- 1Centre for Integrative Ecology, School of Life and Environmental Sciences, Deakin University, Geelong, VIC, Australia
- 2Doñana Biological Station EBD-CSIC, Seville, Spain
Climate change is pushing organisms closer to their physiological limits. Animals can reduce heat exposure – and the associated risks of lethal hyperthermia and dehydration – by retreating into thermal refuges. Refuge use nonetheless reduces foraging and reproductive activities, and thereby potentially fitness. Behavioural responses to heat thus define the selection pressures to which individuals are exposed. However, whether and why such behavioural responses vary between individuals remains largely unknown. Here, we tested whether early-life experience generates inter-individual differences in behavioural responses to heat at adulthood. In the arid-adapted zebra finch, parents incubating at high temperatures emit “heat-calls,” which adaptively alter offspring growth. We experimentally manipulated individual early life acoustic and thermal experience. At adulthood, across two summers, we then repeatedly recorded individual panting behaviour, microsite use, activity (N = 2,402 observations for 184 birds), and (for a small subset, N = 23 birds) body temperature, over a gradient of air temperatures (26–38°C), in outdoor aviaries. We found consistent inter-individual variation in behavioural thermoregulation, and show for the first time in endotherms that early-life experience contributes to such variation. Birds exposed prenatally to heat-calls started panting at lower temperatures than controls but panted less at high temperatures. It is possible that this corresponds to a heat-regulation strategy to improve water saving at high temperature extremes, and/or, allow maintaining high activity levels, since heat-call birds were also more active across the temperature gradient. In addition, microsite use varied with the interaction between early acoustic and thermal experiences, control-call birds from cooler nests using the cooler microsite more than their hot-nest counterparts, whereas the opposite pattern was observed in heat-call birds. Overall, our study demonstrates that a prenatal acoustic signal of heat alters how individuals adjust behaviourally to thermal challenges at adulthood. This suggests that there is scope for selection pressures to act differently across individuals, and potentially strengthen the long-term fitness impact of early-life effects.
Introduction
Global temperatures are increasing at an unprecedented rate, and heatwaves are becoming more frequent and longer (IPCC, 2014). Climate change is transforming ecosystems across the globe and pushing organisms beyond their physiological limits (Conradie et al., 2019, 2020; McKechnie and Wolf, 2019; Stillman, 2019), fuelling population declines across taxa (Dirzo et al., 2014; Urban, 2015; Riddell et al., 2019; Rosenberg et al., 2019). Among endotherms, birds may be particularly at risk, due to their relatively small size and diurnal activity. In the short-term, extreme heat exposes organisms to an immediate risk of lethal dehydration and hyperthermia (McKechnie and Wolf, 2010; Albright et al., 2017), which has caused mass-mortality events, including in birds and bats (Welbergen et al., 2008; McKechnie et al., 2012, 2021). In the longer-term, high temperatures can impose important fitness costs through effects on reproduction and body condition (Cunningham et al., 2021; Oswald et al., 2021). Such selection pressures from high temperatures are exacerbated under climate change.
Yet, at any one place in time, selection by high temperatures does not operate equally across individuals. This is because individuals respond behaviourally to thermal challenges (Smit et al., 2016; Xie et al., 2017; Thompson et al., 2018; Pattinson et al., 2020). To avoid lethal hyperthermia, birds rely on thermoregulatory behaviours, such as panting, to dissipate heat. Panting allows dissipating excess heat through respiratory evaporative water loss (EWL) (Calder and King, 1974; Wolf and Walsberg, 1996; McKechnie and Wolf, 2019). Panting must thus be traded-off against water loss, and lethal dehydration risk. Importantly, however, organisms can considerably buffer their exposure to heat, by seeking thermal refuge into cooler microsites (Cunningham et al., 2015; Martin et al., 2015; Oswald et al., 2019). For instance, in rufous-eared warblers (Malcorus pectoralis), the environmental temperature in shaded off-ground microsites was up to 20°C cooler than sun-exposed sites on the ground, and individuals increased the time spent in these shaded microsites by 131% on hot days (Pattinson and Smit, 2017). However, microsite use physically constrains activity and foraging (Tieleman and Williams, 2002; Cunningham et al., 2015), with potential carry-over effects on body condition (du Plessis et al., 2012; van de Ven et al., 2019; Oswald et al., 2021) and breeding success (Nilsson and Nord, 2018; Sharpe et al., 2019; Cook et al., 2020; van de Ven et al., 2020). Behavioural responses to heat are therefore essential, because they define the selection pressures individuals are exposed to, and the life-history traits affected by selection. Surprisingly however, whether behavioural thermoregulation varies between individuals and the sources of such variation remain largely unknown.
Studies on passerines have increasingly revealed intra-specific variation in thermoregulation and heat tolerance between seasons (Noakes et al., 2016a; Oswald et al., 2018b), years (Noakes and McKechnie, 2019) and populations (Smit et al., 2013; Noakes et al., 2016b), following acclimatisation patterns to different climatic conditions. For inter-individual variation, however, we are aware of only two studies on behavioural thermoregulation. The onset of panting behaviour in zebra finches (Taeniopygia guttata) exposed to a standard increase in air temperature (Tair) in a metabolic chamber is repeatable within individuals, but varies considerably between individuals, with some individuals starting panting at 27°C, while others do not pant until air temperature reaches 40°C (Pessato et al., 2020). This suggests that individuals may consistently vary in their thermoregulation strategies. In addition, in African passerines, microsite use varies with social status (Cunningham et al., 2017): dominant individuals used shaded sites more than subordinates in the territorial fawn-coloured lark (Mirafra africanoides) and the loosely social red-eyed bulbul (Pycnonotus nigricans), but not in the colonial sociable weaver (Philetairus socius) (Cunningham et al., 2017).
Such inter-individual variation in behavioural thermoregulation at adulthood may arise from the long-lasting effects of developmental conditions on phenotypes (i.e., developmental programming) (West-Eberhard, 2003; Durant et al., 2013; Groothuis et al., 2019). Surprisingly this has, to date, only been tested in ectotherms, where incubation temperatures affect thermoregulatory behaviours later in life, such as time spent basking or temperature selection (i.e., microsite preference) (reviewed in Refsnider et al., 2019). In endotherms, and specifically in birds, it was recently suggested that developmental programming for high temperatures may occur through prenatal acoustic communication (Mariette and Buchanan, 2016), as prenatal sounds adaptively alter development in a range of vertebrate and invertebrate species (Mariette and Buchanan, 2016; Noguera and Velando, 2019; Mariette et al., 2021). Notably, in the arid-adapted zebra finch, embryonic exposure to “heat-calls,” emitted by parents incubating at high temperatures, affects nestling growth under high postnatal nest temperatures, with positive effects on reproductive fitness at adulthood (Mariette and Buchanan, 2016). In addition, such prenatal heat-call exposure shifted thermal preferences in males, which preferred breeding in hotter nest sites than males exposed to control calls (Mariette and Buchanan, 2016). While this suggests long-term behavioural changes, we do not know whether prenatal acoustic signals could also affect individual behavioural responses to heat, and thereby modulate the selective pressures operating on these two phenotypes.
Here, we investigated whether individuals differ in their behavioural responses to heat at adulthood, and whether early acoustic and thermal experience contributes to this variation. We tested these hypotheses in adult male and female zebra finches from two replicate experiments, where individuals were prenatally exposed to heat-calls or control-calls in artificial incubators, and then reared under different postnatal nest temperatures. In addition to this experimental cohort (Cexp), we used a small unmanipulated control cohort (Ccont), to verify that inter-individual variation at adulthood also occurs when developmental conditions are not experimentally altered. Then, across two successive summers, we repeatedly recorded individual panting behaviour, microsite use and activity levels, along a gradient of air temperatures (25.8–37.6°C) in outdoor aviaries. We also simultaneously recorded the body temperature (Tb) of a small subgroup, while behaving freely in the aviaries. We predicted that (1) behavioural responses to heat consistently vary between individuals in both cohorts, and (2) that this variation partly arises from early acoustic and thermal experience (tested in Cexp). Specifically, we expected birds exposed to heat-calls and/or reared in hot nests to be better adapted to high temperatures and therefore to: i) pant less, ii) spend less time in cooler microsites, and iii) remain more active, particularly at high temperatures.
Materials and Methods
Experimental Subjects
We carried out the experiment at Deakin University, Geelong, Australia, during austral summers 2017–2018 and 2018–2019. In total, 214 wild-derived zebra finch adults (i.e., born in captivity but 10 to 12th generation descendants of wild individuals from Northern Victoria), were observed, while behaving freely in outdoor aviaries.
For the control cohort, Ccont, developmental conditions were not experimentally manipulated; individuals (N = 30 birds) were incubated and raised by their parents breeding in nest boxes in outdoor aviaries. Birds in the experimental cohorts, Cexp, were obtained from two playback experiments: one original experiment conducted in 2014 (Mariette and Buchanan, 2016) (N = 55 birds), and a replicate experiment conducted in 2017 (N = 129 birds). For both replicates, eggs were collected on laying day and replaced with dummy eggs. Freshly collected eggs were incubated in a main incubator at 37.5°C and 60% humidity. After 9 days, they were transferred to one of the two experimental incubators for the last 4–5 days of incubation. In each incubator, two speakers (Sennheiser HD439) externally connected to an amplifier (Digitech 18W) and an audio player (Zoom H4nSP) broadcast a playback of either contact calls (control) or heat-calls (treatment). Both call types are produced naturally by incubating parents, either when communicating with their partner or when experiencing heat, respectively. In addition, to allow normal stimulation of the auditory system, both playbacks also included whine calls, also produced by parents in the nest and characterised by a complex acoustic structure. The prenatal playbacks were played daily, from 9:30 to 18:30 (averaging 16 min of control- or heat- call per hour), until hatching. Eggs and sound cards were swapped daily between the two experimental incubators to prevent any incubator-specific effect. Further methodological details are provided in Mariette and Buchanan (2016).
Upon hatching, nestlings were identified (by clipping head down feathers) and returned to their parents or foster parents in nest boxes in outdoor aviaries. For the original experiment (Mariette and Buchanan, 2016), natural temperature variation caused by different sun exposure throughout the day resulted in a gradient of warm to hot nest-boxes [i.e., 3–6°C above air temperature during daytime (11:00–17:30)]. For the replicate experiment, nest temperatures were manipulated from hatching to 14 days post-hatch (dph) to obtain a larger gradient of temperatures (i.e., 1 to 8°C above daytime Tair). Specifically, we increased temperature in hot nests (N = 68) using a heat pad (Medi Heat Pack®) under the nest-box roof (when Tair < 30°C) and a second (Hotteeze®) at the back of the nest (when Tair < 25°C). We maintained some other nests cool (N = 54) using shading cloth, as well as a cool pad under the roof (when Tair > 25°C). For each brood, we calculated the average nest temperature above Tair (here shortened to “nest temperature differential,” Td–nest), between 2 and 14 dph, as the average difference between the maximal daily nest temperature and the maximal daily air temperature [Bureau of Meteorology data, details in Section “Air Temperature (Tair)”]. As in Mariette and Buchanan (2016), this nest temperature differential better represents the thermal microenvironment experienced during development, compared to raw nest temperatures.
Behavioural Observations
Each aviary included 3 top perches, at the front and back of the aviary, and a hanging feeder. The floor was made of concrete. Bird location was recorded as “perches” (i.e., perching on the perches or feeder) or “floor.” Both the perches and the floor below were in partial shade under a translucid fibreglass roof during the observations, but the floor was always at least 3.5°C cooler than the perches (see results). The perches and floor were therefore considered as two distinct microsites.
Individuals from Ccont were observed only during the first summer, and those from Cexp during both summers. Observations occurred on “hot days,” forecasted to exceed 26°C, corresponding to the minimum air temperature (i.e., minimum 29–32°C in the nest) at which heat-calls are produced by incubating parents in this population (Mariette and Buchanan, 2016). There were 10 days of observation in the first summer, between 18-Oct-2017 and 7-Feb-2018, and 9 days in the second summer, between 7-Dec-2018 and 2-Feb-2019. These observations were conducted during the hottest time of the day (between 11:00 and 17:00) when Tair exceeded 26°C (range: 25.8–37.6°C).
In total, we collected data during 40 “observation sessions” distributed across the 19 hot days, with 1 to 4 observation sessions per day. For each observation session, all aviaries were observed, in random order, for 10–20 min each. Within aviaries, we aimed to sight each individual once per session, in random order, to record their identity, behaviour, panting and location during 30 s of focal observation per bird. In total, we collected N = 2,654 individual observations (mean = 12.4 observations per individual), including N = 1743 and N = 911 individual observations in the first and second year respectively. Observations were made from 3 to 6 metres away, in a shaded corridor behind the aviary wire mesh, where birds are accustomed to human presence. Nonetheless, observations started after 15 minutes of habituation to the observer presence. Observers were blind to which treatment individual birds belonged.
For each individual observation, we recorded the bird identity, location, and behaviour (presence/absence) as follows: panting (bill open for > 5 s), perching (standing stationary on a perch), resting (crouching and/or closing eyes), hopping (short movements on or between close perches, or on the floor), flying (between front and back of the aviary, or to/from the floor), eating (pecking seeds in the feeder or on the floor), singing/calling (as indicated by sound, posture and throat movements). To obtain individual activity state (thereafter “activity”), behaviours were classified as either active (i.e., hopping, flying, eating or singing/calling) or inactive (perching or resting).
Predictor Variables for Temperature and Time During Observations
Air Temperature (Tair)
Atmospheric air temperature (Tair) every 30 min was provided by the Australian Bureau of Meteorology (BOM), from the Breakwater Geelong Racecourse station, 6.7 km from the aviaries (station number 87184, latitude: −38.1737, longitude: 144.3765, elevation above sea level: 12.9 m).
Microsite Temperature (Tloc)
We recorded the environmental temperature at the perches (Tperch) and 5 cm above the floor (Tfloor) using temperature data loggers (Minnow-1.0TH, Senonics), in six of the ten aviaries (placed in alternate aviaries due to the limited number of loggers). This environmental temperature allows estimating the conditions experienced by the birds, with the integration of Tair with wind, solar and reflecting radiation (Cunningham et al., 2021). Temperature recordings (total N = 5502 at one-minute intervals) occurred on three sunny days (30-Oct-2018; 7 and 12-Dec-2018) between 11:00 and 17:00, with one thermometer on the floor and two on perches in each of three aviaries per day.
Across thermometer deployment days, the difference between Tperch and Tair (i.e., BOM data) increased linearly with air temperature. We therefore use the equation from this linear regression of Tperch over Tair to obtain the Tperch experienced by birds at any one time during observation days. Likewise, as the temperature differential between Tperch and Tfloor decreased linearly with Tair, we used that linear regression to calculate the temperature differential during observations, and then subtracted it from Tperch (calculated above) to determine Tfloor.
Body Temperature (Tb)
A subset of individuals (N = 23) from Cexp were equipped with a passive integrated transponder (PIT) tag (Biomark, Boise, United States), implanted subcutaneously in the flank. For small birds (∼15 g), subcutaneous tags allow measuring the body temperature (Tb) as accurately as intra-peritoneal tags (Oswald et al., 2018a). In the aviaries, Tb was detected every 20 seconds by two PIT tag antennas (HPR plus, Biomark), placed next to the perches, during the six days of observations that occurred between 07-Dec-2018 and 17-Jan-2019 (N = 331 readings). We calculated the average Tb of each individual during the behavioural observation (using Tb values obtained within 5 min of each individual observation). Within sessions, there were 3.72 ± 0.44 Tb values on average per individual observation, and an individual Tb varied by 0.42 ± 0.07°C on average.
Time of Day
Because air temperature varied predictably throughout the day (Figure 1), to investigate time-of-day effects independently of Tair, we used time-of-day as a two-level variable, split at 2 pm when Tair typically plateaus. The two levels were thus: “midday” (11:00–14:00, ascending temperature phase, mean Tair = 32.38°C, N = 103 sessions), and “afternoon” (14:00–17:00, descending phase, 32.41°C, N = 79 sessions).
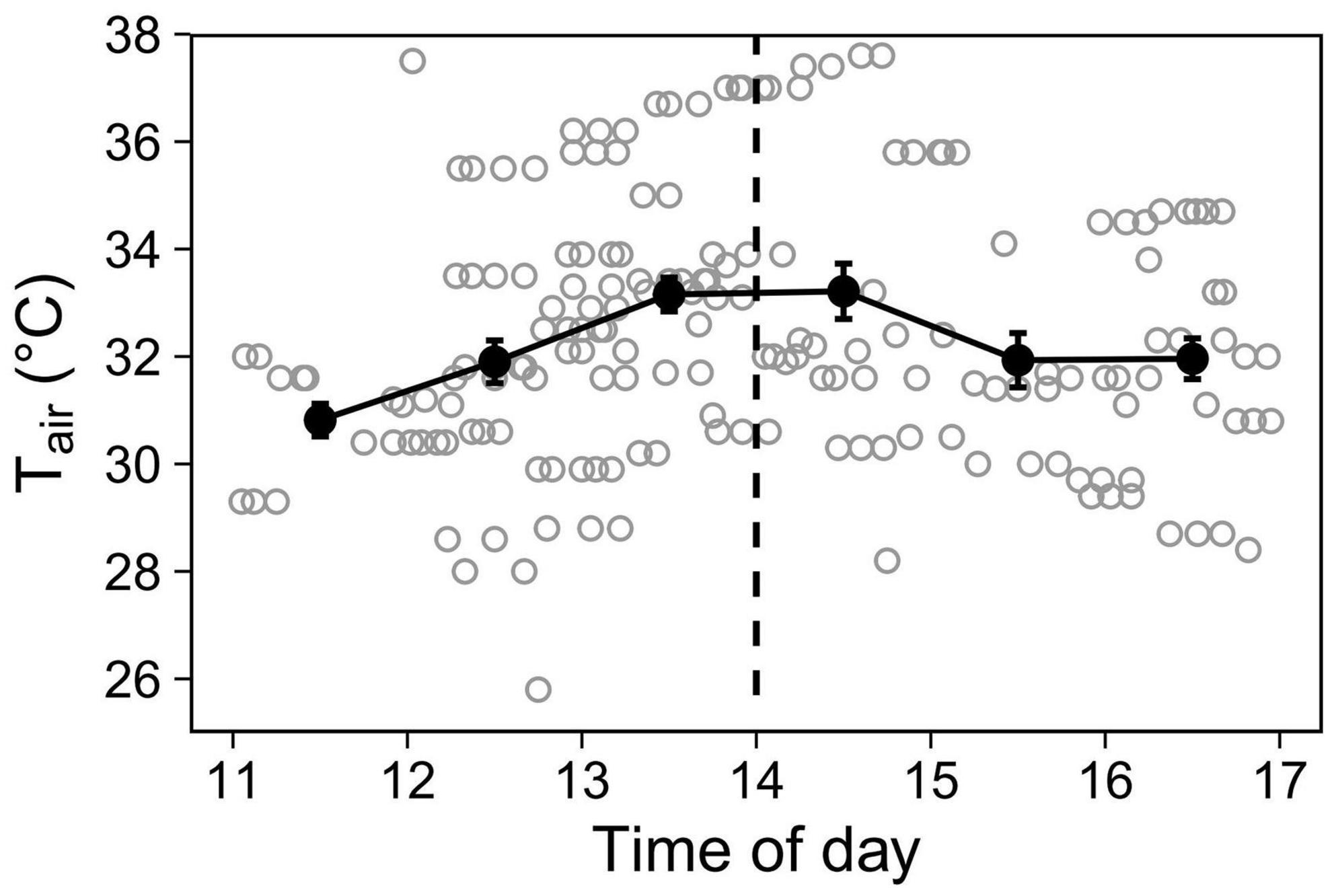
Figure 1. Air temperature (Tair) recorded during observations. Open grey circles represent Tair at the time each aviary observation started (N = 184). To display the overall trend, closed black circles with error bars show hourly means (±SE). The dashed line at 14 h delimitates the two levels (midday and afternoon) for the Time-of-day predictor.
Statistical Analyses
Analyses were performed separately on the unmanipulated cohort Ccont (without early-life predictors; N = 30 birds) on the one hand, and on the manipulated birds from Cexp (from the two replicate experiments; N = 184 birds) on the other hand, including playback and nest temperature as early-life predictors.
All statistical analyses were performed using R (version 4.0.1) via RStudio (RStudio Team, 2021). All models were fitted using the package lme4 (Bates et al., 2021) and continuous predictors were normalised using the scale function, to facilitate estimate interpretation. Full model outputs are presented in the Supplementary Material (Supplementary Tables 1–3). We obtained the reduced models following a backward stepwise procedure, removing non-significant fixed factors, starting with interactions, until only significant or marginal (p < 0.07) terms remained (Crawley, 2012). We then used the Akaike’s Information Criterion corrected for small sample sizes (AICc) to confirm the reduced models were the most parsimonious (i.e., lowest AICc).
Plasticity and Repeatability of Behaviour
The following analyses were restricted to individuals (N = 103) observed more than 10 times in total in Cexp, or more than 5 times in Ccont (N = 23), to ensure repeated observations per individual across the temperature range.
We tested whether panting response to microsite temperature at the cohort level was driven by between- and/or within-individual differences, following the method by van de Pol and Wright (2009) (used in e.g., Dingemanse et al., 2012; Hidalgo Aranzamendi et al., 2019). We used within-individual centering, to obtain two distinct predictors: between- and within-individual variation components. The “between-individual component” was calculated as the individual mean (i.e., average Tloc experienced by each individual across all its observations). The “within-individual component” was calculated, for each of an individual’s observations, as the deviation from this individual mean (by subtracting individuals’ average Tloc from each of their observed Tloc). We ran an initial binomial generalised linear mixed model (GLMM), fitting panting behaviour as the response variable, and both the between- and within-individual components calculated above as predictors. Then, to test whether between- and within-individual variation differed significantly from each other, we ran a second GLMM including panting as the response variable and, as fixed effects, the within-individual component calculated above, and a predictor for the difference between the between- and within-individual effects (actually corresponding to Tloc in this model) (van de Pol and Wright, 2009).
Then, we tested for between-individual differences in the intercept (i.e., elevation) and slopes (i.e., plasticity) of the reaction norm of panting response to Tloc (Dingemanse et al., 2010). We fitted random regression models, including panting as the response variable, and the fixed effects as described below in full models (i.e., Ccont: Tloc, time-of-day, and sex; Cexp: Tloc, time-of-day, sex, prenatal playback (heat-calls or control-calls), nest temperature differential (Td–nest), and playback by Tloc and playback by Td–nest interactions). We included a random intercept for date and, for bird identity (bird-ID), either a random intercept and slope, an intercept only, or no random effect. We used likelihood ratio tests (LRTs) to test the significance of the random terms. To test whether between-individual differences varied between the two playback groups, we also ran separate models for birds exposed to control- or heat-calls. Since not all individuals were observed in all sessions, there was variation in the number of observations per individual (Ccont: mean = 10.66, range = 6–17; Cexp: mean = 22.79, range = 11–47) and the range of temperatures at which each individual was observed (Ccont: min Tloc range = 2.8°C, max Tloc range = 10.71°C; Cexp: min range = 3.37°C; max range = 10.58°C). We used simulated datasets to demonstrate that this sampling design was not creating spurious inter-individual variation, nor masking variation (see results and Supplementary Material, Figure 1). As we found variation in intercept but not slope for bird identity (see section “Results”), all models below included date and bird-ID as random intercepts (unless otherwise specified).
For microsite use and activity, we also applied the within-individual centering method described above to test for between- and/or within-individual differences in microsite use across air temperature (Tair), and activity across Tloc.
Microsite Use
To test how the birds used the microsites, in Ccont, we ran binomial GLMMs with microsite (floor or perches) as a response variable and Tair, time-of-day and sex as fixed factors. For Cexp, we added as fixed factors the prenatal playback (heat-calls or control-calls), nest temperature differential (Td–nest) and the two-way interactions between playback and temperature during observation (Tair) or development (Td–nest).
Panting Behaviour and Activity
For each of the two cohorts, we ran two binomial GLMMs, with either panting or activity as a response variable. First, we assessed how panting or activity varied with microsite, Tair, and their interaction, in both cohorts. Then, because microsites differed in temperature (by 4.9°C on average), to test how birds responded to the actual temperature they were experiencing at their location in the aviary, we ran another two models per cohort (for panting and activity respectively) using Tloc instead of Tair. For Ccont, we used Tloc, time-of-day and sex as predictors. For Cexp, as above, we added as predictors the prenatal playback, Td–nest and the two-way interactions between playback and Tloc, playback and Td–nest, and, Td–nest and Tloc. Using a three-level variable for activity (resting, perching, active) in a Cumulative link mixed model [CLMM; package ordinal, (Christensen, 2019)] led to the same results as using the binomial GLMM; therefore only the latter is presented.
Body Temperature
To establish whether, at moderate microsite temperature (Tloc), panting is indicative of a lesser ability to maintain low Tb, or instead reflects a higher investment in respiratory evaporative water loss to prevent Tb rise, we assessed variation in Tb in a subset of birds equipped with thermosensitive PIT tags (N = 23). We ran a GLMM with Tb as a response variable, and Tloc, panting (absence/presence) and their interaction as predictors, including bird-ID as a random intercept and using a Gamma distribution and a log-link function. We did not include observation date (N = 6 days) as a random effect, due to extremely low variance. To allow meaningful interpretation, we restricted this analysis to when Tloc < minimal Tb (i.e., 40°C). Sample sizes were insufficient to also investigate Tb at Tloc > 40°C. Data was transformed by subtracting the minimal Tb in the dataset from all Tb values and adding a constant of 1 to keep only meaningful variation and obtaining positive values to fit the Gamma distribution.
Results
Plasticity and Repeatability of Behaviour
In both control (Ccont) and experimental (Cexp) cohorts, the panting response to microsite temperature (Tloc) was due to both phenotypic differences between individuals and behavioural flexibility within individuals. Specifically, some individuals consistently panted more than others (between-individual component, Table 1), and, each individual also panted more when Tloc increased (within-individual component, Table 1). The variation between individuals was significantly larger than that within, in Cexp, but not in Ccont (differences between variation components, Table 1).
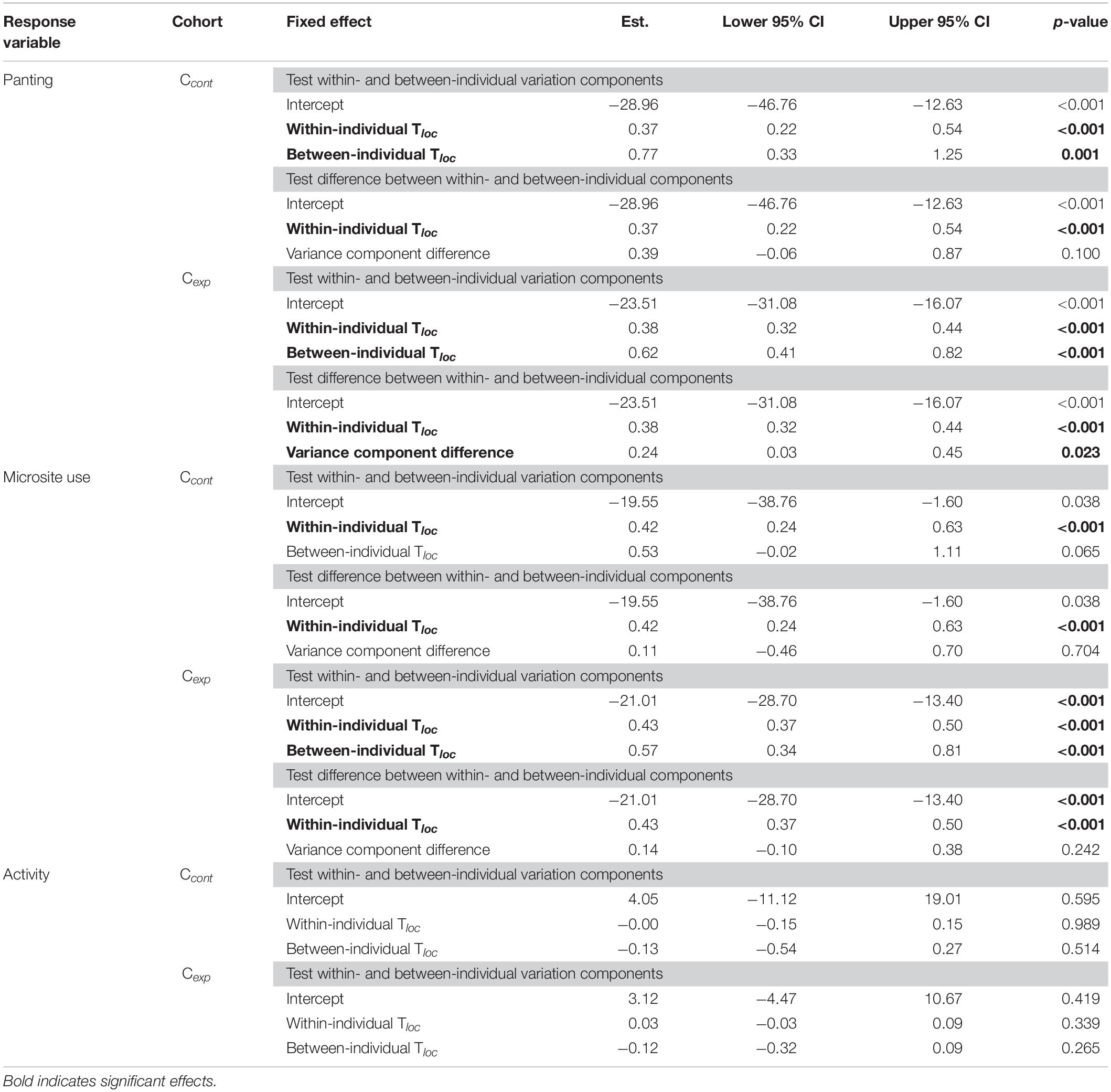
Table 1. Parameter estimates and 95% confidence intervals (CIs) of binomial generalised linear mixed models (GLMMs) using within-individual centering for panting behaviour, microsite use, and activity, in the control (Ccont; N = 226 observations for 23 birds observed > 5 times) and experimental cohorts (Cexp; N = 1,929 observations for 103 birds observed > 10 times).
Individuals differed significantly in the intercept of their panting response (i.e., elevation) but not in the reaction norm slope (Figure 2). This indicates that individuals varied in the temperature threshold at which they started panting, but generally not in how panting increased with temperature past that threshold. This was true in both Ccont (Figure 2A; LRTs: elevation, χ2 = 7.63, p = 0.006; slope: χ2 = 4.72, p = 0.094) and Cexp (LRTs: elevation, χ2 = 80.50, p < 0.001; slope: χ2 = 1.07, p = 0.586), including when control- and heat-call birds were considered separately: intercepts varied in both playback groups (LRTs: χ2 = 35.51, p < 0.001 and χ2 = 42.69, p < 0.001 respectively) but control-call birds were remarkably consistent in slope (Figure 2B, χ2 = 0.06, p = 0.968) while heat-call birds showed slightly more variation, even though non-significant (Figure 2B; χ2 = 2.57, p = 0.277). Our sample size and sampling design, including within playback groups, were nonetheless suitable to detect inter-individual variation in slope, had it been stronger (see simulations in Supplementary Material).
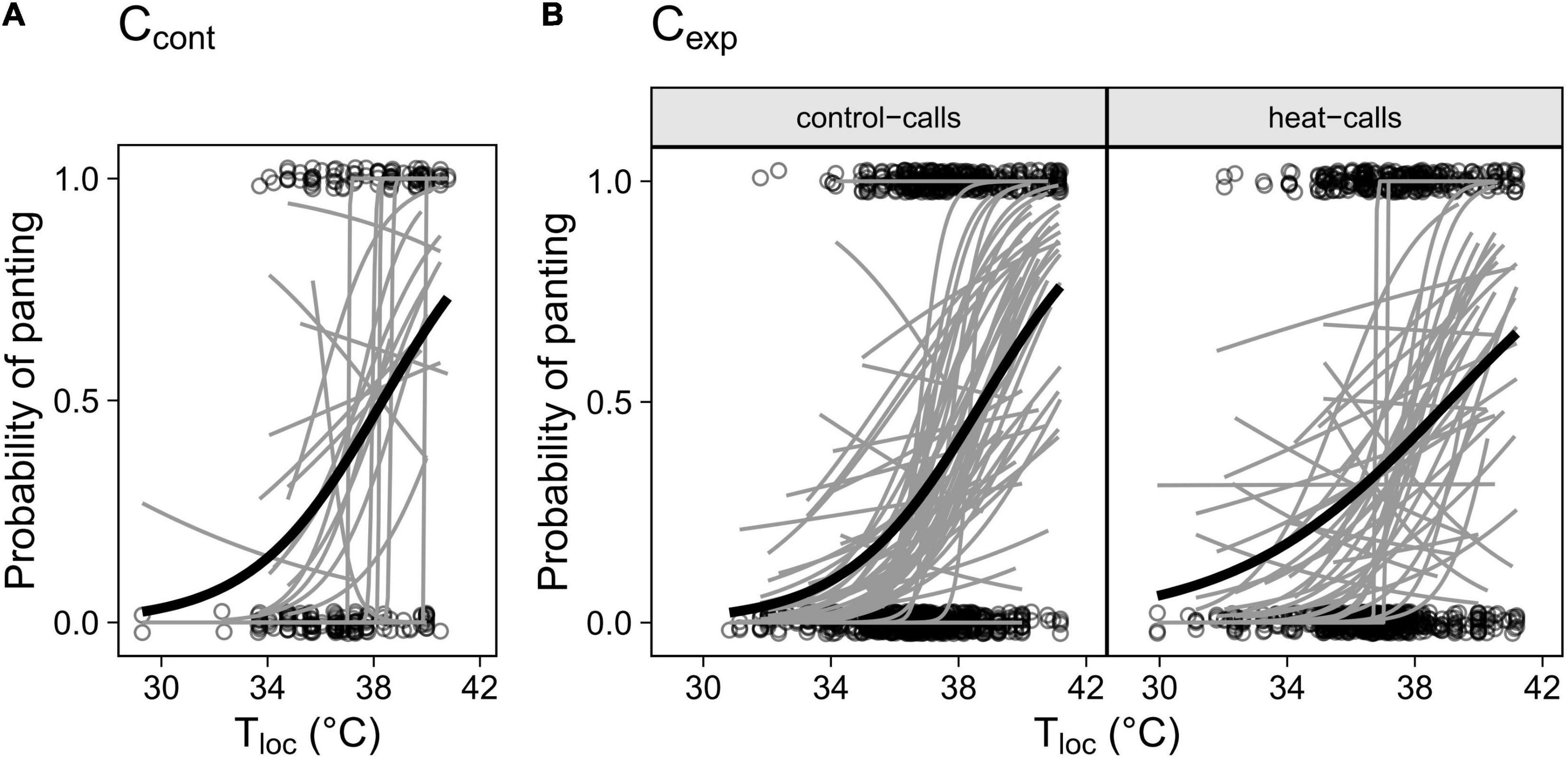
Figure 2. Individual panting responses to Tloc in (A) the control cohort (Ccont, N birds = 23) and (B) the experimental cohort (Cexp) for birds exposed prenatally to control-calls (N = 59) or heat-calls (N = 44). Only birds observed > 5 times (A) or > 10 times (B) are included, to increase reaction norm accuracy. Individual responses are represented by the grey regression lines and the group average response by the black line.
Similarly, for microsite use, variation in response to air temperature (Tair) was explained by the combination of inter-individual phenotypic differences (although only marginally in Ccont) and within-individual behavioural flexibility (Table 1). Unlike for panting response however, the within-individual variation was as large as that between individuals (i.e., difference between variation components, Table 1).
Lastly, for activity, there was no significant between- nor within-individual variation (Table 1), consistent with only a weak effect of Tloc (see below), in both Ccont and Cexp.
Microsite Use
During the observations, the floor was on average 4.9°C cooler than the perches (ranging from 3.6 to 5.8°C). In both cohorts, birds were more likely to be on the floor as Tair increased (Tables 2, 3 and Figure 3A). In addition, in the experimental cohort Cexp, the probability of being on the floor was higher at midday than in the afternoon, and in males (Table 3 and Figures 3B,C.
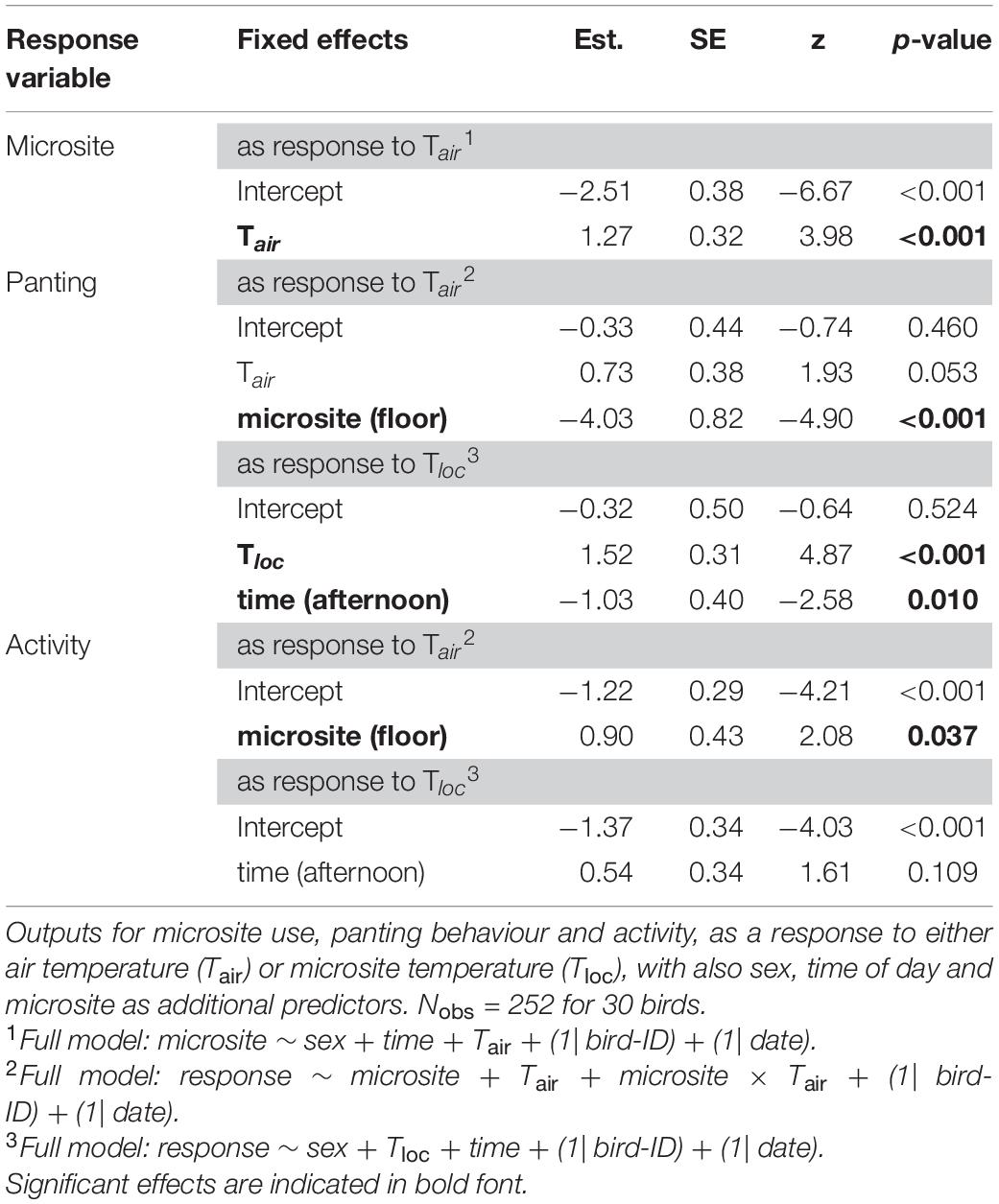
Table 2. Output of reduced binomial generalised linear mixed models (GLMMs) in the control cohort (Ccont), not subject to experimental procedures in early life.
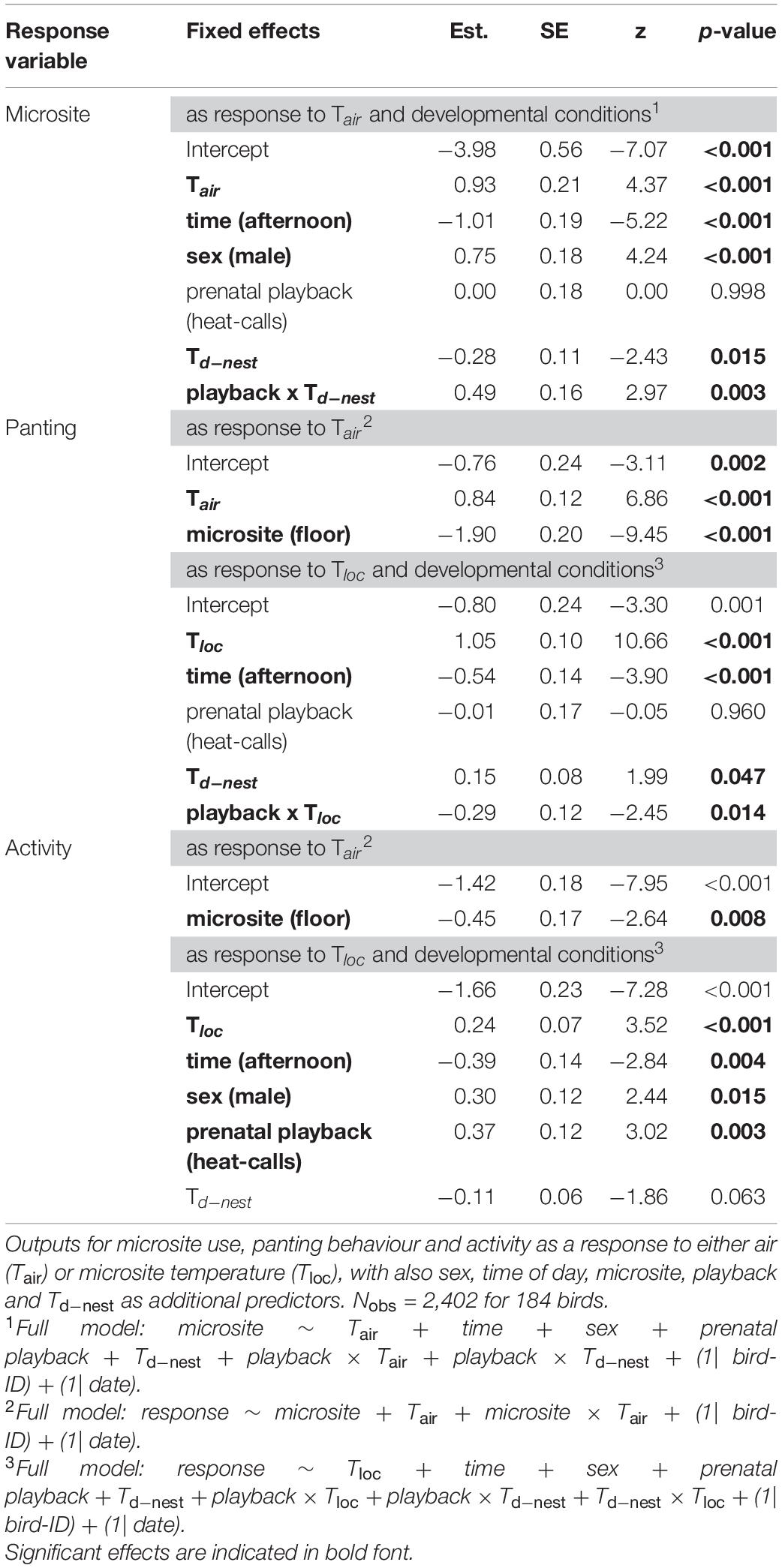
Table 3. Output of reduced binomial GLMMs in the experimental cohort (Cexp), subject to experimental manipulation of prenatal playback (control-calls or heat-calls) and postnatal nest temperature differential (Td–nest).
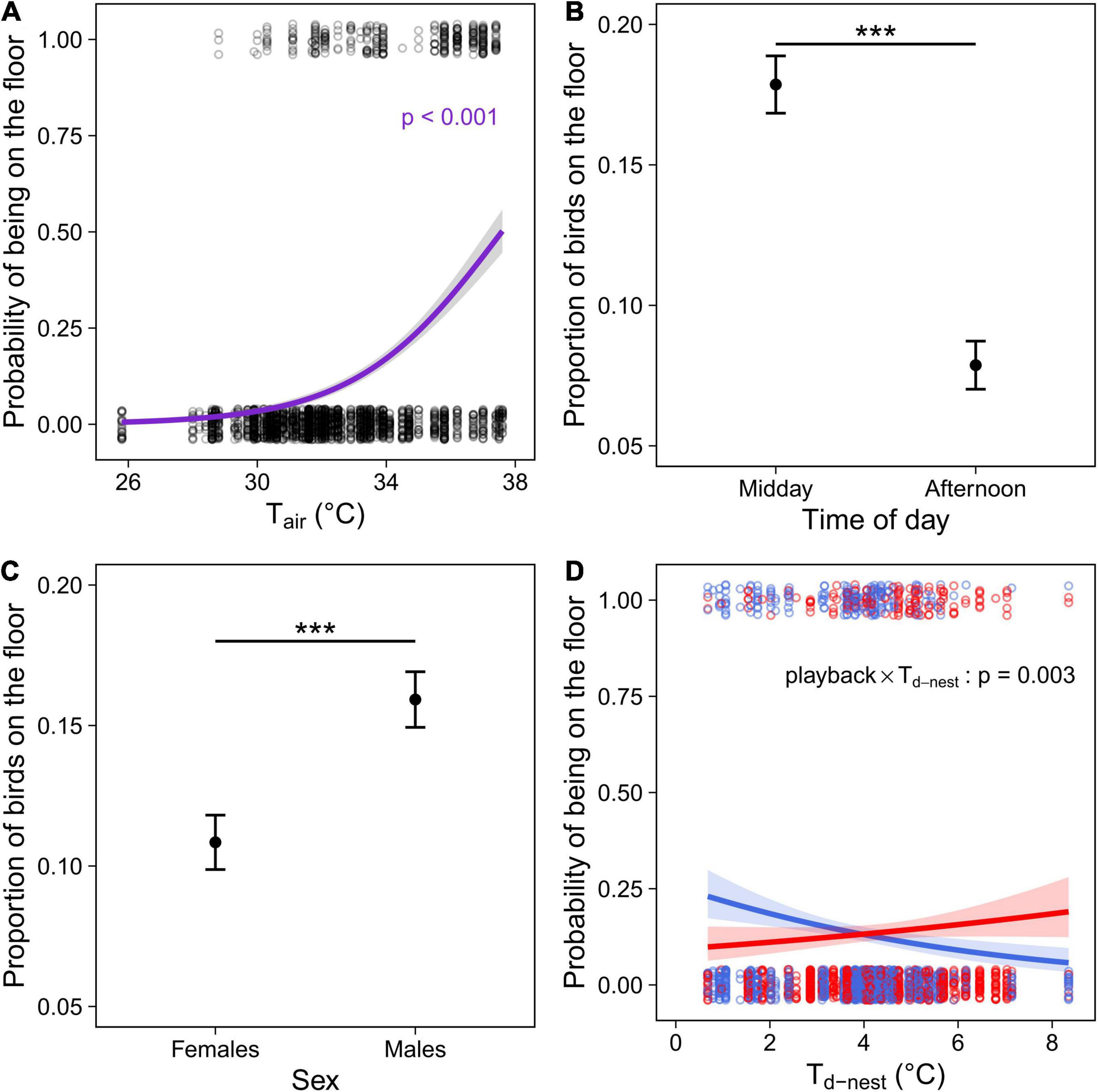
Figure 3. Microsite use (floor versus perches in outdoor aviary) in the experimental cohort (Cexp) comprising individuals that were exposed to a prenatal playback of either heat-calls or control-calls and reared under different nest temperature differentials (Td–nest; Nobs = 2402 for 184 birds). (A) Probability of birds being on the floor depending on the air temperature during observation (Tair); proportion of birds on the floor depending on (B) the time of day or (C) sex; and (D) probability of using the floor as a function of the thermal microenvironment experienced as a nestling (°C above air in the nest), in heat-call (red, N = 1055 observations for 84 birds) or control-call birds (blue, N = 1347 for 100 birds). Open circles show each observation, solid circles with error bars (B,C) represent the mean ± SE. Regression lines (A,D) are shown with 95% CIs. ***p < 0.001.
Early-life experience also affected microsite use. There was a significant interaction between the prenatal playback and nest temperature differential (Td–nest; Table 3 and Figure 3D): in control-call birds, as expected, individuals reared in cooler nests were more likely to use the floor than those from hotter nests. However, the opposite was true in birds exposed to prenatal heat-calls.
Panting Response
As expected given its thermoregulatory function, panting probability increased with environmental temperature. Panting increased with Tair [although p = 0.053 for Ccont: Table 2; Cexp: Table 3)], and was significantly higher on the perches than on the floor (Tables 2, 3 and Figure 4A). Correspondingly, the probability of panting increased with Tloc (i.e., temperature at the bird’s location) in both cohorts (Tables 2, 3). In addition, birds panted significantly more, earlier in the day (Tables 2, 3 and Figure 4B).
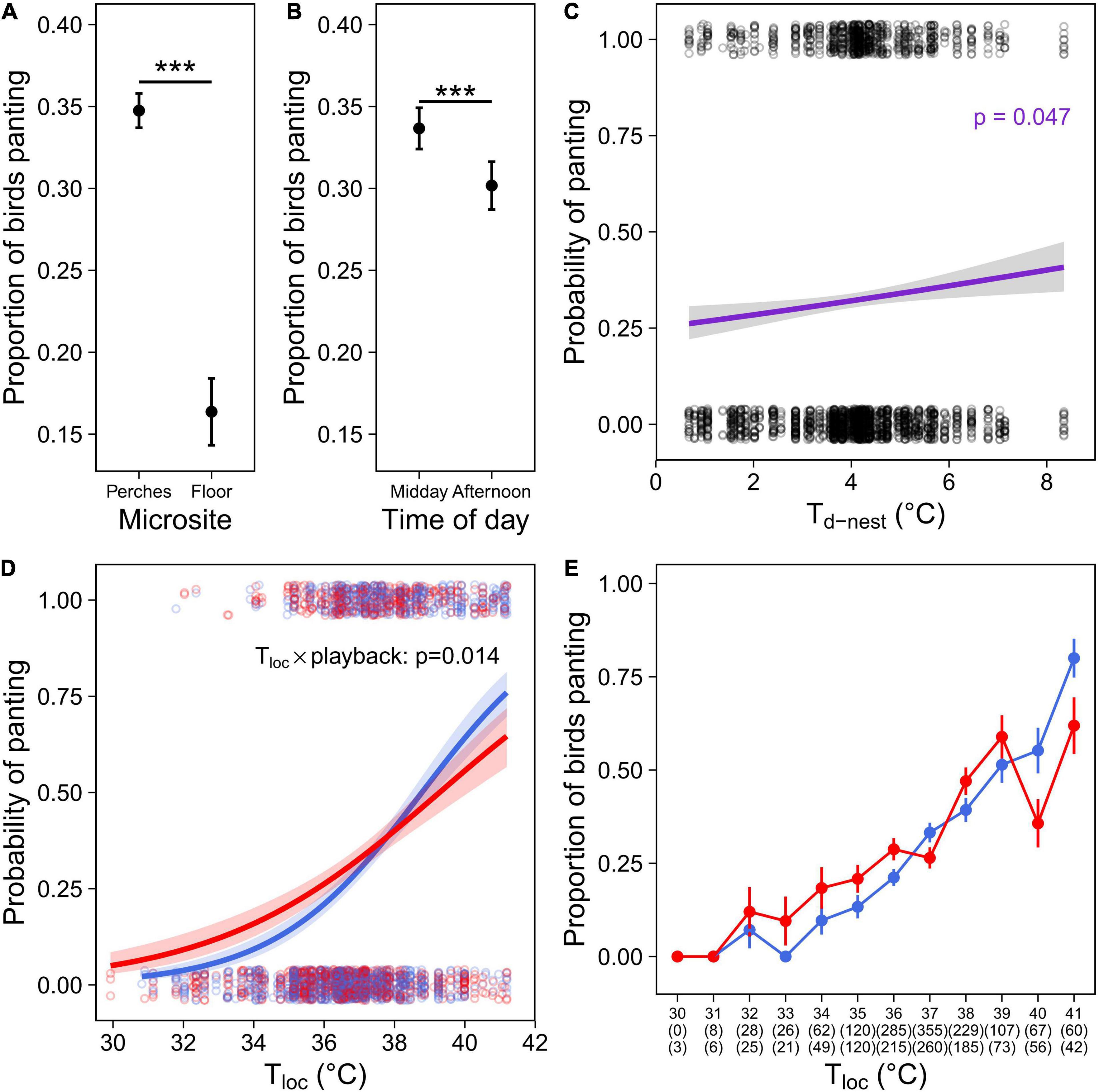
Figure 4. Panting behaviour in the experimental cohort (Cexp), comprising individuals that were exposed to a prenatal playback of either heat-calls or control-calls and reared under different nest temperature differentials (Td–nest; Nobs = 2,402 for 184 birds). Proportion of birds panting depending on (A) microsite use; or (B) the time of day. (C) Probability of panting as function of the thermal microenvironment experienced as a nestling (°C above air in the nest). Panting response to Tloc as: (D) Probability of panting over Tloc or (E) proportion of birds panting (mean per one-degree class ± SE) over Tloc, in heat-calls (red, N = 1,055) or control-calls birds (blue, N = 1,347). For panel (D), the interaction between prenatal playback and Tloc remains significant without the three observations at 30°C (p = 0.0013). For panel (E), numbers in brackets indicate sample sizes (i.e., number of birds observed) per temperature class and playback group: control-calls (top row) or heat-calls (bottom row). Open circles show each observation, solid circles with error bars represent means ± SE. Regression lines are shown with 95% CIs. ***p < 0.001.
Regarding early-life conditions, panting probability increased slightly but significantly with Td–nest (Table 3 and Figure 4C), but there was no interaction between Td–nest and Tloc (Supplementary Table 2). Additionally, there was a significant interaction between the prenatal playback and Tloc (Table 3 and Figure 4D): panting probability increased with Tloc in both playback groups; however, this increase was sharper in control-call birds, as heat-call birds started panting at lower Tloc but panted less than controls at high Tloc. This effect was robust, as it was also visible in the raw data when panting observations were divided up into temperature categories (Figure 4E). This difference between playback groups was not merely explained by their differential microsite use (reported above), since this interaction remained significant when birds on the floor were excluded (p = 0.006, Supplementary Table 4).
At environmental temperature below normothermic body temperature (i.e., Tloc < 40°C), body temperature (in a subset of birds equipped with PIT tags) increased significantly with Tloc, in both panting and non-panting individuals (Table 4 and Figure 5). Tb was nonetheless not higher in panting than non-panting birds (Tb = 41.39°C versus 41.42°C respectively, Figure 5). This suggests that, at Tloc < 40°C, higher panting activity was not indicative of a lesser ability to maintain normothermic Tb.
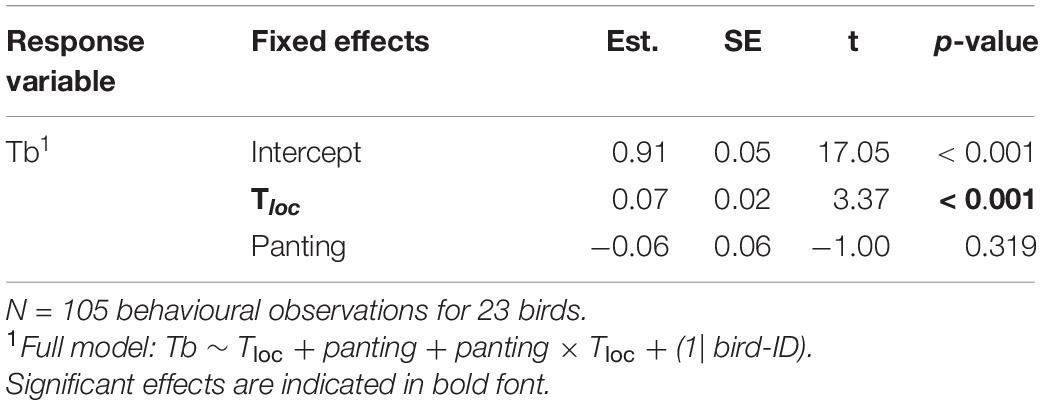
Table 4. Output of main effects from a GLMM in a subset of birds equipped with PIT tags, to measure body temperature (Tb), as function of panting behaviour and microsite temperature (Tloc), up to Tloc = 40°C.
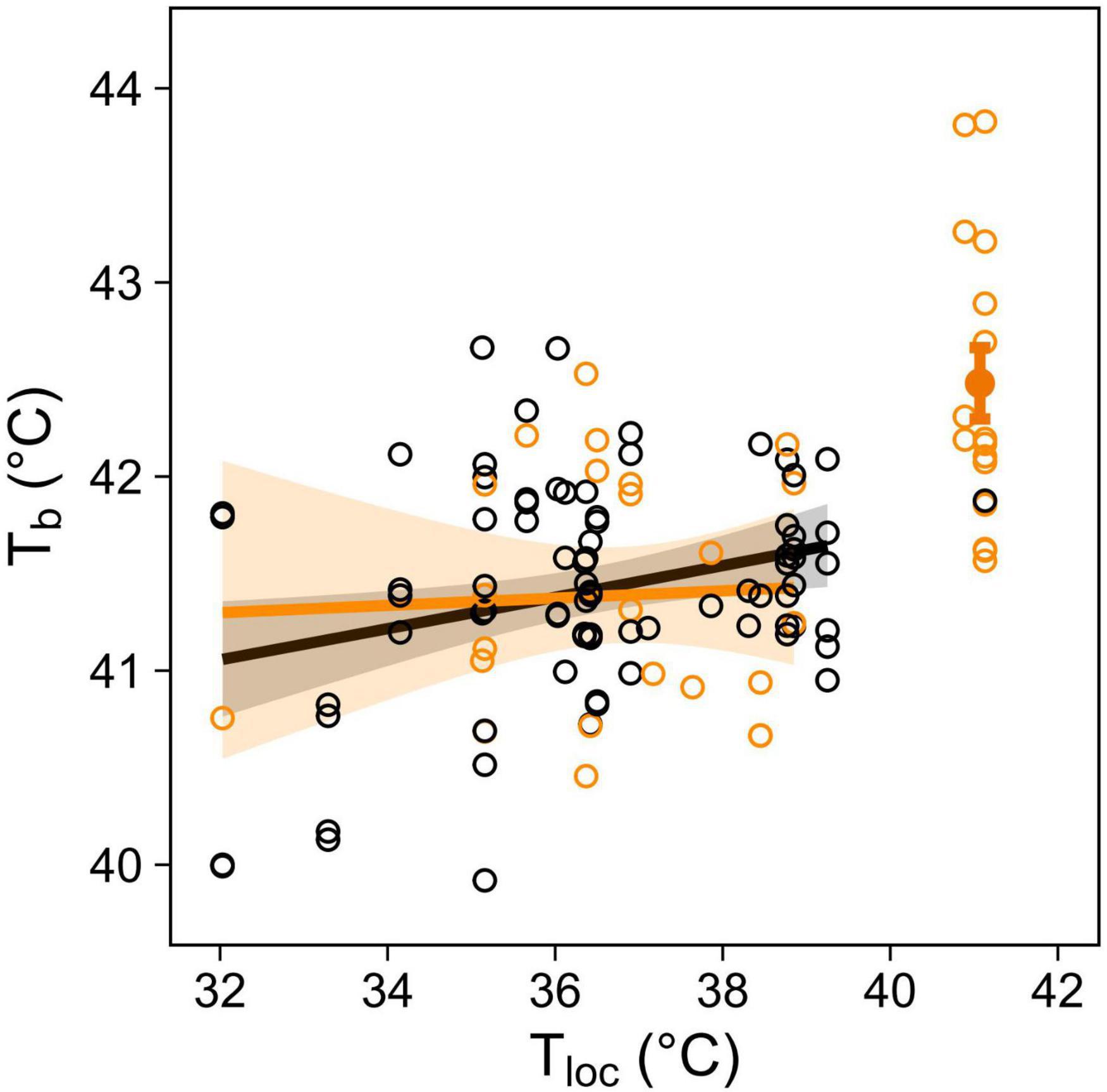
Figure 5. Body temperature (Tb) in response to microsite temperature (Tloc) and panting behaviour (not panting: black, panting: orange) in 23 birds equipped with PIT tags. Open circles show individual mean Tb during each observation session (N = 331 Tb recordings for 122 behavioural observations). Regression lines up to Tloc = 40°C are shown with 95% CIs, and solid circle with error bars at 41°C shows mean Tb (±SE) of panting birds.
Activity
In Cexp, birds were significantly less active on the floor than on the perches (Table 3; N = 2402 observations), although the reverse was true in the smaller sample of Ccont (Table 2; N = 252 observations). Birds in Cexp were also more active at midday than in the afternoon (Table 3) and, surprisingly, they were slightly but significantly more active at higher Tloc (Table 3). Additionally, males were more active than females in Cexp (Table 3).
Furthermore, in Cexp, birds exposed to prenatal heat-calls were more active than those exposed to control-calls (Table 3 and Figure 6A), and so across the temperature gradient (Table 3 and Figure 6B). Lastly, birds reared in hotter nests were marginally less active than those reared in cooler nests (p = 0.063 in reduced model: Table 3; p = 0.018 in full model: Supplementary Table 2).
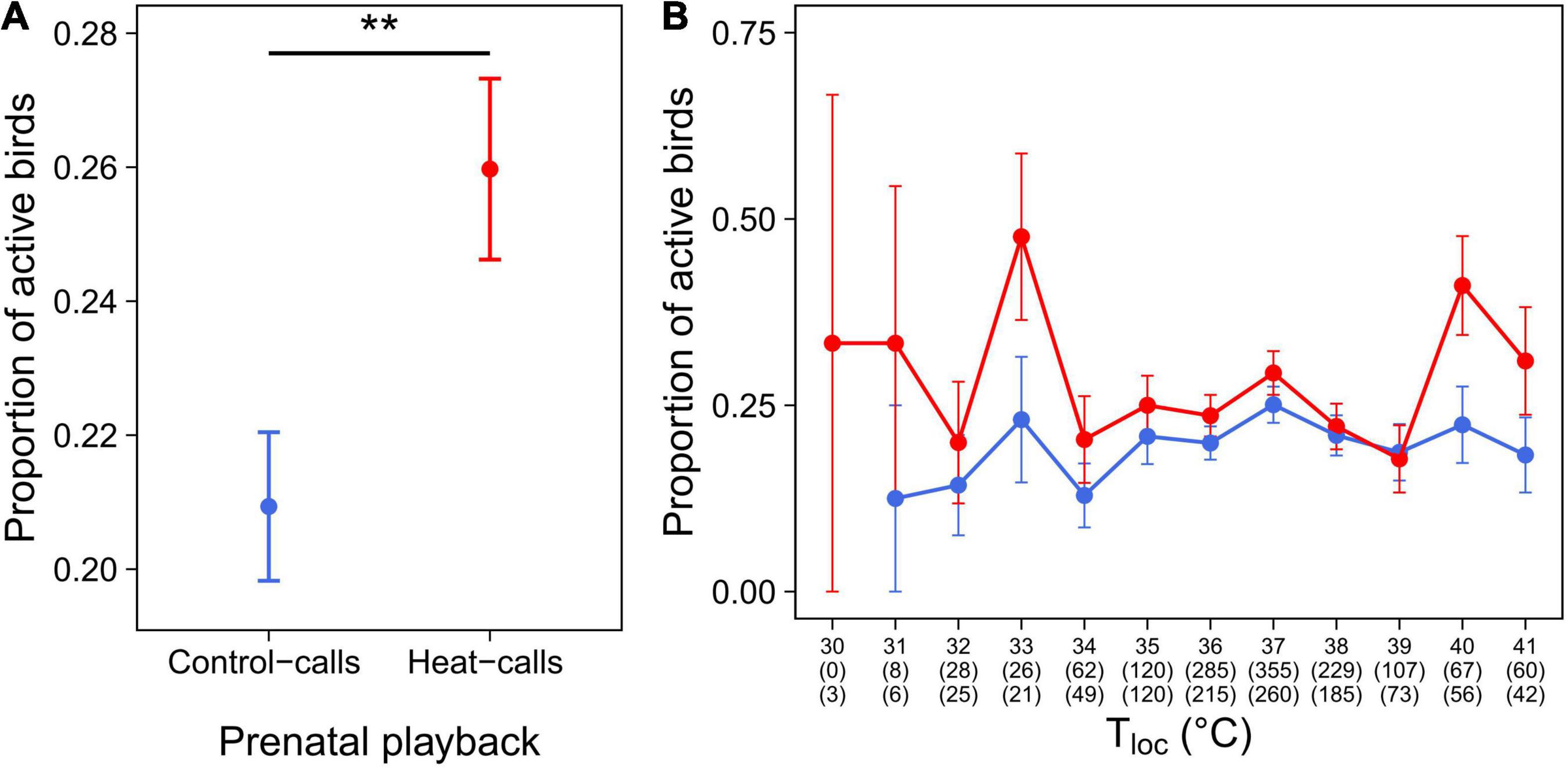
Figure 6. Activity level (mean proportion ± SE) in Cexp birds, exposed to prenatal control-calls (blue, N = 100) or heat-calls (red, N = 84). (A) Mean activity level per playback group and, (B) (for illustration purposes) mean activity level per one-degree class and playback group. In panel (B), numbers in brackets indicate the number of observations for control-call birds (top number) and heat-call birds (bottom number). **p < 0.01.
Discussion
We showed for the first time in endotherms that inter-individual variation in behavioural thermoregulation at adulthood can originate from early-life experience. Indeed, individuals consistently varied in their thermoregulation strategy, with some individuals starting panting at lower air temperature than others (i.e., differences in intercept). Prenatal acoustic experience affected panting, as well as both other behavioural responses tested. Namely, birds exposed to heat-calls started panting at lower temperatures than control birds, but panted less at high temperatures, suggesting different heat-regulation strategies. We propose that such strategy in heat-call birds may improve water saving at high temperature extremes and/or allow maintaining high activity levels, since heat-call birds were also more active than controls, across the gradient of summer temperatures. Lastly, effects on microsite use were more complex, as it varied with the interaction of early acoustic and thermal experiences. Overall, our study reveals that a prenatal acoustic signal of heat can shape how individuals adjust behaviourally to thermal challenges at adulthood. This, in turn, has the potential to alter the selection pressures individuals are exposed to, and thereby the fitness components affected by high temperatures.
Early-life conditions, and most remarkably, prenatal acoustic experience, affected individual behavioural responses to heat on the long-term, with potential fitness consequences. Heat-call birds panted less at high temperatures (Tloc), but more in cooler conditions, compared to control-call birds. Given the significant water loss associated with panting, moderating panting may correspond to a water-saving strategy. In arid environments, where birds may need to fly several kilometres to scarcely distributed drinking water, saving water is likely most crucial at very high temperatures, when flight may lead to excessive metabolic heat production. When environmental temperature rises to the level of normothermic body temperature (c.a. 40°C), small passerines often use hyperthermia (i.e., let Tb rise above normothermia) to save water and energy by reducing investment in thermoregulation (Tieleman and Williams, 1999; Gerson et al., 2019). It is therefore possible that heat-call individuals rely more on hyperthermia at high environmental temperature (>40°C) when water may be less readily accessible (in the wild), but pant more in less extreme conditions, when panting does not incur severe dehydration or hyperthermia risk. Importantly, body temperature was not higher in panting than non-panting birds, which suggests that panting intensity, rather than a sign of higher heat-stress, may reflect differential thermoregulation strategies, in which the reliance on respiratory evaporative water loss versus alternative thermoregulatory pathways varies. The benefits of higher panting activity, also observed overall in birds from hotter nests, remain to be established. However, they may include reducing heat-load accumulation, or maintaining activity. Indeed, heat-call birds remained more active across the range of warm to high temperatures. If the activity levels we measured in our experiment reflect individual ability to maintain foraging and breeding activity in the heat, heat-call exposure may increase reproductive success in summer conditions, as previously demonstrated (Mariette and Buchanan, 2016). Overall, our results suggest that prenatal heat-call exposure did not reduce overall thermoregulatory needs, but instead altered individual panting strategy to better balance water loss and/or maintain higher activity levels. Our findings thus shed light on how acoustic developmental programming may impact life-long fitness by shaping individual behavioural responses to hot conditions.
As expected, and documented in other species (Smit et al., 2013; Cunningham et al., 2015; Pattinson and Smit, 2017; Xie et al., 2017; Oswald et al., 2019), panting and use of cooler microsites (here, the floor) increased with temperature (measured as Tair or Tloc). In addition, we found that, after controlling for microsite temperature, birds panted more, earlier in the day. Such diurnal patterns have also been observed in other species for panting (Grant, 1982; Smit et al., 2013) as well as for heat-calling in zebra finches [associated with higher water loss; (Mariette et al., 2018; Pessato et al., 2020)]. Nonetheless, we also showed that cooler microsite use was higher earlier in the day, which to our knowledge had not been shown elsewhere. Higher panting might occur in anticipation of air temperature peaking in late afternoon, or to sustain higher activity, which was also higher than in the afternoon.
Importantly, our aviary observations seem to mirror expected behavioural patterns in wild birds. Indeed, birds on the floor were less active than individuals remaining on perches (at least in the experimental cohort), similarly to studies in free living birds where individuals either cease activity and seek thermal refuges, or remain active in exposed sites (Smit et al., 2016; Pattinson and Smit, 2017). Interestingly, birds on the floor were also half as likely to pant as those on the perches (16 versus 35% respectively). This points to the occurrence of two alternative behavioural strategies where individuals save water and energy by reducing heat production (activity) and exposure to heat (microsite), or maintain normal behavioural activity at the cost of higher thermoregulatory needs, and thus higher energy and water expenditure. That both phenotypes were expressed even though birds had access to ad libitum food and water in the aviaries is interesting. This might indicate that behavioural responses are not as flexible as commonly assumed, perhaps as a consequence of being dictated by physiological capacities. This is in agreement with our findings that individuals consistently varied in their panting responses [and specifically in their panting threshold (estimated by random intercept)], and that such response was partly determined by early-life effects.
In conclusion, our study shows that early-life conditions have long-term effects on behavioural thermoregulatory strategies, contributing therefore to consistent inter-individual differences at adulthood. This study also reveals the role of prenatal sound in shaping individuals’ behavioural responses to thermal changes. These responses likely reflect differential trade-off modulation between thermoregulation, water balance and activity, and may explain long-lasting positive effects of heat-call exposure on reproductive fitness. Overall, our findings bring us closer to understanding how behavioural flexibility alters selection pressures by high temperatures, which is essential to predict the impact of anthropogenic climate change on species persistence.
Data Availability Statement
The original contributions presented in the study are included in the article/Supplementary Material, further inquiries can be directed to the corresponding author.
Ethics Statement
The animal study was reviewed and approved by Deakin University Animal Ethics Committee (project G23-2018).
Author Contributions
EU and MM designed the study, and wrote the manuscript. MM conducted the prenatal playback experiments. EU collected, processed and analysed behavioural data with help from MM. Both authors contributed to the article and approved the submitted version.
Funding
This work was supported by the Australian Research Council grants DE170100824 to MM and DP180101207 to MM and Katherine L. Buchanan.
Conflict of Interest
The authors declare that the research was conducted in the absence of any commercial or financial relationships that could be construed as a potential conflict of interest.
Publisher’s Note
All claims expressed in this article are solely those of the authors and do not necessarily represent those of their affiliated organizations, or those of the publisher, the editors and the reviewers. Any product that may be evaluated in this article, or claim that may be made by its manufacturer, is not guaranteed or endorsed by the publisher.
Acknowledgments
We thank Rod Collins, Bruce Newell, and the Deakin Animal House staff for their logistical support, and Ineke Goedegebuur, Andrew Katsis, and Naomi Well for their participation in aviary work. We also thank two reviewers for their very helpful comments.
Supplementary Material
The Supplementary Material for this article can be found online at: https://www.frontiersin.org/articles/10.3389/fevo.2022.818278/full#supplementary-material
References
Albright, T. P., Mutiibwa, D., Gerson, A. R., Smith, E. K., Talbot, W. A., O’Neill, J. J., et al. (2017). Mapping evaporative water loss in desert passerines reveals an expanding threat of lethal dehydration. Proc. Natl. Acad. Sci. U. S. A. 114, 2283–2288. doi: 10.1073/pnas.1613625114
Bates, D., Maechler, M., Bolker, B., Walker, S., Christensen, R. H. B., Singmann, H., et al. (2021). lme4: linear Mixed-Effects Models using “Eigen” and S4. Available online at: https://CRAN.R-project.org/package=lme4 (accessed December 19, 2021).
Calder, W. A., and King, J. R. (1974). Thermal and caloric relations of birds. Avian Biol. 4, 259–413.
Christensen, R. H. B. (2019). ordinal: regression Models for Ordinal Data. Available online at: https://CRAN.R-project.org/package=ordinal (accessed December 19, 2021).
Conradie, S. R., Woodborne, S. M., Cunningham, S. J., and McKechnie, A. E. (2019). Chronic, sublethal effects of high temperatures will cause severe declines in southern African arid-zone birds during the 21st century. Proc. Natl. Acad. Sci. U. S. A. 116:201821312. doi: 10.1073/pnas.1821312116
Conradie, S. R., Woodborne, S. M., Wolf, B. O., Pessato, A., Mariette, M. M., and McKechnie, A. E. (2020). Avian mortality risk during heat waves will increase greatly in arid Australia during the 21st century. Conserv. Physiol. 8, 1–14. doi: 10.1093/conphys/coaa048
Cook, T. R., Martin, R., Roberts, J., Häkkinen, H., Botha, P., Meyer, C., et al. (2020). Parenting in a warming world: thermoregulatory responses to heat stress in an endangered seabird. Conserv. Physiol. 8, 1–13. doi: 10.1093/conphys/coz109
Cunningham, S. J., Gardner, J. L., and Martin, R. O. (2021). Opportunity costs and the response of birds and mammals to climate warming. Front. Ecol. Environ. 19, 300–307. doi: 10.1002/fee.2324
Cunningham, S. J., Martin, R. O., and Hockey, P. A. (2015). Can behaviour buffer the impacts of climate change on an arid-zone bird? Ostrich 86, 119–126. doi: 10.2989/00306525.2015.1016469
Cunningham, S. J., Thompson, M. L., and McKechnie, A. E. (2017). It’s cool to be dominant: social status alters short-term risks of heat stress. J. Exp. Biol. 220, 1558–1562. doi: 10.1242/jeb.152793
Dingemanse, N. J., Bouwman, K. M., van de Pol, M., van Overveld, T., Patrick, S. C., Matthysen, E., et al. (2012). Variation in personality and behavioural plasticity across four populations of the great tit Parus major. J. Anim. Ecol. 81, 116–126. doi: 10.1111/j.1365-2656.2011.01877.x
Dingemanse, N. J., Kazem, A. J. N., Réale, D., and Wright, J. (2010). Behavioural reaction norms: animal personality meets individual plasticity. Trends Ecol. Evol. 25, 81–89. doi: 10.1016/j.tree.2009.07.013
Dirzo, R., Young, H. S., Galetti, M., Ceballos, G., Isaac, N. J. B., and Collen, B. (2014). Defaunation in the Anthropocene. Science 345, 401–406. doi: 10.1126/science.1251817
du Plessis, K. L., Martin, R. O., Hockey, P. A. R., Cunningham, S. J., and Ridley, A. R. (2012). The costs of keeping cool in a warming world: implications of high temperatures for foraging, thermoregulation and body condition of an arid-zone bird. Global Change Biol. 18, 3063–3070. doi: 10.1111/j.1365-2486.2012.02778.x
Durant, S. E., Hopkins, W. A., Hepp, G. R., and Walters, J. R. (2013). Ecological, evolutionary, and conservation implications of incubation temperature-dependent phenotypes in birds. Biol. Rev. 88, 499–509. doi: 10.1111/brv.12015
Gerson, A. R., McKechnie, A. E., Smit, B., Whitfield, M. C., Smith, E. K., Talbot, W. A., et al. (2019). The functional significance of facultative hyperthermia varies with body size and phylogeny in birds. Funct. Ecol. 33, 597–607. doi: 10.1111/1365-2435.13274
Grant, G. S. (1982). Avian Incubation: egg Temperature, Nest Humidity, and Behavioral Thermoregulation in a Hot Environment. Ornithol. Monogr. 30, iii-ix, 1–75. doi: 10.2307/40166669
Groothuis, T. G. G., Hsu, B. Y., Kumar, N., and Tschirren, B. (2019). Revisiting mechanisms and functions of prenatal hormone-mediated maternal effects using avian species as a model. Philos. Transac. R. Soc. B Biol. Sci. 374:20180115. doi: 10.1098/rstb.2018.0115
Hidalgo Aranzamendi, N., Hall, M. L., Kingma, S. A., van de Pol, M., and Peters, A. (2019). Rapid plastic breeding response to rain matches peak prey abundance in a tropical savanna bird. J. Anim. Ecol. 88, 1799–1811. doi: 10.1111/1365-2656.13068
IPCC (2014). “Climate Change 2014: synthesis Report” in Contribution of Working Groups I, II and III to the Fifth Assessment Report of the Intergovernmental Panel on Climate Change. eds R. K. Pachauri and L. A. Meyer (Geneva: IPCC).
Mariette, M. M., and Buchanan, K. L. (2016). Prenatal acoustic communication programs offspring for high posthatching temperatures in a songbird. Science 353, 812–814. doi: 10.1126/science.aaf7049
Mariette, M. M., Clayton, D. F., and Buchanan, K. L. (2021). Acoustic developmental programming: a mechanistic and evolutionary framework. Trends Ecol. Evol. 36, 722–736. doi: 10.1016/j.tree.2021.04.007
Mariette, M. M. M., Pessato, A., Buttemer, W. A. W. A., McKechnie, A. E. A. E., Udino, E., Collins, R. N. R. N., et al. (2018). Parent-embryo acoustic communication: a specialised heat vocalisation allowing embryonic eavesdropping. Sci. Rep. 8:17721. doi: 10.1038/s41598-018-35853-y
Martin, R. O., Cunningham, S. J., and Hockey, P. A. (2015). Elevated temperatures drive fine-scale patterns of habitat use in a savanna bird community. Ostrich 86, 127–135. doi: 10.2989/00306525.2015.1029031
McKechnie, A. E., Hockey, P. A. R., and Wolf, B. O. (2012). Feeling the heat: australian landbirds and climate change. Emu - Austr. Ornithol. 112, i–vii. doi: 10.1071/MUv112n2_ED
McKechnie, A. E., Rushworth, I. A., Myburgh, F., and Cunningham, S. J. (2021). Mortality among birds and bats during an extreme heat event in eastern South Africa. Austral Ecol. 46, aec.13025. doi: 10.1111/aec.13025
McKechnie, A. E., and Wolf, B. O. (2010). Climate change increases the likelihood of catastrophic avian mortality events during extreme heat waves. Biol. Lett. 6, 253–256. doi: 10.1098/rsbl.2009.0702
McKechnie, A. E., and Wolf, B. O. (2019). The Physiology of Heat Tolerance in Small Endotherms. Physiology 34, 302–313. doi: 10.1152/physiol.00011.2019
Nilsson, J. -Å, and Nord, A. (2018). Testing the heat dissipation limit theory in a breeding passerine. Proc. R. Soc. B Biol. Sci. 285:20180652. doi: 10.1098/rspb.2018.0652
Noakes, M. J., and McKechnie, A. E. (2019). Seasonal Metabolic Acclimatization Varies in Direction and Magnitude among Years in Two Arid-Zone Passerines. Physiol. Biochem. Zool. 93, 140–152. doi: 10.1086/707679
Noakes, M. J., Wolf, B. O., and McKechnie, A. E. (2016a). Seasonal and geographical variation in heat tolerance and evaporative cooling capacity in a passerine bird. J. Exp. Biol. 219, 859–869. doi: 10.1242/jeb.132001
Noakes, M. J., Wolf, B. O., and McKechnie, A. E. (2016b). Seasonal Metabolic Acclimatization Varies in Direction and Magnitude among Populations of an Afrotropical Passerine Bird. Physiol. Biochem. Zool. 90, 178–189. doi: 10.1086/689030
Noguera, J. C., and Velando, A. (2019). Bird embryos perceive vibratory cues of predation risk from clutch mates. Nat. Ecol. Evol. 3, 1225–1232. doi: 10.1038/s41559-019-0929-8
Oswald, K. N., Evlambiou, A. A., Ribeiro, Â. M., and Smit, B. (2018a). Tag location and risk assessment for passive integrated transponder-tagging passerines. IBIS 160, 453–457. doi: 10.1111/ibi.12558
Oswald, K. N., Lee, A. T. K., and Smit, B. (2018b). Seasonal physiological responses to heat in an alpine range-restricted bird: the Cape Rockjumper (Chaetops frenatus). J. Ornithol. 159, 1063–1072. doi: 10.1007/s10336-018-1582-8
Oswald, K. N., Smit, B., Lee, A. T. K., and Cunningham, S. J. (2019). Behaviour of an alpine range-restricted species is described by interactions between microsite use and temperature. Ani. Behav. 157, 177–187. doi: 10.1016/j.anbehav.2019.09.006
Oswald, K. N., Smit, B., Lee, A. T. K., Peng, C. L., Brock, C., and Cunningham, S. J. (2021). Higher temperatures are associated with reduced nestling body condition in a range-restricted mountain bird. J. Avian Biol. 52:e02756. doi: 10.1111/jav.02756
Pattinson, N. B., and Smit, B. (2017). Seasonal behavioral responses of an arid-zone passerine in a hot environment. Physiol. Behav. 179, 268–275. doi: 10.1016/j.physbeh.2017.06.018
Pattinson, N. B., Thompson, M. L., Griego, M., Russell, G., Mitchell, N. J., Martin, R. O., et al. (2020). Heat dissipation behaviour of birds in seasonally hot arid-zones: are there global patterns? J. Avian Biol. 51, 1–11. doi: 10.1111/jav.02350
Pessato, A., McKechnie, A. E., Buchanan, K. L., and Mariette, M. M. (2020). Vocal panting: a novel thermoregulatory mechanism for enhancing heat tolerance in a desert-adapted bird. Sci. Rep. 10, 1–11. doi: 10.1038/s41598-020-75909-6
Refsnider, J. M., Clifton, I. T., and Vazquez, T. K. (2019). Developmental plasticity of thermal ecology traits in reptiles: trends, potential benefits, and research needs. J. Ther. Biol. 84, 74–82. doi: 10.1016/j.jtherbio.2019.06.005
Riddell, E. A., Iknayan, K. J., Wolf, B. O., Sinervo, B., and Beissinger, S. R. (2019). Cooling requirements fueled the collapse of a desert bird community from climate change. Proc. Natl. Acad. Sci. U. S. A. 116, 21609–21615. doi: 10.1073/pnas.1908791116
Rosenberg, K. V., Dokter, A. M., Blancher, P. J., Sauer, J. R., Smith, A. C., Smith, P. A., et al. (2019). Decline of the North American avifauna. Science 366, 120–124. doi: 10.1126/science.aaw1313
Sharpe, L., Cale, B., and Gardner, J. L. (2019). Weighing the cost: the impact of serial heatwaves on body mass in a small Australian passerine. J. Avian Biol. 50, 1–9. doi: 10.1111/jav.02355
Smit, B., Harding, C. T., Hockey, P. A. R., and McKechnie, A. E. (2013). Adaptive thermoregulation during summer in two populations of an arid-zone passerine. Ecology 94, 1142–1154. doi: 10.1890/12-1511.1
Smit, B., Zietsman, G., Martin, R. O., Cunningham, S. J., McKechnie, A. E., and Hockey, P. A. R. (2016). Behavioural responses to heat in desert birds: implications for predicting vulnerability to climate warming. Climate Change Respons. 3:9. doi: 10.1186/s40665-016-0023-2
Stillman, J. H. (2019). Heat waves, the new normal: summertime temperature extremes will impact animals, ecosystems, and human communities. Physiology 34, 86–100. doi: 10.1152/physiol.00040.2018
Thompson, M. L., Cunningham, S. J., and McKechnie, A. E. (2018). Interspecific variation in avian thermoregulatory patterns and heat dissipation behaviours in a subtropical desert. Physiol. Behav. 188, 311–323. doi: 10.1016/j.physbeh.2018.02.029
Tieleman, B. I., and Williams, J. B. (1999). The Role of Hyperthermia in the Water Economy of Desert Birds. Physiol. Biochem. Zool. 72, 87–100. doi: 10.1086/316640
Tieleman, B. I., and Williams, J. B. (2002). Effects of food supplementation on behavioural decisions of hoopoe-larks in the Arabian Desert: balancing water, energy and thermoregulation. Anim. Behav. 63, 519–529. doi: 10.1006/anbe.2001.1927
Urban, M. C. (2015). Accelerating extinction risk from climate change. Science 348, 571–573. doi: 10.1126/science.aaa4984
van de Pol, M., and Wright, J. (2009). A simple method for distinguishing within- versus between-subject effects using mixed models. Anim. Behav. 77, 753–758. doi: 10.1016/j.anbehav.2008.11.006
van de Ven, T. M. F. N., Mckechnie, A. E., and Cunningham, S. J. (2019). Thecosts of keeping cool: behavioural trade-offs between foraging and thermoregulation are associated with significant mass loss in an arid-zone bird. Oecologia 191, 1–6. doi: 10.1007/s00442-019-04486-x
van de Ven, T. M. F. N., McKechnie, A. E., Er, S., and Cunningham, S. J. (2020). High temperatures are associated with substantial reductions in breeding success and offspring quality in an arid-zone bird. Oecologia 193, 225–235. doi: 10.1007/s00442-020-04644-6
Welbergen, J. A., Klose, S. M., Markus, N., and Eby, P. (2008). Climate change and the effects of temperature extremes on Australian flying-foxes. Proc. R. Soc. B Biol. Sci. 275, 419–425. doi: 10.1098/rspb.2007.1385
West-Eberhard, M. J. (2003). Developmental Plasticity and Evolution. Oxford: Oxford University Press. doi: 10.1093/oso/9780195122343.001.0001
Wolf, B. O., and Walsberg, G. E. (1996). Respiratory and cutaneous evaporative water loss at high environmental temperatures in a small bird. J. Exp. Biol. 199, 451–457.
Keywords: behavioural flexibility, reaction norm, developmental plasticity, trade-off, heat dissipation, heat tolerance
Citation: Udino E and Mariette MM (2022) How to Stay Cool: Early Acoustic and Thermal Experience Alters Individual Behavioural Thermoregulation in the Heat. Front. Ecol. Evol. 10:818278. doi: 10.3389/fevo.2022.818278
Received: 19 November 2021; Accepted: 18 January 2022;
Published: 18 February 2022.
Edited by:
Francois Vezina, Université du Québec à Rimouski, CanadaReviewed by:
Krista Natasha Oswald, Ben Gurion University of the Negev, IsraelSusan Jane Cunningham, University of Cape Town, South Africa
Copyright © 2022 Udino and Mariette. This is an open-access article distributed under the terms of the Creative Commons Attribution License (CC BY). The use, distribution or reproduction in other forums is permitted, provided the original author(s) and the copyright owner(s) are credited and that the original publication in this journal is cited, in accordance with accepted academic practice. No use, distribution or reproduction is permitted which does not comply with these terms.
*Correspondence: Mylene M. Mariette, bS5tYXJpZXR0ZUBkZWFraW4uZWR1LmF1; bXlsZW5lLm1hcmlldHRlQGViZC5jc2ljLmVz