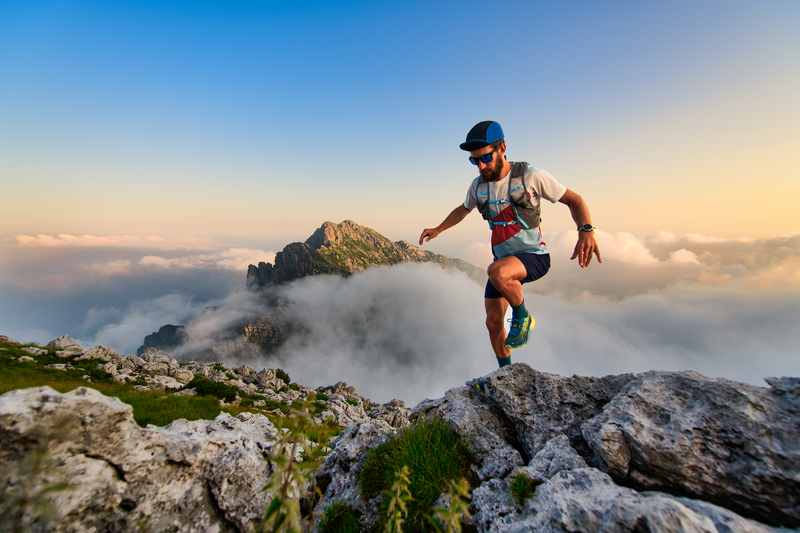
95% of researchers rate our articles as excellent or good
Learn more about the work of our research integrity team to safeguard the quality of each article we publish.
Find out more
ORIGINAL RESEARCH article
Front. Ecol. Evol. , 11 February 2022
Sec. Behavioral and Evolutionary Ecology
Volume 10 - 2022 | https://doi.org/10.3389/fevo.2022.819393
This article is part of the Research Topic Recent Advances in the Ecology and Evolution of the Bathyergidae View all 15 articles
Differences in individual locomotor activity patterns may be linked to a number of ecological factors, such as changes in ambient temperature or photoperiod. Observations on subterranean mammals suggest that they exhibit diel rhythms despite the lack of visual cues in their underground burrows, but it is unknown how seasonality and individual characteristics affect their activity. In this study we use RFID technology to monitor daily activity patterns of wild, social Natal mole-rats (Cryptomys hottentotus natalensis) during the summer and winter to investigate how their activity varies with season and whether their activity depends on individual characteristics such as body mass, sex and reproductive status. We found that in winter, individuals were more active during the time with the highest soil temperatures, whereas in summer, they showed a bimodal activity pattern during early morning and late afternoon coinciding with cooler soil temperatures. Individual characteristics, including reproductive status, did not affect general activity indicating that reproductive and non-reproductive individuals contribute equally to cooperative behaviors. We suggest that the activity patterns may be a behavioral adaptation to avoid extreme burrow temperatures and a mechanism to maintain a stable core body temperature. We highlight the advantages of RFID technology to study wild small mammal movements.
Animal locomotor activity patterns have been the subject of much interest in the study of animal behavior. These activity patterns may be influenced by endogenous (i.e., intrinsic) or exogenous (i.e., environmental) factors, which may or may not persist under constant conditions (Hazlerigg and Tyler, 2019). When a biological rhythm persists in a near-24-h period under constant conditions (e.g., complete darkness), the circadian rhythm is assumed to “free run,” showing evidence for control by a biological clock (Benstaali et al., 2001). Conversely, the lack of an endogenous clock may result in the degradation of rhythmic patterns under constant conditions (Hazlerigg and Tyler, 2019). Biological clocks conform to daily and predictable exogenous cues known as zeitgebers in a process referred to as entrainment in which the clock’s rhythm becomes equal to the cycle of the zeitgeber (Johnson et al., 2003).
It is widely accepted that the light-dark cycle (i.e., rising and setting of the sun) is the most common zeitgeber for the entrainment of circadian rhythms (Benstaali et al., 2001). Ambient temperature may also affect circadian rhythms in both homeothermic (e.g., most mammals) and heterothermic (e.g., birds, squamates, some mammals) vertebrates (Rensing and Ruoff, 2002; Refinetti, 2015). Some mammals dwelling in arid or arctic regions exhibit adaptive heterothermy, where the core body temperature may fluctuate with ambient temperature under certain conditions (Cain et al., 2006; Williams et al., 2012; Allali et al., 2013; Farsi et al., 2020b). For example, camels (Camelus dromedarius) exhibit heterothermy when dehydrated (Allali et al., 2013) and arctic ground squirrels (Urocitellus parryii) during hibernation (Williams et al., 2012). Recently it has been discovered that ambient temperature instead of the light-dark cycle may entrain circadian rhythms in body temperature, melatonin cycles and locomotor activity in some of these species (Allali et al., 2013; Farsi et al., 2020a,b). While ambient temperature may not be as strong a zeitgeber compared to light-dark cycle in homeothermic mammals, many will adjust their activity patterns in response to ambient temperature to avoid exposure in harsh environments (Cain et al., 2006; Roll et al., 2006, however see Farsi et al., 2020b). For example, arid dwelling rodents avoid the hottest period of the day and by becoming nocturnal (Randall, 1993; Roll et al., 2006). Lastly, biotic cues, such as feeding time, food availability, social cues, or predation, may also affect activity patterns (Mistlberger and Skene, 2004; Halle, 2006; Getz, 2009; Refinetti, 2015; Beale et al., 2016). Individuals in a population may synchronize their activity patterns via social cues to be active nearly simultaneously even in complete darkness (e.g., under snowfall, Korslund, 2006), and isolated individuals may exhibit asynchronous activity patterns (Getz, 2009; Lövy et al., 2013). It has been suggested that simultaneous activity in voles provides an individual with protection against predation due to “safety in numbers” or possibly confusing predators by a “swarming” effect (Gerkema and Daan, 1985; Inman and Krebs, 1987) and species may alter their activity patterns in response to predation pressure which may act as a weak zeitgeber (Getz, 2009; Vlasatá et al., 2017).
In the subterranean ecotope, and other photic void environments, occupants are rarely, if ever, exposed to light. Despite limited exposure to light, many subterranean mammals kept in constant darkness and temperature whilst under laboratory conditions still exhibit strong circadian rhythms of locomotor and physiological activity (e.g., melatonin and body temperature cycles). A large body of work has focused on physiological circadian rhythms and entrainment to photoperiod in many species including African mole-rats (Family Bathyergidae: Riccio and Goldman, 2000; Oosthuizen et al., 2003; van Jaarsveld et al., 2019; Hart et al., 2021), blind mole rats (Family Spalacidae: Rado et al., 1993), coruros (Family Octodonidae: Begall et al., 2002), and tuco-tucos (Family Ctenomyidae: Jannetti et al., 2019; Flôres et al., 2021). However, the majority of these experiments lack the complex environmental conditions that these animals may experience in the wild, including changes in infrequent light pulses, atmospheric conditions, burrow microclimate, social cues, or predator-prey interactions. Therefore, other zeitgebers may be involved in entraining circadian rhythms in these animals (reviewed in Beale et al., 2016). Observations of free-ranging subterranean mammals have revealed that some species may exhibit arrhythmic activity patterns over the 24-h period, such as some pocket gophers (Thomomys; Andersen and MacMahon, 1981; Gettinger, 1984), yet many other species exhibit distinct daily peaks of activity, including root rats (Tachyoryctes: Jarvis, 1973), African mole-rats (Heterocephalus: Jarvis, 1973; Heliophobius: Šklíba et al., 2007; Fukomys: Šklíba et al., 2014, 2016a), blind mole rats (Spalax: Rado et al., 1993; Šklíba et al., 2016b), coruros (Spalacopus: Rezende et al., 2003), and tuco-tucos (Ctenomys: Jannetti et al., 2019; Flôres et al., 2021). These activity patterns suggest entrainment of a circadian clock, which has led some authors to conclude that temperature may be an essential zeitgeber for the entrainment of activity rhythms in subterranean mammals (Benedix, 1994; Šklíba et al., 2014; Hart et al., 2021). Homeothermic regulation of body temperature suggests that homeothermic animals are less sensitive to environmental changes (Rensing and Ruoff, 2002), but some African mole-rats exhibit heterothermy (Buffenstein and Yahav, 1991; Bennett et al., 1993a; Oosthuizen et al., 2021) and therefore, fluctuations in ambient temperature are more likely to affect their activity patterns.
African mole-rats are a unique clade of subterranean rodents, with some species being strictly solitary and others occurring in large family groups (Bennett and Faulkes, 2000). Living underground offers thermal buffering to extreme temperature fluctuations with burrow depth varying with species (Bennett et al., 1988). Soil properties and food availability likely explain why tunnels are constructed at specific depths (Broekman et al., 2006; Lövy et al., 2015). The depth of tunnels and the nests are greatest for mole-rats inhabiting arid regions with sandy soils, where foraging tunnels are between 30 and 40 cm below ground, and nests may exceed 2 m in depth (Lovegrove and Painting, 1987; Bennett et al., 1988). In other soil types foraging tunnels are at more shallow depths of around 10–20 cm with nests between 30 and 60 cm (Hickman, 1979; Spinks et al., 1999; Šklíba et al., 2012; Šumbera et al., 2012). Deep dead-end tunnels may help regulate burrow temperatures or humidity, and these features vary between 78 cm in loamy soil types of the Natal region in South Africa (Hickman, 1979) to over 3 m in the sand of the Kalahari (Lovegrove and Painting, 1987). The deeper tunnels of the Damaraland mole-rat (Fukomys damarensis) provide a greater thermal buffer against the extreme daily and seasonal temperatures of the Kalahari compared to the shallow tunnels of common mole-rats (Cryptomys hottentotus), which generally occur in more mesic habitats, such as the Natal region in South Africa (Bennett et al., 1988). Even with deep tunnels, the foraging burrows of mole-rats experience seasonal temperature fluctuations, and it has been suggested that temperature may act as a zeitgeber for daily activity patterns in mole-rats (Goldman et al., 1997; Šklíba et al., 2007; Hart et al., 2021). The activity patterns and circadian rhythms of mole-rats have been well studied in laboratory settings (Hickman, 1980; Bennett, 1992; Riccio and Goldman, 2000; Oosthuizen et al., 2003; van Jaarsveld et al., 2019; Hart et al., 2021). In two recent studies under laboratory conditions, homeothermic mole-rat species showed decreased activity during periods of higher temperature and the authors suggested that temperature may drive nocturnal behavior (van Jaarsveld et al., 2019; Hart et al., 2021). The observed activity entrainment by ambient temperature may be due to a thermoregulatory response to avoid over-heating while digging during periods of increased burrow temperatures (Hart et al., 2021). Few studies have been conducted on wild mole-rats (Šklíba et al., 2007, 2014, 2015) and even fewer still on seasonal variation in activity (Šklíba et al., 2007).
This study set out to determine if daily activity patterns fluctuated with seasonal temperature changes in the Natal mole-rat (Cryptomys hottentotus natalensis), a social species inhabiting mesic grasslands of southeastern South Africa and Lesotho at altitudes from sea level to the Drakensberg escarpment (ca. 3,000 m). The montane regions they inhabit experience hot summer days and cold winter nights, with snow often accumulating at the highest elevations during winter. Unlike most other bathyergids, Natal mole-rats exhibit heterothermy (Oosthuizen et al., 2021), and they would be expected to respond to changes in burrow temperature to a greater degree in comparison to their homeothermic counterparts (Šumbera, 2019; Wallace et al., 2021). The link between ambient temperature and activity patterns in African mole-rats has not been thoroughly investigated in a field-based study, and never in a heterothermic mole-rat species. Previous laboratory experiments on entrainment of circadian rhythms in Natal mole-rats indicate the entrainment in both melatonin and activity cycles under a light-dark cycle and free-running cycles under constant conditions (Hart et al., 2004). In this study, we used a radio-frequency identification (RFID) reader array to monitor individual activity in free-ranging Natal mole-rats. This non-invasive method allows for automated continuous monitoring of activity patterns in a natural habitat without disturbing the subjects (Korslund, 2006; Francioli et al., 2020). Activity rhythms may show plasticity, where individuals or populations adjust to seasonal changes in the environment (Halle, 1995; Rezende et al., 2003; Halle, 2006; Beale et al., 2016). Therefore, it was predicted that Natal mole-rats would alter their activity patterns seasonally to cope with the fluctuating burrow temperatures due to their shallow tunnels (Hickman, 1979). Additionally, trapping efforts indicated that mole-rats appeared to exhibit reduced activity during periods of rain when compared to clear days. It is possible that mole-rats may be able to respond to the change in air pressure or temperature caused by the rain or cold fronts as seen in other animals (Garthe et al., 2009; Boyer and MacDougall-Shackleton, 2020). Lastly, because Natal mole-rats exhibit only behavioral reproductive suppression compared to that of the Damaraland mole-rats, which exhibit behavioral and physiological suppression (Bennett et al., 1996; Molteno and Bennett, 2000; Oosthuizen et al., 2008), we predicted that locomotor activity differences between the breeders and non-reproductive individuals would be less distinct than in species with higher reproductive skew. We emphasize that in the current study we did not investigate entrainment of circadian rhythms by environmental zeitgebers, but rather investigated the potential role of environmental variables on the diel activity rhythms of colonies of a subterranean mammal during the summer and winter months of the Southern Hemisphere.
Natal mole-rats were captured using Hickman live traps during the wet summer (February to March) and dry winter (July to October) seasons from August 2019 to July 2021 at Glengarry Holiday Farm in KwaZulu-Natal, South Africa (–29.322530°, 29.712982°, 1,600 m a. s. l.; Supplementary Figure 1). Natal mole-rats are a social species of mole-rat that live in family groups (referred to as colonies) with one reproductive female, a couple of reproductive males, and the remaining non-reproductive group members include the overlapping generations of offspring from the reproductive individuals (Oosthuizen et al., 2021). A total of 13 distinct colonies (including 118 unique animals) were used for the study, with a mean colony size of 8.8 ± 3.2 (range 4–13); 10 of the 13 groups were complete colonies (i.e., included reproductive individuals and all non-reproductive subordinates). Traps were placed in exposed tunnels at each colony, baited with sweet potato, and checked at 2-h intervals from 07 h00 to 22 h00, depending on air temperature. Colonies were recaptured at 6-month intervals. Colony membership of individuals was initially determined by capture location, a lack of aggression between individuals, and later confirmed by recapture of individuals in the same colony (as opposed to capture in neighboring colonies) during subsequent captures. Capture methods have been standardized in many field studies of bathyergids (Jarvis and Bennett, 1993; Šklíba et al., 2007, 2012, 2016a; Lövy et al., 2013; Finn et al., 2018). Captured mole-rats were housed with family members at ambient temperature (15–25°C), provided with wood shavings as bedding and fed sweet potato ad libitum. All animals were sexed, weighed to the nearest gram (Pelouze SP5, Rubbermaid, United States), and assigned a reproductive status at capture. Reproductive females were identified by a perforate vagina and prominent teats (Bennett and Faulkes, 2000). Male reproductive status was determined by body mass, presence of testes, and prolonged presence in the colony (Finn et al., 2018). All individuals greater > 20 g were implanted with a subcutaneous passive integrated transponder tag (Trovan Unique, DorsetID, Netherlands) to uniquely identify them. After 48 h without any signs of individuals present in the burrow system (i.e., no blocked tunnels, fresh mounds, triggered traps, or bait eaten), the colony was assumed to be completely captured and the colony was released back into their burrow.
Hourly air pressure, air temperature, soil temperature, and rainfall during winter 2020 and summer 2021 were obtained for the study site from the global atmospheric reanalysis dataset ERA5-Land (Table 1; Muñoz-Sabater, 2019). The ERA5-Land dataset is a global climate analysis model based on satellite and weather station climate observations allowing for accurate estimates of climate variables at specific locations with a 9 km accuracy (0.1°× 0.1°; Muñoz-Sabater et al., 2021). Users can specify the boundary GPS points of less than 9 km to query data from the database, and we selected a 1 km radius to encompass the study site. The dataset is freely available from the Copernicus Climate Change Service, and holds the intellectual property rights of the raw data. The results contain modified Copernicus Climate Change Service information. We used soil temperature measured at depths of 0–7 cm (TS1), 7–28 cm (TS2), and 28–100 cm (TS3), which correspond to the depths of foraging tunnels (TS1 and TS2) and the nest (TS3). A temperature logger (DS1922L iButton, Maxim Integrated Products, Dallas, TX, United States) was buried at the study site at 15 cm below the soil surface in an area of direct sunlight and set to record hourly temperatures from August to October 2020. We compared the soil temperatures from the data logger and TS2 from the ERA5-Land dataset. We found that soil temperatures from the data logger were on average 3.67 ± 1.32°C higher than TS2, yet the daily fluctuation in temperature was similar between temperature loggers and ERA5-Land values (Supplementary Figure 2). Therefore, we assumed that soil temperatures from ERA5-Land were a modest representation of actual variability of soil temperatures. It is important to note that the soil temperatures in this study may not accurately represent burrow temperatures, and furthermore, that ambient temperature in tunnels may be higher than the surrounding soil (sensu Holtze et al., 2018). The change in hourly air pressure (ΔP) from 1 to 2 h was calculated to determine whether changes in air pressure predicted activity. To determine if climate varied between hour and season the climatic variables were compared by conducting a linear regression with the climate variable as response and season, hour and interaction between season and hour as predictors. Hourly climate variables were then combined with the activity data to determine the environmental effects of daily and seasonal activity patterns.
We utilized an RFID reader array (LID650/608, DorsetID, Netherlands; Supplementary Figure 2) and the methods of Francioli et al. (2020) to monitor mole-rat activity during winter (August–October 2020) and summer (February–April 2021). The array consisted of a scanning panel (Trovan ANT 612) measuring 47.5 × 40 × 4 cm and a decoder box (Supplementary Figure 3). The array was powered by a 12 v battery and could be attached to a solar panel to prolong battery life (Supplementary Figure 4). The array was programmed to scan continuously for transponder tags within the range with a read delay of 3 s. When an animal with a transponder tag passed within range, the numeric code of the tag was recorded with a time stamp on a USB drive attached to the decoder. Thus, if an animal remained in the range of the panel for longer than 3 s, it would have multiple entries at 3-s intervals.
The array was placed at a mole-rat group and left stationary during each reading session. Mole-rat mounds were probed until the tunnel was located and the reader panel was placed directly over the tunnel. The panel had a detection range of 20–30 cm, which is greater than the depth of mole-rat tunnels at the study site, and therefore the chance of animals passing undetected under the panel is minimal. Reading sessions had a mean duration 3.5 ± 1.59 days (range 2–7). We attempted to repeat reading sessions for each group in both seasons; however, some groups went missing and additional groups were selected to maintain an even sample size. Therefore, the mean number of reading sessions per group was 1.3 ± 0.46.
Radio telemetry studies have indicated that activity bouts of mole-rats can last for up to 1 h (Lovegrove, 1988), and therefore we assumed that activity bouts lasted for 1 h (see Supplementary Material for validation). Due to the nature of the study design, we do not know the exact duration of activity bouts, and the results rather include the times and frequency of individual animal activity. Animals were given an active score of 1 if at least one reading was detected on the array during a 60-min interval, and a score of 0 if not detected during this interval. An animal would not gain a further activity point until detected beyond the activity period. Visual inspection of the raw data indicated that animals rarely remained motionless under the reader array. Individuals captured in the colony but not recorded by the RFID reader array were assigned activity scores of zero. All potential variables affecting daily activity were analyzed by fitting a generalized linear mixed model with negative binomial distribution using the glmmTMB package (Brooks et al., 2017) in R 4.0.5 (R Core Team, 2021). The negative binomial distribution (values of 0 or 1 in the response) prevents overdispersion in parameters. Predictors for the full model included life history variables such as sex, body mass, reproductive status (reproductive/non-reproductive), group size; abiotic factors such as Tair, TS1, TS2, TS3, ΔP, rainfall, season (wet/dry), time (as sine and cosine wave expressed in radians—where 0 is 00:01, π is 12:00, and 2π is midnight), and the daily cumulative hours of sunlight from sunrise each hour until sunset (with hours of darkness equal to 0). Non-significant predictor variables were dropped until a model with the lowest AIC was generated (Supplementary Material). Predictors for the final model included: interactions between sex and reproductive status; sex and body mass; season and time; season, time, and Tair; season, time, and TS1; and season, time, and TS2. Individual identity and reading session were specified as nested random intercepts to control for non-independence among observations. All continuous predictors were scaled, and body mass was scaled within sex because males were heavier than females. The proportion of explained variance was estimated using marginal and conditional R2. An excess mass test for unimodality using the package multimode (Ameijeiras-Alonso et al., 2019, 2021) was used to determine if the peaks of activity were unimodal or bimodal by comparing the hours of activity within each season. This package allows for testing the kernel density of data against the null hypothesis that the number of modes is equal to a set number, and can calculate the exact number of modes in a density plot. The calibrated excess mass test by Ameijeiras-Alonso et al. (2019) is more accurate than previous modality tests, such as Silverman’s and Hartigan’s methods among others, by allowing the user to set the number of modes to test against the null.
All hourly climate variables except rainfall varied significantly between seasons, and all variables except TS3 varied significantly with time of day within each season (Tables 2, 3 and Supplementary Figures 6, 7). The greatest daily difference in soil temperatures occurred during winter. The maximum day length was 13.28 h during summer and 12.75 h during winter, with a minimum day length of 11.68 and 11.00 h, respectively.
The dataset included 9,972 raw activity points at 3-s intervals from 77 wild mole-rats (41 individuals were captured before array placement, but not recorded) over 16 reader sessions at independent groups (winter n = 8, summer n = 8). Assuming an activity period of 1 h, the dataset was reduced to 365 total activity points of detected mole-rats (summer n = 153, winter n = 212) with individuals being detected a mean of 0.89 ± 1.27 times per day during winter (range 0–7) and 0.88 + 1.02 times per day during summer (range 0–4). The results from the model revealed that body mass, Tair, TS1 and interactions between those temperatures with time of day and season had the greatest effect on predicting periods of activity (Table 4). Hours of daylight, rainfall, TS2, Ts3, and ΔP as well as life-history traits (sex, reproductive status, and group size) did not affect the likelihood of activity. Mole-rats were most active when soil temperatures were between 12 and 16°C. Locomotor activity patterns were similar for reproductive and non-reproductive individuals in the colony suggesting no specific specialization of activity based on reproductive status. The total amount of activity did not differ between seasons (i.e., season variable), but the time of day during which individuals were active was significantly different between seasons (i.e., season * time variable). During winter, animals exhibited a single peak in activity during midday when burrow temperatures were highest (E = 0.02, p-value = 0.92; Figure 1). While during summer, daily activity exhibited a bimodal distribution where animals were more active during the cooler morning and evening temperatures (E = 0.10, p-value < 0.001; Figure 2). Further linear regression analysis of the relationship between activity and Tair as well as TS1 indicated a significant relationship in both seasons for TS1 (winter: df = 7,455, SE 0.0014, p-value < 0.01; summer: df = 4,616, SE = 0.0024, p-value < 0.001), while the relationship between activity and Tair was only significant in summer (winter: df = 7,455, SE = 0.0012, p-value = 0.17; summer: df = 4,616, SE = 0.0021, p-value < 0.001).
Figure 1. Histogram of daily activity patterns of mole-rats during winter (August–October). Gray bars indicate the total activity points per hour of all animals across the sampled groups during winter. Mean daily air temperature (red) and soil temperature at 0–7 cm (blue) during winter is plotted on the right axis. The median time mole-rats were active is highlighted in purple. Hours of darkness are indicated by the shaded blocks. Climate data were obtained from the Copernicus Climate Change Service, which holds the intellectual property rights of the raw data.
Figure 2. Histogram of daily activity patterns of mole-rats during summer (February–April). Gray bars indicate the total activity points per hour of all animals across the sampled groups during summer. Mean daily air temperature (red) and soil temperatures at 0–7 cm (blue) during summer is plotted on the right axis. The median time mole-rats were active is highlighted in purple. Hours of darkness are indicated by the shaded blocks. Climate data were obtained from the Copernicus Climate Change Service, which holds the intellectual property rights of the raw data.
In this study, soil temperatures at < 7 cm and time of day were the best predictors of activity patterns in the heterothermic Natal mole-rat, with soil temperature varying significantly over the course of a day and between seasons. During summer individuals avoided activity during the hottest part of the day (becoming more crepuscular), while in winter they were more active during this time (becoming diurnal). These patterns are similar to responses observed in other rodents (both heterothermic and homeothermic) living in high altitudes or latitudes (Hinze and Pillay, 2006; Williams et al., 2012; Vlasatá et al., 2017; Flôres et al., 2021). This result also confirms recent work on activity patterns in mole-rats observed in a captive setting (Hart et al., 2021). Oosthuizen et al. (2021) observed that wild Natal mole-rats at the same study site exhibited unimodal body temperature fluctuations in winter and bimodal fluctuations in summer and proposed that increased body temperature coincided with time of activity. Our results may validate this prediction because in summer, Natal mole-rats showed bimodal peaks in activity during the cooler soil temperatures and a unimodal peak in activity during winter when soil temperatures were highest. Oosthuizen et al. (2021) found that mean body temperature was higher during winter than during summer, and this result may have indicated increased activity during winter. In contrast to that suggestion and previous studies on a related mole-rat species (Šklíba et al., 2007), we did not find a significant difference in the frequency of daily activity between seasons.
Previous work on bathyergids, including the Natal mole-rat, has shown that circadian rhythms free-run under constant conditions and both light and temperature may entrain circadian rhythms (Hart et al., 2004, 2021; van Jaarsveld et al., 2019). In laboratory studies, mole rats preferred temperatures between 18 and 22°C while in complete darkness (Hart et al., 2021). Interestingly, we found that even though TS1 was consistently below this range during winter (5–17°C, Supplementary Figure 4) and rarely greater than 20°C during summer (12–21°C, Supplementary Figure 5), mole-rats did not show an even distribution of activity periods over a 24-h period during either of the two seasons. However, we must note that soil temperatures from the ERA5-Land dataset were (1) 3°C lower than temperatures recorded via temperature loggers at the study site, and (2) may not reflect actual burrow temperatures. Minute temperature changes may be enough to elicit a change in activity rhythms in subterranean rodents (Goldman et al., 1997; Šklíba et al., 2007, this study). Temperature changes in foraging tunnels may well be a primary driving force in the entrainment of circadian rhythms since bimodal and unimodal activity patterns were found in various subterranean mammals (this study, Benedix, 1994; Rezende et al., 2003; Šklíba et al., 2007). Mole-rats spend over 70% of their time in the nest (Bennett, 1990), which may be at the deepest part of their burrow system where the microclimate is stable (Bennett et al., 1988). Animals resting inside a nest at a stable temperature may be unable to detect temperature changes to trigger an activity bout (Oster et al., 2002; Šklíba et al., 2014). Temperatures in the nest may be higher than the surrounding burrow system due to increased heat generated through huddling and body heat of animals confined in a small space (Buffenstein and Yahav, 1991; Kotze et al., 2008). Hazlerigg and Tyler (2019) suggested that the better a retreat insulates an animal against environmental extremes, the less information the animal may obtain about external conditions and when to emerge. However, the relatively shallow nests of the Natal mole-rat may experience greater temperature fluctuations despite the thermal benefits of huddling (Marhold and Nagel, 1995). Exact thermal conditions inside mole-rat nests are still unknown and would be of interest to understand thermoregulation and activity patterns.
Seasonal changes in environmental characteristics may also affect locomotor activity such as the availability of food, and specifically in the subterranean ecotope, changes in humidity and gas composition in the microhabitat of the burrows. Decreased food availability during the warmer late dry season caused a related decrease in outside the nest forays in the Ethiopian giant root rat (Tachyoryctes macrocephalus) which feeds on aboveground grasses and forbs (Vlasatá et al., 2017). Vlasatá et al. (2017) concluded that food caching and availability may be of minimal importance compared to thermoregulatory demands. The seasonal differences in activity observed in this study are unlikely to be affected by seasonal food availability since mole-rats rely upon the underground storage organs of plants (tubers and corms, collectively referred to as geophytes) for their nutritional needs (Bennett and Faulkes, 2000). These geophytes are available year-round, and bathyergids famously cache food, which root rats tend not to do, and caching is presumed to provide the group with resources through droughts and periods of decreased availability (Bennett and Faulkes, 2000). Seasonal changes in carbon dioxide and oxygen concentrations could potentially affect locomotor activity. The seasonal fluctuation of burrow gasses of bathyergids is understudied and completely unknown at the study site. Levels of carbon dioxide may increase during periods of rain due to increased soil moisture and decreased gas permeability, which may in turn trigger increased digging activity (Burda et al., 2007). While our study did not find an effect of rainfall on locomotor activity, our methods prevented an assessment of digging behavior. A study on seasonal changes in gas composition in the burrows of the giant root rat that lives at a similar elevation, climate, and annual rainfall to our study site found minimal fluctuations in carbon dioxide and oxygen levels between the seasons (Šumbera et al., 2020). Furthermore, humidity was not found to change between seasons in the burrows of the silvery mole-rat (Heliophobius argenteocinereus) in Malawi (Šumbera et al., 2004).
Natal mole-rats are among the few heterothermic mammals, exhibiting the lowest body temperatures of bathyergids, the narrowest thermal neutral zone, and one of the highest resting metabolic rates in bathyergids (Bennett et al., 1993b; Šumbera, 2019; Oosthuizen et al., 2021). However, the thermal neutral zone of bathyergids may increase at higher altitudes, and a lower body temperature has been suggested to be an adaptation to colder environments, enabling animals to expend less energy maintaining their body temperature at lower ambient temperatures (Broekman et al., 2006). We found that soil temperatures in foraging tunnels vary between 5 and 30°C below their thermal neutral zone during summer and winter, respectively, and these physiological adaptations may be an evolutionary response to shallow tunnels and the resultant seasonal climate fluctuations they experience. Body temperature was found to fluctuate with activity periods in laboratory studies, but the authors could not determine if activity caused an increase in body temperature (Haupt et al., 2017; van Jaarsveld et al., 2019). Digging and other burrow maintenance behaviors may increase body temperature in mole-rats (Zelová et al., 2010; Okrouhlík et al., 2015). Mole-rats living in shallow tunnels which experience greater temperature variation may need to be selective in their activity periods to prevent overheating due to their poor heat dissipating abilities (Luna et al., 2020; Wallace et al., 2021). However, increased soil moisture during summer may provide a cooling effect via conduction to decrease body temperature during or after activity bouts by pressing their ventral surface against a cooler surface (Okrouhlík et al., 2015; Vejmělka et al., 2021). Seasonal changes in daily activity would be an effective thermoregulatory adaptation to reduce the risk of overheating while digging (Goldman et al., 1997; McGowan et al., 2020). Locomotor activity was concentrated during periods of lower temperatures in laboratory studies (van Jaarsveld et al., 2019; Hart et al., 2021) and in periods of moderate temperature in wild studies (this study, Šklíba et al., 2014, 2016b). It cannot be determined if body temperature fluctuated due to fluctuations in burrow temperature or as a response to activity, or a combination of the two. Therefore, we cannot discern if a drop in body temperature stimulated animal activity to increase core body temperature via thermogenesis (Block, 1994) or if body temperature increased due to rising burrow temperatures as seen in naked mole-rats (Buffenstein and Yahav, 1991). While increased body temperature may be associated with higher activity levels in other rodents, circadian rhythms of body temperature may not result from daily activity rhythms (Refinetti, 1999; Refinetti and Kenagy, 2018). Future studies should attempt to determine the correlates of body temperature, tunnel temperature and activity patterns utilizing temperature loggers, RFID technology, or accelerometers (Williams et al., 2020).
Laboratory studies found differences in activity patterns between the sexes (Haupt et al., 2017; van Jaarsveld et al., 2019), yet we did not find sex-biased differences in activity patterns in colonies of free-ranging Natal mole-rats. Our study did identify an effect of body size on activity similar to radio-telemetry studies on wild mole-rats of the genus Fukomys which found that larger individuals were less active than smaller ones (Lovegrove, 1988; Lövy et al., 2013; Šklíba et al., 2016a). However, Francioli et al. (2020) found that body mass did not predict activity even though reproductive individuals were overall heavier than non-reproductive individuals. Relatedly, we did not find a difference in activity patterns between reproductive and non-reproductive individuals contrary to studies on the social mole-rats of the genus Fukomys which found that reproductive individuals were less active than non-reproductive individuals (Lovegrove, 1988; Oosthuizen and Bennett, 2015; Šklíba et al., 2016a; Van Daele et al., 2019; Francioli et al., 2020; Houslay et al., 2020). These results may indicate that reproductive individuals of Fukomys mole-rats must regulate energy expenditure between reproductive and burrow defense tasks. In Damaraland mole-rats reproductive suppression is controlled by physiological and behavioral mechanisms. Ovulation is blocked in non-reproductive females, but incest avoidance prevents breeding in males (Bennett et al., 1996; Molteno and Bennett, 2000). This suppression remains until the individual disperses from the group or an unrelated individual joins the group. In contrast, Natal mole-rats lack physiological reproductive suppression, and incest avoidance or aggressive interactions appear enough to prevent inbreeding (Oosthuizen et al., 2008). The similarities in activity patterns in Natal mole-rats may indicate more equal contributions to burrow maintenance and other cooperative behaviors between dominant and subordinate group members. It may also indicate that food resources are not as limited or scattered at the study site compared to the Kalahari where Damaraland mole-rats occur. Therefore, reproductive Natal mole-rats may not be required to conserve energy by reducing activity or relying on other group members to collect food. It may also indicate that reproductive individuals may need to be constantly on guard to prevent the intrusion of non-group members since groups are much closer together at this study site (Finn, unpublished data).
Activity patterns in social species may be affected by social cues, and social mole-rats observed singly may exhibit up to double the activity period of those in a complete colony (Hickman, 1980; Riccio and Goldman, 2000; Lövy et al., 2013). The findings of Lövy et al. (2013) particularly highlight the importance of maintaining social groups during observations. The authors found that a solitary female giant mole-rat (Fukomys mechowii) in natural conditions was more active during midday when temperatures were highest, compared to complete family groups which were more active at night (Lövy et al., 2013). Social mole-rats conserve heat via huddling and are more prone to heat loss during periods of lower burrow temperatures (Šumbera, 2019). Lövy et al. (2013) proposed that being active during the hottest part of the day minimized heat loss because solitary individuals cannot conserve heat via huddling. Mole-rats can shed excess heat from their feet, ventral surface, and via behavioral adjustments, such as rolling on their back or pressing their belly to the cooler soil of the tunnel (Vejmělka et al., 2021). This dissipation stays relatively constant regardless of ambient temperature. When ambient temperature drops, heat can be conserved through the vascular constriction in their feet, changes in body posture, or huddling with conspecifics (Kotze et al., 2008; Vejmělka et al., 2021). The fur of common mole-rats (Cryptomys hottentotus) was found to have better insulating properties compared to related species that live in tropical environments, and this may be an adaptation to greater fluctuations in burrow temperatures (Vejmělka et al., 2021). Behavioral thermoregulation (huddling, changes in body posture, or moving to warmer or colder areas of the burrow) may help mole-rats to maintain body temperature within their thermal neutral zone. It can be assumed that a decrease in body temperature may trigger locomotor activity, forcing an animal to become active to increase its body temperature (Block, 1994). However, mole-rats huddle with other group members in a nest during rest periods to conserve heat (Kotze et al., 2008). Thus, it would be unlikely that body temperature would significantly decrease while huddling. Therefore, mole-rats may time their periods of wakefulness and rest to coincide with daily burrow temperatures. Future studies investigating activity patterns in social mole-rats should maintain group cohesion to account for the effects of social cues on activity patterns. RFID technology can easily be applied in a laboratory setting to track individual activity patterns of group-living animals with the implementation of new open-source and 3D printing technology to decrease costs (Schielke et al., 2012; Habedank et al., 2021).
Predation avoidance has been suggested to affect activity patterns in other small mammals such as voles (Microtus ochrogaster, Getz, 2009). Activity periods for black-backed jackals (Canis mesomelas) and serval (Leptailurus serval), both known predators of Natal mole-rats, were primarily nocturnal during winter and crepuscular during summer (Ramesh and Downs, 2013; Humphries et al., 2015). Our results indicated that mole-rats reduced activity during these periods in winter, but during summer were more active during predator activity periods. In both serval and jackal, mole-rats make up < 5% of their diet and may be a seasonally available prey item (Humphries et al., 2015; Ramesh and Downs, 2015). Therefore, it is more likely that the low instances of predation on mole-rats by serval and jackal are due to a low encounter rate or greater effort required for capture, instead of mole-rats altering their daily activity patterns in response to predation pressure.
van Jaarsveld et al. (2019) argued that tunnel breaches did not occur frequently enough to allow circadian rhythm entrainment via photoperiod. However, exposure to light pulses at infrequent intervals was enough to entrain the circadian rhythm in other subterranean rodents (Decoursey and Menon, 1991; Rado et al., 1993). Recent work in South American tuco-tucos (Ctenomys aff. knighti) has shown that individuals may entrain their circadian rhythms by infrequent exposure to light during surface excursions (Jannetti et al., 2019; Flôres et al., 2021). At the study site, many groups of mole-rats occupied areas covered with a thick carpet of grass which would require chewing through before soil could be extruded from the burrow. Most mounds encountered at this site had a neat circular hole leading into the tunnel, indicating that mole-rats may spend considerable effort to “clean” the tunnel exit (K. Finn, A. Janse van Vuuren, pers. obs). Eloff (1951) noted that Cryptomys would “open a hole to the surface [and] put its nose out” during mound construction. At the study site, mole-rats continued to make mounds through the dry season, likely due to increased soil moisture of mesic grasslands in the Natal region compared to more arid regions of South Africa (K. Finn, A. Janse van Vuuren, pers. obs.). Thus, mole-rats may be exposed to light throughout the year during mound construction. A family group of mole-rats may produce between 6 and 7 mounds per day during the rainy season (Genelly, 1965; Jarvis et al., 1998). During mound construction, one individual may lead digging while other group members assist with sweeping excess soil away and most individuals in a group contribute to digging (Zelová et al., 2010). Since light may penetrate along the tunnel for less than a meter when the surface is breached (Kott et al., 2014), other individuals in the digging chain may be exposed to light instead of just the lead digging animal. Therefore, whether light or temperature is the dominant entrainer of circadian rhythms in mole-rats remains unclear.
This study used RFID technology as a non-invasive method to monitor activity patterns in a wild subterranean mammal. This method can be easily adapted to monitor activity in small mammals in areas that may involve safety risks to researchers, such as African reserves occupied by large predators. Hickman (1980) recommended studies to understand the effects of temperature and other non-photic cues on activity patterns in subterranean mammals. Surprisingly, in the 40 years since this suggestion, few laboratory studies have explored the effects of varying ambient temperatures on activity and only recently started gaining attention (Goldman et al., 1997; Haupt et al., 2017; van Jaarsveld et al., 2019; Flôres et al., 2021; Hart et al., 2021). There have been many field studies in various subterranean species where burrow or soil temperatures and activity patterns were measured (Gettinger, 1984; Cameron et al., 1988; Lovegrove, 1988; Šklíba et al., 2007, 2014, 2015, 2016b; Lövy et al., 2013; Vlasatá et al., 2017; Jannetti et al., 2019), with only a handful combining both laboratory and field observations (Rezende et al., 2003; Flôres et al., 2021). Results from studies on activity may differ significantly between wild and laboratory subjects of the same species due to artificial laboratory conditions (Rezende et al., 2003; Šklíba et al., 2014 and references therein). To date, only two studies have employed daily temperature fluctuations during observation while keeping animals under constant darkness (van Jaarsveld et al., 2019; Hart et al., 2021); however, these studies were on isolated individuals of a social species. One study has mimicked the periodic light pulses from mound construction (Rado et al., 1993), but they maintained a constant temperature during the experiment. Laboratory studies would benefit from maintaining social species in a tunnel-like atmosphere (sensu Zöttl et al., 2016) kept under constant darkness with and without infrequent light pulses and exposed to daily temperature fluctuations to simulate natural variations they experience in foraging tunnels during both winter and summer. The ingenious addition of photo-sensors and accelerometers on wild subterranean mammals (Flôres et al., 2021) would provide valuable data on individual daily activity bouts, quantify exposure to light, and shed light on the effects of photoperiod on the entrainment of circadian rhythms in bathyergids.
The datasets presented in this study can be found in online repositories. The names of the repository/repositories and accession number(s) can be found below: Figshare: https://doi.org/10.6084/m9.figshare.17041496.v3.
The animal study was reviewed and approved by the University of Pretoria Ethics Committee (EC001-19) and permits for the capture of mole-rats were provided by the Ezemvelo KZN Wildlife Authority (OP27-2020 and OP1545-2021).
KF, DH, NB, and MZ conceived and designed the study. KF, AJ, and TS collected the data. KF and DH analyzed the data. NB and MZ provided funding for research. KF wrote the first draft of the manuscript. All authors contributed to revisions.
Funding for this study was provided by a SARChI Chair of Mammal Behavioral Ecology and Physiology award from the Department of Science and Technology as well as National Research Foundation (grant no. 64756) to NB.
The authors declare that the research was conducted in the absence of any commercial or financial relationships that could be construed as a potential conflict of interest.
All claims expressed in this article are solely those of the authors and do not necessarily represent those of their affiliated organizations, or those of the publisher, the editors and the reviewers. Any product that may be evaluated in this article, or claim that may be made by its manufacturer, is not guaranteed or endorsed by the publisher.
We thank Tim Clutton-Brock for the use of the RFID reader array. We thank Yannick Francioli for providing code and assistance with data analysis. We thank Gareth and Kelly Sivright, as well as Vincent and Caitlin van Wyk at Glengarry Holiday Farm for accommodation and permission to capture mole-rats. We thank two reviewers for their valuable comments and suggestions which greatly improved the manuscript.
The Supplementary Material for this article can be found online at: https://www.frontiersin.org/articles/10.3389/fevo.2022.819393/full#supplementary-material
Allali, K., Achaâban, M. R., Bothorel, B., Piro, M., Bouâouda, H., Allouchi, M., et al. (2013). Entrainment of the circadian clock by daily ambient temperature cycles in the camel (Camelus dromedarius). Am. J. Physiol. Regulat. Integr. Compar. Physiol. 304, R1044–R1052. doi: 10.1152/ajpregu.00466.2012
Ameijeiras-Alonso, J., Crujeiras, R. M., and Rodríguez-Casal, A. (2019). Mode testing, critical bandwidth and excess mass. TEST 28, 900–919. doi: 10.1007/s11749-018-0611-5
Ameijeiras-Alonso, J., Crujeiras, R. M., and Rodríguez-Casal, A. (2021). multimode: an R Package for Mode Assessment. J. Statist. Softw. 97, 1–32.
Andersen, D. C., and MacMahon, J. A. (1981). Population dynamics and bioenergetics of a fossorial herbivore, Thomomys talpoides (Rodentia: geomyidae), in a spruce-fir sere. Ecol. Monogr. 51, 179–202. doi: 10.2307/2937262
Beale, A. D., Whitmore, D., and Moran, D. (2016). Life in a dark biosphere: a review of circadian physiology in “arrhythmic” environments. J. Compar. Physiol. B 186, 947–968. doi: 10.1007/s00360-016-1000-6
Begall, S., Daan, S., Burda, H., and Overkamp, G. J. F. (2002). Activity patterns in a subterranean social rodent, Spalacopus cyanus (Octodontidae). J. Mammal. 83, 153–158. doi: 10.1644/1545-15422002083<0153:APIASS<2.0.CO;2
Benedix, J. H. (1994). A predictable pattern of daily activity by the pocket gopher Geomys bursarius. Anim. Behav. 48, 501–509. doi: 10.1006/anbe.1994.1271
Bennett, N. C. (1990). Behaviour and social organization in a colony of the Damaraland mole-rat Cryptomys damarensis. J. Zool. Lond. 220, 225–248. doi: 10.1111/j.1469-7998.1990.tb04305.x
Bennett, N. C. (1992). The locomotory activity patterns of a functionally complete colony of Cryptomys hottentotus hottentotus (Rodentia: bathyergidae). J. Zool. Lond. 288, 435–443. doi: 10.1111/j.1469-7998.1992.tb04446.x
Bennett, N. C., and Faulkes, C. G. (2000). African Mole-rats: ecology and Eusociality. Cambridge: Cambridge University Press.
Bennett, N. C., Jarvis, J. U. M., and Cotterill, F. P. D. (1993a). Poikilothermic traits and thermoregulation in the Afrotropical social subterranean Mashona mole-rat (Cryptomys hottentotus darlingi) (Rodentia: bathyergidae). J. Zool. Lond. 231, 179–186. doi: 10.1111/j.1469-7998.1993.tb01910.x
Bennett, N. C., Taylor, P. J., and Aguilar, G. H. (1993b). Thermoregulation and metabolic acclimation in the Natal mole-rat (Cryptomys hottentotus natalensis) (Rodentia:Bathyergidae). Z. Säugetierkund. 58, 362–367. doi: 10.1007/BF00323153
Bennett, N. C., Jarvis, J. U. M., and Davies, K. C. (1988). Daily and seasonal temperatures in the burrows of African rodent moles. South Afr. J. Zool. 236, 189–198. doi: 10.1080/02541858.1988.11448101
Bennett, N. C., Faulkes, C. G., and Molteno, A. J. (1996). Reproductive suppression in subordinate, non-breeding female Damaraland mole-rats: two components to a lifetime of socially induced infertility. Proc. R. Soc. Lond. B 263, 1599–1603. doi: 10.1098/rspb.1996.0234
Benstaali, C., Mailloux, A., Bogdan, A., Auzéby, A., and Touitou, Y. (2001). Circadian rhythms of body temperature and motor activity in rodents their relationships with the light-dark cycle. Life Sci. 68, 2645–2656. doi: 10.1016/s0024-3205(01)01081-5
Burda, H., Šumbera, R., Begall, S. (2007). “Microclimate in burrows of subterranean rodents–revisited,” in Subterranean Rodents: News from Underground, eds Begall, S., Burda, H., and Schleich, C. E. (Berlin Heidelberg: Springer-Verlag), pp. 21–34.
Block, B. A. (1994). Thermogenesis in muscle. Annu. Rev. Physiol. 56, 535–577. doi: 10.1146/annurev.ph.56.030194.002535
Brooks, M. E., Kristensen, K., van Benthem, K. J., Magnusson, A., Berg, C. W., Nielsen, A., et al. (2017). glmmTMB balances speed and flexibility among packages for zero-inflated generalized linear mixed modeling. R J. 9, 378–400. doi: 10.32614/rj-2017-066
Boyer, A. C., and MacDougall-Shackleton, S. A. (2020). High rates of exposure to simulated winter storm cues negatively affect White-Throated Sparrow (Zonotrichia albicollis) energy reserves. Front. Ecol. Evol. 8:222. doi: 10.3389/fevo.2020.00222
Broekman, M., Bennett, N. C., Jackson, C. R., and Scantlebury, M. (2006). Mole-rats from higher altitudes have greater thermoregulatory capabilities. Physiol. Behav. 89, 750–754. doi: 10.1016/j.physbeh.2006.08.023
Buffenstein, R., and Yahav, S. (1991). Is the naked mole-rat Hetercephalus glaber an endothermic yet poikilothermic mammal? J. Ther. Biol. 16, 227–232. doi: 10.1016/0306-4565(91)90030-6
Cain, J. W., Krausman, P. R., Rosenstock, S. S., and Turner, J. C. (2006). Mechanisms of thermoregulation and water balance in desert ungulates. Wildlife Soc. Bull. 34, 570–581. doi: 10.2193/0091-7648(2006)34[570:motawb]2.0.co;2
Cameron, G. N., Spencer, S. R., Eshelman, B. D., Williams, L. R., and Gergory, M. J. (1988). Activity and burrow structure of Attwater’s pocket gopher (Geomys attwateri). J. Mammal. 69, 667–677. doi: 10.2307/1381621
Decoursey, P. J., and Menon, S. A. (1991). Circadian photo-entrainment in a nocturnal rodent: quantitative measurement of light-sampling activity. Anim. Behav. 41, 781–785. doi: 10.1016/S0003-3472(05)80344-6
Eloff, G. (1951). Orientation in the mole-rat Cryptomys. Br. J. Psychol. 42, 134–145. doi: 10.1111/j.2044-8295.1951.tb00285.x
Farsi, H., Achaâban, M. R., Piro, M., Bothorel, B., Ouassat, M., Challet, E., et al. (2020a). Entrainment of circadian rhythms of locomotor activity by ambient temperature cycles in the dromedary camel. Sci. Rep. 10:19515. doi: 10.1038/s41598-020-76535-y
Farsi, H., Harti, D., Achaâban, M. R., Piro, M., Raverot, V., Bothorel, B., et al. (2020b). Melatonin rhythm and other outputs of the master circadian clock in the desert goat (Capra hircus) are entrained by daily cycles of ambient temperature. J. Pineal Res. 68:e12634. doi: 10.1111/jpi.12634
Finn, K. T., Parker, D. M., Bennett, N. C., and Zöttl, M. (2018). Contrasts in body size and growth suggest that high population density results in a faster pace of life in Damaraland mole-rats (Fukomys damarensis). Can. J. Zool. 96, 920–927. doi: 10.1139/cjz-2017-0200
Flôres, D. E. F. L., Jannetti, M. G., Improta, G. C., Tachinardi, P., Valentinuzzi, V. S., and Oda, G. A. (2021). Telling the seasons underground: the circadian clock and ambient temperature shape light exposure and photoperiodism in a subterranean rodent. Front. Physiol. 12:738471. doi: 10.3389/fphys.2021.738471
Francioli, Y., Thorley, J., Finn, K., Clutton-Brock, T., and Zöttl, M. (2020). Breeders are less active foragers than non-breeders in wild Damaraland mole-rats. Biol. Lett. 16:20200475. doi: 10.1098/rsbl.2020.0475
Jarvis, J. U. M., and Bennett, N. C. (1993). Eusociality has evolved independently in two genera of bathyergid mole-rats - but occurs in no other subterranean mammal. Behav. Ecol. Sociobiol. 33, 353–360. doi: 10.1007/BF02027122
Garthe, S., Markones, N., Hüppop, O., and Adler, S. (2009). Effects of hydrographic and meteorological factors on seasonal seabird abundance in the southern North Sea. Mar. Ecol. Progress Ser. 391, 243–255. doi: 10.3354/meps08170
Genelly, R. E. (1965). Ecology of the common mole-rat (Cryptomys hottentotus) in Rhodesia. J. Mammal. 46, 647–665. doi: 10.2307/1377935
Gettinger, R. D. (1984). A field study of activity patterns of Thomomys bottae. J. Mammal. 65, 76–84. doi: 10.2307/1381202
Getz, L. L. (2009). Circadian activity rhythm and potential predation risk of the prairie vole, Microtus ochrogaster. Southwestern Natural. 54, 146–150. doi: 10.1894/PS-40.1
Gerkema, M. P., and Daan, S. (1985). Ultradian rhythms in behavior: the case of the common vole (Microtus arvalis). Exp. Brain Res. Suppl. 12, 11–31. doi: 10.1007/978-3-642-70483-3_3
Goldman, B. D., Goldman, S. L., Riccio, A. P., and Terkel, J. (1997). Circadian patterns of locomotor activity and body temperature in blind mole-rats Spalax ehrenbergi. J. Biol. Rhythms 12, 348–361. doi: 10.1177/074873049701200407
Habedank, A., Urmersbach, B., Kahnau, P., and Lewejohann, L. (2021). O mouse, where art thou? The mouse position surveillance system (MoPSS) – an RFID-based tracking system. Behav. Res. Methods doi: 10.3758/s13428-021-01593-7
Halle, S. (1995). Diel patterns of locomotor activity in populations of root voles, Microtus oeconomus. J. Biol. Rhythms 10, 211–224. doi: 10.1177/074873049501000304
Halle, S. (2006). Polyphasic activity patterns in small mammals. Folia Primatol. 77, 15–26. doi: 10.1159/000089693
Hart, L., Bennett, N. C., Malpaux, B., Chimimba, C. T., and Oosthuizen, M. K. (2004). The chronobiology of the Natal mole-rat, Cryptomys hottentotus natalensis. Physiol. Behav 82, 563–569. doi: 10.1016/j.physbeh.2004.05.008
Hart, D. W., van Jaarsveld, B., Lasch, K. G., Grenfell, K. L., Oosthuizen, M. K., and Bennett, N. C. (2021). Ambient temperature as a strong zeitgeber of circadian rhythms in response to temperature sensitivity and poor heat dissipation abilities in subterranean African mole-rats. J. Biol. Rhythms 36, 461–469. doi: 10.1177/07487304211034287
Haupt, M., Bennett, N. C., and Oosthuizen, M. K. (2017). Locomotor activity and body temperature patterns over a temperature gradient in the Highveld mole-rat (Cryptomys hottentotus pretoriae). PLoS One 12:e0169644. doi: 10.1371/journal.pone.0169644
Hazlerigg, D. G., and Tyler, N. J. C. (2019). Activity patterns in mammals: circadian dominance challenged. PLoS Biol. 17:e3000360. doi: 10.1371/journal.pbio.3000360
Hickman, G. C. (1979). Burrow system structure of the Bathyergid Cryptomys hottentotus in Natal, South Africa. Z. Säugetierkund. 44, 153–162.
Hickman, G. C. (1980). Locomotory activity of captive Cryptomys hottentotus (Mammalia: bathyergidae), a fossorial rodent. J. Zool. Lond. 192, 225–235. doi: 10.1111/j.1469-7998.1980.tb04231.x
Hinze, A., and Pillay, N. (2006). Life in an African alpine habitat: diurnal activity patterns of the ice rat Otomys sloggetti robertsi. Arct. Antarct Alp. Res. 38, 540–546.
Holtze, S., Braude, S., Lemma, A., Koch, R., Morhart, M., Szafranski, K., et al. (2018). The microenvironment of naked mole-rat burrows in East Africa. Afr. J. Ecol. 56, 279–289. doi: 10.1111/aje.12448
Houslay, T. M., Vullioud, P., Zöttl, M., and Clutton-Brock, T. H. (2020). Benefits of cooperation in captive Damaraland mole-rats. Behav. Ecol. 31, 711–718. doi: 10.1093/beheco/araa015
Humphries, B. D., Ramesh, T., and Downs, C. T. (2015). Diet of black-backed jackals (Canis mesomelas) on farmlands in the KwaZulu-Natal Midlands. South Africa. Mammalia 80, 405–412. doi: 10.1515/mammalia-2014-0103
Inman, A. J., and Krebs, J. (1987). Predation and group living. Trends Ecol. Evol. 2, 31–32. doi: 10.1016/0169-5347(87)90093-0
Jannetti, M. G., Buck, C. L., Valentinuzzi, V. S., and Oda, G. A. (2019). Day and night in the subterranean: measuring daily activity patterns of subterranean rodents (Ctenomys aff. knighti) using bio-logging. Conserv. Physiol. 7:coz044. doi: 10.1093/conphys/coz044
Jarvis, J. U. M. (1973). Activity patterns in the mole-rats Tachyoryctes splendens and Heliophobius argenteocinereus. Zool. Afr. 8, 101–119. doi: 10.1080/00445096.1973.11447470
Jarvis, J. U. M., Bennett, N. C., and Spinks, A. C. (1998). Food availability and foraging by wild colonies of Damaraland mole-rats (Cryptomys damarensis): implications for sociality. Oecologia 113, 290–298. doi: 10.1007/s004420050380
Johnson, C. H., Elliott, J. A., and Foster, R. (2003). Entrainment of circadian programs. Chronobiol. Int. 20, 741–774. doi: 10.1081/CBI-120024211
Korslund, L. (2006). Activity of root voles (Microtus oeconomus) under snow: social encounters synchronizes individual activity rhythms. Behav. Ecol. Sociobiol. 61, 255–263. doi: 10.1007/s00265-006-0256-3
Kott, O., Moritz, R. E., Šumbera, R., Burda, H., and Nìmec, P. (2014). Light propagation in burrows of subterranean rodents: tunnel system architecture but not photoreceptor sensitivity limits light sensation range. J. Zool. Lond. 294, 68–76. doi: 10.1111/jzo.12152
Kotze, J., Bennett, N. C., and Scantlebury, M. (2008). The energetics of huddling in two species of mole-rat (Rodentia: bathyergidae). Physiol. Behav. 93, 215–221. doi: 10.1016/j.physbeh.2007.08.016
Lovegrove, B. G. (1988). Colony size and structure, activity patterns and foraging behaviour of a colony of the social mole-rat Cryptomys damarensis (Bathyergidae). J. Zool. Lond. 216, 391–402. doi: 10.1111/j.1469-7998.1988.tb02437.x
Lovegrove, B. G., and Painting, S. (1987). Variations in the foraging behaviour and burrow structures of the Damara molerat Cryptomys damarensis in the Kalahari Gemsbok National Park. Koedoe 30, 149–163.
Lövy, M., Šklíba, J., Hrouzková, E., Dvoøáková, V., Nevo, E., and Šumbera, R. (2015). Habitat and burrow system characteristics of the blind mole rat Spalax galili in an area of supposed sympatric speciation. PLoS One 10:e0133157. doi: 10.1371/journal.pone.0133157
Lövy, M., Šklíba, J., and Šumbera, R. (2013). Spatial and temporal activity patterns of the free-living giant mole-rat (Fukomys mechowii), the largest social Bathyergid. PLoS One 8:e55357. doi: 10.1371/journal.pone.0055357
Luna, F., Šumbera, R., Okrouhlík, J., Mladìnková, N., and Antenucci, C. D. (2020). Evaporative water loss in seven species of fossorial rodents: does effect of degree of fossoriality and sociality exist? J. Ther. Biol. 89:102564. doi: 10.1016/j.jtherbio.2020.102564
Marhold, S., and Nagel, A. (1995). The energetics of the common mole rat Cryptomys, a subterranean eusocial rodent from Zambia. J. Compar. Physiol. B 164, 636–645. doi: 10.1007/BF00389805
McGowan, N. E., Scantlebury, D. M., Bennett, N. C., Maule, A. G., and Marks, N. J. (2020). Thermoregulatory differences in African mole-rat species from disparate habitats: responses and limitations. J. Ther. Biol. 88:102495. doi: 10.1016/j.jtherbio.2019.102495
Mistlberger, R. E., and Skene, D. J. (2004). Social influences on mammalian circadian rhythms: animal and human studies. Biol. Rev. 79, 533–556. doi: 10.1017/S1464793103006353
Molteno, A. J., and Bennett, N. C. (2000). Anovulation in non-reproductive female Damaraland mole-rats (Cryptomys damarensis). J. Reprod. Fertil. 119, 25–41. doi: 10.1530/jrf.0.1190035
Muñoz-Sabater, J. (2019). ERA5-Land Hourly Data From 1981 to Present. United Kingdom: european Centre for Medium-Range Weather Forecasts. doi: 10.24381/cds.e2161bac
Muñoz-Sabater, J., Dutra, E., Agustí-Panareda, A., Albergel, C., Arduini, G., Balsamo, G., et al. (2021). ERA5-Land: a state-of-the-art global reanalysis dataset for land applications. Earth Syst. Sci. Data 13, 4349–4383. doi: 10.5194/essd-13-4349-2021
Okrouhlík, J., Burda, H., Kunc, P., Knížková, I., and Šumbera, R. (2015). Suprisingly low risk of overheating during digging in two subterranean rodents. Physiol. Behav. 138, 236–241. doi: 10.1016/j.physbeh.2014.10.029
Oosthuizen, M. K., and Bennett, N. C. (2015). The effect of ambient temperature on locomotor activity patterns in reproductive and non-reproductive female Damaraland mole-rats. J. Zool. Lond. 297, 1–8. doi: 10.1111/jzo.12254
Oosthuizen, M. K., Bennett, N. C., Lutermann, H., and Coen, C. W. (2008). Reproductive suppression and the seasonality of reproduction in the social Natal mole-rat (Cryptomys hottentotus natalensis). Gener. Compar. Endocrinol. 159, 236–240. doi: 10.1016/j.ygcen.2008.09.004
Oosthuizen, M. K., Cooper, H. M., and Bennett, N. C. (2003). Circadian rhythms of locomotor activity in solitary and social species of African mole-rats (Family: bathyergidae). J. Biol. Rhythms 18, 481–490. doi: 10.1177/0748730403259109
Oosthuizen, M. K., Robb, G., Harrison, A., Froneman, A., Joubert, K., and Bennett, N. C. (2021). Flexibility in body temperature rhythms of free-living natal mole-rats (Cryptomys hottentotus natalensis). J. Ther. Biol. 99:102973. doi: 10.1016/j.jtherbio.2021.102973
Oster, H., Avivi, A., Joel, A., Albrecht, U., and Nevo, E. (2002). A switch from diurnal to nocturnal activity in S. ehrenbergi is accompanied by an uncoupling of light input and the circadian clock. Curr. Biol. 12, 1919–1922. doi: 10.1016/S0960-9822(02)01263-0
R Core Team (2021). R: a Language and Environment for Statistical Computing. Vienna: R Foundation for Statistical Computing.
Rado, R., Wollberg, Z., and Terkel, J. (1993). Dispersal of young mole rats (Spalax ehrenbergi) from the natal burrow. J. Mammal. 73, 885–890. doi: 10.2307/1382211
Ramesh, T., and Downs, C. T. (2013). Impact of farmland use on population density and activity patterns of serval in South Africa. J. Mammal. 94, 1460–1470.
Ramesh, T., and Downs, C. T. (2015). Diet of serval (Leptailurus serval) on farmlands in the Drakensberg Midlands, South Africa. Mammalia 79, 399–407. doi: 10.1515/mammalia-2014-0053
Randall, J. A. (1993). Behavioural adaptations of desert rodents. Anim. Behav. 45, 263–287. doi: 10.1006/anbe.1993.1032
Refinetti, R. (1999). Relationship between the daily rhythms of locomotor activity and body temperature in eight mammalian species. Am. J. Physiol. 277, R1493–R1500. doi: 10.1152/ajpregu.1999.277.5.R1493
Refinetti, R. (2015). Comparison of light, food, and temperature as environmental synchronizers of the circadian rhythm of activity in mice. J. Physiol. Sci. 65, 359–366. doi: 10.1007/s12576-015-0374-7
Refinetti, R., and Kenagy, G. J. (2018). Circadian rhythms of body temperature and locomotor activity in the antelope ground squirrel, Ammospermophilus leucurus. J. Ther. Biol. 72, 67–72. doi: 10.1016/j.jtherbio.2018.01.001
Rezende, E. L., Cortes, A., Bacigalupe, L. D., Nespolo, R. F., and Bozinovic, F. (2003). Ambient temperature limits above-ground activity of the subterranean rodent Spalacopus cyanus. J. Arid Environ. 55, 63–74. doi: 10.1016/S0140-1963(02)00259-8
Rensing, L., and Ruoff, P. (2002). Temperature effect on entrainment, phase shifting, and amplitude of circadian clocks and its molecular bases. Chronobiol. Int. 19, 807–864. doi: 10.1081/cbi-120014569
Riccio, A. P., and Goldman, B. D. (2000). Circadian rhythms of locomotor activity in naked mole-rats (Hetercephalus glaber). Physiol. Behav. 71, 1–13. doi: 10.1016/s0031-9384(00)00281-x
Roll, U., Dayan, T., and Kronfeld-Schor, N. (2006). On the role of phylogeny in determining activity patterns of rodents. Evol. Ecol. 20, 479–490. doi: 10.1007/s10682-006-0015-y
Schielke, C. K. M., Begall, S., and Burda, H. (2012). Reproductive state does not influence activity budgets of eusocial Ansell’s mole-rats, Fukomys anselli (Rodentia, Bathyergidae): a study of locomotor activity by means of RFID. Mammal. Biol. 77, 1–5. doi: 10.1016/j.mambio.2011.09.004
Šklíba, J., Lövy, M., Hrouzková, E., Kott, O., Okrouhlík, J., and Šumbera, R. (2014). Social and environmental influences on daily activity pattern in free-living subterranean rodents: the case of a eusocial Bathyergid. J. Biol. Rhythms 29, 203–214. doi: 10.1177/0748730414526358
Šklíba, J., Lövy, M., Burda, H., and Šumbera, R. (2016a). Variability of space-use patterns in a free living eusocial rodent, Ansell’s mole-rat indicates age-based rather than caste polyethism. Sci. Rep. 6:37497. doi: 10.1038/srep38497
Šklíba, J., Lövy, M., Koepepen, S. C. W., Pleštilová, L., Vitámvás, M., Nevo, E., et al. (2016b). Activity of free-living subterranean blind mole rats Spalax galili (Rodentia: spalacidae) in an area of supposed sympatric speciation. Biol. J. Linnean Soc. 118, 280–291. doi: 10.1111/bij.12741
Šklíba, J., Mazoch, V., Patzenhauerová, H., Hrouzková, E., Lövy, M., Kott, O., et al. (2012). A maze-lover’s dream: burrow architecture, natural history and habitat characteristics of Ansell’s mole-rat (Fukomys anselli). Mammal. Biol. 77, 420–427. doi: 10.1016/j.mambio.2012.06.004
Šklíba, J., Šumbera, R., Chitaukali, W. N., and Burda, H. (2007). Determinants of daily activity patterns in a free-living Afrotropical solitary subterranean rodent. J. Mammal. 88, 1009–10016. doi: 10.1644/06-MAMM-A-235R1.1
Spinks, A. C., Branch, T. A., Croeser, S., Bennett, N. C., and Jarvis, J. U. M. (1999). Foraging in wild and captive colonies of the common mole-rat Cryptomys hottentotus hottentotus (Rodentia: bathyergidae). J. Zool. Lond. 249, 143–152. doi: 10.1111/j.1469-7998.1999.tb00752.x
Šumbera, R. (2019). Thermal biology of a strictly subterranean mammalian family, the African mole-rats (Bathyergidae, Rodentia) – a review. J. Ther. Biol. 79, 166–189. doi: 10.1016/j.jtherbio.2018.11.003
Šumbera, R., Chitaukali, W. N., Elichová, M., Kubová, J., and Burda, H. (2004). Microclimatic stability in burrows of an Afrotropical solitary bathyergid rodent, the silvery mole-rat (Heliophobius argenteocinereus). J. Zool. Lond. 263, 409–416. doi: 10.1017/S095283690400545X
Šumbera, R., Lövy, M., Marino, J., Šimek, M., and Šklíba, J. (2020). Gas composition and its daily changes within burrows and nests of an Afroalpine fossorial rodent, the giant root-rat Tachyoryctes macrocephalus. Zoology 142:125819. doi: 10.1016/j.zool.2020.125819
Šumbera, R., Mazoch, V., Patzenhauerová, H., Lövy, M., Šklíba, J., Bryja, J., et al. (2012). Burrow architecture, family composition and habitat characteristics of the largest social African mole-rat: the giant mole-rat constructs really giant burrow systems. Acta Theriol. 57, 121–130. doi: 10.1007/s13364-011-0059-4
Van Daele, P. A. A. G., Desmet, N., Šumbera, R., and Adriaens, D. (2019). Work behaviour and biting performance in the cooperative breeding Micklem’s mole-rat Fukomys micklemi (Bathyergidae, Rodentia). Mammal. Biol. 95, 69–76. doi: 10.1016/j.mambio.2019.02.002
van Jaarsveld, B., Bennett, N. C., Hart, D. W., and Oosthuizen, M. K. (2019). Locomotor activity and body temperature rhythms in the Mahali mole-rat (C. h. mahali): the effect of light and ambient temperature variations. J. Ther. Biol. 79, 24–32. doi: 10.1016/j.jtherbio.2018.11.013
Vejmělka, F., Okrouhlík, J., Lövy, M., Šaffa, G., Nevo, E., Bennett, N. C., et al. (2021). Heat dissipation in subterranean rodents: the role of body region and social organization. Sci. Rep. 11:2029. doi: 10.1038/s41598-021-81404-3
Vlasatá, T., Šklíba, J., Lövy, M., Meheretu, Y., Sillero-Zubiri, C., and Šumbera, R. (2017). Daily activity patterns in the giant root rat (Tachyoryctes macrocephalus), a fossorial rodent from the Afro-alpine zone of the Bale Mountains, Ethiopia. J. Zool. Lond. 302, 157–163. doi: 10.1111/jzo.12441
Wallace, K. M. E., van Jaarsveld, B., Bennett, N. C., and Hart, D. W. (2021). The join effect of micro- and macro-climate on the thermoregulation and heat dissipation of two African mole-rats (Bathyergidae) sub-species, Cryptomys hottentotus mahali and C. h. pretoriae. J. Ther. Biol. 99:103025. doi: 10.1016/j.jtherbio.2021.103025
Williams, C. T., Barnes, B. M., and Buck, C. L. (2012). Daily body temperature rhythms persist under the midnight sun but are absent during hibernation in free-living arctic ground squirrels. Biol. Lett. 8, 31–34. doi: 10.1098/rsbl.2011.0435
Williams, H. J., Taylor, L. A., Benhamou, S., Bijleveld, A. I., Clay, T. A., de Grissac, S., et al. (2020). Optimizing the use of biologgers for movement ecology research. J. Anim. Ecol. 89, 186–206. doi: 10.1111/1365-2656.13094
Zelová, J., Šumbera, R., Okrouhlík, J., and Burda, H. (2010). Cost of digging is determined by intrinsic factors rather than by substrate quality in two subterranean rodent species. Physiol. Behav. 99, 54–58. doi: 10.1016/j.physbeh.2009.10.007
Keywords: locomotor activity, temperature, subterranean mammal, mole-rat, RFID, circadian rhythm
Citation: Finn KT, Janse van Vuuren AK, Hart DW, Süess T, Zöttl M and Bennett NC (2022) Seasonal Changes in Locomotor Activity Patterns of Wild Social Natal Mole-Rats (Cryptomys hottentotus natalensis). Front. Ecol. Evol. 10:819393. doi: 10.3389/fevo.2022.819393
Received: 21 November 2021; Accepted: 18 January 2022;
Published: 11 February 2022.
Edited by:
Ralph E. Mistlberger, Simon Fraser University, CanadaReviewed by:
Roberto Refinetti, University of New Orleans, United StatesCopyright © 2022 Finn, Janse van Vuuren, Hart, Süess, Zöttl and Bennett. This is an open-access article distributed under the terms of the Creative Commons Attribution License (CC BY). The use, distribution or reproduction in other forums is permitted, provided the original author(s) and the copyright owner(s) are credited and that the original publication in this journal is cited, in accordance with accepted academic practice. No use, distribution or reproduction is permitted which does not comply with these terms.
*Correspondence: Kyle T. Finn, a3lsZXRmaW5uQGdtYWlsLmNvbQ==
†ORCID: Kyle T. Finn, orcid.org/0000-0003-3119-9510; Andries K. Janse van Vuuren, orcid.org/0000-0001-6683-1542; Daniel W. Hart, orcid.org/0000-0002-4592-558X; Tobias Süess, orcid.org/0000-0003-1422-7364; Markus Zöttl, orcid.org/0000-0002-5582-2306; Nigel C. Bennett, orcid.org/0000-0001-9748-2947
‡These authors have contributed equally to this work and share last authorship
Disclaimer: All claims expressed in this article are solely those of the authors and do not necessarily represent those of their affiliated organizations, or those of the publisher, the editors and the reviewers. Any product that may be evaluated in this article or claim that may be made by its manufacturer is not guaranteed or endorsed by the publisher.
Research integrity at Frontiers
Learn more about the work of our research integrity team to safeguard the quality of each article we publish.