- Schiermeier Olentangy River Wetland Research Park, School of Environment and Natural Resources, The Ohio State University, Columbus, OH, United States
Urbanization in stream catchments can have strong effects on stream channel hydrogeomorphic features including channel dimensions, channel-floodplain connectivity, and flood regime. However, the consequences of hydrogeomorphic alterations on aquatic-terrestrial subsidy dynamics are largely unexplored. We examined the associations among hydrogeomorphic characteristics, emergent aquatic insect assemblages, and the density and trophic dynamics of riparian spiders of the family Tetragnathidae at 23 small urban stream reaches in the Columbus, OH (United States) Metropolitan Area. Naturally abundant stable isotopes of 13C and 15N were used to quantify the relative contribution of aquatically derived energy (i.e., nutritional pathways deriving from algae) to tetragnathid spiders and their trophic position. Bankfull discharge was negatively related to both emergence rate and family richness. On average, tetragnathid spiders relied on aquatically derived energy for 36% of their nutrition, with the greatest reliance found for spiders next to channels with wider flood-prone widths and proportionally fewer emergent insects of the family Chironomidae. Mean emergent aquatic insect reliance on aquatically derived energy was 32% and explained 44% of the variation in tetragnathid aquatically derived energy. A positive relationship between δ13C of tetragnathid spiders and emergent insects provides additional evidence of tetragnathid reliance on emergent insects. Mean tetragnathid trophic position was 2.85 and was positively associated with channel sinuosity and negatively associated with aquatic insect emergence rate. Sinuosity was also positively related to aquatically derived energy of emergent aquatic insects; as well as emergent insect family richness; % Ephemeroptera, Plecoptera, and Trichoptera (EPT); and aquatic insect emergence rate; implicating instream habitat-mediated shifts in emergent aquatic insect communities as an indirect mechanistic link between hydrogeomorphic processes and spiders. Our findings underscore that the impacts of stream hydrogeomorphic alterations can cascade into terrestrial food webs. These results suggest that monitoring and restoration of fluvial geomorphic form and function (e.g., sinuosity, slope, and hydrology) confer benefits to both aquatic and terrestrial riparian ecosystems in urban catchments.
Introduction
Streams are closely linked to the surrounding landscape through bidirectional fluxes of organisms, material, and energy between the stream and riparian zone (Baxter et al., 2005; Richardson et al., 2010; Sullivan and Rodewald, 2012). Fluxes of energy from the stream to adjacent riparian areas provide critical nutritional subsidies for a suite of terrestrial consumers, including riparian spiders (Sanzone et al., 2003; Kato et al., 2004; Tagwireyi and Sullivan, 2015), arthropods (Bastow et al., 2002; Paetzold et al., 2006; Gratton et al., 2008), lizards (Bastow et al., 2002), mammals (Fukui et al., 2006; Kautza and Sullivan, 2016), and birds (Iwata et al., 2003; Rowse et al., 2014; Kautza and Sullivan, 2016). Benthic aquatic insects that emerge from the water as winged adults (hereafter, emergent aquatic insects) have been shown to be more nutritionally advantageous to riparian consumers (Hanson et al., 1985; Gratton et al., 2008; Twining et al., 2016) than terrestrial insects and represent a critical prey item to many riparian consumers, thus representing an important aquatic-to-terrestrial energetic vector.
Riparian spiders can be heavily dependent on aquatic energy sources (Sanzone et al., 2003; Alberts and Sullivan, 2016), with web-building spiders such as those of the family Tetragnathidae especially reliant on emergent aquatic insects (Collier et al., 2002; Kato et al., 2004; Laeser et al., 2005). Because of this reliance, tetragnathid spiders have become increasingly used to quantify and evaluate aquatic-to-terrestrial energy and contaminant fluxes (Walters et al., 2010; Alberts and Sullivan, 2016). Both emergent aquatic insect biomass and emergence rate have been positively correlated with riparian spider biomass and web density (Burdon and Harding, 2008). Furthermore, the relative dependence on emergent aquatic insects, as well as the spatial and temporal distribution of orb-weaving spiders such as Tetragnathidae, vary in accordance with emergent aquatic insect emergence rates (Kato et al., 2003, 2004; Chan et al., 2009).
Aquatic-to-terrestrial subsidies can be influenced by terrestrial landscape (e.g., riparian vegetation structure and cover type; Alberts and Sullivan, 2016) as well as instream conditions including disturbance (Greenwood and McIntosh, 2008), habitat availability (Laeser et al., 2005; Chan et al., 2009), in-stream macroinvertebrate assemblage characteristics (Paetzold et al., 2011; Gergs et al., 2014), and physical stream characteristics (Laeser et al., 2005; Kennedy and Turner, 2011). For shoreline spiders, low-disturbance environments have been associated with high aquatic insect emergence rates but low web-building habitat availability, whereas high disturbance environments create the opposite condition, in which aquatic prey is sparse but web-building habitat is highly available (Greenwood and McIntosh, 2008). Web-building substrate in the riparian zone as well as riparian vegetation characteristics are also important factors associated with riparian spider density and reliance on aquatic nutritional subsidies (Chan et al., 2009; Krell et al., 2015; Tagwireyi and Sullivan, 2015). Decreases in emergent aquatic insect abundance can lead to decreases in tetragnathid spider density as well as broader community-wide shifts in riparian arthropods (Baxter et al., 2004; Paetzold et al., 2011; Gergs et al., 2014).
Urban streams are commonly characterized by increased runoff volume as a result of larger, more frequent floods and decreased baseflows (Chin, 2006; Walsh et al., 2012; Vietz et al., 2016), resulting in a geomorphic evolution of channel form, commonly progressing from stable states through incision and widening, and finally aggradation as streams regain quasi-stable forms of diverse nature (Schumm, 1984; Hawley et al., 2012; Rieck and Sullivan, 2020). Urban stream channels are frequently, though not always, enlarged (Doll et al., 2002; Cianfrani et al., 2006; O’Driscoll et al., 2010). Collectively, urban-associated alterations to stream channels can lead to a wide variety of hydrogeomorphic conditions across an urbanization gradient (e.g., Rieck and Sullivan, 2020).
Riparian landscape characteristics play a critical role in the magnitude of channel response to urban flow regimes (Finkenbine et al., 2000; Bledsoe and Watson, 2001; Kang et al., 2010). Flow regime can influence the density, rate, and composition of aquatic-to-terrestrial subsidies: high-scour floods result in low periphyton standing stocks and filterer-dominated macroinvertebrate assemblages, whereas lower-scour areas tend to have more abundant periphyton and a shift to a predominance of collector-gatherers and scrapers (Fuller et al., 2010). Low flows and dry-downs may decrease the biomass of the emergent aquatic insect flux, resulting in decreased biomass and average body size of riparian spiders (Greenwood and McIntosh, 2010). Seasonally predictable streamflow regimes and faster streamflow velocities can result in greater densities of larvae with winged adult phases, increasing emergence from streams subject to these conditions (Greenwood and Booker, 2016).
Ultimately, the interplay between riparian conditions (e.g., canopy cover and detrital contributions) and instream conditions (e.g., light availability and streamflow variability) are likely to shape characteristics of the emergent insect flux (Kraus and Vonesh, 2012). Urban stream channels are often more homogeneous in both habitat and form (Booth and Jackson, 1997; Cianfrani et al., 2006; O’Driscoll et al., 2010), whereas riparian habitat may be altered by geomorphic alterations that reduce groundwater levels in the riparian zone (e.g., incision; Groffman et al., 2003; Hardison et al., 2009). These habitat changes may alter aquatic-to-terrestrial energy fluxes via effects on the benthic insect assemblage, and thus emergent aquatic insects (Sullivan et al., 2004; Fend et al., 2005; Kennedy and Turner, 2011). Further, instream and riparian habitat changes can alter both the transport of subsidies into the riparian zone as well as the suitability of the riparian habitat itself for consumers (Chan et al., 2009; Kautza and Sullivan, 2015; Tagwireyi and Sullivan, 2015).
Quantifying trophic impacts of altered bidirectional aquatic-terrestrial exchanges can be accomplished using several metrics. Reliance on aquatically derived energy (ADE; nutritional pathways originating from benthic algae; sensu Kautza and Sullivan, 2016) in the diet of consumers can be used to assess the relative reliance of consumers on instream primary production (i.e., algae) versus terrestrial production (i.e., terrestrial detritus, vegetation; Kautza and Sullivan, 2016). Trophic position (TP) describes the number of trophic steps between basal resources and a consumer, providing a metric of food-web complexity (Jackson and Sullivan, 2018). Both metrics allow for assessments of how stressors impact the basal energetic resources most important to and the structure (e.g., size and length) of the coupled terrestrial-aquatic food-web. For example, aquatically derived energy and TP of emergent aquatic insects have both been shown to be influenced by invasive vegetation in the riparian zone (Diesburg et al., 2021), while ecosystem size and hydrological disturbance have been shown to influence the ADE and TP of riparian spiders (Jackson and Sullivan, 2018), and riparian vegetation characteristics and urban development influence the ADE and TP of riparian ants (Tagwireyi and Sullivan, 2015).
Our goal was to explore associations between variability in hydrogeomorphic characteristics and aquatic-terrestrial ecological linkages. To do this, we investigated the associations between variability in stream hydrogeomorphic features and aquatic-terrestrial nutritional subsidies to shoreline riparian spiders at a suite of 23 small urban streams in the Columbus Metropolitan Area (CMA), OH, United States. We expected that hydrogeomorphic conditions that weaken stream-floodplain connectivity (e.g., incision, entrenchment, reduced width-of-floodprone area) would reduce the density, reliance on ADE, and TP of spiders of the family Tetragnathidae via reduced quantities and altered composition of emergent aquatic insect fluxes. In addition, we expected that riparian vegetation characteristics would be related to habitat availability for tetragnathid spiders, and thus density, but have less impact on ADE or TP. We anticipate that these findings will have important implications for current understanding and management of coupled stream-riparian systems in urban landscapes.
Materials and Methods
Study Reaches
Based on more than a decade of extensive research experience in the CMA, we selected 12, 1st-3rd order urban stream reaches of similar drainage area that were reliably accessible and represented a range of hydrogeomorphic conditions. During the summers of 2011–2013, all study reaches were surveyed once for hydrogeomorphic characteristics, chemical water quality, riparian habitat and vegetation, emergent aquatic insects, riparian spiders, detritus, and periphyton. Study reaches were 25–30 × estimated bankfull width in length (Kondolf and Larson, 1995; Kondolf and Micheli, 1995) and ranged from 89 to 390 m (Supplementary Table 1). All study reaches were located in the lower Scioto River catchment (Figure 1). Hydrogeomorphic characteristics, emergent aquatic insects, detritus, and periphyton were measured at all sites in the summer of 2011, riparian spiders were surveyed at all sites in the summer of 2012, and chemical water quality and riparian habitat and vegetation were surveyed at all sites in the summer of 2013.
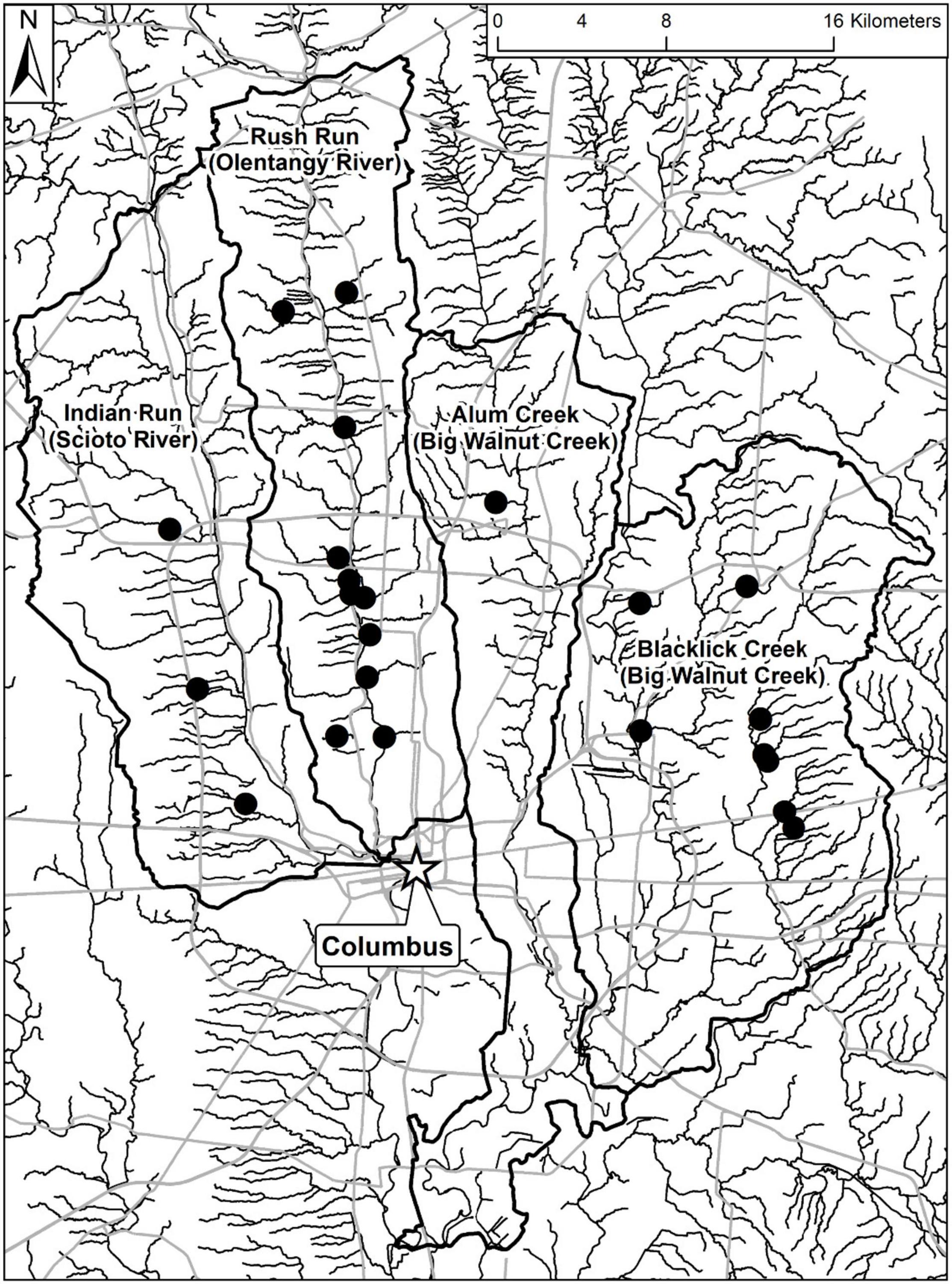
Figure 1. Study-reach locations overlaid on major roadways and waterways and within their major drainage sub-catchments of the Columbus Metropolitan Area, OH, United States. The Scioto River drains 16,822 km2, Olentangy River 1,406 km2, and Big Walnut Creek 890 km2. The Blacklick, Rocky Fork, and Alum Creek sub-catchments drain 159, 49, and 320 km2, respectively.
We used the StreamStats online tool developed for Ohio (U.S. Geological Survey, 2016) to delineate catchments upstream of the terminal end of each study reach, then used geographic information systems (ArcGIS 10.3.1, Esri, Redlands, California, United States) to derive land-cover types (e.g., high-, medium-, and low-density urban cover). Stream length was derived from the National Hydrography Dataset Plus (U.S. Geological Survey, 2014), and a 30-m buffer was created around each stream to represent the riparian area upstream of the terminal end of the study reach, given evidence that riparian zones of small streams may need to be ≥ 30 m to be ecologically functional (Lind et al., 2019). We then used the 2011 National Land Cover Database (Homer et al., 2015) to derive percentages of developed land use and impervious surface coverage (i.e., imperviousness) for each catchment upstream of the terminal end of each study reach as well as within the 30-m riparian buffer. Catchment total development ranged from 19.5 (Leeds) to 100.0% (Linworth, Beechwold, Waterman), while catchment imperviousness ranged from 3.3 to 67.7% (Supplementary Table 1).
Hydrogeomorphic Features
Fluvial geomorphic features were surveyed using techniques outlined in Ward and Trimble (2004) with further details in MacFarland (2012), using a laser level (SPECTRA self-leveling laser, Trimble Construction Tools Division, Dayton, OH, United States), laser receiver mounted on a stadia rod, 30-m measuring tapes, a compass, and a 254-cm ruler (for pebble counts). Longitudinal surveys ran along the thalweg of the channel, measuring channel azimuth as well as bed and water elevation at each point of noticeable grade change and bankfull elevation at 8–12 points along the reach where bankfull indicators were clear (Ward and Trimble, 2004). At three representative riffles along the reach, we conducted cross-sectional surveys measuring surface elevation across the entire estimated floodprone width and performed a Wolman (1954) pebble count of n = 100 bed particles per cross-section (n = 300 bed particles per reach).
Directly measured (e.g., bankfull width, D50) and calculated (e.g., shear stress, estimated bankfull discharge) hydrogeomorphic variables were compiled or calculated using the Reference Reach Spreadsheet 4.3L (Powell et al., 2006) in Microsoft Excel (2019). This spreadsheet tool was developed to calculate second-order variables using first-order variables as well as standards derived from regional curves for central Ohio (Supplementary Table 2). For instance, entrenchment ratio, which is the ratio of floodprone to bankfull width (Supplementary Table 2), measures the vertical containment of the stream, either through incision or the containment via dikes or other structures and indicates the degree to which the stream can access its floodplain. Incision ratio, which is the ratio of low bank height to bankfull depth (Supplementary Table 2), indicates the extent to which the stream has cut down into the channel bed, and while similar to entrenchment ratio due to its description of vertical confinement, more directly addresses hydrogeomorphic mechanisms.
Chemical Water-Quality
Owing to the potential influence of water quality on aquatic invertebrates in urban catchments (Wang and Kanehl, 2003; Sterling et al., 2016; Luo et al., 2018), chemical water-quality and nutrient samples [total nitrogen (N; mg L–1), total phosphorus (P; mg L–1), total mercury (Hg; ppt), and total dissolved solids (TDS; mg L–1)] were obtained from one grab sample of water composited from 5–7 locations throughout each study reach. Refrigerated samples were sent to The Ohio State University’s Service Testing and Research (STAR) laboratory for analysis within 24 h of collection. The STAR laboratory used cold vapor atomic fluorescence using a CETAC M8000 mercury analyzer (CETAC Technologies, Omaha, Nebraska, United States), calibrated with NIST-traceable 100 mg L–1 mercury standard (SPEC CertiPrep, Metuchen, New Jersey, United States), on samples that had been digested using a 1:1 mixture of trace metal grade perchloric acid and nitric acid.
Temperature (°C), conductivity (μS), dissolved oxygen (DO; % and mg L–1), pH, and oxidation-reduction potential (ORP; mV) were measured at 16 of the study reaches using a multimeter sonde (YSI, Inc. 600QS, Yellow Springs, OH, United States). Loss of access due to landowner changes or equipment failure prevented measurement of these variables at the remaining six study reaches.
Instream, Streambank, and Riparian Vegetation Characteristics
We assessed instream canopy cover using a densiometer following Lemmon (1957; utilized in streams in Hassett et al., 2018), performing three longitudinal transects (one along the right bank, one along the thalweg, one along the left bank) along 200 m centered within each reach, with measurements taken facing each cardinal direction every 20 m. We also estimated riparian canopy cover using a similar technique, using the densiometer along four randomly placed lateral (to the stream) 30-m riparian transects using a modified point-intercept method (see details in Jackson and Sullivan, 2009), measuring canopy cover every 10 m (Lemmon, 1957).
Along these same four transects (2 left bank, 2 right bank), we identified the nearest woody vegetation to species and its diameter at breast height (DBH) every 5 m and conducted one herbaceous vegetation cover survey at a randomly selected distance away from the stream on each transect (for a total of four herbaceous cover plots, 1 m2 each). At each 1-m2 herbaceous cover plot, we estimated total percentage herbaceous cover as well as the first, second, and third most common (when present) vegetation species [further details in Jackson and Sullivan (2009)]. Streambank vegetation was assessed along transects along both right and left banks throughout the entire reach length. At every 10 cm along the reach length on both the left and right banks, we noted the presence of streambank cover and the species of vegetation (when present; Symonds, 1958, 1973), which also allowed estimates of streambank honeysuckle (Lonicera maackii and L. tatarica) cover, a common and pervasive invasive plant in the study system. Invasive honeysuckle was of interest due to its potential to enhance web-building substrate for tetragnathid spiders (Loomis et al., 2014; Tagwireyi and Sullivan, 2015), facilitate certain aquatic insect taxa (e.g., Culex pipiens; Shewhart et al., 2014) while harming others (Watling et al., 2011), and alter aquatic basal resource quality and timing (Watling et al., 2011; McNeish et al., 2015).
Emergent Aquatic Insects and Orb-Weaving Spiders
Owing to our focus on the ecological linkages between the stream and the riparian zone, we focused our collection efforts on emergent aquatic insects, which more accurately represent the realized aquatic-to-terrestrial subsidy than benthic macroinvertebrates. Multiple factors – including light availability, allochthonous resource availability (Kraus and Vonesh, 2012), canopy cover (Banks et al., 2007), the presence of predators (Pope et al., 2009; Wesner, 2013) and contamination (Wesner, 2019) – can influence aquatic insect emergence and, thus, lead to benthic-emergent community differences. We collected emergent aquatic insects using Mundie-style traps (Mundie, 1956) placed in one riffle, reach, and pool, respectively, in each study reach once for 8–10 days; all insects were preserved in 70% ethanol. From multiple studies in the study system quantifying ecological linkages between land and water (e.g., Rowse et al., 2014; Sullivan et al., 2018, 2021), we considered our sampling effort sufficiently robust to capture heterogeneity in emergent aquatic insects through careful selection of representative habitats in which traps were placed. Moreover, our sampling methodology and effort were generally consistent with other studies that have measured emergent insect fluxes in streams (e.g., Malison et al., 2010; Kautza and Sullivan, 2015).
In the laboratory, all individuals were counted and identified to family following Merritt et al. (2008). We then calculated assemblage characteristics, including family richness, Shannon-Weiner diversity index (H’, using families in place of species), evenness (E), and percentage of individuals belonging to the families Chironomidae (the most numerically abundant insect taxon across all reaches) and Ephemeroptera, Plecoptera, and Trichoptera (EPT; Supplementary Table 2). Subsequently, we sorted individuals by family before categorizing them based on functional feeding groups according to Merritt et al. (2008) for subsequent stable-isotope analysis, except for Chironomidae, which we kept as a separate group based on their high densities at all sites.
To assess tetragnathid spider density, we conducted longitudinal surveys of both right and left banks at each study reach shortly after 2100 h once per study reach. During 15-min surveys along 30 m (centered within the study reach), we visually inspected the streambank and immediate riparian zone for orb webs, counting the number of webs per m up to 2 m in height and 3 m landward from the streambank (Williams et al., 1995). Using orb webs as a 1:1 proxy for spiders is justified by the habits of tetragnathid spiders, which are known re-build their webs on a daily basis, making it possible to estimate the number of small, reclusive spiders by surveying the number of webs present at night, when these spiders are known to be most active (Williams et al., 1995; Diesburg et al., 2021). At each study reach, we also collected 7–12 tetragnathid spiders from orb webs for stable-isotope analysis (Akamatsu et al., 2004).
Periphyton and Terrestrial Detritus
Periphyton was obtained by selecting at least 10 bed substrate particles, distributed evenly throughout the reach. Algal material (primarily diatoms) was scrubbed from particles and rinsed into sample jars containing 70% ethanol. We collected instream detritus by separating out detrital material from three separate 90-s Surber samples (0.31 × 0.31 m, 500-μm mesh, Forestry Suppliers, Jackson, Mississippi, United States) conducted at the same riffles, runs, and pools as those in which emergent traps were placed.
Stable-Isotopes
We rinsed tetragnathid spiders and emergent aquatic insects in deionized water and then dried individuals for 48 h at 60° C (Lorrain et al., 2003). Tetragnathid spiders were sorted by study reach, while emergent insects were sorted and grouped by study reach, family, and functional feeding group. We used periphyton and terrestrial detritus as isotopic baselines. Periphyton was sorted to remove non-algal material and terrestrial detritus. All samples were dried at 60° C for 48 h, then ground using a mortar and pestle (periphyton, spiders, emergent insects) or ball mill grinder (terrestrial detritus).
Stable isotopes (13C, 15N) were analyzed at the Washington State University Stable Isotope Core (Pullman, Washington, United States) using continuous flow isotope ratio mass spectrometry (Delta Plus XP, Thermofinnigan, Bremen, Germany), and reported as δ13C and δ15N as parts per thousand deviation from an estimated standard (e.g., Vienna Peedee belemnite limestone for δ13C, atmospheric N for δ15N). Typical precision was 0.08‰ (δ15N) and 0.19‰ (δ13C). δ13C and δ15N were calculated as:
where,
We used epilithic algae as δ13Cbase1 and terrestrial detritus as δ13Cbase2 for basal food-source end members. We calculated estimated TP for Tetragnathidae and emergent aquatic insects using per trophic step fractionation (Post, 2002), estimating the number of trophic transfers between consumer and basal resources by dividing the difference between consumer δ15N and mean basal resource δ15N by an estimated trophic fractionation rate of 2.75‰, a value increasingly used for orb-weaving spiders in other studies in lotic systems (Eq. 3; Kelly et al., 2015). Some variability is inherent in the use of this fractionation value, as others have found that 2.54‰ may be a more appropriate value for certain consumers (Vanderklift and Ponsard, 2003) or that a fixed fractionation rate may be inappropriate in certain situations (Caut et al., 2009; Hussey et al., 2014). We addressed this variability by utilizing a sufficiently large standard deviation in mixing models (SD = 2.20). The use of primary producers [compared to longer-lived primary consumers (e.g., molluscs)] as isotopic baselines also introduces further sources of variability due to high spatiotemporal variability of stable-isotope signatures of primary producers (Cabana and Rasmussen, 1996; Post, 2002). However, we reduced this error to the extent possible by sampling primary producers in the same habitats and as close to the same timeframe as consumers as possible (Post, 2002).
Reliance on ADE was calculated as the ratio of the difference between consumer and detrital δ13C divided by the difference between periphyton δ13C and detrital δ13C (Eq. 4).
We used the MixSIAR (Stock et al., 2018, 2020) package in R (R Core Team, 2020) to estimate both TP and ADE. MixSIAR is a Bayesian mixing-model approach that improves parameterization of error structure, more realistically estimates predation rates, and allows for construction of informative priors (Stock et al., 2018). For δ13C, we used fractionation values of 0.08 ± SD 1.90 per trophic step (Kelly et al., 2015). We used posterior density plots, the Gelman-Rubin diagnostic test, and the Geweke diagnostic test to evaluate model performance.
Analytical Methods
We selected 10 candidate hydrogeomorphic variables (width-of-floodprone area, discharge, shear stress, bankfull width, width:depth ratio, entrenchment ratio, sinuosity, D50, slope, and incision ratio) that were found to be correlated with biotic-assemblage characteristics [e.g., diversity (H’), density] in previous work in the study system (e.g., Rieck and Sullivan, 2020). We then used canonical correspondence analysis (CCA) to investigate associations between these 10 variables and relative abundances of emergent aquatic insect families using the vegan package (Oksanen et al., 2019) in R (R Core Team, 2020), eliminating hydrogeomorphic variables to keep variance inflation factors < 10 (Greenacre, 1984; Gross, 2003). This process resulted in the inclusion of seven hydrogeomorphic variables for the final analyses of emergent aquatic insect and hydrogeomorphic variables: slope, incision ratio, entrenchment ratio, width-of-floodprone area, discharge, D50, and sinuosity. Emergent aquatic insect flux metrics [emergence rate, diversity (H’), % chironomids], water-quality variables [total N (total P excluded due to very high correlation with total N), total Hg, TDS], and riparian vegetation characteristics (% instream canopy cover, riparian canopy cover) were also considered in building models addressing tetragnathid density, TP, and ADE. This set of variables was selected from the full suite of emergent aquatic insect flux, water-quality, and riparian vegetation predictors to include only those that maintained variance inflation factors < 10 (Greenacre, 1984; Gross, 2003) when including the seven previously selected hydrogeomorphic variables. Models investigating emergent aquatic insect response variables considered the same hydrogeomorphic, chemical water quality, and riparian vegetation predictor variables. Sonde data, temperature, conductivity, pH, DO, and ORP were not included in the formal analysis due to missing data, but were used to visually screen for notable variability in these water-quality parameters across study reaches.
All model analyses were completed in R (R Core Team, 2020). We identified the most appropriate distributions for all variables using the fitdistrplus package (Delignette-Muller and Dutang, 2015; Table 1). We used an AICc model selection approach following Feld et al. (2016). All predictor variables were transformed to best approximate normal distributions and scaled to have a mean of zero and standard deviation of 1 (zscale function) and assessed for collinearity (library usdm, vifstep function), ensuring variance inflation factors were ≤ 5 (Feld et al., 2016). Using fitdistrplus (Delignette-Muller and Dutang, 2015) after transformation showed that transformed variables best fit a normal distribution. Response variables were not transformed, but instead we built a “full” generalized or general linear model (glm or lm function, depending on the distribution that best fit the specific response variable; Table 1) from which all possible combinations of predictors could be assessed using the “dredge” function within MuMIn (Bartón, 2020). Full models for tetragnathid spider response variables included slope, incision ratio, entrenchment ratio, width-of-floodprone area, D50, sinuosity, emergence rate, % Chironomidae, total mercury, % instream canopy cover, total nitrogen, and TDS as predictor variables. Full models for emergent aquatic insect variables included slope, incision ratio, entrenchment ratio, D50, sinuosity, total mercury, % instream canopy cover, total nitrogen, and TDS as predictor variables. Models with AICc < 2 were considered well supported and further assessed after excluding those models containing uninformative parameters (i.e., those whose addition to an existing model resulted in an improvement in AICc of < 2; Arnold, 2010). Within each model, we defined the cutoffs for a relationship as p < 0.05 and a trend as 0.05 < p ≤ 0.10 (Rowse et al., 2014; Diesburg et al., 2021).
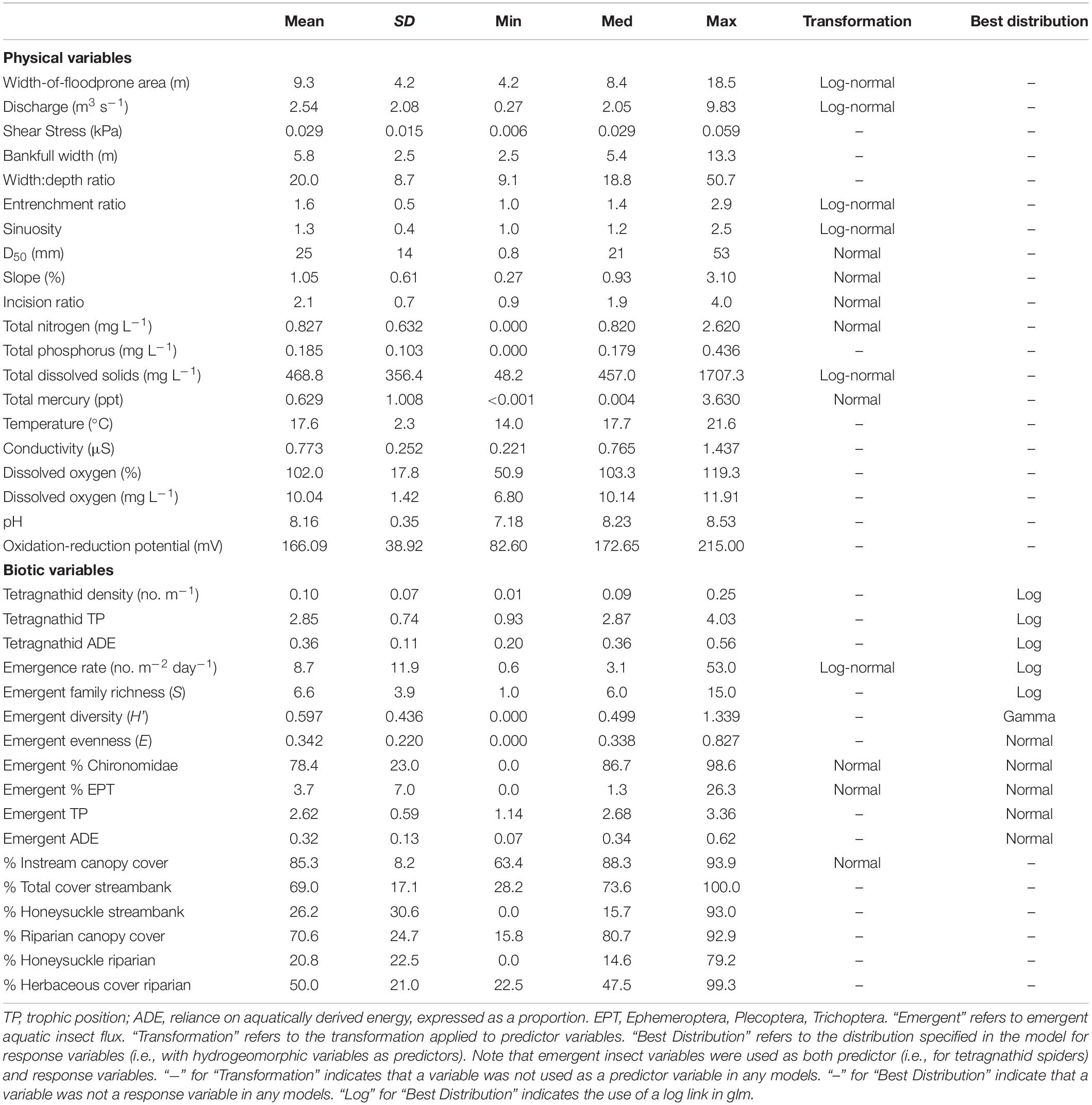
Table 1. Descriptive statistics for all physical and biotic variables included in the analysis, including mean, standard deviation (SD), minimum (Min), median (Med), and maximum (Max).
Results
Vegetation, Water Quality, and Hydrogeomorphic Characteristics
Instream canopy cover and streambank cover exhibited means of 85.3 and 69.0%, respectively (Table 1). Canopy cover in the riparian zone had a mean of 70.6% (SD = 24.7%; Table 1). Mean coverage of invasive honeysuckle in the riparian zone was 20.8% (SD = 22.5%) while mean honeysuckle coverage on streambanks was 26.2% (SD = 30.6%). Coverage of honeysuckle in the riparian zone ranged from 0% at Beechwold, Big, Blendon, Dysart, Jefferson, and Rush to 79.2% at Waterman (Supplementary Table 3).
Total N and total P had means of 0.827 mg L–1 (SD = 0.632 mg L–1) and 0.185 mg L–1 (SD = 0.103 mg L–1), ranging from < 0.01 and < 0.001 mg L–1 to 2.62 and 0.436 mg L–1, respectively (Table 1 and Supplementary Table 4) and were highly correlated with one another (r = 0.82, p < 0.001). Hg in streamwater ranged from < 0.001 to 3.630 ppt ( = 0.629), while TDS ranged from 48.17 to 1707.30 mg L–1 ( = 468.8 mg L–1; Table 1 and Supplementary Table 4). The highly urbanized Waterman study reach had markedly higher concentrations of total N, total P, and TDS (Supplementary Table 4) than the other study reaches.
Stream bankfull width and width-of-floodprone area ranged from 2.5 and 4.2 m at Greenridge to 13.3 and 18.5 m at Adena, respectively ( = 5.8 m, 9.3 m; SD = 2.5 m, 4.2 m, respectively; Table 1 and Supplementary Table 5). Some channels showed signs of entrenchment (entrenchment ratio = 1.6, SD = 0.5; Table 1 and Supplementary Table 5) or incision (incision ratio = 2.1, SD = 0.70; Table 1 and Supplementary Table 5). Discharge ranged from 0.27 to 9.83 m s–1 ( = 2.54 m s–1, SD = 2.08 m s–1), sinuosity ranged from 1 to 2.5 ( = 1.3, SD = 0.4), and D50 ranged from 0.8 to 53 mm ( = 25 mm, SD = 14 mm; Table 1 and Supplementary Table 5).
Emergent Aquatic-Insect Flux
The rate of aquatic insect emergence ranged from 0.6 m–2 day–1 at Greenridge and Highbanks to 53.0 m–2 day–1 at Indian ( = 8.7 m–2 day–1, SD = 11.9 m–2 day–1; Table 1 and Supplementary Table 6). Family richness ( = 6.6, SD = 3.9), H’ ( = 0.597, SD = 0.436), and evenness ( = 0.342, SD = 0.220) also exhibited substantial variability across study reaches (Table 1). Assemblages were largely dominated by Chironomidae ( = 78.4% of numerical total, SD = 23.0%; Table 1), with very low relative percentages of EPT taxa [ = 3.7%, SD = 7.0% (Table 1); EPT was 0% at nine reaches (Supplementary Table 6)].
Reducing the number of hydrogeomorphic variables in our dataset to keep variance inflation factors < 10 resulted in a canonical correspondence analysis using seven hydrogeomorphic variables: slope, incision ratio, entrenchment ratio, width-of-floodprone area, D50, discharge, and sinuosity (Figure 2). The primary axis of our canonical correspondence analysis (CCA) explained 20.53% of the variation in emergent aquatic-insect flux assemblage composition, while the secondary axis explained 19.54% (Figure 2). Sinuosity was negatively associated with CCA2, slope was negatively associated with CCA1, and width-of-floodprone area and D50 were positively associated with CCA1 (Figure 2). Incision ratio, entrenchment ratio, and discharge were not strongly associated with either axis. The most ubiquitously distributed family- Chironomidae- was not strongly associated with either axis, while less common families were associated with at least one axis. For instance, Cecidomyiidae (gall midges) and Calopterygidae (broad-winged damselflies) were negatively associated with CCA1, and were also strongly and positively associated with slope, while Ephydidae (shore flies), Hydropsychidae (net-spinning caddisflies), Heptageniidae (clinger mayflies), and Phoridae (humpbacked flies) were negatively associated with CCA2 and positively associated with sinuosity.
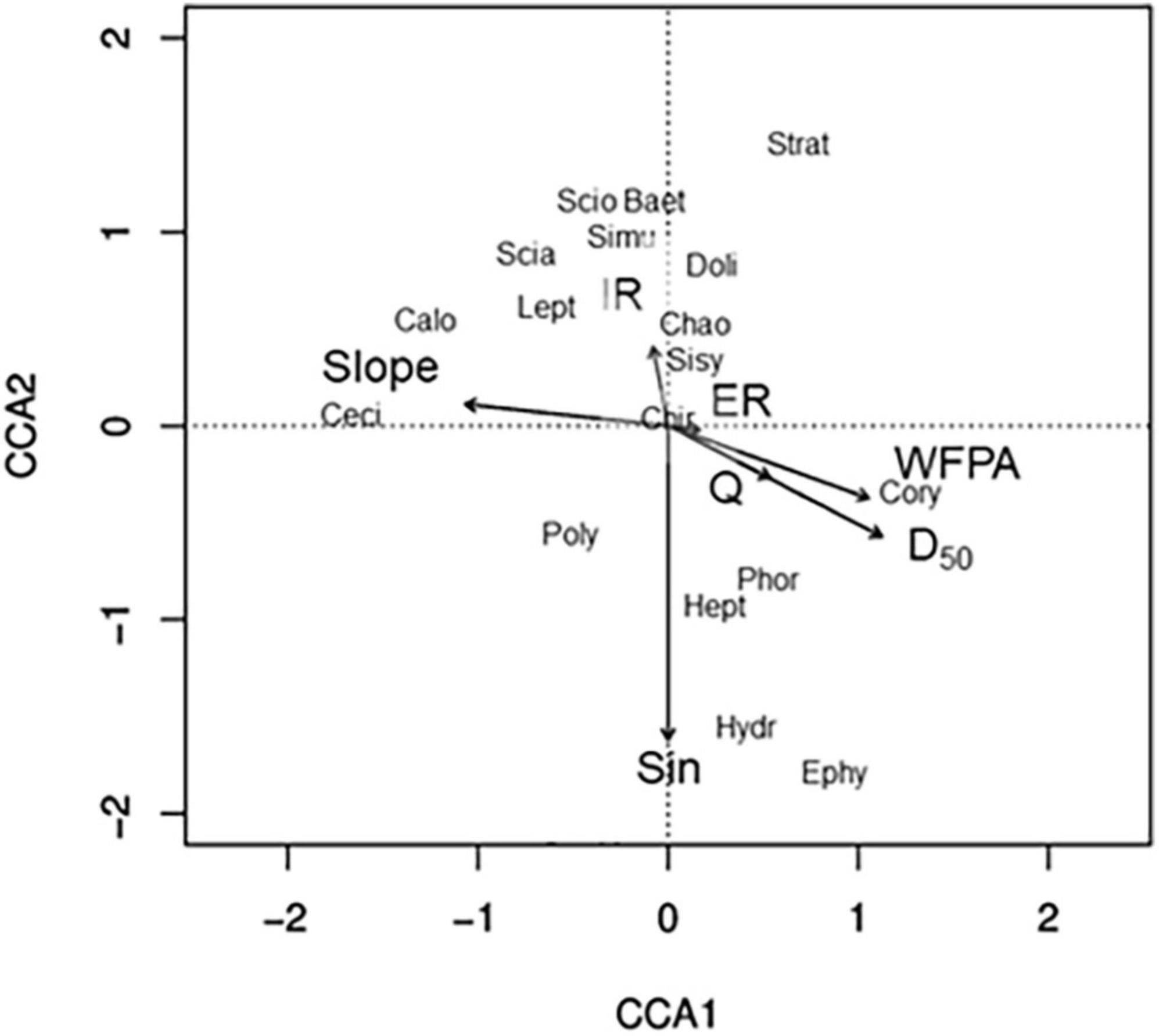
Figure 2. Ordination biplot based on canonical correspondence analysis of seven hydrogeomorphic variables that minimized variance inflation factors and the relative abundance of emergent aquatic insect families. CCA1 and CCA2 explained 20.53 and 19.54% of the variation in emergent aquatic insect assemblages, respectively. Only the nine most common and nine rarest emergent-insect families are shown. Strat, Stratiomyidae; Baet, Baetidae; Scio, Sciomyzidae; Simu, Simulidae; Scia, Sciaridae; Lept, Leptohyphidae; Doli, Dolichopodidae; Sisy, Sisyridae; Doli, Dolichopodidae; Chao, Chaoboridae; Cory, Corydalidae; Phor, Phoridae; Chir, Chironomidae; Hept, Heptageniidae; Ephy, Ephydidae; Poly, Polycentropodidae; Calo, Calopterygidae; Ceci, Cecidomyiidae, Hydr, Hydropsychidae. Slope, channel bed slope; IR, incision ratio; ER, entrenchment ratio; WFPA, width-of-floodprone area; Q, discharge; D50, median sediment particle size; Sin, sinuosity.
Emergent Aquatic-Insect and Tetragnathid Spider Density and Trophic Dynamics
Periphyton δ13C was more enriched ( = −19.96‰, SD = 4.50‰) than detrital δ13C ( = −27.57‰, SD = 0.73‰), as was periphyton δ15N ( = 6.55‰, SD = 2.71‰; detrital δ15N = 1.73‰, SD = 1.76‰). General agreement with values for other stream systems in the study region suggest that our efforts to remove non-algal material from our periphyton samples to obtain adequate primary producer isotopic signatures (which can be challenging; Delong and Thorp, 2006; Zeug and Winemiller, 2008) appear to have been successful. For example, Kautza and Sullivan (2016) reported periphyton δ13C of −21.68‰ in the Scioto River and Weber et al. (2017) reported values of 30.0‰ in the Muskingum River, OH. Our periphyton δ15N values were also similar to those for primary producers in the region (e.g., 9.57‰; Kautza and Sullivan, 2016). We also observed adequate separation between our basal resources, with periphyton being separated from detritus by 7.6‰ (δ13C) and 4.8‰ (δ15N), leading to our mixing models converging based on Geweke’s diagnostic tests (all p > 0.10) and visual assessment of trace plots (Supplementary Figure 1).
Stable isotope results were pooled for emergent aquatic insects, such that values for ADE and TP represented the average value for each study reach. Mean emergent aquatic emergent TP across all taxa and study reaches was 2.62 (SD = 0.59, Table 1), ranging from 1.14 at Sycamore to 3.08 at Sharon and Rush (Supplementary Table 6). Overall, emergent aquatic insects relied on ADE for 0.32 (SD = 0.13) of their nutritional needs, with the remaining 0.68 coming from terrestrially derived energetic pathways (Table 1).
Tetragnathid spider density ranged from 0.01 m–1 at Kempton to 0.25 m–1 at Wetlands ( = 0.1 m–1, SD = 0.07 m–1; Table 1 and Supplementary Table 7), both of which were highly urbanized. Tetragnathid reliance on ADE was generally less than 0.50 ( = 0.36, Table 1), though variable (SD = 0.11; Table 1), ranging from near 0.20 at Sycamore to 0.56 at Big and Leeds (Supplementary Table 7). Tetragnathid TP ranged from 0.93 (Cole) to 4.03 (Leeds; Table 1 and Supplementary Table 7). Tetragnathid TP was not significantly associated with emergent aquatic insect TP (R2 = 0.06, F = 1.15, p = 0.300), but tetragnathid ADE was positively correlated with emergent aquatic insect ADE (R2 = 0.44, F = 13.59, p = 0.002; Figure 3A). Tetragnathid δ13C was also positively correlated with aquatic insect δ13C (R2 = 0.20, F = 4.55, p = 0.047; Figure 3B), further suggesting a nutritional dependence of spiders on aquatic emergent insects.
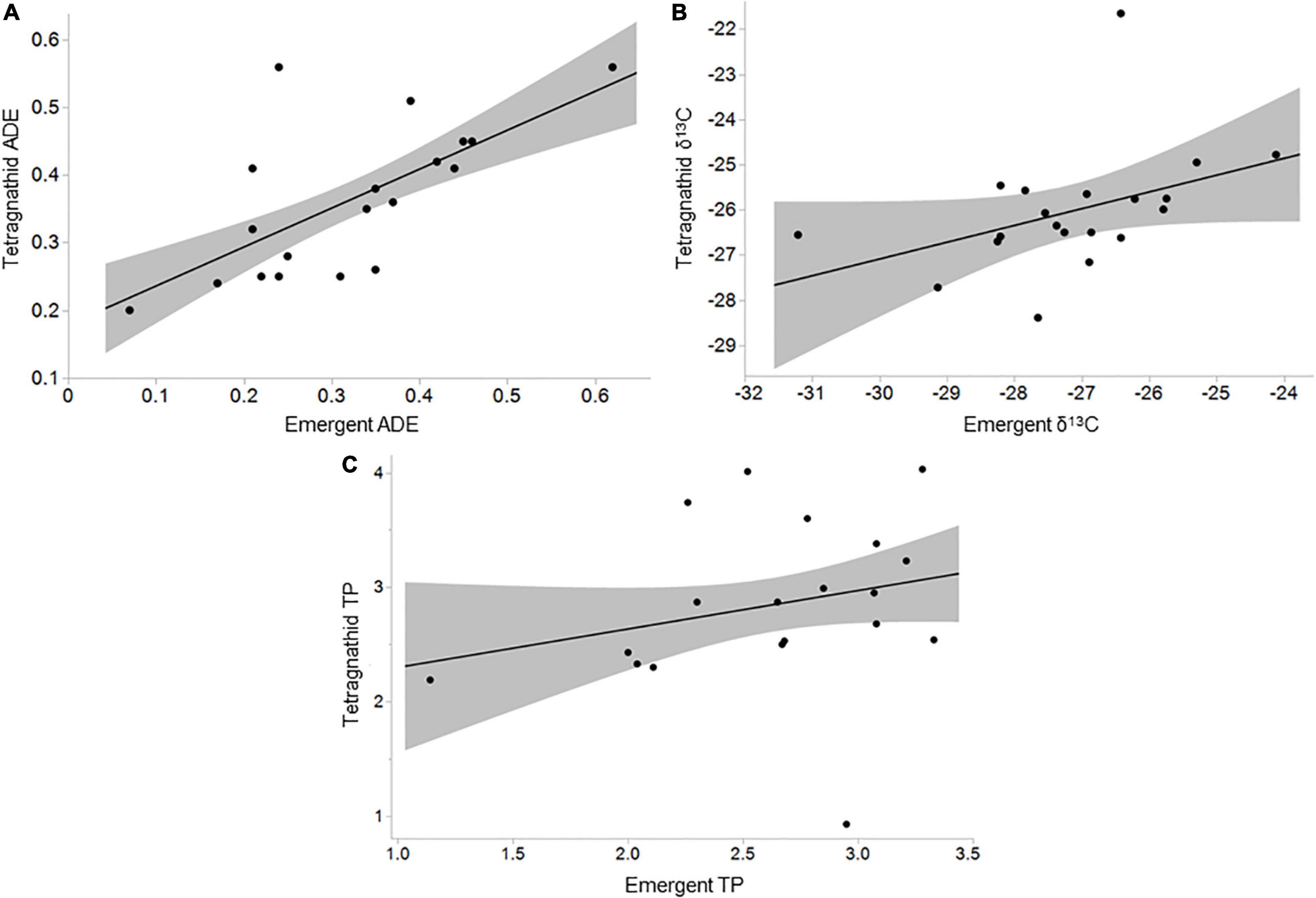
Figure 3. Relationships between (A) emergent aquatic insect aquatically derived energy (ADE) and tetragnathid spider ADE (R2 = 0.44, F = 13.59, p = 0.002); (B) emergent aquatic insect and tetragnathid spider δ13C (R2 = 0.20, F = 4.55, p = 0.047); and (C) emergent aquatic insect trophic position (TP) and tetragnathid spider TP (R2 = 0.06, F = 1.16, p = 0.297). Shaded area indicates 95% confidence interval.
Predictors of Emergent Aquatic Insect Fluxes
The null model was the best supported model (ΔAICc = 0) for emergent aquatic insect TP, and no variables included in strongly supported (ΔAICc < 2) models were significant for aquatic insect TP (Table 2). All other emergent aquatic insect models had non-null models with the strongest support (ΔAICc = 0). Bankfull discharge was featured in supported models of emergence rate (Figure 4A) and family richness (negative relationships) and evenness (positive relationship; Table 2). Sinuosity displayed positive relationships with emergence rate, family richness, % EPT, and ADE (Table 2). The concentration of TDS was the predominant factor in supported models of diversity (Figure 4B) and % Chironomidae (positive relationships) and evenness (negative relationship; Table 2). Total dissolved solids was also a salient predictor in several strongly supported models of emergence rate (positive relationship) and % EPT (negative relationship; Table 2).
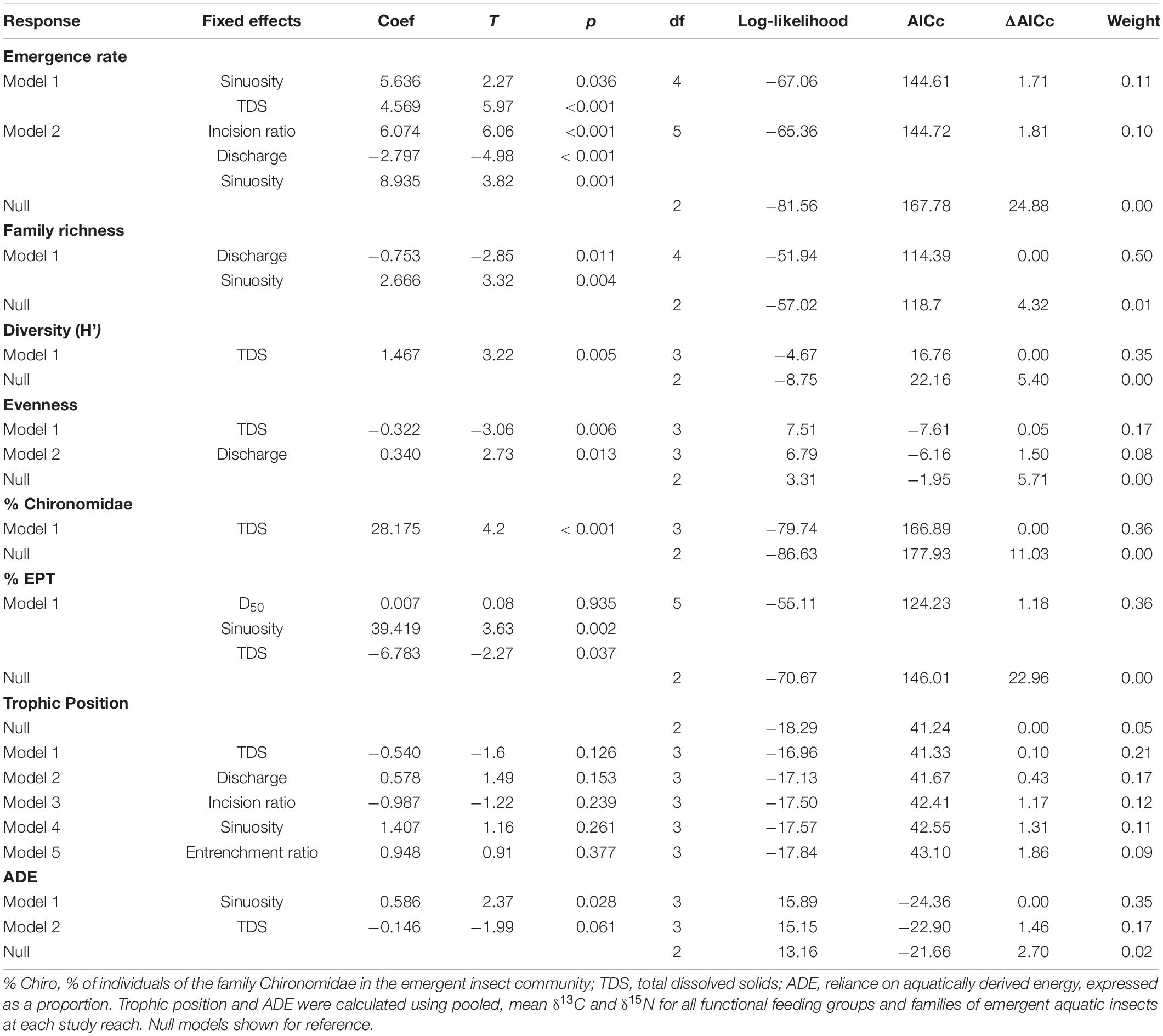
Table 2. Model results with AICc < 2 (excluding those with uninformative parameters) from general (normal distribution for evenness, % EPT, % Chironomidae, TP, and ADE) and generalized (lognormal distribution for emergence rate [no. m−2 day−1] and family richness; gamma for diversity [H’]) linear models with hydrogeomorphic characteristics as predictors of emergent aquatic insect flux metrics.
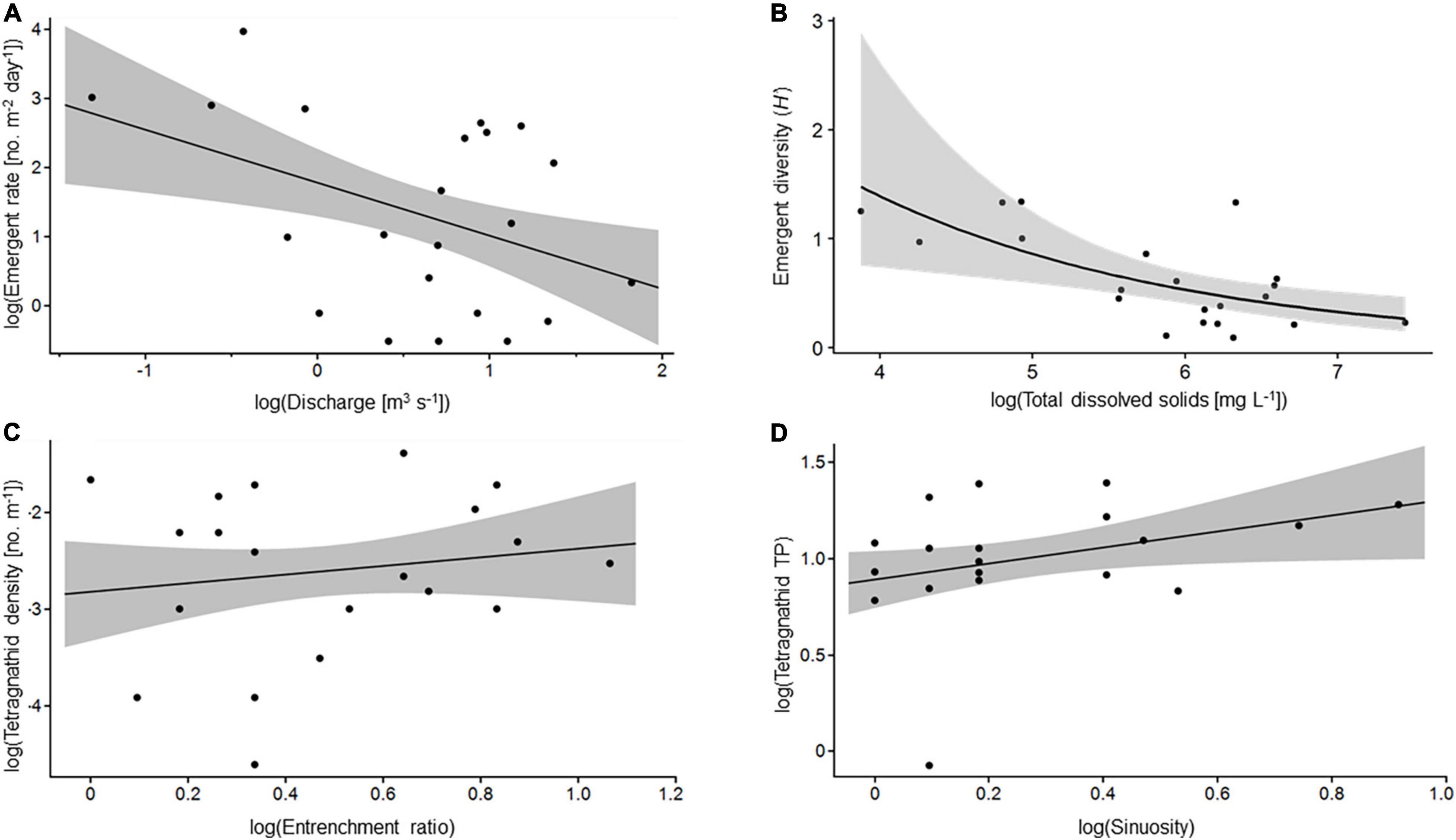
Figure 4. Example bivariate relationships from models presented in Tables 2, 3. (A) Log bankfull discharge and log emergent aquatic insect emergence rate. (B) Log total dissolved solids and emergent aquatic insect diversity (H’) on a Gamma scale. (C) Log entrenchment ratio and log tetragnathid density. (D) Log sinuosity and log tetragnathid trophic position. Shaded area indicates 95% confidence interval.
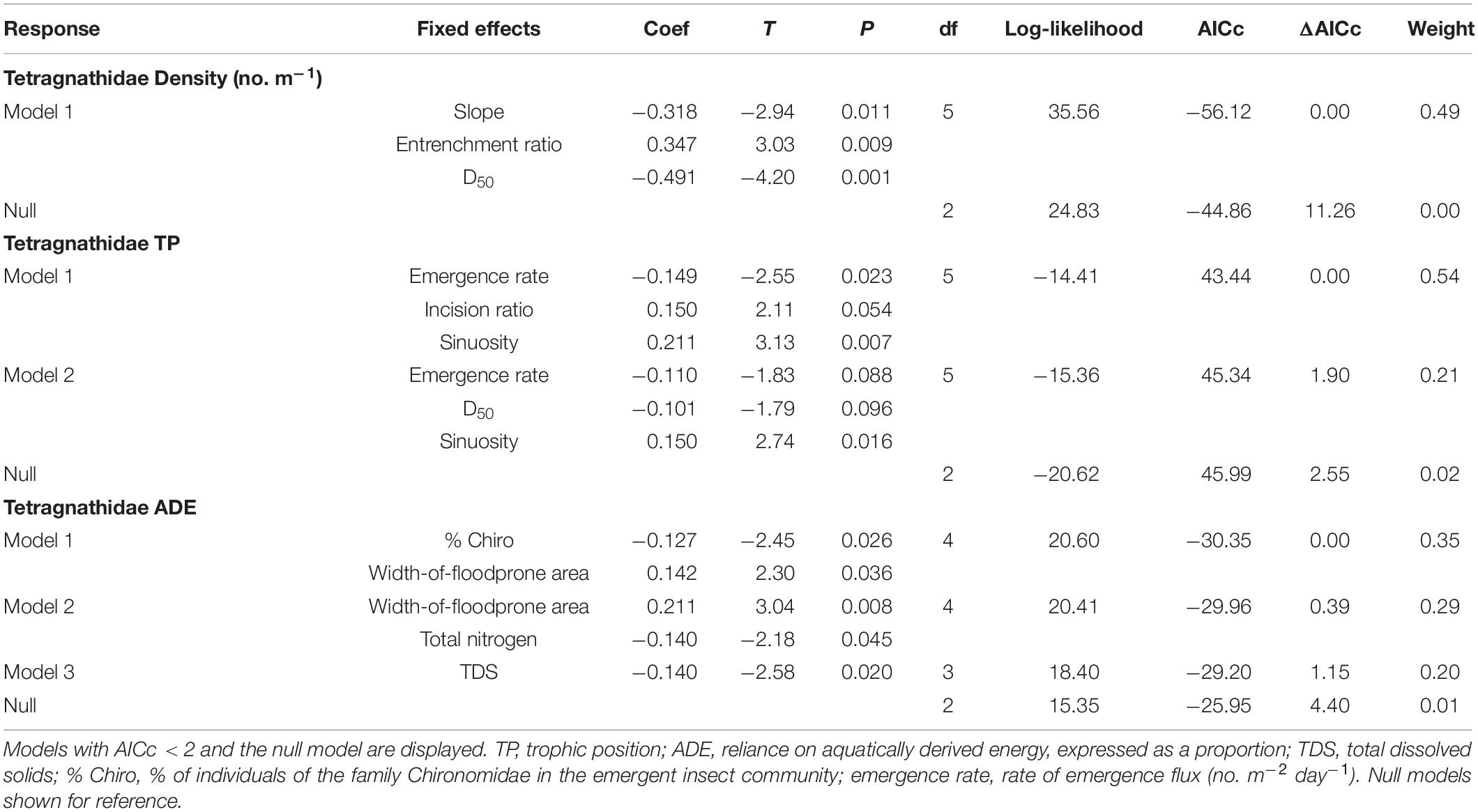
Table 3. Model results with AICc < 2 (excluding those with uninformative parameters) from generalized linear models (using lognormal distributions) for tetragnathid spider characteristics with hydrogeomorphic, emergent aquatic insect, riparian vegetation, and chemical water-quality characteristics as predictor variables.
Predictors of Riparian Spiders
One model of tetragnathid density, which included slope and D50 (negative relationships) and entrenchment ratio (positive relationship; Figure 4C) had strong (i.e., ΔAICc < 2) support after removing those with uninformative parameters (Table 3). Two models of Tetragnathidae TP also had strong support, and both featured combinations of emergence rate (negative relationship), and sinuosity (positive relationship; Table 3 and Figure 4D). The best supported (ΔAICc = 0) model for Tetragnathidae ADE featured % Chironomidae (negative relationship) and width-of-floodprone area (positive relationship; Table 3). Other supported models for tetragnathid ADE featured the additional variables of total nitrogen and TDS (negative relationships; Table 3). Salient relationships among hydrogeomorphic characteristics, emergent aquatic insects, and tetragnathid spiders are summarized in Figure 5.
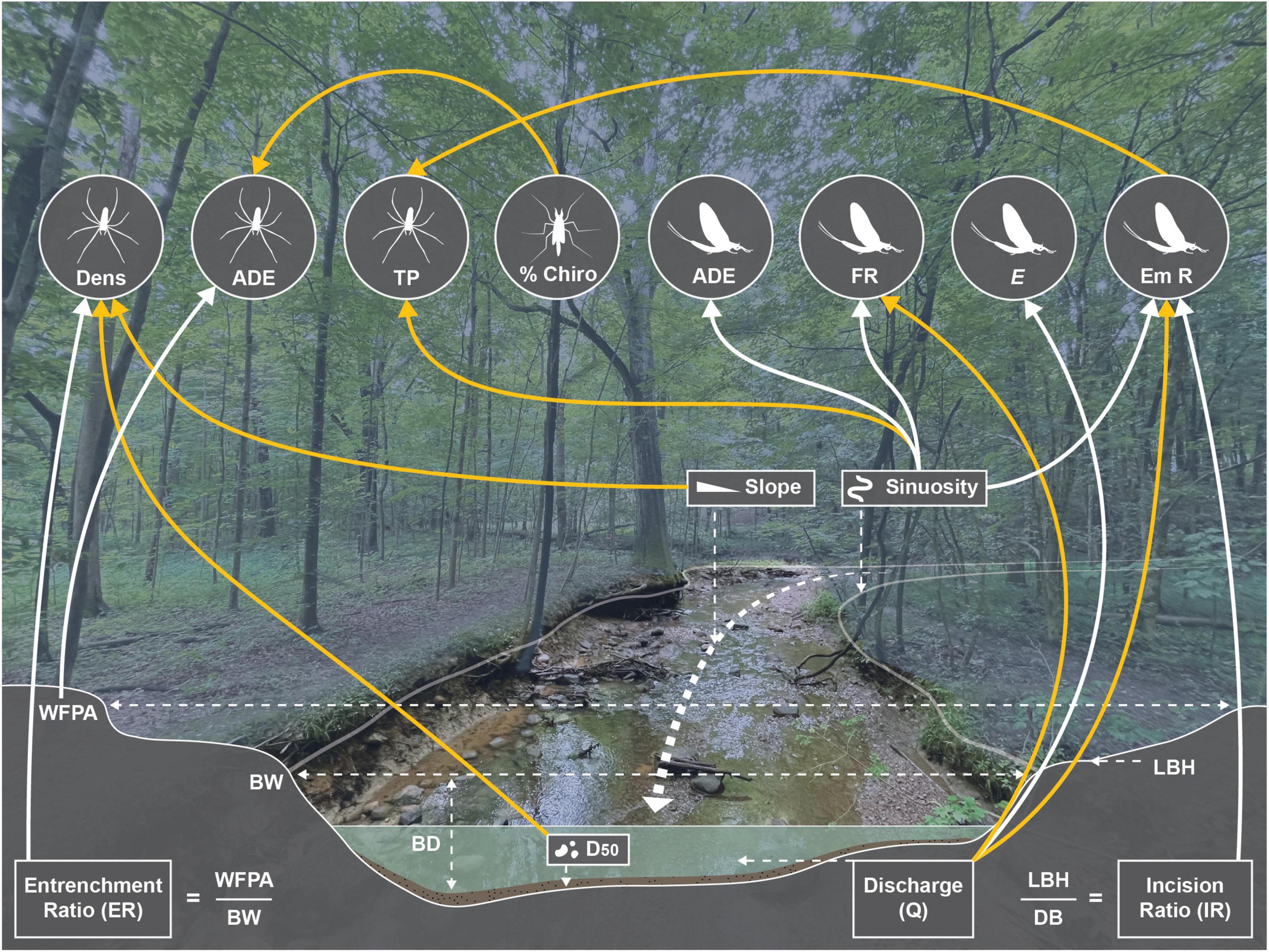
Figure 5. Visual representation of key relationships among hydrogeomorphology, emergent aquatic insects, and tetragnathid spiders. Relationships derived from AICc model results (Tables 2, 3). Yellow lines indicate negative relationships, black lines indicate positive relationships. Dens, density; ADE, reliance on aquatically derived energy; TP, trophic position; Chiro, Chironomidae; FR, family richness; E, evenness; Em R, emergence rate; WFPA, width of floodplain area; BW, bankfull width; LBH, low bank height; BD, bankfull depth; D50, median sediment particle size. Figure by R. B. Keast.
Discussion
We observed several associations between tetragnathid spiders and hydrogeomorphic characteristics of our urban streams, but with only limited evidence linking these shifts to the quantity and composition of the emergent aquatic insect flux into the riparian zone (Figure 5). Emergence rate was associated with a combination of hydrogeomorphic (i.e., discharge, sinuosity, and incision ratio) and chemical water-quality (i.e., TDS) variables. Emergent aquatic insect family richness was predominately influenced by discharge and sinuosity in the best supported model, while the remaining emergent aquatic insect metrics [i.e., diversity (H’), evenness, % Chironomidae, % EPT] were associated with TDS (although note that evenness was also positively associated with discharge and % EPT with sinuosity). Sinuosity was also a dominant factor in models of emergent aquatic insect ADE, although no variables were significant in models of emergent aquatic insect TP.
While predominately stream hydrogeomorphic factors influenced tetragnathid density (i.e., slope, entrenchment ratio, D50), a mixture of hydrogeomorphic (i.e., sinuosity, width-of-floodprone area) and emergent aquatic insect flux descriptors (i.e., emergence rate and % Chironomidae) best explained variability in tetragnathid trophic dynamics (though total N and TDS featured in some strongly supported models of tetragnathid ADE; Figure 5). These results imply that whereas hydrogeomorphic variability may directly influence the abundance of tetragnathid spiders along a stream reach, associations between hydrogeomorphic characteristics and spider trophic dynamics were primarily mediated by emergent aquatic insects. Positive relationships between emergent insect and tetragnathid reliance on aquatically derived energy further support this mechanism, as does the positive relationship between emergent insect and tetragnathid δ13C (Figure 3). While these correlations likely reflect underlying shifts in benthic insect communities, numerous studies have shown a decoupling between larval benthic insect densities and emergence rates in response to the allochthonous resource availability (Kraus and Vonesh, 2012), the presence or absence of predators (Pope et al., 2009; Wesner, 2013), canopy cover (Banks et al., 2007), and contamination (Wesner, 2019), among other factors. Chemical water-quality variables, most notably TDS, appear to also play an influential role in impacting both riparian spider and emergent aquatic insect assemblage structure and trophic dynamics in urban systems.
Emergent Aquatic Insect Flux
Canonical correspondence analysis indicated several strong relationships between specific emergent aquatic insect families and hydrogeomorphic alterations, of which the positive relationships between sinuosity and Hydropsychidae and Heptageniidae relative abundances were intriguing (Figures 2, 5), owing to the positive relationships we observed between sinuosity and tetragnathid TP and emergent aquatic insect emergence rate, family richness, ADE, and % EPT. Hydropsychid caddisflies are net-spinning filter-feeders (Merritt et al., 2008) that may benefit from increased sinuosity via increased terrestrial detrital material entering the stream (via increased interaction area with the riparian zone; Gratton and Vander Zanden, 2009). Heptageniid mayflies are flat-bodied scrapers (Merritt et al., 2008) that may be advantaged by the increased habitat heterogeneity often associated with more sinuous streams (Kauffman et al., 1997). Based on our results, these taxa might be expected to benefit from an increase in autochthonous resources in more sinuous reaches. An interesting avenue of future research may be to explore whether an increase in mayfly taxa as prey for predatory aquatic insects has an impact on coupled stream-riparian food-chain length and, thus, tetragnathid TP.
Increased shear stress and erosion resulting in altered energetic resources and physical habitat may underlie linkages between bankfull discharge and emergent aquatic insect variables. For example, our linear models indicated that increased bankfull discharge was associated with lower emergence rate, family richness, and, in a few models, slightly increased evenness of the emergent insect flux (Table 2 and Figure 4A). Bankfull flows are a principal agent of erosion in streams, responsible for high shear stress and scour (Ward and Trimble, 2004; Wohl et al., 2019). Enlarged urban streams may experience higher bankfull discharges than would be expected given their drainage area, and also experience them more frequently (Chin, 2006; Cianfrani et al., 2006; Vietz et al., 2016). Thus, these streams may support lower densities of benthic macroinvertebrates, with those taxa able to withstand high-flow environments (e.g., burrowers or strong clingers) becoming proportionally more abundant.
Total dissolved solids were positively related to emergent aquatic insect emergence rate, diversity (Figure 4B) and % Chironomidae, negatively related to % EPT, emergent aquatic insect evenness, and, in some models, ADE. TDS – particularly bicarbonate, calcium, and chloride – are commonly elevated in urban areas owing to urban runoff and, perhaps more importantly, leaching from concrete drainage structures (Garie and McIntosh, 1986; Wright et al., 2011; Tippler et al., 2014). Previous studies have documented shifts in aquatic macroinvertebrate assemblages stemming from urban-associated increases in TDS and, correspondingly, conductivity and pH (Chatzinikolaou et al., 2006; Mangadze et al., 2019). These assemblage changes may be the result of species-specific adaptations to extreme low or high TDS concentrations (Olson and Hawkins, 2017), potentially leading to increases in the relative abundance of taxa commonly associated with urban catchments (e.g., Gastropoda, Chironomidae, and Culicidae; Clark et al., 2004; Kefford et al., 2007; Herbst et al., 2019). Importantly, several studies have found that water chemistry, specifically TDS, can play at least as large a role as physical habitat factors in shaping macroinvertebrate communities (Chatzinikolaou et al., 2006; Mangadze et al., 2019).
Collectively, these results suggest that there is an elaborate set of hydrogeomorphic-, chemical-, and habitat-related environmental filters that controls the abundance and composition of emergent aquatic insect fluxes into the riparian zone. Thus, future research should delve further into mechanisms that target the interaction of multiple stressors on emergent aquatic insect assemblages in streams to parse out which factors to prioritize in restoring stream systems, and in particular relative to streams undergoing urbanization.
Riparian Tetragnathid Spiders
Tetragnathidae ADE ( = 0.36) was higher than that found in some studies in the region [e.g., 0.24 (large river; Tagwireyi and Sullivan, 2015); 0.16 (small streams; Sullivan et al., 2019)] though lower than that found in others ( = 0.50; large river; Kautza and Sullivan, 2016). TP ( = 2.85) was more similar to that found in similarly-sized systems in the region [e.g., 3.16 (large river; Tagwireyi and Sullivan, 2015) vs. 2.66 (small streams; Sullivan et al., 2019)].
Partially in line with our expectations, we observed that tetragnathid TP was positively associated with sinuosity and tetragnathid ADE was positively associated with width-of-floodprone area (Table 3 and Figure 5). Sinuosity typically decreases (resulting in a more channelized stream) as imperviousness increases (Booth and Jackson, 1997; Pizzuto et al., 2000; Cianfrani et al., 2006), which would also decrease the lateral area within the stream valley with which the stream interacts (i.e., decreasing width-of-floodprone area). The surface area available to interact with a recipient environment is critically important for determining the magnitude of a cross-ecosystem energetic flux (Gratton and Vander Zanden, 2009) and here may have directly impacted tetragnathid spiders’ ability to access instream energy resources.
Reduced sinuosity (i.e., channelization) is also often associated with increased habitat homogeneity (Eitzmann and Paukert, 2010), lower densities of aquatic macroinvertebrates (Kennedy and Turner, 2011), decreased aquatic-terrestrial fluxes of emergent aquatic insects, and reduced riparian spider density (Laeser et al., 2005; Kennedy and Turner, 2011). Here, we saw that emergent aquatic insect family richness, % EPT, ADE, and emergence rate all increased in concert with sinuosity, pointing to shifts in the emergent aquatic insect community as a likely mechanism underlying the observed increases in tetragnathid TP in response to increasing sinuosity (Figure 4D). Overall, we saw that sinuosity and width-of-floodprone area impact emergent aquatic insects and tetragnathid spiders directly via shifts in habitat quality and availability as well as by moderating the exchange of energetic resources between the terrestrial and aquatic environments. These results suggest that increased stream-floodplain physical connectivity (e.g., increased sinuosity, width-of-floodprone area; decreased entrenchment and incision ratios) may benefit tetragnathid spider assemblages (and likely other riparian consumers) by increasing aquatic-terrestrial energetic connectivity in the form of greater or more beneficial emergent aquatic insect subsidies.
Tetragnathid density was highest in greatest entrenched, low gradient, fine-grained stream reaches (Table 3 and Figure 4C). Low slopes, small median sediment particle sizes, and high entrenchment ratios are typical characteristics of impaired streams (Booth and Jackson, 1997; Pizzuto et al., 2000; Walsh et al., 2005). Slope was highly correlated with shear stress in our study reaches. Both metrics are normally good predictors of benthic invertebrate distributions (Statzner et al., 1988), taxonomic richness, and community composition (Merigoux and Doledec, 2004). Slope and shear stress also play a role in determining D50 (Ward and Trimble, 2004). D50 can strongly influence aquatic macroinvertebrate communities, with increasing D50 associated with an increase in hyporheic invertebrate taxa (Xu et al., 2012), while also playing some role in determining benthic invertebrate distributions, richness, and the relative proportion of functional feeding groups (e.g., filterer-collectors; Merigoux and Doledec, 2004). Although neither slope nor D50 was found to be strong a predictor of emergent aquatic insect assemblage attributes in our study, our CCA results show that several taxa (e.g., Phoridae, Heptageniidate, and Hydropsychidae) increased in relative abundance as D50 increased across our study reaches (Figure 2), suggesting the potential for subtle shifts in emergent aquatic insect assemblages that may have not been detectable at the taxonomic resolution used here. Tetragnathid density was likely highly influenced by indirect impacts of hydrogeomorphic alterations on emergent aquatic insect assemblages, most importantly the impact of increasing slope on shear stress (as a hydraulic stressor for macroinvertebrate assemblages) and D50 (as an indicator of benthic habitat quality).
Emergent Aquatic Insect – Tetragnathidae Relationships
Tetragnathid ADE’s negative association with % Chironomidae (Table 3 and Figure 5) is in line with results from similar studies (Gratton et al., 2008; Alberts and Sullivan, 2016). In addition, tetragnathid ADE tracked emergent aquatic insect ADE, and tetragnathid δ13C tracked emergent aquatic insect δ13C (Figure 3), indicating tetragnathid reliance on the flux of emergent aquatic insects as a nutritional resource. This trophic relationship is widely recognized (Kato et al., 2004; Speir et al., 2014; Tagwireyi and Sullivan, 2015). The reliance on emergent aquatic insects by spiders in our study system further supports a mechanism by which urban-induced hydrogeomorphic change (i.e., instream and riparian alterations) leads to altered tetragnathid riparian spider density and trophic dynamics via shifts in the emergent aquatic insect assemblages. Tetragnathid TP, however, did not display a strong relationship with emergent aquatic insect TP, perhaps due to the additional influence of stream hydrogeomorphology on terrestrial arthropods that may also be prey for tetragnathid spiders and that may also use aquatically derived resources (e.g., Paetzold et al., 2008; Albertson et al., 2018). This mechanism would suggest that stream hydrogeomorphology can impact riparian spiders through multiple biotic pathways. Future work should investigate this hypothesis using terrestrial arthropod data collected from these or similar study reaches.
Both tetragnathid spider and emergent aquatic insect attributes shifted in response to similar hydrogeomorphic variables (Figure 5), with some mechanisms suggesting that tetragnathid responses are driven by shifts in aquatic-to-terrestrial prey subsidies. However, differences in tetragnathid density and emergent assemblage responses imply that tetragnathid assemblages are also heavily influenced by physical habitat variables. Alterations to stream-riparian food-web structure of urban streams appear to be multivariate and complex, with hydrogeomorphic change working through multiple avenues (e.g., altering energy flux, physical habitat) to affect the distribution, composition, and feeding habits of both instream and riparian biota. These findings have consequential implications for not just ecosystem stability and function of streams, but also contaminant export to terrestrial environments, as emergent aquatic insects are a major vector of contaminants, and can move into larger, more mobile consumers that can potentially transfer contaminants to uplands (Sullivan and Rodewald, 2012; Muehlbauer et al., 2014; Rowse et al., 2014).
The complexity of the interactions observed here may explain a previously observed weak relationship between fluvial geomorphic features and the aquatic-terrestrial transfer of contaminants in a subset of these stream reaches (Sullivan et al., 2016), as it was not solely emergent aquatic insects driving hydrogeomorphic-Tetragnathidae linkages, but also complex interactions with habitat quality and availability. Developing a better understanding of how catchment land use shapes these interactions may help develop management and restoration plans that address the complicated physicochemical-biotic alterations and feedbacks that have the potential to destabilize stream ecosystems and impair environmental quality. Incorporating rapid geomorphic assessments (e.g., Sullivan et al., 2006; Sullivan, 2012) that evaluate fluvial geomorphic condition, including stream-floodplain connectivity and expanding macroinvertebrate assessments to include emergent aquatic insects may yield better information on the functioning of impaired stream systems and provide an improved understanding of avenues toward stream and riparian restoration, especially in urban catchments.
Data Availability Statement
The datasets generated and analyzed for this study can be found in the Figshare repository at https://figshare.com/articles/dataset/Aquatic-Terrestrial_Urban_Data/16797643.
Author Contributions
LOR conceived of the analysis, collated and analyzed the data, and wrote the manuscript. SMPS conceived of the analysis, secured funding to support the analysis, contributed to analyzing the data sets, and wrote the manuscript. Both authors developed the experimental design and contributed to the article.
Funding
This work was supported by The Ohio State University (through state and federal funds), Ohio Agricultural Research and Development Center, Ohio Department of Natural Resources, Division of Wildlife (USFWS State Wildlife Grant Program), and Ohio Biodiversity Conservation Partnership (SMPS) provided research support.
Conflict of Interest
The authors declare that the research was conducted in the absence of any commercial or financial relationships that could be construed as a potential conflict of interest.
Publisher’s Note
All claims expressed in this article are solely those of the authors and do not necessarily represent those of their affiliated organizations, or those of the publisher, the editors and the reviewers. Any product that may be evaluated in this article, or claim that may be made by its manufacturer, is not guaranteed or endorsed by the publisher.
Acknowledgments
We want to thank members of the Stream and River Ecology (STRIVE) Laboratory, in particular Kristen Diesburg, Lars Meyer, Danielle Cook, and Lindsey Boaz, for assistance in both the field and lab, and Jason Bohenek for his statistical and modeling assistance.
Supplementary Material
The Supplementary Material for this article can be found online at: https://www.frontiersin.org/articles/10.3389/fevo.2022.817289/full#supplementary-material
References
Akamatsu, F., Toda, H., and Okino, T. (2004). Food source of riparian spiders analyzed by using stable isotope ratios. Ecol. Res. 19, 655–662. doi: 10.1111/j.1440-1703.2004.00680.x
Alberts, J. M., and Sullivan, S. M. P. (2016). Factors influencing aquatic-to-terrestrial contaminant transport to terrestrial arthropod consumers in a multiuse river system. Environ. Pollut. 213, 53–62. doi: 10.1016/j.envpol.2016.02.003
Albertson, L. K., Ouellet, V., and Daniels, M. D. (2018). Impacts of stream riparian buffer land use on water temperature and food availability for fish. J. Freshwater Ecol. 33, 195–210. doi: 10.1080/02705060.2017.1422558
Arnold, T. W. (2010). Uninformative parameters and model selection using Akaike’s information criterion. J. Wildlife Manag. 74, 1175–1178. doi: 10.1111/j.1937-2817.2010.tb01236.x
Banks, J. L., Li, J., and Herlihy, A. T. (2007). Influence of clearcut logging, flow duration, and season on emergent aquatic insects in headwater streams of the Central Oregon Coast Range. J. North Am. Benthol. Soc. 26, 620–632. doi: 10.1899/06-104.1
Bartón, K. (2020). MuMIn: Multi-Model Inference. R package version 1.43.17. Available online at: https://CRAN.R-project.org/package=MuMIn.
Bastow, J. L., Sabo, J. L., Finlay, J. C., and Power, M. E. (2002). A basal aquatic-terrestrial trophic link in rivers: algal subsidies via shore-dwelling grasshoppers. Oecologia 131, 261–268. doi: 10.1007/s00442-002-0879-877
Baxter, C. V., Fausch, K. D., and Saunders, W. C. (2005). Tangled webs: reciprocal flows of invertebrate prey link streams and riparian zones. Freshwater Biol. 50, 201–220. doi: 10.1111/j.1365-2427.2004.01328.x
Baxter, C. V., Fausch, K. D., Murakami, M., and Chapman, P. L. (2004). Fish invasion restructures stream and forest food webs by interrupting reciprocal prey subsidies. Ecology 85, 2656–2663. doi: 10.1890/04-138
Bledsoe, B. P., and Watson, C. C. (2001). Effects of urbanization on channel instability. J. Am. Water Resources Assoc. 37, 255–270. doi: 10.1111/j.1752-1688.2001.tb00966.x
Booth, D. B., and Jackson, C. R. (1997). Urbanization of aquatic systems: degradation thresholds, stormwater detection, and the limits of mitigation. J. Am. Water Resources Assoc. 33, 1077–1090. doi: 10.1111/j.1752-1688.1997.tb04126.x
Burdon, F. J., and Harding, J. S. (2008). The linkage between riparian predators and aquatic insects across a stream-resource spectrum. Freshwater Biol. 53, 330–346. doi: 10.1111/j.1365-2427.2007.01897.x
Cabana, G., and Rasmussen, J. B. (1996). Comparison of aquatic food chains using nitrogen isotopes. Proc. Natl. Acad. Sci. U S A. 93, 10844–10847. doi: 10.1073/pnas.93.20.10844
Caut, S., Angulo, E., and Courchamp, F. (2009). Variation in discrimination factors (Delta N-15 and Delta C-13): the effect of diet isotopic values and applications for diet reconstruction. J. Appl. Ecol. 46, 443–453. doi: 10.1111/j.1365-2664.2009.01620.x
Chan, E. K. W., Zhang, Y. X., and Dudgeon, D. (2009). Substrate availability may be more important than aquatic insect abundance in the distribution of riparian orb-web spiders in the tropics. Biotropica 41, 196–201. doi: 10.1111/j.1744-7429.2008.00463.x
Chatzinikolaou, Y., Dakos, V., and Lazaridou, M. (2006). Longitudinal impacts of anthropogenic pressures on benthic macroinvertebrate assemblages in a large transboundary Mediterranean river during the low flow period. Acta Hydrochimica Hydrobiol. 34, 453–463. doi: 10.1002/aheh.200500644
Chin, A. (2006). Urban transformation of river landscapes in a global context. Geomorphology 79, 460–487. doi: 10.1016/j.geomorph.2006.06.033
Cianfrani, C. M., Hession, W. C., and Rizzo, D. M. (2006). Watershed imperviousness impacts on stream channel condition in southeastern Pennsylvania. J. Am. Water Resources Assoc. 42, 941–956. doi: 10.1111/j.1752-1688.2006.tb04506.x
Clark, T. M., Flis, B. J., and Remold, S. K. (2004). Differences in the effects of salinity on larval growth and developmental programs of a freshwater and a euryhaline mosquito species (Insecta: Diptera, Culicidae). J. Exp. Biol. 207, 2289–2295. doi: 10.1242/jeb.01018
Collier, K. J., Bury, S., and Gibbs, M. (2002). A stable isotope study of linkages between stream and terrestrial food webs through spider predation. Freshwater Biol. 47, 1651–1659. doi: 10.1046/j.1365-2427.2002.00903.x
Delignette-Muller, M. L., and Dutang, C. (2015). fitdistrplus: an R package for fitting distributions. J. Statistical Software 64, 1–34.
Delong, M. D., and Thorp, J. H. (2006). Significance of instream autotrophs in trophic dynamics of the Upper Mississippi River. Oecologia 147, 76–85. doi: 10.1007/s00442-005-0241-y
Diesburg, K. M., Sullivan, S. M. P., and Manning, D. W. P. (2021). Consequences of a terrestrial insect invader on stream-riparian food webs of the central Appalachians, USA. Biol. Invasions 23, 1263–1284. doi: 10.1007/s10530-020-02435-x
Doll, B. A., Wise-Frederick, D. E., Buckner, C. M., Wilkerson, S. D., Harman, W. A., Smith, R. E., et al. (2002). Hydraulic geometry relationships for urban streams throughout the piedmont of North Carolina. J. Am. Water Resources Assoc. 38, 641–651. doi: 10.1111/j.1752-1688.2002.tb00986.x
Eitzmann, J. L., and Paukert, C. P. (2010). Urbanization in a Great Plains river: effect on fishes and food webs. River Res. Appl. 26, 948–959. doi: 10.1002/rra.1326
Feld, C., Segurado, P., and Gutiérrez-Cánovas, T. (2016). Analysing the impact of multiple stressors in aquatic biomonitoring data: a ‘cookbook’ with applications in R. Sci. Total Environ. 573, 1320–1339. doi: 10.1016/j.scitotenv.2016.06.243
Fend, S. V., Carter, J. L., and Kearns, F. R. (2005). Relationships of field habitat measurements, visual habitat indices, and land cover to benthic macroinvertebrates in urbanized streams of the Santa Clara Valley, California. Effects Urbanization Stream Ecosystems 47, 193–212.
Finkenbine, J. K., Atwater, J. W., and Mavinic, D. S. (2000). Stream health after urbanization. J. Am. Water Resources Assoc. 36, 1149–1160. doi: 10.1111/j.1752-1688.2000.tb05717.x
Fukui, D., Murakami, M., Nakano, S., and Aoi, T. (2006). Effect of emergent aquatic insects on bat foraging in a riparian forest. J. Animal Ecol. 75, 1252–1258. doi: 10.1111/j.1365-2656.2006.01146.x
Fuller, R. L., Griego, C., Muehlbauer, J. D., Dennison, J., and Doyle, M. W. (2010). Response of stream macroinvertebrates in flow refugia and high-scour areas to a series of floods: a reciprocal replacement study. J. North Am. Benthol. Soc. 29, 750–760. doi: 10.1899/09-107.1
Garie, H. L., and McIntosh, A. (1986). Distribution of benthic macroinvertebrates in a stream exposed to urban runoff. Water Resources Bull. 22, 447–455. doi: 10.1111/j.1752-1688.1986.tb01899.x
Gergs, R., Koester, M., Schulz, R. S., and Schulz, R. (2014). Potential alteration of cross-ecosystem resource subsidies by an invasive aquatic macroinvertebrate: implications for the terrestrial food web. Freshwater Biol. 59, 2645–2655. doi: 10.1111/fwb.12463
Gratton, C., and Vander Zanden, M. J. (2009). Flux of aquatic insect productivity to land: comparison of lentic and lotic ecosystems. Ecology 90, 2689–2699. doi: 10.1890/08-1546.1
Gratton, C., Donaldson, J., and vander Zanden, M. J. (2008). Ecosystem linkages between lakes and the surrounding terrestrial landscape in northeast Iceland. Ecosystems 11, 764–774. doi: 10.1007/s10021-008-9158-9158
Greenacre, M. J. (1984). Theory and Applications of Correspondence Analysis. London: Academic Press.
Greenwood, M. J., and Booker, D. J. (2016). Influence of hydrological regime and land cover on traits and potential export capacity of adult aquatic insects from river channels. Oecologia 180, 551–566. doi: 10.1007/s00442-015-3462-3468
Greenwood, M. J., and McIntosh, A. R. (2008). Flooding impacts on responses of a riparian consumer to cross-ecosystem subsidies. Ecology 89, 1489–1496. doi: 10.1890/07-0749.1
Greenwood, M. J., and McIntosh, A. R. (2010). Low river flow alters the biomass and population structure of a riparian predatory invertebrate. Freshwater Biol. 55, 2062–2076. doi: 10.1111/j.1365-2427.2010.02462.x
Groffman, P. M., Bain, D. J., Band, L. E., Belt, K. T., Brush, G. S., Grove, J. M., et al. (2003). Down by the riverside: Urban riparian ecology. Front. Ecol. Environ. 1:315–321. doi: 10.2307/3868092
Hanson, B. J., Cummins, K. W., Cargill, A. S., and Lowry, R. R. (1985). Lipid-content, fatty-acid composition, and the effect of diet on fats of aquatic insects. Comp. Biochem. Physiol. B-Biochem. Mol. Biol. 80, 257–276. doi: 10.1016/0305-0491(85)90206-90208
Hardison, E. C., O’Driscoll, M. A., DeLoatch, J. P., Howard, R. J., and Brinson, M. M. (2009). Urban land use, channel incision, and water table decline along Coastal Plain streams, North Carolina. J. Am. Water Resources Assoc. 45, 1032–1046. doi: 10.1111/j.1752-1688.2009.00345.x
Hassett, B. A., Sudduth, E. B., Somers, K. A., Urban, D. L., Violin, C. R., Wang, S. Y., et al. (2018). Pulling apart the urbanization axis: patterns of physiochemical degradation and biological response across stream ecosystems. Freshwater Sci. 37, 653–672. doi: 10.1086/699387
Hawley, R. J., Bledsoe, B. P., Stein, E. D., and Haines, B. E. (2012). Channel evolution model of semiarid stream response to urban-induced hydromodification. J. Am. Water Resources Assoc. 48, 722–744. doi: 10.1111/j.1752-1688.2012.00645.x
Herbst, D. B., Cooper, S. D., Medhurst, R. B., Wiseman, S. W., and Hunsaker, C. T. (2019). Drought ecohydrology alters the structure and function of benthic invertebrate communities in mountain streams. Freshwater Biol. 39:L10401 doi: 10.1111/fwb.13270
Homer, C. G., Dewitz, J. A., Yang, L., Jin, S., Danielson, P., Xian, G., et al. (2015). Completion of the 2011 National Land Cover Database for the conterminous United States - representing a decade of land cover change information. Photogrammetric Eng. Remote Sensing 81, 345–354.
Hussey, N. E., MacNeil, M. A., McMeans, B. C., Olin, J. A., Dudley, S. F. J., Cliff, G., et al. (2014). Rescaling the trophic structure of marine food webs. Ecol. Lett. 17, 239–250. doi: 10.1111/ele.12226
Iwata, T., Nakano, S., and Murakami, M. (2003). Stream meanders increase insectivorous bird abundance in riparian deciduous forests. Ecography 26, 325–337. doi: 10.1034/j.1600-0587.2003.03355.x
Jackson, B. K., and Sullivan, S. M. P. (2009). Influence of wildfire severity on riparian plant community heterogeneity in an Idaho, USA wilderness. Forest Ecol. Manag. 259, 24–32. doi: 10.1016/j.foreco.2009.09.036
Jackson, B. K., and Sullivan, S. M. P. (2018). Ecosystem size and flooding drive trophic dynamics of riparian spiders in a fire-prone Sierra Nevada river system. Canadian J. Fish. Aquatic Sci. 75, 308–318. doi: 10.1139/cjfas-2017-2045
Kang, R. S., Storm, D. E., and Marston, R. A. (2010). Downstream effects of urbanization on Stillwater Creek, Oklahoma. Phys. Geography 31, 186–201. doi: 10.2747/0272-3646.31.2.186
Kato, C., Iwata, T., and Wada, E. (2004). Prey use by web-building spiders: stable isotope analyses of trophic flow at a forest-stream ecotone. Ecol. Res. 19, 633–643. doi: 10.1111/j.1440-1703.2004.00678.x
Kato, C., Iwata, T., Nakano, S., and Kishi, D. (2003). Dynamics of aquatic insect flux affects distribution of riparian web-building spiders. Oikos 103, 113–120. doi: 10.1034/j.1600-0706.2003.12477.x
Kauffman, J. B., Beschta, R. L., Otting, N., and Lytjen, D. (1997). An ecological perspective of riparian and stream restoration in the western United States. Fisheries 22, 12–24. doi: 10.1577/1548-8446(1997)022<0012:aepora>2.0.co;2
Kautza, A., and Sullivan, S. M. P. (2015). Shifts in reciprocal river-riparian arthropod fluxes along an urban-rural landscape gradient. Freshwater Biol. 60, 2156–2168. doi: 10.1111/fwb.12642
Kautza, A., and Sullivan, S. M. P. (2016). The energetic contributions of aquatic primary producers to terrestrial food webs in a mid-size river system. Ecology 97, 694–705. doi: 10.1890/15-1095.1
Kefford, B. J., Nugegoda, D., Zalizniak, L., Fields, E. J., and Hassell, K. L. (2007). The salinity tolerance of freshwater macroinvertebrate eggs and hatchlings in comparison to their older life-stages: a diversity of responses. Aquatic Ecol. 41, 335–348. doi: 10.1007/s10452-006-9066-y
Kelly, S. P., Cuevas, E., and Ramírez, A. (2015). Stable isotope analyses of web-spinning spider assemblages along a headwater stream in Puerto Rico. PeerJ 3:e1324. doi: 10.7717/peerj.1324
Kennedy, T. L., and Turner, T. F. (2011). River channelization reduces nutrient flow and macroinvertebrate diversity at the aquatic terrestrial transition zone. Ecosphere 2:35. doi: 10.1890/es11-00047.1
Kondolf, G. M., and Larson, M. (1995). Historical channel analysis and its application to riparian and aquatic habitat restoration. Aquatic Conservation-Mar. Freshwater Ecosystems 5, 109–126. doi: 10.1002/aqc.3270050204
Kondolf, G. M., and Micheli, E. R. (1995). Evaluating stream restoration projects. Environ. Manag. 19, 1–15. doi: 10.1007/bf02471999
Kraus, J. M., and Vonesh, J. R. (2012). Fluxes of terrestrial and aquatic carbon by emergent mosquitoes: a test of controls and implications for cross-ecosystem linkages. Oecologia 170, 1111–1122. doi: 10.1007/s00442-012-2369-x
Krell, B., Roder, N., Link, M., Gergs, R., Entling, M. H., and Schafer, R. B. (2015). Aquatic prey subsidies to riparian spiders in a stream with different land use types. Limnologica 51, 1–7. doi: 10.1016/j.limno.2014.10.001
Laeser, S. R., Baxter, C. V., and Fausch, K. D. (2005). Riparian vegetation loss, stream channelization, and web-weaving spiders in northern Japan. Ecol. Res. 20, 646–651. doi: 10.1007/s11284-005-0084-83
Lemmon, P. E. (1957). A new instrument for measuring forest overstory density. J. Forestry 55, 667–669.
Lind, L., Hasselquist, E. M., and Laudona, H. (2019). Towards ecologically functional riparian zones: a meta-analysis to develop guidelines for protecting ecosystem functions and biodiversity in agricultural landscapes. J. Environ. Manage. 249:109391. doi: 10.1016/j.jenvman.2019.109391
Loomis, J. D., Cameron, G. N., and Uetz, G. W. (2014). Impact of the invasive shrub Lonicera maackii on shrub-dwelling Araneae in a deciduous forest in eastern North America. Am. Midland Natural. 171, 204–218. doi: 10.1674/0003-0031-171.2.204
Lorrain, A., Savoye, N., Chauvaud, L., Paulet, Y. M., and Naulet, N. (2003). Decarbonation and preservation method for the analysis of organic C and N contents and stable isotope ratios of low-carbonated suspended particulate material. Analytica Chimica Acta 491, 125–133. doi: 10.1016/s0003-2670(03)00815-818
Luo, K., Hu, X., He, Q., Wu, Z., Cheng, H., Hu, Z., et al. (2018). Impacts of rapid urbanization on the water quality and macroinvertebrate communities of streams: a case study in Liangjiang New Area, China. Sci. Total Environ. 621, 1601–1614. doi: 10.1016/j.scitotenv.2017.10.068
MacFarland, M. F. (2012). Determining Equilibrium Drivers in Central Ohio Urban Streams. Master of Science thesis, Columbus, OH: The Ohio State University.
Malison, R. L., Benjamin, J. R., and Baxter, C. V. (2010). Measuring adult insect emergence from streams: the influence of trap placement and a comparison with benthic sampling. J. North Am. Benthol. Soc. 29, 647–656. doi: 10.1899/09-086.1
Mangadze, T., Wasserman, R. J., Froneman, P. W., and Dalu, T. (2019). Macroinvertebrate functional feeding group alterations in response to habitat degradation of headwater Austral streams. Sci. Total Environ. 695:133910. doi: 10.1016/j.scitotenv.2019.133910
McNeish, R. E., Moore, E. M., Benbow, M. E., and McEwan, R. W. (2015). Removal of the invasive shrub, Lonicera maackii, from riparian forests influences headwater stream biota and ecosystem function. River Res. Appl. 31, 1131–1139. doi: 10.1002/rra.2808
Merigoux, S., and Doledec, S. (2004). Hydraulic requirements of stream communities: a case study on invertebrates. Freshwater Biol. 49, 600–613. doi: 10.1111/j.1365-2427.2004.01214.x
Merritt, R. W., Cummins, K. W., and Berg, M. B. (2008). An Introduction to the Aquatic Macroinvertebrates of North America. Dubuque, IA: Kendall-Hunt Publishing.
Muehlbauer, J. D., Collins, S. F., Doyle, M. W., and Tockner, K. (2014). How wide is a stream? spatial extent of the potential “stream signature” in terrestrial food webs using meta-analysis. Ecology 95, 44–55. doi: 10.1890/12-1628.1
Mundie, J. H. (1956). Emergence traps for aquatic insects. Internationale Vereinigung für Theoretische und Angewandte Limnologie: Mitteilungen 7, 1–13. doi: 10.1080/01650428309361115
O’Driscoll, M., Clinton, S., Jefferson, A., Manda, A., and McMillan, S. (2010). Urbanization effects on watershed hydrology and in-stream processes in the Southern United States. Water 2, 605–648. doi: 10.3390/w2030605
Oksanen, J., Blanchet, F. G., Friendly, M., Kindt, R., Legendre, P., McGlinn, D., et al. (2019). vegan: Community Ecology Package Version 2.5-4. Available online at: https://CRAN.R-project.org/package=vegan. (accessed 6 May, 2019).
Olson, J. R., and Hawkins, C. P. (2017). Effects of total dissolved solids on growth and mortality predict distributions of stream macroinvertebrates. Freshwater Biol. 62, 779–791. doi: 10.1111/fwb.12901
Paetzold, A., Bernet, J. F., and Tockner, K. (2006). Consumer-specific responses to riverine subsidy pulses in a riparian arthropod assemblage. Freshwater Biol. 51, 1103–1115. doi: 10.1111/j.1365-2427.2006.01559.x
Paetzold, A., Smith, M., Warren, P. H., and Maltby, L. (2011). Environmental impact propagated by cross-system subsidy: Chronic stream pollution controls riparian spider populations. Ecology 92, 1711–1716. doi: 10.1890/10-2184.1
Paetzold, A., Yoshimura, C., and Tockner, K. (2008). Riparian arthropod responses to flow regulation and river channelization. J. Appl. Ecol. 45, 894–903. doi: 10.1111/j.1365-2664.2008.01463.x
Pizzuto, J. E., Hession, W. C., and McBride, M. (2000). Comparing gravel-bed rivers in paired urban and rural catchments of southeastern Pennsylvania. Geology 28, 79–82. doi: 10.1130/0091-7613(2000)028<0079:cgbrip>2.3.co;2
Pope, K. L., Piovia-Scott, J., and Lawler, S. P. (2009). Changes in aquatic insect emergence in response to whole-lake experimental manipulations of introduced trout. Freshwater Biol. 54, 982–993. doi: 10.1111/j.1365-2427.2008.02145.x
Post, D. M. (2002). Using stable isotopes to estimate trophic position: models, methods, and assumptions. Ecology 83, 703–718. doi: 10.1111/j.1095-8649.2012.03251.x
Powell, G. E., Mecklenburg, D. E., and Ward, A. D. (2006). Spreadsheet Tools for River Evaluation, Assessment and Monitoring: The STREAM Diagnostic Modules. Available online at: http://www.dnr.state.oh.us/defauly/tabid/9188/Default.aspx. (accessed 3 February, 2012)
Richardson, J. S., Zhang, Y. X., and Marczak, L. B. (2010). Resource subsidies across the land-freshwater interface and responses in recipient communities. River Res. Appl. 26, 55–66. doi: 10.1002/rra.1283
Rieck, L. O., and Sullivan, S. M. P. (2020). Coupled fish-hydrogeomorphic responses to urbanization in streams of Columbus. Ohio. PLoS One 15:e0234303. doi: 10.1371/journal.pone.0234303
Rowse, L. M., Rodewald, A. D., and Sullivan, S. M. P. (2014). Pathways and consequences of contaminant flux to Acadian flycatchers (Empidonax virescens) in urbanizing landscapes of Ohio, USA. Sci. Total Environ. 485, 461–467. doi: 10.1016/j.scitotenv.2014.03.095
Sanzone, D. M., Meyer, J. L., Marti, E., Gardiner, E. P., Tank, J. L., and Grimm, N. B. (2003). Carbon and nitrogen transfer from a desert stream to riparian predators. Oecologia 134, 238–250. doi: 10.1007/s00442-002-1113-1113
Shewhart, L., McEwan, R. W., and Benbow, M. E. (2014). Evidence for facilitation of Culex pipiens (Diptera: Culicidae) life history traits by the nonnative invasive shrub Amur Honeysuckle (Lonicera maackii). Environ. Entomol. 43, 1584–1593. doi: 10.1603/en14183
Speir, S. L., Chumchal, M. M., Drenner, R. W., Cocke, W. G., Lewis, M. E., and Whitt, H. J. (2014). Methyl mercury and stable isotopes of nitrogen reveal that a terrestrial spider has a diet of emergent aquatic insects. Environ. Toxicol. Chem. 33, 2506–2509. doi: 10.1002/etc.2700
Statzner, B., Gore, J. A., and Resh, V. H. (1988). Hydraulic stream ecology – observed patterns and potential applications. J. North Am. Benthol. Soc. 7, 307–360. doi: 10.2307/1467296
Sterling, J. L., Rosemond, A. D., and Wenger, S. J. (2016). Watershed urbanization affects macroinvertebrate community structure and reduces biomass through similar pathways in Piedmont streams, Georgia, USA. Freshwater Sci. 35, 676–688. doi: 10.1086/686614
Stock, B. C., Jackson, A. L., Ward, E. J., Parnell, A. C., Phillips, D. L., and Semmens, B. X. (2018). Analyzing mixing systems using a new generation of Bayesian tracer mixing models. PeerJ 6:e5096. doi: 10.7717/peerj.5096
Stock, B., Semmens, B., Ward, E., Parnell, A., Jackson, A., and Phillips, D. (2020). MixSIAR: Bayesian Mixing Models in R. Available online at: https://CRAN.R-project.org/package=MixSIAR (accessed February 6, 2019).
Sullivan, S. M. P. (2012). Geomorphic-ecological relationships highly variable between headwater and network mountain streams of northern Idaho, United States. JAWRA J. Am. Water Resources Assoc. 48, 1221–1232. doi: 10.1111/j.1752-1688.2012.00682.x
Sullivan, S. M. P., and Rodewald, A. D. (2012). The energetic pathways that move contaminants from aquatic to terrestrial environments. Environ. Toxicol. Manag. 31, 1175–1183. doi: 10.1002/etc.1842
Sullivan, S. M. P., Boaz, L. E., and Hossler, K. (2016). Fluvial geomorphology and aquatic-to-terrestrial Hg export are weakly coupled in small urban streams of Columbus, Ohio. Water Resources Res. 52, 2822–2839. doi: 10.1002/2015wr018416
Sullivan, S. M. P., Corra, J. W., and Hayes, J. T. (2021). Urbanization mediates the effects of water quality and climate on a model aerial insectivorous bird. Ecol. Monographs 91:e01442. doi: 10.1002/ecm.1442
Sullivan, S. M. P., Hossler, K., and Meyer, L. A. (2019). Artificial lighting at night alters aquatic-riparian invertebrate food webs. Ecol. Appl. 29:e01821. doi: 10.1002/eap.1821
Sullivan, S. M. P., Manning, D. W. P., and Davis, R. P. (2018). Do the ecological impacts of dam removal extend across the aquatic-terrestrial boundary? Ecosphere 9:e02180. doi: 10.1002/ecs2.2180
Sullivan, S. M. P., Watzin, M. C., and Hession, W. C. (2004). Understanding stream geomorphic state in relation to ecological integrity: evidence using habitat assessments and macroinvertebrates. Environ. Manag. 34, 669–683. doi: 10.1007/s00267-004-4032-8
Sullivan, S. M. P., Watzin, M. C., and Hession, W. C. (2006). Influence of stream geomorphic condition on fish communities in Vermont, USA. Freshwater Biol. 51, 1811–1826. doi: 10.1111/j.1365-2427.2006.01616.x
Symonds, G. W. D. (1958). The Tree Identification Book: A New Method for Practical Identification and Recognition of Trees. New York, NY: William Morrow and Co.
Tagwireyi, P., and Sullivan, S. M. P. (2015). Distribution and trophic dynamics of riparian tetragnathid spiders in a large river system. Mar. Freshwater Res. 67, 309–318. doi: 10.1071/mf14335
Tippler, C., Wright, I. A., Davies, P. J., and Hanlon, A. (2014). The influence of concrete on the geochemical qualities of urban streams. Mar. Freshwater Res. 65, 1009–1017. doi: 10.1071/mf13164
Twining, C. W., Brenna, J. T., Lawrence, P., Shipley, J. R., Tollefson, T. N., and Winkler, D. W. (2016). Omega-3 long-chain polyunsaturated fatty acids support aerial insectivore performance more than food quantity. Proc. Natl. Acad. Sci. U S A. 113, 10920–10925. doi: 10.1073/pnas.1603998113
U.S. Geological Survey (2014). National Hydrography Dataset. Available online at: http://nhd.usgs.gov/. (Accessed 16 December, 2014)
U.S. Geological Survey (2016). The StreamStats Program for Ohio. Available online at: http://water.usgs.gov/osw/streamstats/ohio.html. (accessed 16 December, 2014)
Vanderklift, M. A., and Ponsard, S. (2003). Sources of variation in consumer-diet delta(15)N enrichment: a meta-analysis. Oecologia 136, 169–182. doi: 10.1007/s00442-003-1270-z
Vietz, G. J., Walsh, C. J., and Fletcher, T. D. (2016). Urban hydrogeomorphology and the urban stream syndrome: treating the symptoms and causes of geomorphic change. Prog. Phys. Geography 40, 480–492. doi: 10.1177/0309133315605048
Walsh, C. J., Fletcher, T. D., and Burns, M. J. (2012). Urban stormwater runoff: a new class of environmental flow problem. PLoS One 7:e45814. doi: 10.1371/journal.pone.0045814
Walsh, C. J., Roy, A. H., Feminella, J. W., Cottingham, P. D., Groffman, P. M., and Morgan, R. P. (2005). The urban stream syndrome: current knowledge and the search for a cure. J. North Am. Benthol. Soc. 24, 706–723.
Walters, D. M., Mills, M. A., Fritz, K. M., and Raikow, D. F. (2010). Spider-mediated flux of PCBs from contaminated sediments to terrestrial ecosystems and potential risks to arachnivorous birds. Environ. Sci. Technol. 44, 2849–2856. doi: 10.1021/es9023139
Wang, L. H., and Kanehl, P. (2003). Influences of watershed urbanization and instream habitat on macroinvertebrates in cold water streams. J. Am. Water Resources Assoc. 39, 1181–1196. doi: 10.1111/j.1752-1688.2003.tb03701.x
Watling, J. I., Hickman, C. R., Lee, E., Wang, K., and Orrock, J. L. (2011). Extracts of the invasive shrub Lonicera maackii increase mortality and alter behavior of amphibian larvae. Oecologia 165, 153–159. doi: 10.1007/s00442-010-1777-z
Weber, A. M., Bauer, J. E., and Watters, G. T. (2017). Assessment of nutritional subsidies to freshwater mussels using a multiple natural abundance isotope approach. Freshwater Biol. 62, 615–629. doi: 10.1111/fwb.12890
Wesner, J. (2019). Using stage-structured food webs to assess the effects of contaminants and predators on aquatic–terrestrial linkages. Freshwater Sci. 38, 928–935. doi: 10.1086/706103
Wesner, J. S. (2013). Fish predation alters benthic, but not emerging, insects across whole pools of an intermittent stream. Freshwater Sci. 32, 438–449. doi: 10.1899/12-124.1
Williams, D. D., Ambrose, L. G., and Browning, L. N. (1995). Trophic dynamics of two sympatric species of riparian spider (Araneae: Tetragnathidae). Canadian J. Zool. 73, 1545–1553. doi: 10.1139/z95-183
Wohl, E., Brierley, G., Cadol, D., Coulthard, T. J., Covino, T., Fryirs, K. A., et al. (2019). Connectivity as an emergent property of geomorphic systems. Earth Surface Processes Landforms 44, 4–26. doi: 10.1002/esp.4434
Wolman, M. G. (1954). A method of sampling coarse river-bed material. Trans. Am. Geophys. Union 35, 951–956.
Wright, I. A., Davies, P. J., Findlay, S. J., and Jonasson, O. J. (2011). A new type of water pollution: concrete drainage infrastructure and geochemical contamination of urban waters. Mar. Freshwater Res. 62, 1355–1361. doi: 10.1071/mf10296
Xu, M., Wang, Z., Pan, B., and Zhao, N. (2012). Distribution and species composition of macroinvertebrates in the hyporheic zone of bed sediment. Int. J. Sediment Res. 27, 129–140. doi: 10.1016/s1001-6279(12)60022-5
Keywords: urban streams, stable isotopes, aquatic-terrestrial connectivity, fluvial geomorphology, Tetragnathidae, emergent aquatic insects
Citation: Rieck LO and Sulliván SMP (2022) Ecological Impacts of Altered Stream Hydrogeomorphic Characteristics Extend Beyond the Channel Boundary: Evidence From Urban Streams of Columbus, OH, United States. Front. Ecol. Evol. 10:817289. doi: 10.3389/fevo.2022.817289
Received: 17 November 2021; Accepted: 07 February 2022;
Published: 15 March 2022.
Edited by:
Jeff Wesner, University of South Dakota, United StatesReviewed by:
Jeffrey Muehlbauer, United States Geological Survey (USGS), United StatesFernando Nardi, University for Foreigners of Perugia, Italy
Copyright © 2022 Rieck and Sulliván. This is an open-access article distributed under the terms of the Creative Commons Attribution License (CC BY). The use, distribution or reproduction in other forums is permitted, provided the original author(s) and the copyright owner(s) are credited and that the original publication in this journal is cited, in accordance with accepted academic practice. No use, distribution or reproduction is permitted which does not comply with these terms.
*Correspondence: Leslie O. Rieck, cmllY2tAbHljb21pbmcuZWR1
†ORCID: Leslie O. Rieck, orcid.org/0000-0003-0378-5256; S. Mažeika Patricio Sulliván, orcid.org/0000-0003-2341-5316