ring and why some species have evolved to become mimics while close relatives have not. Ant mimicry complexes are of particular note for the astonishing morphological and behavioral adaptations mimics have evolved to resemble ants. Aculeates are also ideal for studying automimicry, which has been a challenging concept to understand and model evolutionarily. DSLM has scarcely been studied at all since its description over 50 years ago and may also be best addressed in aculeate systems in which multiple distinct mimetic patterns are known to overlap, such as with velvet ants (Wilson et al., 2015, 2018). Promising aculeate groups for future studies on mimicry include large carpenter bees (Blaimer et al., 2018), vespid wasps (Perrard et al., 2014; Marchini et al., 2016), and spider wasps (Schmidt, 2004).
Integrative studies across Hymenoptera could allow researchers to tackle broad evolutionary questions in a comparative framework. Collaboration between research groups studying the genetic basis of color pattern variation across taxa will likely yield exciting new insight into the mechanisms by which similar color patterns are produced and whether these patterns have a common genetic basis or function. The work on melanism in bumble bees and velvet ants is an example of the potential for collaborative studies to enhance our understanding of these patterns (Hines et al., 2017). Other goals of collaborative work could include: reconstructing the origin of aposematism and mimicry in different clades, identification of the selective pressures driving the current distribution of color patterns in communities and across groups, and comparing the relative rates at which honest and dishonest warning signals are gained and lost. Comparing multiple, distantly related, co-occurring species may also help us identify the mechanisms by which factors such as predation pressure, sexual selection, and other biotic and abiotic environmental variables contribute to warning signal local optima. Our understanding of color adaptations has come a long way since Wallace’s letter to Darwin, but a great deal of work remains to be done. The Aculeata represent an exciting system for studying conspicuous color patterns due to their taxonomic and phenotypic diversity, and future studies in aculeates hold great promise for answering many outstanding questions concerning aposematism and mimicry.
Author Contributions
The author confirms being the sole contributor of this work and has approved it for publication.
Conflict of Interest
The author declares that the research was conducted in the absence of any commercial or financial relationships that could be construed as a potential conflict of interest.
Publisher’s Note
All claims expressed in this article are solely those of the authors and do not necessarily represent those of their affiliated organizations, or those of the publisher, the editors and the reviewers. Any product that may be evaluated in this article, or claim that may be made by its manufacturer, is not guaranteed or endorsed by the publisher.
Acknowledgments
I thank Bonnie Blaimer, Elsa Youngsteadt, and Brian Wiegmann for feedback on earlier drafts, Jen Schlauch for illustrating Figure 1, and two anonymous reviewers whose comments improved this manuscript.
References
Bates, H. W. (1862). Contributions to an insect fauna of the Amazon valley (Lepidoptera: Heliconidae). Trans. Linn. Soc. Lond. 23, 495–566. doi: 10.1111/j.1096-3642.1860.tb00146.x
Baxter, S. W., Papa, R., Chamberlain, N., Humphray, S. J., Joron, M., Morrison, C., et al. (2008). Convergent evolution in the genetic basis of Mullerian mimicry in Heliconius butterflies. Genetics 180, 1567–1577. doi: 10.1534/genetics.107.082982
Blaimer, B. B., Mawdsley, J. R., and Brady, S. G. (2018). Multiple origins of sexual dichromatism and aposematism within large carpenter bees. Evolution 72, 1874–1889. doi: 10.1111/evo.13558
Branstetter, M. G., Danforth, B. N., Pitts, J. P., Faircloth, B. C., Ward, P. S., Buffington, M. L., et al. (2017). Phylogenomic insights into the evolution of stinging wasps and the origins of ants and bees. Curr. Biol. 27, 1019–1025. doi: 10.1016/j.cub.2017.03.027
Briolat, E. S., Burdfield-Steel, E. R., Paul, S. C., Katja, H. R., Seymoure, B. M., and Stankowich, T. (2018). Diversity in warning coloration: selective paradox or the norm? Biol. Rev. 94, 388–414. doi: 10.1111/brv.12460
Brower, L. P., Van Zandt, J., and Corvino, J. M. (1967). Plant poisons in a terrestrial food chain. Proc. Natl. Acad. Sci. U.S.A. 57, S893–S898. doi: 10.1073/pnas.57.4.893
Cuthill, I. C., and Bennett, A. T. (1993). Mimicry and the eye of the beholder. Proc. R. Soc. Lond. Ser. B Biol. Sci. 253, 203–204. doi: 10.1371/journal.pcbi.1000821
Dittrigh, W., Gilbert, F., Green, P., McGregor, P., and Grewcock, D. (1993). Imperfect mimicry: a pigeon’s perspective. Proc. R. Soc. Lond. Ser. B 251, 195–200. doi: 10.1098/rspb.1993.0029
Dunning, D. C., and Kruger, M. (1995). Aposematic sounds in African moths. Biotropica 27, 227–231. doi: 10.2307/2388998
Eisner, T., and Grant, R. P. (1981). Toxicity, odor aversion and ‘olfactory aposematism’. Science 213:476. doi: 10.1126/science.7244647
Endler, J. (1980). Natural selection on color patterns in poecilia reticulata. Evolution 34, 76–91. doi: 10.2307/2408316
Endler, J. A., and Mappes, J. (2004). Predator mixes and the conspicuousness of aposematic signals. Am. Natural. 163, 532–547. doi: 10.1086/382662
Evans, H. (1968). Studies on neotropical pompilidae (Hymenoptera) IV. Examples of dual sex-limited mimicry in Chirodamus. Psyche 75, 1–22. doi: 10.1155/1968/76089
Ezray, B. D., Wham, D. C., Hill, C. E., and Hines, H. M. (2019). Unsupervised machine learning reveals mimicry complexes in bumblebees occur along a perceptual continuum. Proc. R. Soc. B 286:20191501. doi: 10.1098/rspb.2019.1501
Gabritschevsky, E. (1926). Convergence of coloration between American pilose flies and bumblebees (Bombus). Biol. Bull. 51, 269–286. doi: 10.2307/1536943
Gallant, J. R., Imhoff, V. E., Martin, A., Savage, W. K., Chamberlain, N. L., Pote, B. L., et al. (2014). Ancient homology underlies adaptive mimetic diversity across butterflies. Nat. Commun. 5:4817. doi: 10.1038/ncomms5817
Heliconius Genome Consortium. (2012). Butterfly genome reveals promiscuous exchange of mimicry adaptations among species. Nature 487, 94–98. doi: 10.1038/nature11041
Hines, H. M. (2008a). Bumble Bees (Apidae: Bombus) Through the Ages: Historical Biogeography and the Evolution of Colour Diversity. Champaign, IL: University of Illinois, Urbana-Champaign.
Hines, H. M. (2008b). Historical biogeography, divergence times, and diversification patterns of bumble bees (Hymenoptera: Apidae: Bombus). Syst. Biol. 57, 58–75. doi: 10.1080/10635150801898912
Hines, H. M., Witkowski, P., Wilson, J. S., and Wakamatsu, K. (2017). Melanic variation underlies aposematic color variation in two hymenopteran mimicry systems. PLoS One 12:e0182135. doi: 10.1371/journal.pone.0182135
Hristov, N. I., and Conner, W. E. (2005). Sound strategy: acoustic aposematism in the bat–tiger moth arms race. Naturwissenschaften 92, 164–169. doi: 10.1007/s00114-005-0611-7
Jiggins, C., Naisbit, R., Coe, R., and Mallet, J. (2001). Reproductive isolation caused by colour pattern mimicry. Nature 411, 302–305. doi: 10.1038/35077075
Joron, M., Frezal, L., Jones, R. T., Chamberlain, N. L., Lee, S. F., and Haag, C. R. (2011). Chromosomal rearrangements maintain a polymorphic supergene controlling butterfly mimicry. Nature 477, 203–206. doi: 10.1038/nature10341
Joron, M., Papa, R., Beltran, M., Chamberlain, N., Mavarez, J., Baxter, S., et al. (2006). A conserved supergene locus controls colour pattern diversity in Heliconius butterflies. PLoS Biol. 4:e303. doi: 10.1371/journal.pbio.0040303
Kapan, D. D., Flanagan, N. S., Tobler, A., Papa, R., Reed, R. D., Acevedo Gonzalez, J., et al. (2006). Localization of Mullerian mimicry genes on a dense linkage map of Heliconius erato. Genetics 173, 735–757. doi: 10.1534/genetics.106.057166
Kikuchi, D. W., and Pfennig, D. W. (2013). Imperfect mimicry and the limits of natural selection. Q. Rev. Biol. 88, 297–315. doi: 10.1086/673758
Kroiss, J., Strohm, E., Vandenbem, C., and Vigneron, J. P. (2009). An epicuticular multilayer reflector generates the iridescent coloration in chrysidid wasps (Hymenoptera, Chrysididae). Naturwissenschaften 96, 983–986. doi: 10.1007/s00114-009-0553-6
Kronforst, M. R., and Papa, R. (2015). The functional basis of wing patterning in Heliconius butterflies: the molecules behind mimicry. Genetics 200, 1–19. doi: 10.1534/genetics.114.172387
Kunte, K. (2008). Evolution of Sex-Limited Mimicry in Swallowtail Butterflies. Ph.D. thesis. Austin, TX: The University of Texas at Austin, 132.
Kunte, K., Zhang, W., Tenger-Trolander, A., Palmer, D. H., Martin, A., Reed, R. D., et al. (2014). Doublesex is a mimicry supergene. Nature 507, 229–232. doi: 10.1038/nature13112
Low, M. L., Naranjo, M., and Yack, J. E. (2021). Survival sounds in insects: diversity, function, and evolution. Front. Ecol. Evol. 9:91.
Mallet, J., and Barton, N. H. (1989). Strong natural selection in a warning-color hybrid zone. Evolution 43, 421–431. doi: 10.1111/j.1558-5646.1989.tb04237.x
Mallet, J., and Gilbert, L. E. (1995). Why are there so many mimicry rings? Correlations between habitat, behavior and mimicry in Heliconius butterflies. Biol. J. Linn. Soc. 55, 159–180. doi: 10.1111/j.1095-8312.1995.tb01057.x
Malm, T., and Nyman, T. (2015). Phylogeny of the symphytan grade of Hymenoptera: new pieces into the old jigsaw (fly) puzzle. Cladistics 31, 1–17. doi: 10.1111/cla.12069
Manley, D. G. (2000). “Defense adaptations in velvet ants (Hymenoptera: Mutillidae) and their possible selective pressures,” in Hymenoptera: Evolution, Biodiversity and BiologicalControl, eds A. D. Austin and M. Dowton (Collingwood, VIC: CSIRO Publishing), 285–289.
Mappes, J., Kokko, H., Ojala, K., and Lindstrom, L. (2014). Seasonal changes in predator community switch the direction of selection for prey defences. Nat. Commun. 5:5016. doi: 10.1038/ncomms6016
Marchini, M., Sommaggio, D., and Minelli, A. (2016). Playing with black and yellow: the evolvability of a Batesian mimicry. Evol. Biol. 44, 100–112. doi: 10.1007/s11692-016-9397-0
Marples, N. M., and Mappes, J. (2011). Can the dietary conservatism of predators compensate for positive frequency dependent selection against rare, conspicuous prey? Evol. Ecol. 25, 737–749.
Martin, A., Papa, R., Nadeau, N. J., Hill, R. I., Counterman, B. A., Halder, G., et al. (2012). Diversification of complex butterfly wing patterns by repeated regulatory evolution of a Wnt ligand. Proc. Natl. Acad. Sci. U.S.A. 109, 12632–12637. doi: 10.1073/pnas.1204800109
McIver, J. D., and Stonedahl, G. (1993). Myrmecomorphy: morphological and behavioral mimicry of ants. Annu. Rev. Entomol. 38, 351–377.
McMillan, W. O., Livraghi, L., Concha, C., and Hanly, J. J. (2020). From patterning genes to process: unraveling the gene regulatory networks that pattern Heliconius wings. Front. Ecol. Evol. 8:221. doi: 10.3389/fevo.2020.00221
Mora, R., and Hanson, P. E. (2019). Widespread occurrence of black-orange-black color pattern in Hymenoptera. J. Insect Sci. 19, 13. doi: 10.1093/jisesa/iez021
Nemésio, A. (2005). Fluorescent colors in orchid bees (Hymenoptera: Apidae). Neotrop. Entomol. 34, 933–936.
Nishikawa, H., Iijima, T., Kajitani, R., Yamaguchi, J., Ando, T., Suzuki, Y., et al. (2015). A genetic mechanism for female-limited Batesian mimicry in Papilio butterfly. Nat. Genet. 47, 405–409. doi: 10.1038/ng.3241
Ojala, K., Lindström, L., and Mappes, J. (2007). Life-history constraints and warning signal expression in an arctiid moth. Funct. Ecol. 21, 1162–1167. doi: 10.1111/j.1365-2435.2007.01322.x
Pan, A. D., Williams, K. A., and Wilson, J. S. (2017). Are diurnal iguanian lizards the evolutionary drivers of New World female velvet ant(Hymenoptera: Mutillidae) Müllerian mimicry rings? Biol. J. Linn. Soc. 120, 436–447. doi: 10.1111/bij.12894
Pekár, S., Petráková, L., Bulbert, M. W., Whiting, M. J., and Herberstein, M. E. (2017). The golden mimicry complex uses a wide spectrum of defence to deter a community of predators. Elife 6:e22089. doi: 10.7554/eLife.22089
Penney, H. D., Hassall, C., Skevington, J. H., Abbott, K. R., and Sherratt, T. N. (2012). A comparative analysis of the evolution of imperfect mimicry. Nature 483, 461–464. doi: 10.1038/nature10961
Perrard, A., Arca, M., Rome, Q., Muller, F., Tan, J., Bista, S., et al. (2014). Geographic variation of melanisation patterns in a hornet species: genetic differences, climatic pressures or aposematic constraints? PLoS One 9:e94162. doi: 10.1371/journal.pone.0094162
Peters, R. S., Krogmann, L., Mayer, C., Donath, A., Gunkey, S., Meusemann, K., et al. (2017). Evolutionary history of the Hymenoptera. Curr. Biol. 27, 1013–1018. doi: 10.1016/j.cub.2017.01.027
Pianka, E. R. (1967). On lizard species diversity: North American flatland deserts. Ecology 48, 333–351. doi: 10.2307/1932670
Plowright, R. C., and Owen, R. E. (1980). The evolutionary significance of bumble bee color patterns: a mimetic interpretation. Evolution 34, 622–637. doi: 10.2307/2408017
Rojas, B., Burdfield-Steel, E., De Pasqual, C., Gordon, S., Hernández, L., Mappes, J., et al. (2018). Multimodal aposematic signals and their emerging role in mate attraction. Front. Ecol. Evol. 6:93. doi: 10.3389/fevo.2018.00093
Rojas, B., Valkonen, J. K., and Nokelainen, O. (2015). Aposematism. Curr. Biol. 25, R350–R351. doi: 10.1016/j.cub.2015.02.015
Rowe, C., and Halpin, C. (2013). Why are warning displays multimodal? Behav. Ecol. Sociobiol. 67, 1425–1439. doi: 10.1007/s00265-013-1515-8
Rowland, H. M., Mappes, J., Ruxton, G. D., and Speed, M. P. (2010). Mimicry between unequally defended prey can be parasitic: evidence for quasi-Batesian mimicry. Ecol. Lett. 13, 1494–1502. doi: 10.1111/j.1461-0248.2010.01539.x
Ruxton, G. D., Allen, W. L., Sherratt, T. N., and Speed, M. P. (2018). Avoiding Attack, 2nd Edn. Oxford: Oxford University Press, doi: 10.1093/oso/9780199688678.001.0001
Sann, M., Niehuis, O., Peters, R. S., Mayer, C., Kozlov, A., Podsiadlowski, L., et al. (2018). Phylogenomic analysis of Apoidea sheds new light on the sister group of bees. BMC Evol. Biol. 18:71. doi: 10.1186/s12862-018-1155-8
Schmidt, J. O. (2004). Venom and the good life in tarantula hawks (Hymenoptera: Pompilidae): how to eat, not be eaten, and live long. J. Kansas Entomol. Soc. 77, 402–413. doi: 10.2317/E-39.1
Schmidt, J. O., and Blum, M. S. (1977). Adaptations and responses of Dasymutilla occidentalis (Hymenoptera: Mutillidae) to predators. Entomol. Exp. Appl. 21, 99–111. doi: 10.1111/j.1570-7458.1977.tb02663.x
Shamim, G., Ranjan, S. K., Pandey, D. M., and Ramani, R. (2014). Biochemistry and biosynthesis of insect pigments. Eur. J. Entomol. 111, 149–164. doi: 10.14411/eje.2014.021
Sheppard, P. M., Turner, J. R. G., Brown, K. S., Benson, W. W., and Singer, M. C. (1985). Genetics and the evolution of Muellerian mimicry in Heliconius butterflies. Philos. Trans. R. Soc. B Biol. Sci. 308, 433–610. doi: 10.1098/rstb.1985.0066
Skelhorn, J., Halpin, C. G., and Rowe, C. (2016). Learning about aposematic prey. Behav. Ecol. 27, 955–964.
Skelhorn, J., and Rowe, C. (2007). Automimic frequency influences the foraging decisions of avian predators on aposematic prey. Anim. Behav. 74, 1563–1572. doi: 10.1016/j.anbehav.2007.03.021
Speed, M. P. (1990). Mimicry and the Psychology of Predation. Doctoral dissertation. Leeds: University of Leeds.
Stiles, E. W. (1979). Evolution of color pattern and pubescence characteristics in male bumblebees: automimicry vs. thermoregulation. Evolution 33, 941–957. doi: 10.2307/2407657
Stuart-Fox, D., Newton, E., and Clusella-Trullas, S. (2017). Thermal consequences of colour and near-infrared reflectance. Philos. Trans. R. Soc. B Biol. Sci. 372:B37220160345. doi: 10.1098/rstb.2016.0345
Tian, L., Rahman, S. R., Ezray, B. D., Franzini, L., Strange, J. P., Lhomme, P., et al. (2019). A homeotic shift late in development drives mimetic color variation in a bumble bee. Proc. Natl. Acad. Sci. U.S.A. 116, 11857–11865. doi: 10.1073/pnas.1900365116
Tullberg, B. S., Merilaita, S., and Wiklund, C. (2005). Aposematism and crypsis combined as a result of distance dependence: functional versatility of the colour pattern in the swallowtail butterfly larva. Proc. R. Soc. B Biol. Sci. 272, 1315–1321. doi: 10.1098/rspb.2005.3079
Urban, S., Nater, A., Meyer, A., and Kratochwil, C. F. (2021). Different sources of allelic variation drove repeated color pattern divergence in cichlid fishes. Mol. Biol. Evol. 38, 465–477. doi: 10.1093/molbev/msaa237
Vitt, L. J., and Cooper, W. E. (1988). Feeding responses of skinks (Eumeces laticeps) to velvet ants (Dasymutilla occidentalis). J. Herpetol. 22, 485–488. doi: 10.2307/1564347
Vogt, O. (1909). Studien über das Art Problem. 1. Mitteilung. Über das Variieren der Hummeln. 1. Teil. Sitzungsberichte der Gesellschaft Naturforschender Freunde Zu Berlin. 28–84.
Wham, D. C., Ezray, B., and Hines, H. M. (2019). Measuring perceptual distance of organismal color pattern using the features of deep neural networks. bioRxiv [Preprint] doi: 10.1101/736306
Williams, P. H. (2007). The distribution of bumblebee colour patterns world-wide: possible significance for thermoregulation, crypsis, and warning mimicry. Biol. J. Linn. Soc. 92, 97–118. doi: 10.1111/j.1095-8312.2007.00878.x
Wilson, J. S., Jahner, J. P., Forister, M. L., Sheehan, E. S., Williams, K. A., and Pitts, J. P. (2015). North American velvet ants form one of the world’s largest known Müllerian mimicry complexes. Curr. Biol. 25, R704–R706. doi: 10.1016/j.cub.2015.06.053
Wilson, J. S., Jahner, J. P., Williams, K. A., and Forister, M. L. (2013). Ecological and evolutionary processes drive the origin and maintenance of imperfect mimicry. PLoS One 8:e0061610. doi: 10.1371/journal.pone.0061610
Wilson, J. S., Pan, A. D., Limb, E. S., and Williams, K. A. (2018). Comparison of African and North American velvet ant mimicry complexes: another example of Africa as the ‘odd man out’. PLoS One 13:e0189482. doi: 10.1371/journal.pone.0189482
Wilson, J. S., Sidwell, J. S., Forister, M. L., Williams, K. A., and Pitts, J. P. (2020). Thistledown velvet ants in the Desert Mimicry Ring and the evolution of white coloration: Müllerian mimicry, camouflage and thermal ecology. Biol. Lett. 16:20200242. doi: 10.1098/rsbl.2020.0242
Keywords: aposematism, mimicry, Aculeata hymenoptera, predator-prey interactions, visual signals, defense
Citation: Willadsen PC (2022) Aculeate Hymenopterans as Aposematic and Mimetic Models. Front. Ecol. Evol. 10:827319. doi: 10.3389/fevo.2022.827319
Received: 01 December 2021; Accepted: 18 January 2022;
Published: 10 February 2022.
Edited by:
Ann Valerie Hedrick, University of California, Davis, United StatesReviewed by:
Stano Pekar, Masaryk University, CzechiaMelissa R. L. Whitaker, ETH Zürich, Switzerland
Copyright © 2022 Willadsen. This is an open-access article distributed under the terms of the Creative Commons Attribution License (CC BY). The use, distribution or reproduction in other forums is permitted, provided the original author(s) and the copyright owner(s) are credited and that the original publication in this journal is cited, in accordance with accepted academic practice. No use, distribution or reproduction is permitted which does not comply with these terms.
*Correspondence: Peter C. Willadsen, cGN3aWxsYWRAbmNzdS5lZHU=