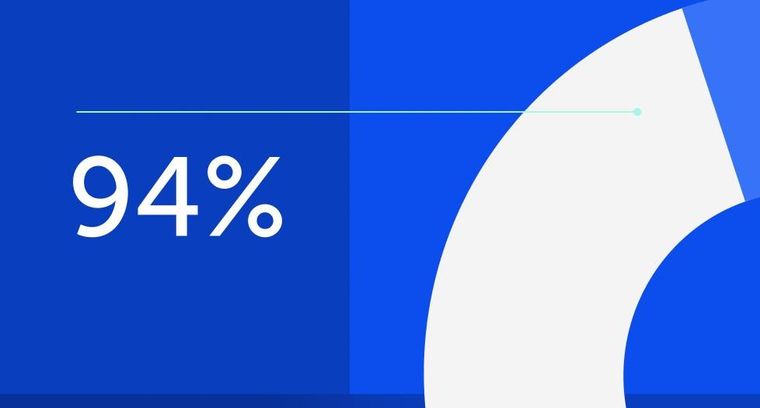
94% of researchers rate our articles as excellent or good
Learn more about the work of our research integrity team to safeguard the quality of each article we publish.
Find out more
ORIGINAL RESEARCH article
Front. Ecol. Evol., 15 March 2022
Sec. Behavioral and Evolutionary Ecology
Volume 10 - 2022 | https://doi.org/10.3389/fevo.2022.825110
This article is part of the Research TopicNesting in Reptiles: Natural and Anthropogenic Threats and Evolutionary ResponsesView all 18 articles
Climate warming is forecasted to cause extinctions, but populations could theoretically avoid extinction in a rapidly changing environment via adaptive evolution (i.e., evolutionary rescue), precluding the need for intervention. Although strong links between a changing climate and the physiology of an organism are expected, climate effects can be buffered by behavior. Nest site choice behavior, for example, can reduce environmental variation that would be experienced by embryos placed randomly with respect to environmental temperatures. We tested four provisions of this prediction by quantifying nest sites and “potential” nest sites in the Florida softshell turtle (Apalone ferox). First, turtles chose nest sites with mean canopy openness values (32–47%) that were intermediate between the shadiest (14–17%) and the sunniest potential nest sites (36–57%) available. Second, canopy openness, incident radiation intensity, and nest temperatures were generally, positively related to one another, indicating definitive thermal consequences of nest site choice. Third, our study revealed ample, cooler nest sites available to turtle mothers within close proximity to nest sites utilized; by nesting in the most shaded sites, softshell turtle mothers could depress mean nest temperatures by ∼2°C. Fourth, the growth of vegetative cover throughout incubation had negligible effects on canopy openness, incident radiation intensity, and nest temperatures, supporting the potential for mothers to “predict” developmental temperatures using temperature cues during nest site choice. Finally, our data revealed considerable variation in canopy openness chosen by nesting mothers; such behavior could thus, be subject to natural selection via embryonic mortality under future warming. Collectively, our study suggests that Florida softshell turtles, and probably other turtle species nesting in relatively open areas, may be able to counter climate change effects on developing embryos by nesting in more shaded microhabitats, assuming nest site choice behavior is heritable and can evolve at a sufficient rate to keep pace with climate warming. The evolutionary and behavioral mechanisms (e.g., assessing substrate temperatures directly vs. indirect choice of canopy cover) in the repertoire of nesting mother turtles for responding to climate warming remain elusive and are required for a more complete understanding of climate responses.
Current, anthropogenic climate change is projected to continue indefinitely, and is putting pressure on biological systems due to its unprecedented rate (Intergovernmental Panel on Climate Change [IPCC], 2019). Drivers of this change include sea level rises, frequencies of rainfall, wind and storms, ocean acidification, and eutrophication, but the most direct and predictable driver is increased air and surface temperatures (Intergovernmental Panel on Climate Change [IPCC], 2007). As such, understanding potential responses to increasing environmental temperatures has become a major focus of ecological and evolutionary biology (e.g., Parmesan, 2006; Pacifici et al., 2017; Radchuk et al., 2019), especially given the current biodiversity crisis (Barnosky et al., 2011; Urban, 2015). Ideally for conservationists, populations can avoid extinction in a rapidly changing environment via adaptive evolution, precluding the need for intervention (i.e., “evolutionary rescue”; Bell, 2017).
Predicting evolutionary responses to climate change is not trivial, and requires understanding links between environmental temperatures and key life processes. Changes in environmental temperatures can alter body temperatures, which in turn affect physiological processes and performance, and thus potentially survival, especially in ectotherms (Huey et al., 2003, 2012; Kearney et al., 2009 and papers cited within). Although an organism’s vulnerability to climate warming is complex and could involve other climate change drivers and changes in a plethora of biotic and abiotic interactions, understanding an organism’s response to temperature is fundamental to predicting that vulnerability (Kearney et al., 2009; Huey et al., 2012).
Although strong links between a changing climate and the physiology of an organism are expected, climate effects can be buffered by behavior. Using the example that lizards at higher (cooler) elevations basked more, resulting in body temperatures that were more similar among different elevations than would be expected from null models, Huey et al. (2003) coined and described the “Bogert effect,” whereby behavioral adjustments can reduce the environmental variation that would be experienced by a non-regulating ectothermic organism. The authors demonstrated that thermoregulatory behaviors likely inhibit selection for evolutionary shifts in thermal physiology across environmental gradients, a notion somewhat counter to the classic “behavioral drive” theory proposing that behavior initiates new evolutionary events (Mayr, 1963).
Doody and Moore (2010) proposed that the Bogert effect could be extended to the egg or embryo stage, after finding (predictable) latitudinal and elevational variation in nest site attributes in a lizard (Doody et al., 2006a; Doody, 2009). In other words, nest site choice behavior can reduce environmental variation that would be experienced by embryos placed randomly with respect to environmental temperatures (Doody and Moore, 2010). It follows that nest site choice could theoretically also buffer developing embryos in nests against temporal environmental gradients such as climate change (Doody et al., 2006a; Angilletta et al., 2009).
Turtle nesting behaviors offer desirable systems for studying potential climate change responses in nature; turtles are oviparous ectotherms that lay eggs in ground nests that are typically subject to air and ground temperatures for 2–3 months. Moreover, many turtle species possess temperature-dependent sex determination (Ewert et al., 1994; Valenzuela and Lance, 2004) meaning that choice of nest site can influence offspring sex ratios (Vogt and Bull, 1984; Janzen, 1994). While free-living adults and juveniles can seek cooler microclimates as the climate warms, the eggs cannot. Moreover, like most oviparous ectotherms, turtles rarely exhibit parental care after laying (Shine, 1988), focusing research attention to the mother’s nesting decisions as the main avenue for countering climate change effects of temperatures on developing embryos. Indeed, two studies have unequivocally demonstrated mother reptiles used nest site choice behavior—specifically the choice of canopy cover—to offset environmental gradients across latitudes and elevations (Ewert et al., 2005; Doody et al., 2006a). Both snapping turtles (Chelydra serpentina) and water dragons (Intellagama lesueurii) nested under more open canopies in cooler climates than their counterparts in warmer climates. Such variation strongly suggests that mothers could also use canopy cover to offset climate warming (Doody et al., 2006a; Doody and Moore, 2010) because canopy cover is highly correlated with nest temperatures (Ewert et al., 1994; Janzen, 1994; Janzen and Morjan, 2001).
The Florida softshell turtle, Apalone ferox, is a common but geographically restricted species that nests in terrestrial areas around a wide variety of freshwater wetlands in hot climates (Krysko et al., 2019). Although the species is thought to possess genetic sex determination due to the finding of sex chromosomes in a congener (Badenhorst et al., 2013), recent research indicates that offspring sex determination may be thermosensitive in the genus (Bista et al., 2021). Although nests can be difficult to find, nest predation, mainly by raccoons (Procyon lotor), is often high in A. ferox (G. L. Heinrich, unpubl. data, J. S. Doody, unpubl. data), as in many other turtle populations (e.g., Congdon et al., 1994; Burke et al., 1998; Tomás et al., 2010), providing a solution to finding large numbers of nests. Raccoons preying upon turtle nests leave the eggshells on the surface near the excavated nest. While turtle ecologists aiming to quantify nest site choice generally use intact nests, depredated nests can facilitate quantifying nest site choice attributes such as amount of shading vegetation, distance from and height above water, aspect, and distance to other nests. These attributes reflect some combination of direct and indirect behavioral choices. In particular, canopy or understory openness can be quantified using hemispherical photography, and incident solar radiation intensity striking the nest site can be subsequently estimated using gap light analysis (Frazer et al., 1999; Doody et al., 2006a,b).
Assuming that nest site choice behavior is heritable and can freely evolve at a rate to keep pace with the rate of climate change, we predict that softshell turtle mothers could use nest site choice behavior to offset current and future climate warming by buffering eggs against the effects of increasing temperatures on developing embryos (Doody et al., 2006a), provided that (1) there are potential nest sites with more shading vegetation available to nesting mothers, that (2) exhibit cooler nest temperatures, (3) attributes (e.g., shading vegetation) of nest sites affecting developmental temperatures during nest site choice do not change markedly throughout incubation, and (4) there is sufficient individual variation in canopy openness of nest sites chosen by mothers. We tested these provisions by quantifying nest site choice, potential nest site choice, and consequences of those choices in nest and potential nest temperatures in the Florida softshell turtle in three populations in south Florida. We also quantified seasonal timing of nesting to clarify seasonal changes in nest temperatures. We discuss the implications of our findings for the evolution of nest site choice and climate change responses in turtles, reptiles, and other oviparous ectotherms without parental care.
The Florida softshell turtle is a large, common species inhabiting a wide variety of freshwater habitats in Florida and surrounding states (Krysko et al., 2019). Although it does not range widely across latitudes, it is essentially allopatric with its closest relatives that do span considerable latitudes (A. mutica and A. spinifera). Florida softshell turtles typically nest in relatively open, sandy areas along watercourses; nests tend to be close to water when suitable sites are available, but have been found > 160 m from water (G. L. Heinrich, unpubl. data). Like most other turtles, A. ferox constructs a flask-shaped hole in the ground with the hind feet and backfills the hole after laying. Based on dissections from turtles harvested for meat in south Florida, mothers lay up to 5–6 clutches of 9–38 eggs annually between early March and early August (Iverson and Moler, 1997). Eggs incubate for 56–82 days depending on incubation temperatures (Meylan and Moler, 2006).
We studied softshell nesting at three sites in southwest Florida: Boyd Hill Nature Preserve (BHNP), Myakka River State Park (MRSP), and Sawgrass Lake Park (SLP). BHNP is a 97-ha city park in St. Petersburg, Pinellas County (27.734160°N, -82.65635°W). The BHNP nesting areas bordered Lake Maggiore, a 147-ha permanent lake. MRSP is a 15,054-ha state park in Sarasota and Manatee counties (27.252255°N, -82.295581°W). Nesting areas in MRSP were along canals near the Myakka River. SLP is a 162-ha county park in St. Petersburg, Pinellas County (27.837384°N, -82.665372°W). Nesting areas at SLP were mainly along a manmade pond, but also along a canal feeding Sawgrass Lake. BHNP and SLP are ∼11 km apart, while MRSP is ∼64 km southeast of BHNP and ∼75 km southeast of SLP.
Nest surveys were conducted on foot in riparian areas along lakes, ponds, or canals in 2019 from March to September at BHNP (6 days/week) and MRSP (twice/week), and May to September at SLP (once every 3 weeks). Nests were found by looking for eggshells left by nest predators (Figure 1). Apalone ferox eggshells are composed of a brittle outer shell that is easily distinguishable from other species. The main predator was the raccoon and depredated nests featured an empty excavated nest chamber with eggshells scattered on the ground within a few meters of the chamber.
Figure 1. Seasonal timing of nesting of A. ferox at BHNP, the study site with the most survey coverage (six visits/week).
Nests were flagged for further processing; data on nest site choice included estimated lay date, aspect (measured with a compass), distance from water (measured with a meter stick), and ground cover (estimated by photographing the square meter surrounding each nest to the nearest 10%). Within 1 week of the estimated lay date, we measured openness of the canopy and understory, and incident radiation intensity using hemispherical photography and gap light analysis (after Doody et al., 2006a,b). Hemispherical photographs were taken by placing a Nikon Coolpix® 900 series digital camera fitted with a fisheye wide-angle lens (Nikon FC-E8®) on each nest and taking a photograph with the camera body facing due north (the fisheye lens pointing upward). Photographs were taken during the first or last hour of daylight to preclude reflection of light off vegetation (i.e., leaves) that can introduce error in the calculation of openness. Openness (%) and incident radiation intensity were calculated by running each photograph through Gap Light Analyzer (GLA) 2.0® (Frazer et al., 1999).
To facilitate comparing our canopy openness and incident radiation data results to those from other species we herein list the settings we used in Gap Light Analyzer 2.0® (Frazer et al., 1999). We pointed the camera due north during hemispherical photographs to allow the program to accurately track the path of the sun across the image. Under configuration/image, we used geographic north rather than magnetic north; although the latter gives a slightly more accurate compass direction due to the dynamic nature of earth’s magnetic field, the correction is minor and both attributes provide a relative measure. Under configuration/site, we entered the latitude of the site (allows the program to know where the sun tracks across the photograph), but not elevation or longitude. We used horizontal orientation (no significant slope or aspect) and we turned the topographic mask to “OFF” (there was no need to separate the canopy from mountains on the horizon when determining shading). Under configuration/resolution, we left the solar time step at the default of 2 min., we left the sky regions at the default (36, 9), and the start date and end date were chosen based on the lay date and hatching date, respectively. The hatching date was estimated to be 60 days after the lay date, based on data from Meylan and Moler (2006). Under configuration/radiation, we used the “modeled data source” (computes above-canopy radiation estimates without entering data from each site); we used Megajoules/m2/day as the units, the UOC model (assumes that all regions of the sky are equally as bright), and we ignored cloudiness index (this is useful when comparing multiple sites in which there is a consistent difference in cloudiness at one or some sites, but not others). Finally, under calculations, we ticked canopy structure, and we used % openness and “trans total radiation” as our outputs for canopy openness and incident radiation intensity, respectively.
To quantify the thermal consequences of nest site choice, specifically openness and incident radiation, we deployed temperature data loggers (Thermochron i-buttons®; DS1921G; accuracy = ± 1.0°C) into 13 randomly-chosen, backfilled, depredated nests at BHNP. Loggers were placed at the average nest chamber depth (16.0 cm), based on the average of an intact nest at BHNP (21.0 cm) and from two nests from previous reports (13.0 cm, 14.0 cm; Hamilton, 1947; Heinrich and Richardson, 1993). Data loggers were deployed within 1 week of the estimated lay date and set to record temperature every 90 min. Analyses using temperature data from data loggers utilized only the first 60 days of data, a typical incubation period for the species (Meylan and Moler, 2006).
To determine if our temperature data from backfilled nests accurately represented real nest temperatures we tested for metabolic heating in one nest (Massey et al., 2019). We employed a data logger into the core of a fresh nest and a second data logger 8.0 cm away in the ground, at the same depth (12.0 cm). The nest was caged with hardware wire to protect the eggs from predators, and data loggers were removed 56 days later for analysis.
To determine if mothers could offset future climate warming by nesting in more shaded areas, we quantified openness and incident radiation from the “most shaded” potential nest site within 20 m of each nesting area (a nesting area here is defined as a cluster of nests within 20 m of one another, within a study site). For comparison, we also did the same for the “sunniest” (most open, canopy-wise) potential nest site for each nesting area (same criteria as for the shaded potential nest sites). As with nest sites, we quantified the thermal consequences of potential nest sites using i-button data loggers. There were 10 such potential nest sites at BHNP and five at MRSP (we did not quantify potential nest sites at SLP). Data loggers recorded temperatures at the same intervals as those in nests (every 90 min) and were buried in artificial nests at the same depth as those in actual nests (16.0 cm).
Because turtle eggs typically incubate in the ground for 2–3 months during the growing season, vegetation such as grasses and understory can grow significantly during that time and potentially change the amount of solar radiation that strikes the nest site. This raises the question of how well mothers can predict nest temperatures throughout incubation by sampling nesting conditions during nest site choice. To address this, we again quantified openness and incident radiation at the nest sites and potential nest sites at 30 and 60 days of incubation for each nest, using the same methods as we did for actual nests (above).
We tested normality and homogeneity of variance using a Shapiro–Wilk test and a Levene’s test, respectively. We used t-tests to compare openness and incident radiation intensity between nest sites and potential nest sites (sunny and shaded sites), and a Kruskal–Wallis test and Dunn’s multiple comparison ad hoc test to analyze differences in openness and incident radiation intensity among study sites. To test for seasonal differences in openness, incident radiation intensity, and ground cover we used a Friedman test with a post hoc Conover test. To explore the effects of openness, study site, and lay date on incident radiation intensity we used a linear regression model. We fit six regression models with different combinations of lay date, nest site, and percent openness. We used Akaike’s Information Criterion with a small sample bias adjustment (AICc) to assess the best model. We used 95% confidence intervals to assess the precision of the parameters of the model. Linear regression analysis was used to test whether canopy openness and incident radiation intensity were related to mean, maximum, and minimum temperatures. All analyses were performed using R 3.6.1 (R Core Team, 2018) with the packages “FSA,” “PMCMR,” “lmtest,” and “MuMIn.”
We found 156 softshell nests, including 102 from BHNP, 27 from MRSP, and 27 from SLP. Most nesting occurred between late March and mid-July and over 50% of the year’s nesting was completed by 1 June (Figure 1). All nests were taken by predators; the presence of eggshells on the surface was consistent with predation by raccoons, but it is possible that some were taken by other predators; crows (Corvus spp.) are also nest predators at the sites (JSD and GLH, pers. obs.).
Mean canopy openness for all nests was 36.9 ± 13.92% and was significantly different among study sites (Figure 2; Kruskal–Wallis: H = 23, df = 2, p < 0.0001); openness of nest sites at MRSP was significantly greater than openness of nest sites at both BHNP (p < 0.0001) and SLP (P < 0.0002). Mean canopy openness did not differ between BHNP and SLP (p = 0.375). At BHNP, mean canopy openness of nest sites was 35.3 ± 14.55% (range = 8.7–66.7%, N = 102), compared to 46.8 ± 10.04% (range = 21.4–70.9%, N = 27) at MRSP and 32.7 ± 10.07% (range = 19.3–53.3%, N = 27) at SLP.
Figure 2. Canopy openness and incident radiation intensity at A. ferox nest sites across three study sites. Boyd, BHNP in text; Myakka, MRSP; Sawgrass, SLP.
Canopy openness values of nest sites consistently fell in between those for shaded and sunny potential nest sites. Although openness values for nest sites were more often closer to those of sunny potential nest sites, at some nesting areas they were closer to values of shaded potential nest sites (Figure 3). At BHNP, mean canopy openness of nests was significantly greater than shaded potential nest sites (Figure 3; t = 11.26, df = 110, p < 0.0001); although nest sites were generally less open than sunny potential nest sites, the difference was not statistically significant (t = 0.80, df = 110, p = 0.440). At MRSP, mean canopy openness of nests was significantly greater than shaded potential nest sites (Figure 3; t = 4.89, df = 30, p < 0.0001) and significantly lower than in sunny potential nest sites (t = 2.60, df = 30, p = 0.0145).
Figure 3. Comparison of canopy openness and incident radiation intensity between A. ferox nest sites and potential nest sites across study sites. (Top graph) population-level results across the three study sites. (Bottom graph) individual-level data within specific nesting areas (e.g., B2) across two study sites. Potential nest sites comprised those in full sun and full shade and were within 20 m of nest sites. Note that values for nest sites generally fell in between sun and shade potential nest sites. Boyd = BHNP in text; Myakka = MRSP.
As expected, canopy openness was highly, significantly positively related to incident radiation intensity (Figure 4; r2 = 0.848, F1,54 = 836.0, p < 0.0001). The mean incident radiation intensity for all nests combined was 5.6 ± 2.06 MJ/m2/d, but like canopy openness, was also significantly different among the study sites (Figure 2; Kruskal–Wallis: H = 23.02, df = 2, p < 0.0001). Incident radiation intensity was significantly higher at MRSP than at BHNP (Z = -4.24, df = 1, p < 0.0001) and at SLP (Z = 4.41, df = 1, p < 0.0001); incident radiation intensity did not differ between BHNP and SLP (Z = 1.31, df = 1, p = 0.189).
Figure 4. The strongly significant, positive relationship between incident radiation intensity and openness at A. ferox nest sites.
Mean incident radiation intensity of the sunny potential nest sites at all study sites combined was 7.4 ± 1.92 MJ/m2/day. As with canopy openness, incident radiation intensity at nest sites generally fell between the values for shaded and sunny potential nest sites (Figure 3). Mean incident radiation intensity of sunny potential nest sites was significantly higher than nest sites at MRSP (t = 2.65, df = 30, p = 0.013), but not at BHNP (t = 1.82, df = 110; p = 0.097; Figure 3). Mean incident radiation intensity of shaded potential nest sites at all study sites was 2.3 ± 1.41 SD MJ/m2/d (Figure 3). Mean incident radiation intensity of shaded potential nest sites was significantly lower than nest sites at both BHNP (t = 10.74, df = 110, p < 0.0001) and MRSP (t = 6.13, df = 30, p < 0.0001) (Figure 3).
At BHNP, mean incident radiation intensity of nest sites was significantly greater than that of shaded potential nest sites (t = 10.74, df = 111, p < 0.0001) and significantly lower than that for sunny potential nest sites (t = 1.82, df = 110, p = 0.097; Figure 3). The same relationships were found for MRSP: mean incident radiation intensity of nest sites was significantly greater than that of shaded potential nest sites (t = 4.28, df = 30, p = 0.01), but significantly lower than that of sunny potential nest sites (t = 4.60, df = 30, p < 0.0001; Figure 3). A linear regression model revealed that incident radiation intensity was explained by canopy openness (Figure 4; r2 = 0.864; t = 26.49, p < 0.0001), lay date (t = -2.93, p = 0.0040), and study site (t = 2.20, p = 0.0292).
Canopy openness and incident radiation intensity decreased significantly with season at BHNP, but not at MRSP (Figure 5). At BHNP, openness decreased significantly (X2 = 53.33, df = 2, p < 0.0001) between day 0 and day 30 (p < 0.0001) and between day 30 and day 60 (p < 0.0001) (Figure 5). Also at BHNP, incident radiation intensity decreased significantly (X2 = 30.62, df = 2, p < 0.0001) between day 30 and day 60 (p < 0.0001), but not between day 0 and day 30 (p = 0.830; Figure 5). In contrast, at MRSP openness did not change significantly over the season (X2 = 4.38, df = 2, p = 0.112), nor did incident radiation intensity (X2 = 1.46, df = 2, p = 0.482; Figure 5).
Figure 5. Seasonal changes in ground cover, openness and incident radiation intensity at A. ferox nest sites at Boyd (BHNP in text) and Myakka (MRSP).
Ground cover increased significantly with season at both BHNP (X2 = 98.22, df = 2, p < 0.0001) and MRSP (X2 = 11.28, df = 2, p = 0.004; Figure 5). At BHNP, ground cover increased significantly between day 0 and day 30 (p < 0.0001) and between day 30 and day 60 (p < 0.0001; Figure 5). Similarly, ground cover increased significantly between day 0 and day 30 (p = 0.005) and between day 30 and day 60 (p < 0.0001) at MRSP (Figure 5).
Nest temperatures tracked air temperatures, warming throughout the nesting season and leveling off in July-August as cloud cover, rainfall, and vegetative cover increased (Figure 6). Continuous nest temperatures from the core of a fresh nest were nearly identical to those in the soil 8.0 cm from the nest at the same depth, for most of the incubation period (Figure 7). Nest temperatures were ∼0.5 C higher in the last 13 days of incubation (Figure 7), indicating a small effect of metabolic heating of eggs in this warm climate.
Figure 7. (Minimal) Effect of metabolic heating in a clutch of A. ferox eggs incubating in situ. Eggs in the core of the clutch were up to ∼0.5°C warmer than the soil adjacent to the clutch during the last ∼16 days of incubation.
Grand mean nest site temperatures differed significantly among nest sites and potential nest sites (F2,25 = 26.81, p < 0.0001) and were, on average, 1.9°C warmer than shaded potential nest site temperatures (Tukey’s HSD, p < 0.0001), and 0.8°C cooler than sunny potential nest site temperatures (p = 0.078). Similarly, grand mean maximum nest site temperatures differed significantly among nest sites and potential nest sites (F2,25 = 15.07, p < 0.0001) and were 2.6°C warmer than those in shaded potential nest sites (p = 0.009) and 1.9°C cooler than those in sunny potential nest sites (p = 0.059). Conversely, grand mean minimum nest temperatures did not differ significantly among nest sites and potential nest sites (F2,25 = 1.91, p = 0.170).
Nest temperatures generally increased with increasing openness and increasing incident radiation intensity (Figure 8), but not all of these relationships were statistically significant (Table 1). Grand mean temperatures and mean maximum temperatures were both significantly positively related to canopy openness at shaded and sunny potential nest sites, but not at nest sites (Figure 8 and Table 1). Similarly, grand mean temperatures and grand mean maximum temperatures were both significantly, positively related to incident radiation intensity at nest sites, shaded potential nest sites, and sunny potential nest sites (Figure 8 and Table 1). Grand mean minimum temperatures were not significantly related to openness or incident radiation intensity at nest sites, shaded potential nest sites, or sunny potential nest sites (Figure 8 and Table 1).
Figure 8. Relationships between mean and maximum (back-filled) nest temperatures and either openness or incident radiation intensity of A. ferox nest sites and potential nest sites. Potential nest sites included the shadiest and sunniest sites within 20 m of each nesting area.
Table 1. Relationships of canopy openness and incident radiation intensity to temperatures (grand mean, maximum, and minimum) from nest sites and potential nest sites (sun, shade).
Considerable variation existed in both the openness and incident radiation intensity of nest sites at BHNP (Figure 9). An order of magnitude separated the lowest and highest values for both openness and incident radiation intensity (Figure 9).
Figure 9. Frequency distribution of openness and incident radiation intensity of A. ferox nest sites at BHNP, showing marked variation, most of which reflected the efforts of different mothers.
Oviparous ectothermic animals can theoretically offset climate warming effects on developing embryos by nesting in cooler microhabitats, given certain assumptions such as the heritability of nest site choice behaviors, the rate of climate change relative to the rate of evolutionary response, and the availability of cooler potential nest sites. Surprisingly few studies have quantified the availability of thermally heterogeneous potential nest sites in oviparous animals within the context of climate change (but see Refsnider et al., 2013a; Czaja et al., 2020). Our study produced four lines of evidence that softshell turtle mothers may be able to use nest site choice behavior to offset climate warming effects on incubation temperatures. First, shaded potential nest sites (essentially those in full shade) were available in close proximity to nest sites at all three study sites. Second, we revealed thermal consequences of nesting in shade; variation in openness within and among nest sites and potential nest sites influenced the amount of incident radiation received in nests, and ultimately, nest temperatures (Figures 3, 4, 8). Thermal traces from data loggers deployed in full shade (e.g., under understory and tree cover) revealed mean temperatures that were ∼2°C lower than temperatures from actual nest sites, while maximum temperatures were ∼2.5°C lower (Figure 6). Third, nest temperatures throughout incubation were reasonably predictable from temperatures during nest site choice; that is, vegetative cover had a negligible effect on openness and radiation intensity, and thus, nest temperatures throughout the incubation period (Figure 5). Fourth, our data revealed considerable variation in canopy openness chosen by nesting mothers (Figure 9). This variation had predictable consequences in incident radiation striking the nest site (Figure 9) and in nest temperatures (Figure 8), and presents the opportunity for natural selection to favor the behavioral choice of nesting in more shaded nest sites under current and future climate warming. Our data suggest that this hot-climate, open-nesting species is likely buffered, to some extent, against potential climate-induced declines caused by increasing nest temperatures because cooler microclimates (shaded potential nest sites) are available to nesting mothers.
Adjusting nest site choice, either within or among generations, is only possible if there is heterogeneity in ground temperatures. Mothers could then assess microhabitat temperatures directly, or indirectly through the choice of some attribute (e.g., canopy cover) that affects those temperatures, or both. Nesting areas at our three study sites provided an abundance of shaded potential nest sites, generally within just a few meters of nest sites. Mean openness of nest sites at the study site with the most nests (BHNP) was 35%, and only 12 of 102 (12%) nests had canopies with openness values < 20% (Figure 9). Those shaded potential nest sites were close to nest sites and easily accessible because nesting softshell turtles are known to move laterally once on land (Fitch and Plummer, 1975; Doody, 1995). Our data revealed that shaded potential nest sites received significantly less solar radiation that, in turn, depressed artificial nest temperatures considerably (mean = ∼2°C, maximum = ∼2.5°C; Figure 6). The relationship between canopy cover and nest temperatures has been demonstrated for turtle nests previously (Ewert et al., 1994; Janzen, 1994; Refsnider et al., 2013a). Although shaded areas tended to have more ground cover due to leaf litter, this would be easily swept aside by nesting females and sandy soils were ubiquitous across the study sites. Indeed, we have observed mothers scratch away dead material when constructing a nest.
Although there was some seasonal increase in grasses and shading understory vegetation throughout incubation (the growing season = April–July), the resultant change in openness and incident radiation intensity data was negligible. Openness and incident radiation intensity of nest sites at all three study sites combined decreased by only 3.8 and 6.4%, respectively (Figure 5). Similar trends were evident when considering openness and incident radiation intensity for each study site (Figure 5). More pronounced seasonal increases in vegetation height and thickness affecting openness and incident radiation intensity may be experienced at other nesting areas for this and other species; seasonal changes in vegetation should thus, be quantified in each study.
Nest sites in the present study exhibited a wide range of openness, and thus incident radiation intensity values (Figure 9). For example, openness ranged from 7–70% (Figure 9). Although we did not follow individual turtles, the large sample size and thermal consequences of nesting in shade would suggest that (decreased) openness could be the target of natural selection in a climate warming scenario.
Collectively, our data suggest that this open-area nester has reasonable potential to use behavioral means to offset current and future climate warming, at least at a magnitude of ∼2–2.5°C. As the climate continues to warm, softshell mothers have the potential to counter the effects of increasing temperatures in nests by choosing to nest in more shaded areas. Most areas inhabited by softshells contain at least patches of shading vegetation, and among-population variation in chosen and available canopy/understory cover should buffer against declines in the species due to climate effects on developing embryos, provided that certain assumptions are met (heritability of nest site choice, rate of evolutionary response relative to rate of climate change). However, as shaded sites were the coolest microhabitats available, continued warming beyond ∼2–2.5°C could exhaust maternal behavioral avenues for keeping softshell eggs at current suitable temperatures. However, determination of the extent of how much nest site choice could buffer developing embryos from climate warming would require quantifying the thermal limits of developing softshell embryos in the laboratory. Without behavioral compensation, softshells would be forced to either shift their distribution toward the poles, or shift their embryological tolerance to rising nest temperatures. Air temperatures have been predicted to warm by 1.4–4.8°C by 2100 without considerable (new) efforts to reduce greenhouse gas emissions (Intergovernmental Panel on Climate Change [IPCC], 2014). If softshell turtle mothers in our populations do not shift nesting into more shaded areas accordingly, then embryos would spend more time at potentially lethal temperatures; moreover, extreme temperatures could cause severe sub-lethal effects in turtles that do hatch. Although mean temperatures of 35 monitored (back-filled) nests did not exceed 32°C, maximum temperatures in 5 of 35 (7%) nests exceeded 36°C, and one nest recorded 39°C. While there are no data on the thermal limits of developing embryos in A. ferox, in the congener A. spinifera constant incubation temperatures of 34°C decreased embryonic survival and reduced performance and endurance in neonates that did hatch (Doody, 1999).
Another possible, climate response option is for softshell mothers to excavate deeper nests. Although recently authors have posited that nest depth is constrained by hindleg length in turtles (Refsnider et al., 2013b), there is evidence that softshell mothers can excavate forms or “troughs” prior to nesting (Doody et al., 2020) that can result in deeper nests (for a discussion on congeners see Plummer and Doody, 2010). By digging a form mothers could uncouple hindleg length from nest depth. We were not able to quantify nest depth in the present study because our nests were depredated; chamber depth would have been compromised during excavation by nest predators. A final way in which turtle mothers could behaviorally offset climate change effects is by adjusting seasonal timing of nesting (Doody et al., 2006a; Doody and Moore, 2010; Nelson et al., 2018). In our study, softshells nested from late March to late July (Figure 1). It is conceivable that mothers could advance or retreat the nesting season into cooler months to achieve cooler nest temperatures as the climate warms. However, shifting seasonal timing of nesting could be difficult due to tradeoffs and/or constraints on other aspects of the species’ biology. For example, seasonal timing of mating or hatching may be adaptive in their own right; shifting those to accommodate shifting timing of nesting could incur survival costs. In another example, the onset of nesting in animals, including turtles, is often underpinned by energy acquisition, which in turn is driven by seasonal climatic conditions (e.g., Rowe, 1994); advancing timing of nesting might thus not be possible due to insufficient energy for vitellogenesis or the production of sex hormones (Kuchling, 1999).
There are two critical missing pieces needed for a fuller understanding of how nesting turtle mothers might respond to climate change to offset increasing temperatures on developing embryos. The first concerns among-generation change vs. phenotypic plasticity. Our conceptual model focuses on among-generation change (adaptation) in nest site choice behavior (see also Janzen and Morjan, 2001), while others have implicated phenotypic plasticity in nesting behavior as a potential response (e.g., Refsnider et al., 2013a). In turn, these mechanisms can only be clarified within the context of the other missing piece, the behavioral mechanism. Do mothers assess ground temperatures directly as suggested by Belzer et al. (2007), or indirectly through canopy cover as posited by others (Janzen, 1994; Morjan and Valenzuela, 2001)? For example, if a mother uses a canopy gap as a surrogate for temperature, that same canopy gap in a future warmer climate will result in hotter developmental (nest) temperatures; continued warming thus, would render canopy cover as a poor predictor of suitable developmental (nest) temperatures. If on the other hand mothers can assess temperature directly, then mothers might use canopy cover as a rough guide to find reasonably thermally-suitable nesting areas, but then fine-tune their choice of nest site by directly assessing substrate temperatures. In this latter case, mothers would not be reliant on a particular canopy cover value or range of values to predict developmental temperatures. More research including experiments is needed to disentangle direct vs. indirect cues for assessing temperature, and to evaluate among-generation change vs. phenotypic plasticity in the potential climate warming responses of turtle mothers.
A potential limitation of our study was that all of our 156 nests were taken by predators (although we did not confirm each event, most of these were by raccoons). We thus assumed that our nests reflected a representative subset of all nests in terms of nest site choice attributes. Although our large sample size and coverage of multiple nesting areas within three study areas might seem to be robust to bias, there could be differences in nest site location, and thus canopy cover, between intact and depredated nests. For example, predation rates of simulated (chicken egg) nests by raccoons were higher in hedge (84%) than in open habitats (45%) (Grosse et al., 2014). However, raccoons, the main softshell nest predator at our study sites, are olfactory-driven predators that can successfully locate nests regardless of microhabitat. There is no published direct evidence that canopy cover influences the probability of predation by raccoons in turtle nests.
How might our results apply to other turtles, reptiles, and other oviparous ectotherms under future warming? Our study species and its two North American relatives A. mutica and A. spinifera nest in open areas (Plummer, 1976; Doody, 1995), providing the opportunity for shifting their nesting into shade in a climate warming scenario. Although rarely quantified, other turtle species nest in semi-shaded areas (e.g., Pseudemys concinna, Jackson and Walker, 1997; P. floridana, J. S. Doody, unpubl. data), a few species nest in full shade (e.g., Kinosternon baurii, Wilson, 1998; K. steindachneri, J. S. Doody, unpubl. data), and at least one wide-ranging species nests in open areas in cooler climates and more shaded areas in warmer climates (e.g., Chelydra serpentina, Ewert et al., 2005; see also Doody et al. (2006a) for an example in lizards). Shade nesters would theoretically be at a disadvantage in a climate warming scenario because they may already be nesting in the shadiest sites (coolest microclimates), especially at the hot end of their range. This behavioral “dead-end” would force a shift in geographic distribution, in the physiological tolerance of developing embryos, or in nest depth, or the population/species could face extirpation or even extinction. Species restricted to hot climates (e.g., the tropics) or with populations at hot climate range margins could also be disadvantaged because the rate of range contraction (extirpations) at the hotter range edge could outpace the rate of range expansion (dispersal) at the cooler range edge. The same should apply to other reptiles and ectotherms. This predicted pattern in the egg life history stage is parallel to the notion that tropical ectotherms (i.e., adults and juveniles) are more vulnerable to climate warming than temperate species because the former need to thermoregulate to keep cool (rather than warm) by using shade, and continued warming could result in heat stress even in deep shade (Deutsch et al., 2008), allowing little or no opportunity for compensation (Kearney et al., 2009).
The raw data supporting the conclusions of this article will be made available by the authors, without undue reservation.
The animal study was reviewed and approved by IACUC.
JSD designed the project with help from GLH. SS collected the data with help from JSD and GLH. DC, SS, and JSD performed the data analysis. JSD and NM accomplished the formatting. JSD wrote the manuscript with help from SS, GLH, NM, and DC. All authors contributed to the article and approved the submitted version.
This project was funded in part by the USF startup fund provided to JSD.
GLH was employed by the company Heinrich Ecological Services.
The remaining authors declare that the research was conducted in the absence of any commercial or financial relationships that could be construed as a potential conflict of interest.
All claims expressed in this article are solely those of the authors and do not necessarily represent those of their affiliated organizations, or those of the publisher, the editors and the reviewers. Any product that may be evaluated in this article, or claim that may be made by its manufacturer, is not guaranteed or endorsed by the publisher.
We would like to thank T. Graham (City of St. Petersburg Parks and Recreation), K. Rogers (Myakka River State Park), S. J. Harper, and P. Leasure (Pinellas County Parks and Conservation Resources) for providing access to study sites, as well as logistical support during the study. T. J. Walsh (Bruce Museum) kindly assisted with locating publications.
Angilletta, M. J. Jr., Sears, M. W., and Pringle, R. M. (2009). Spatial dynamics of nesting behavior: lizards shift microhabitats to construct nests with beneficial thermal properties. Ecology 90, 2933–2939. doi: 10.1890/08-2224.1
Badenhorst, D., Stanyon, R., Engstrom, T., and Valenzuela, N. (2013). A ZZ/ZW microchromosome system in the spiny softshell turtle, Apalone spinifera, reveals an intriguing sex chromosome conservation in Trionychidae. Chromosome Res. 12, 137–147. doi: 10.1007/s10577-013-9343-2
Barnosky, A. D., Matzke, N., Tomiya, S., Wogan, G. O. U., Swartz, B., Quental, T. B., et al. (2011). Has the Earth’s sixth mass extinction already arrived? Nature 471, 51–57. doi: 10.1038/nature09678
Bell, G. (2017). Evolutionary rescue. Annu. Rev. Ecol. Evol. Syst. 48, 605–627. doi: 10.1146/annurev-ecolsys-110316-023011
Belzer, W. R., Belzer, A., and Seibert, S. (2007). Soil temperature and nest site selection by the eastern box turtle, Terrapene carolina carolina. Turt. Tort. Newsl. 10, 10–15.
Bista, B., Wu, Z., Literman, R., and Valenzuela, N. (2021). Thermosensitive sex chromosome dosage compensation in ZZ/ZW softshell turtles, Apalone spinifera. Philos. Trans. R. Soc. B 376:20200101. doi: 10.1098/rstb.2020.0101
Burke, V. J., Rathbun, S. L., Bodie, J. R., and Gibbons, J. W. (1998). Effect of density on predation rate for turtle nests in a complex landscape. Oikos 83, 3–11. doi: 10.2307/3546540
Congdon, J. D., Dunham, A. E., and van Loben Sels, R. C. (1994). Demographics of common snapping turtles (Chelydra serpentina): implications for conservation and management of long-lived organisms. Am. Zool. 34, 397–408. doi: 10.1093/icb/34.3.397
Czaja, R. A., Scholz, A. L., Figueras, M. P., and Burke, R. L. (2020). The role of nest depth and site choice in mitigating the effects of climate change on an oviparous reptile. Diversity 12:151. doi: 10.3390/d12040151
Deutsch, C. A., Tewksbury, J. J., Huey, R. B., Sheldon, K. S., Ghalambor, C. K., Haak, D. C., et al. (2008). Impacts of climate warming on terrestrial ectotherms across latitude. Proc. Natl. Acad. Sci. U.S.A. 105, 6668–6672. doi: 10.1073/pnas.0709472105
Doody, J. S. (1995). A Comparative Nesting Study of Two Syntopic Species of Softshell Turtles (Apalone mutica and Apalone spinifera) in Southcentral Louisiana. Master’s thesis. Hammond, LA: Southeastern Louisiana University.
Doody, J. S. (1999). A test of the comparative influences of constant and fluctuating incubation temperatures on phenotypes of hatchling turtles. Chelonian Conserv. Biol. 3, 529–531.
Doody, J. S. (2009). Superficial lizards in cold climates: nest site choice along an elevational gradient. Austral Ecol. 34, 773–779. doi: 10.1111/j.1442-9993.2009.01983.x
Doody, J. S., Guarino, E., Georges, A., Corey, B., Murray, G., and Ewert, M. (2006a). Nest site choice compensates for climate effects on sex ratios in a lizard with environmental sex determination. Evol. Ecol. 20, 307–330. doi: 10.1007/s10682-006-0003-2
Doody, J. S., Guarino, E., Harlow, P., Corey, B., and Murray, G. (2006b). Quantifying nest site choice in reptiles using hemispherical photography and gap light analysis. Herpetol. Rev. 37, 49–52.
Doody, J. S., and Moore, J. A. (2010). Conceptual model for thermal limits on the distribution of reptiles. Herpetol. Conserv. Biol. 5, 283–289.
Doody, J. S., Sullivan, S., and Donini, J. (2020). Apalone ferox (Florida Softshell Turtle). Nesting behavior. Herpetol. Rev. 51:105.
Ewert, M. A., Jackson, D. R., and Nelson, C. E. (1994). Patterns of temperature-dependent sex determination in turtles. J. Exp. Zool. 270, 3–15. doi: 10.1002/jez.1402700103
Ewert, M. A., Lang, J. W., and Nelson, C. E. (2005). Geographic variation in the pattern of temperature-dependent sex determination in the American snapping turtle (Chelydra serpentina). J. Zool. 265, 81–95. doi: 10.1017/S0952836904006120
Fitch, H. S., and Plummer, M. V. (1975). A preliminary ecological study of the soft-shelled turtle Trionyx muticus in the Kansas River. Isr. J. Zool. 24, 28–42.
Frazer, G. W., Canham, C. D., and Lertzman, K. P. (1999). Gap Light Analyzer (GLA), Version 2.0: Imaging Software to Extract Canopy Structure and Gap Light Transmission Indices from True-Colour Fisheye Photographs, Users Manual and Program Documentation. Burnaby: Simon Fraser University.
Grosse, A. M., Crawford, B. A., Maerz, J. C., Buhlmann, K. A., Norton, T., Kaylor, M., et al. (2014). Effects of vegetation structure and artificial nesting habitats on hatchling sex determination and nest survival of diamondback terrapins. J. Fish Wildl. Manag. 6, 19–28. doi: 10.3996/082014-jfwm-063
Heinrich, G., and Richardson, D. E. (1993). Apalone ferox (Florida Softshell): reproduction. Herpetol. Rev. 24:31.
Huey, R. B., Hertz, P. E., and Sinervo, B. (2003). Behavioral drive versus behavioral inertia in evolution: a null model approach. Am. Nat. 161, 357–366. doi: 10.1086/346135
Huey, R. B., Kearney, M. R., Krockenberger, A., Holtum, J. A. M., Jess, M., and Williams, S. E. (2012). Predicting organismal vulnerability to climate warming: roles of behaviour, physiology and adaptation. Philos. Trans. R. Soc. B Biol. Sci. 367, 1665–1679. doi: 10.1098/rstb.2012.0005
Intergovernmental Panel on Climate Change [IPCC] (2007). Climate Change 2007: Synthesis Report. Contribution of Working Groups I, II and III to the Fourth Assessment Report of the Intergovernmental Panel on Climate Change, eds Core Writing Team, R. K. Pachauri, and A. Reisinger (Geneva: IPCC).
Intergovernmental Panel on Climate Change [IPCC] (2014). Climate Change 2014: Synthesis Report. Contribution of Working Groups I, II and III to the Fifth Assessment Report of the Intergovernmental Panel on Climate Change, eds Core Writing Team, R. K. Pachauri, and L. A. Meyer (Geneva: IPCC).
Intergovernmental Panel on Climate Change [IPCC] (2019). Climate Change and Land: an IPCC Special Report on Climate Change, Desertification, Land Degradation, Sustainable Land Management, Food Security, and Greenhouse Gas Fluxes in Terrestrial Ecosystems, eds P. R. Shukla, J. Skea, E. Calvo Buendia, V. Masson-Delmotte, H.-O. Pörtner, D. C. Roberts, et al. (Geneva: IPCC).
Iverson, J. B., and Moler, P. E. (1997). The female reproductive cycle of the Florida softshell turtle (Apalone ferox). J. Herpetol. 31, 399–409. doi: 10.2307/1565669
Jackson, D. R., and Walker, R. N. (1997). Reproduction in the Suwannee cooter, Pseudemys concinna suwanniensis. Bull. Fla. Mus. Nat. Hist. 41, 69–167.
Janzen, F. J. (1994). Climate change and temperature-dependent sex determination in reptiles. Proc. Natl. Acad. Sci. U.S.A. 91, 7487–7490. doi: 10.1073/pnas.91.16.7487
Janzen, F. J., and Morjan, C. L. (2001). Repeatability of microenvironment-specific nesting behaviour in a turtle with environmental sex determination. Anim. Behav. 62, 73–82.
Kearney, M., Shine, R., and Porter, W. P. (2009). The potential for behavioral thermoregulation to buffer “cold-blooded” animals against climate warming. Proc. Natl. Acad. Sci. U.S.A. 106, 3835–3840. doi: 10.1073/pnas.0808913106
Krysko, K. L., Enge, K. M., and Moler, P. E. (2019). Amphibians and Reptiles of Florida. Gainesville, FL: University of Florida Press.
Massey, M. D., Congdon, J. D., Davy, C., and Rollinson, N. (2019). First evidence of metabolic heating in a freshwater turtle (Chelydra serpentina). Chelonian Conserv. Biol. 18, 145–152. doi: 10.2744/CCB-1356.1
Meylan, P. A., and Moler, P. E. (2006). “Apalone ferox – Florida softshell turtle,” in Biology and Conservation of Florida Turtles, Vol. 3, ed. P. A. Meylan (Lunenberg, MA: Chelonian Research Foundation), 160–168.
Morjan, C. L., and Valenzuela, N. (2001). Is ground-nuzzling by female turtles associated with soil surface temperatures? J. Herpetol. 35, 668–672. doi: 10.2307/1565908
Nelson, N. J., Keall, S. N., Refsnider, J. M., and Carter, A. L. (2018). Behavioral variation in nesting phenology may offset sex-ratio bias in tuatara. J. Exp. Zool. A Ecol. Integr. Physiol. 329, 373–381. doi: 10.1002/jez.2196
Pacifici, M., Visconti, P., Butchart, S. H. M., Watson, J. E. M., Cassola, F. M., and Rondinini, C. (2017). Species’ traits influenced their response to recent climate change. Nat. Clim. Change 7, 205–208. doi: 10.1038/NCLIMATE3223
Parmesan, C. (2006). Ecological and evolutionary responses to recent climate change. Annu. Rev. Ecol. Evol. Syst. 37, 637–669. doi: 10.1146/annurev.ecolsys.37.091305.110100
Plummer, M. V. (1976). Some aspects of nesting success in the turtle, Trionyx muticus. Herpetologica 32, 353–359.
Plummer, M. V., and Doody, J. S. (2010). Terrestrial burrowing in nesting softshell turtles (Apalone mutica and A. spinifera). Reptiles Amphib. 17, 79–81.
R Core Team (2018). R: A Language and Environment for Statistical Computing. Vienna: R Foundation for Statistical Computing.
Radchuk, V., Reed, T., Teplitsky, C., van de Pol, M., Charmantier, A., Hassall, C., et al. (2019). Adaptive responses of animals to climate change are most likely insufficient. Nat. Commun. 10:3109. doi: 10.1038/s41467-019-10924-4
Refsnider, J. M., Bodensteiner, B. L., Reneker, J. L., and Janzen, F. J. (2013a). Nest depth may not compensate for sex ratio skews caused by climate change in turtles. Anim. Conserv. 16, 481–490. doi: 10.1111/acv.12034
Refsnider, J. M., Warner, D. A., and Janzen, F. J. (2013b). Does shade cover availability limit nest-site choice in two populations of a turtle with temperature-dependent sex determination? J. Therm. Biol. 38, 152–158. doi: 10.1016/j.jtherbio.2013.01.003
Rowe, J. W. (1994). Reproductive variation and the egg size-clutch size trade-off within and among populations of painted turtles (Chrysemys picta bellii). Oecologia 99, 35–44. doi: 10.1007/BF00317081
Shine, R. (1988). The evolution of large body size in females: a critique of Darwin’s “fecundity advantage” model. Am. Nat. 131, 124–131. doi: 10.1086/284778
Tomás, J., Godley, B. J., Castroviejo, J., and Raga, J. A. (2010). Bioko: critically important nesting habitat for sea turtles of West Africa. Biodivers. Conserv. 19, 2699–2714. doi: 10.1007/s10531-010-9868-z
Urban, M. C. (2015). Accelerating extinction risk from climate change. Science 348, 571–573. doi: 10.1126/science.aaa4984
Valenzuela, N., and Lance, V. A. (eds) (2004). Temperature-Dependent Sex Determination in Vertebrates. Washington, DC: Smithsonian Books.
Vogt, R. C., and Bull, J. J. (1984). Ecology of hatchling sex ratio in map turtles. Ecology 65, 582–587.
Keywords: climate change, behavior, evolution, nesting, softshell turtle
Citation: Sullivan S, Heinrich GL, Mattheus NM, Cassill D and Doody JS (2022) Can Reptiles Use Nest Site Choice Behavior to Counter Global Warming Effects on Developing Embryos? Potential Climate Responses in a Turtle. Front. Ecol. Evol. 10:825110. doi: 10.3389/fevo.2022.825110
Received: 30 November 2021; Accepted: 07 February 2022;
Published: 15 March 2022.
Edited by:
Hope Klug, University of Tennessee at Chattanooga, United StatesReviewed by:
Blair Bentley, University of Massachusetts Amherst, United StatesCopyright © 2022 Sullivan, Heinrich, Mattheus, Cassill and Doody. This is an open-access article distributed under the terms of the Creative Commons Attribution License (CC BY). The use, distribution or reproduction in other forums is permitted, provided the original author(s) and the copyright owner(s) are credited and that the original publication in this journal is cited, in accordance with accepted academic practice. No use, distribution or reproduction is permitted which does not comply with these terms.
*Correspondence: J. Sean Doody, anNlYW5kb29keUBnbWFpbC5jb20=
Disclaimer: All claims expressed in this article are solely those of the authors and do not necessarily represent those of their affiliated organizations, or those of the publisher, the editors and the reviewers. Any product that may be evaluated in this article or claim that may be made by its manufacturer is not guaranteed or endorsed by the publisher.
Research integrity at Frontiers
Learn more about the work of our research integrity team to safeguard the quality of each article we publish.