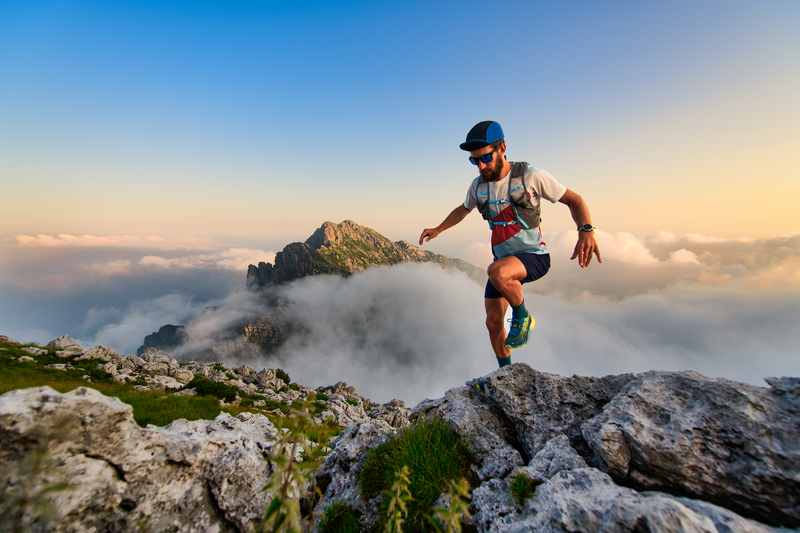
95% of researchers rate our articles as excellent or good
Learn more about the work of our research integrity team to safeguard the quality of each article we publish.
Find out more
ORIGINAL RESEARCH article
Front. Ecol. Evol. , 10 February 2022
Sec. Paleoecology
Volume 10 - 2022 | https://doi.org/10.3389/fevo.2022.810069
This article is part of the Research Topic Integrating Conservation Biology and Paleobiology to Manage Biodiversity and Ecosystems in a Changing World View all 22 articles
Despite growing concerns over global fisheries, the stock status of most commercially exploited species are poorly understood. Fossil data provide pre-anthropogenic baselines for data-poor fisheries, yet are underutilized in fisheries management. Here, we provide the first use of predation traces to assess the status of fisheries (crab). We compared crab predation traces on living individuals of the crab prey gastropod, Tegula funebralis, to Pleistocene individuals from the same regions in southern California. There were fewer crab predation traces on modern gastropods than their Pleistocene counterparts, revealing reductions in crab abundances today compared to the Pleistocene. We conclude that: (1) regardless of the cause, immediate actions are required to avoid further population reductions of commercially exploited crabs in southern California, (2) predation traces are a rapid, cost-effective method to assess otherwise data-poor fisheries, and (3) the inclusion of fossil data provides key new insights for modern resource and fisheries management.
Fisheries management is vital to ensure the sustainability of fisheries in the face of continued climate change and overfishing (Harley et al., 2006; Allison et al., 2009; Ekstrom et al., 2015), particularly for vulnerable coastal communities that are reliant on marine resources (Dolan and Walker, 2006; Cinner et al., 2012; Whitney and Ban, 2019). However, many exploited marine species remain understudied due to limited resources and information (Pilling et al., 2008; Wiedenmann et al., 2013; Baum and Fuller, 2016). Fossil and historical data are alternative sources that can be used to determine the extent of change due to human activities, as well as to establish pre-anthropogenic baselines (Dietl and Flessa, 2011; Mcclenachan et al., 2012; Kidwell and Tomasovych, 2013; Rick and Lockwood, 2013; Dietl et al., 2015; Kidwell, 2015; Barnosky et al., 2017; Fordham et al., 2020), but their use as a tool for fisheries management is still largely unimplemented (Jackson and Sala, 2001; Lotze et al., 2011; Toniello et al., 2019). In particular, predation scars on prey (known as repair scars) are an unused resource that can be used to infer predator abundance in both modern and fossil settings (Schindler et al., 1994; Molinaro et al., 2014; Stafford et al., 2015b), with large datasets that can be collected quickly and easily. By comparing predation traces on a common prey item from similar fossil and modern environments, we demonstrate the utility of fossil data and predation traces as alternative, cost-effective methods to determine the current status of exploited predator species.
Along the west coast of North America, cancrid crab species, such as Dungeness (Metacarcinus magister) and rock crabs (Cancer productus, Romaleon antennarium, Metacarcinus anthonyi), are important fisheries, valued at over $230 million USD in the United States in 2019 ($54 million in California) (NOAA Fisheries Landings, 2019). First-hand accounts suggest that overfishing is already affecting multispecies rock crab populations and body sizes in southern California (Fitzgerald et al., 2018, 2019). However, management of these fisheries is hampered by a lack of data other than gross landings (Culver et al., 2010; NOAA Fisheries Landings, 2019). In California, permit numbers are restricted, and a catch size limit is in place, but the effectiveness of these restrictions is unknown (Culver et al., 2010). California’s crab species also face additional pressure from rapid development/urbanization of southern California’s coast (Woodring et al., 1946; Whitaker et al., 2010), ocean acidification (Bednaršek et al., 2020), harmful algal blooms (HABs) (Moore et al., 2019), and human exploitation for at least the past 12 – 13,000 years (Rick et al., 2014; Erlandson et al., 2015). Cancrids from the Pleistocene of southern California are known mostly from occasional chela (claws), which preserve more readily than the rest of the body (Menzies, 1951; Nations, 1975). Pleistocene species and their distributions are comparable to the present, consisting of the same major cancrid taxa, including Cancer productus, Metacarcinus anthonyi, M. gracilis, M. magister, Romaleon antennarium, and R. branneri (Menzies, 1951; Nations, 1975). The only notable difference is the presence of Glebocarcinus oregonensis (the pygmy rock crab) in the Pleistocene of southern California, which does not extend south of Santa Barbara today (Nations, 1975). Given that the shell-crushing crab taxa are similar between the Pleistocene and modern, and that it is unknown if repair scars allow differentiation between species of cancrid crabs, it is assumed that repair scars represent an overall cancrid crab signal that should appear similar between the Pleistocene and modern of southern California. Faced with a lack of current or historical data, limited fisheries management resources, and a generally poor body fossil record of crabs, alternative evidence is needed to guide the management of current crab stocks.
For both ecological and paleontological studies, predation traces on prey are often the only source of information to indicate the activity or presence of predators. Crab predation scars (repair scars) on mollusks have been used to assess patterns as broad as the development of antipredatory adaptations and mollusk diversity through time (Schindel et al., 1982; Vermeij, 1982c; Alexander and Dietl, 2003; Dietl et al., 2010; Mondal et al., 2014; Mondal and Harries, 2015), to variation in predation in modern ecosystems (Vermeij, 1982a,b; Schmidt, 1989; Schindler et al., 1994; Cadée et al., 1997; Alexander and Dietl, 2001; Dietl and Alexander, 2009; Molinaro et al., 2014; Stafford et al., 2015b). Predation traces also provide useful information on how crabs and their ecosystems are affected by environmental disturbances (Tyler et al., 2019). Most importantly for stock assessments, repair frequencies accurately track crab abundances in modern coastal studies (Schindler et al., 1994; Molinaro et al., 2014; Stafford et al., 2015b), especially when limited to repairs found on a single, common prey species.
However, comparing repair frequencies over evolutionary time scales in which selection for improved defenses or weaponry may have occurred, becomes more challenging. Because repairs are caused by failed attacks, a decline in repair frequency over time may be the result of either a decrease in predator success (more failures = more repairs) or an increase in predator attacks (more attacks at the same success rate = more repairs) (Vermeij, 1987); the reverse is also true. In studies of modern crab-mollusk predator-prey systems along crab population gradients from British Columbia, Canada (Molinaro et al., 2014; Stafford et al., 2015b) and Georgia, United States (Schindler et al., 1994), repair frequency on single prey species increased with crab abundance. Although not indicated in their study, data in Cadée et al. (1997) from the northern Gulf of California, Mexico, also supports this pattern. While the intent of these studies was to test whether repairs were a function of attack frequency, and predator abundance is just one of several mechanisms for changing attack frequency, the evidence demonstrates an association of repairs with crab abundance. As such, if a change in success can be excluded as an explanation for a change in repair frequency, then it is likely that the change in repairs is caused by a change in crab abundance.
To discern potential changes in predator success, Leighton (2002) suggested that prey size, particularly the size of the largest individuals attacked, could provide a means of distinguishing between success and attack frequency as causal mechanisms for changes in repair frequency; this approach was subsequently used in Richards and Leighton (2012) and Pruden et al. (2018). For example, if a prey species has not strengthened its defenses (e.g., increased shell thickness or ornament, etc.) over time, then larger, stronger predators would not only have an increased likelihood of success, but would also be able to take larger prey. The same pattern can and has also been considered from the perspective of the prey. For example, if prey improve their defenses, predator success and the size of prey predators are able to attack and/or the severity of the damage predators can inflict is likely to decrease over time (Mondal et al., 2014). Comparing prey size and repair size has also been used to determine potential prey size refugia from sublethal shell breakage (Alexander and Dietl, 2001).
For durophagous crabs, strength refers to the force delivered by the chelae. In all animal systems, force is proportional to the cross-sectional area of the muscle bundle, perpendicular to the line of action of the muscle. The chelae closer muscle in cancrids increases in size through ontogeny; thus, larger crabs are stronger crabs. In some crab-prey systems (e.g., the stone-crab, Menippe and its oyster prey), the crab is so strong relative to the prey that its success rate approaches 100%; in such systems, there is no size refuge for the prey, and no size selection on the part of the predator. However, for many specialized durophagous crab-prey systems, the success rate is much lower than 100% (as evidenced by the existence of repairs), and in such systems, crab size, strength, success, and the ability to handle larger prey are strongly associated (Hughes and Elner, 1979; Whetstone and Eversole, 1981; Lake et al., 1987; Behrens Yamada and Boulding, 1998; Whitenack and Herbert, 2015). Note that the ability to take larger prey does not necessarily imply a preferred size range of prey.
This relationship between crab size, success, and maximum size of the prey taken also holds true for the cancrid-Tegula system (Mendonca, 2020). Not only are larger cancrids stronger and therefore more likely to be successful, but larger crabs are able to handle larger Tegula. Cancrid crabs typically attack gastropod prey by either attempting to crush the shell directly, or by peeling back the aperture with a series of chips (Stafford et al., 2015a). The latter method is time consuming (Barclay et al., 2020b) (increasing the likelihood of the crab being interrupted by its own predators and competitors) and the gastropod retracts farther into its shell with each peel, decreasing the crab’s chances of success (Stafford et al., 2015a; Mendonca, 2020). In contrast, crushing is quick, and when the shell fractures, the gastropod has no escape. The choice of crushing versus peeling is in large part a function of the relative sizes of the crab and the gastropod (Mendonca, 2020). Larger crabs have large enough chelae to grasp a large Tegula across its width and attempt a crush, whereas smaller crabs are forced to peel. Thus, aside from their greater strength, larger crabs also have the option of utilizing a more successful attack strategy more frequently. However, they also can and do take larger Tegula by peeling. Strength, size, success, and largest prey are therefore expected to correlate, and the Mendonca (2020) thesis is consistent with this prediction. Assuming that these results hold across time, maximum size at attack (SAA) is an indicator of both predator strength (relative to the prey) and success.
A change in repair frequency due to a change in success can also show a change in SAA. However, it is also possible to use SAA to distinguish changes in crab strength/success as well as evaluate whether these changes have impacted the observed repair frequency (i.e., the success of the predator compared to the prey available to them) when comparing samples. For example, consider a hypothetical scenario where SAA increases, but so does the size of prey. If the distribution of attacks (SAA) remains relative to the size distribution of prey available, the relative rate of predator success against the prey available to them has not changed. In this scenario, while we can observe that crab strength and success has increased, because prey size also increased, the relative success rate of the predator is the same and therefore changes in crab strength/success are not likely to have impacted the observed repair frequency. Therefore, by considering the size at which repairs occur, it is not only possible to distinguish changes in predator success, but the SAA can also be used to evaluate whether these changes in success have impacted the observed repair frequency. Here, we analyze repairs on Late Pleistocene and living individuals of a common and widespread crab prey item, the black turban snail (Tegula funebralis) (Geller, 1982, 1983; Molinaro et al., 2014; Stafford et al., 2015b), to assess patterns of modern crab predation relative to a pre-Industrial baseline in the Pleistocene. This approach also demonstrates the value of repair scars and paleontological data as a cost-effective, rapid assessment tool to fill the critical gap in our knowledge of the current crab fisheries in southern California.
Fossil T. funebralis material came from the Natural History Museum of Los Angeles County invertebrate paleontology (LACMIP) collections. Any lot containing T. funebralis was assessed, and of those, all adult T. funebralis (those containing at least three body whorls) that were “intact” (minimally a complete apex and aperture, and no more than 25% of any whorl missing) were measured and assessed for all repair scars. Some of the fossil localities and/or lots also contained T. gallina, a closely related gastropod that co-occurs with T. funebralis in southern California and is often very difficult to distinguish from T. funebralis (Alf, 2019). To be conservative, any specimen that was possibly T. gallina was excluded from this study (about 100 specimens in total). The material was from Late Pleistocene high stand terraces (120 – 80 ka) (Muhs et al., 2006) with temperatures (Muhs et al., 2006, 2014) and marine invertebrate faunal zoogeographic ranges (Valentine, 1962; Roy et al., 1995; Muhs et al., 2014) similar to present conditions (with possibly slightly cooler temperatures at 80 ka). The bulk of southern California T. funebralis material in the collections came from the lower terraces of the Palos Verdes Hills and San Pedro area (as identified by comparing lot GPS coordinates with terrace maps (Figure 6 from Muhs et al., 2006 if the exact terrace was not specified), and from terraces from the San Diego area (Figure 1). We therefore restricted ourselves to including only specimens from those areas and terraces in subsequent analyses. As some of the lots lacked specific terrace information, or stated that they were taken from multiple terraces at one locality, we conservatively assumed that there was at least some mixing of the 120 and 80 ka terraces in both areas. We therefore treat all fossil material as a potentially time averaged “Late Pleistocene” assemblage, representing up to approximately 40,000 years of time, but still separated from the modern material by about 80,000 years. Therefore, any biological signals that were apparent despite potential time averaging of the Pleistocene material were likely robust and conservative (Kowalewski et al., 1998; Tomasovych and Kidwell, 2010; Kidwell, 2013). Furthermore, as this study also focuses on a single species, and had strict criteria for the completeness and size of individuals, preservational biases that are sometimes a concern when comparing fossil and modern material are not an appreciable issue here. We had a total of 712 fossil specimens (261 from the Palos Verdes Hills area, and 451 from the San Diego area).
Figure 1. Map of southern California between Los Angeles and San Diego with images of fossil and modern T. funebralis with repair scars. Red arrows on T. funebralis indicate location of repair scars (wedge-shaped scar/line on body whorl). Map insets show the two study areas (Palos Verdes Hills and San Diego) in more detail. Modern localities are indicated in red stars (four from the Palos Verdes Hills area, and three from north of San Diego). Approximate fossil localities are indicated by blue lines which indicate the terraces and/or GPS coordinate information that accompanied fossil lots. Terraces in the Palos Verdes Hills are based on Figure 6 of Muhs et al. (2006).
Live specimens of T. funebralis were measured in situ at seven localities (four from the Palos Verdes Hills area near Los Angeles, and three from the Bird Rock area north of San Diego) in the early morning during the first low tide cycle of July 2019 (Figure 1). The two study areas were selected based on their proximity to the areas where the fossil material had been collected, and all rocky intertidal outcrops in those areas that were publicly accessible were surveyed for T. funebralis. Any site which contained T. funebralis was surveyed, with the exception of a single site of abundant T. funebralis near San Pedro (Point Fermin Park) which became inaccessible due to several earthquakes that occurred during the survey period, causing instability of the cliffs above the site. The seven sites are therefore representative of the range of moderately wave-exposed and cobbled, rocky shore habitats typical of T. funebralis in southern California.
While modern sites were not “time averaged” to the same extent as is expected for fossil assemblages, T. funebralis is a long lived species (upward of 30 years) (Frank, 1975), and individuals with at least three body whorls are already several years old (Paine, 1969). Given that gastropod shells grow by accretion, sampling of repairs scars can be considered to be on the scale of the animals’ lifespan. Furthermore, repair scar frequencies on T. funebralis are not impacted by short term, but extreme, environmental disturbances, indicating that T. funebralis repair scar patterns in the modern are operating on at least the decadal scale (Tyler et al., 2019). Thus, repair scar patterns can be considered to capture more robust biological signals “time averaged” over the lifespan of the organism, as is also an advantage of more time averaged fossil assemblages (Kowalewski et al., 1998; Kidwell, 2013), and useful when comparing sampling efforts between live or recent samples versus death assemblages (Powell et al., 2020). As with the fossil assemblages, only those gastropods that had at least three body whorls were measured to ensure consistency of body size sampling protocol between modern and fossil specimens. At each site, approximately 150 averaged-sized adults (those that had at least three body whorls) were randomly chosen for measuring. Collection was spread out as evenly as possible over the entire site to ensure there were no biases due to spatial or tidal/vertical positions of the snails. Strictly measured transects and quadrats were therefore not used given that each study site was a different size and we were more concerned with capturing a representative sample of individuals from the entire site. Two researchers would go to either end of the site and walk up and down in straight lines perpendicular to the shoreline spaced roughly two meters apart, depending on the size of the site. Approximately 10 – 20 snails would be collected from each “line,” depending on the size of the site, with collection being spread evenly across the line. Specimens were measured, and then quickly returned to their approximate location to ensure minimal disturbance before the tidewaters returned. We measured a total of 1,152 modern specimens across the seven sites.
We measured prey body size and compared repair frequency and the size at which repair scars occurred (size at attack, SAA) (Pruden et al., 2018) as a means of assessing the strength and relative success of Pleistocene and modern crabs to ensure that crab success was not impacting the observed repair frequencies (Figure 1). For example, if a prey species has not strengthened its defenses (e.g., increased shell thickness or ornament, etc.) over time, then larger, stronger predators would not only have an increased likelihood of success, but would also be able to take larger prey. Thus, maximum SAA is an indicator of both predator strength (relative to the prey) and success. If maximum SAA increases through time, we would expect predators to be more successful. Using prey body size and size at repair/attack has also been used in other studies to examine the relationship between predator strength/success and prey defenses (Alexander and Dietl, 2001; Mondal et al., 2014). However, this approach can also help distinguish between changes in repair frequency due to changes in success versus changes in attack frequency; a change in repair frequency due to a change in success should also show a change in SAA. In contrast, if there were no obvious changes in SAA (and therefore no changes in crab success) between the modern and fossil assemblages, then any changes in observed repair frequencies would indicate a change in crab abundance.
In both the fossil collections and modern sites, each specimen was assessed for repair scars, and measurements of size were taken (both maximum height and width). If a scar was present, the size at attack (SAA) was also taken. As repair scars are formed when a crab attempts to peel back the aperture, and gastropods grow their shells by accretion, the SAA measurement indicates the width of the animal at the time it was attacked. SAA was measured for all scars from the point at which the repair met the top of the whorl along a line segment passing through the apex to the point on the opposite side of the gastropod (Figure 2). If a gastropod had more than one repair, the SAA was measured for each, as they indicated separate attack events. Data collected are available as Supplementary Datasets 1, 2.
Figure 2. Examples of repair scars on T. funebralis and their measurement. (A) A typical repair scar generated by a failed apertural peel by a crab (red arrow). (B) Size of the gastropod at the time of attack (SAA), measured from where the scar (red arrow, traced by red line) met the top of the whorl where it occurred along a line segment through the center of the gastropod to the opposite edge of the shell (red dotted line). Overall body width measurements were taken as the maximum width (diameter) of the gastropod shell (blue dotted line).
To determine if (1) the presence or number of scars on each gastropod, (2) size, or (3) SAA, differed between the Pleistocene and the modern, we ran a series of Wilcoxon rank sum tests on each. We then tested if the presence or number of scars on individual gastropods were also affected by size by fitting generalized mixed models of scars, one to examine scar presence, and a second to examine number of scars. As each gastropod could have multiple SAA measurements, SAA was independent of measures from the individual gastropod (such as size or the number of scars) and was therefore not added to the models. The presence or number of scars on each gastropod was the dependent (response) variable, with size and time (Pleistocene versus modern) as independent (fixed) variables. Region (Palos Verdes versus San Diego) was also included as an independent variable to confirm that region was not affecting the results. Lot/locality was included as a random effect. For the model of presence/absence of scars, specimens were coded as scarred (1) or unscarred (0), and a binomial family link was used to fit the model. To test which independent variables affected the total number of scars on each specimen, a Poisson family link was used to fit the second model, in which the number of scars was treated as discrete/count data. The best model was determined by comparison of the Akaike Information Criterion (AIC) scores, where the lowest score indicated the best model. We used log-likelihood ratio tests to determine if the best fitted model performed significantly better than a null model (which only included lot/locality as the required random effect for generalized mixed models, and no independent variables). Significance of the independent variables on predicting scars was then examined using Wald Z-scores. If both size and time were found to have significant effects on the presence or number of scars, we then assessed the strength/direction of the relationship between scars and size by running a partial correlation which backed out the effects of time. All analyses were performed with R (version 4.1.1 using the lme4 and DHARMa packages).
Size at attack was used to gauge potential differences in (1) the success of crabs, both in terms of the overall strength of crabs (size of SAA) and relative distribution of repair sizes compared to the distribution of prey sizes, and (2) the potential consequences for the number of failures/repair frequencies observed between the two time periods. In terms of success, samples with greater average SAA between samples would indicate stronger, and therefore more successful crabs that only failed on larger prey. However, it is important to note that even if the overall success or size of prey that a crab can kill changes between assemblages, the observed repair frequency might not change if there is also a relative change in the size of the prey (e.g., Figures 3B–E). Hypothetically, even if the samples being compared did not overlap in terms of prey size or SAA, as long as the relative distribution of prey size and SAA in each sample remains constant (e.g., as prey in one sample grew larger, so did SAA), the observed repair frequency would not be impacted. Success in terms of the overall and relative strength of predators against the prey available to them can therefore be evaluated independently of observed repair frequencies if SAA and the relative distribution of prey size are considered. In other words, even if there are differences in crab strength/success, if the distributions of repairs to prey size remain similar between samples (e.g., both prey size and SAA get larger), we would not expect to see a difference in the observed number of repairs, and therefore any changes in repair frequency likely indicate a change in the number of attacks experienced (Figure 3).
Figure 3. Hypothetical distributions of repair scar sizes (SAA) (red line) and overall prey body size (blue line) as they relate to both predator success and comparability of repair frequency (RF) between samples. (A) Null distributions of SAA vs. body size used to compare with other scenarios (figure panels). Potential measures of success for comparison: [1] distance between minimum SAA (5th percentile) and body size (minimum size at which predators are likely to fail, resulting in a repair – note that crabs may still attack smaller prey, but below [1], attacks must be successful, as repairs do not occur), [2] proportion of repairs below the minimum repair size, [3] distance between mean SAA and prey size, and [4] distance between the maximum SAA (95th percentile) and prey size (indicating the largest prey the predator is able to attack, as well as the prey in a size refuge from predation). There may also be additional scenarios and relevant measures other than [1] – [4] to those shown. Red arrow = changes in predator strength, blue arrow = changes to prey size. (B–E) Because the shift in the distribution of SAA or prey size is proportional ([1] and [4] remain relative), RF is not affected (equal symbol to right of graph), even if success changes. (F–K) Scenarios where the shift in the distribution of repairs is not proportional, indicating that RF might be affected (black arrows to right of panel indicate whether RF is greater or less than expected). (L,M) If distributions of SAA and prey size are relative between samples, changes to RF indicate differences in the number of attacks (red dotted line).
We compared crab strength (SAA) via the Wilcoxon rank sum test of SAA. The relative success between the Pleistocene and modern was then compared by plotting histograms of SAA and gastropod size (width) to determine whether there were any obvious visual differences in the distributions of SAA and gastropod size relative to each other between samples (see Figure 3 for hypothetical distributions). To further assess whether there were any shifts in the relative distributions of SAA compared to body size between the samples, we calculated the distance between the minimum body width and SAA (5th percentile), mean body width and SAA, and maximum body width and SAA (95th percentile) ([1], [3], and [4] of Figure 3A), as well as quartiles 1, 2, and 3, and the interquartile range (IQR). We then ran a Fisher’s exact test to see if any of these distribution metrics were significantly different in the modern compared to the Pleistocene. If the distributions of SAA and gastropod size shift in position relative to each other, then we consider the direction of change in SAA (e.g., greater SAA values relative to prey may indicate greater success on the part of the predator) (Figure 3). If there were no differences in the position of the distributions of SAA relative to gastropod size between samples, the observed repair frequencies should not be affected by success (i.e., relative success rate of the predator against the prey available to them remains the same), any changes in repair frequency between samples would be indicative of a change in the number of attacks (Figures 3L,M).
Modern T. funebralis (1) have fewer scars (Wilcoxon rank sum test, p < 0.001), (2) are larger than their Pleistocene counterparts (p < 0.001), and (3) are repaired at larger sizes (p < 0.001) (Figure 4 and Table 1). When including prey size in mixed model analyses, the same patterns of fewer repairs and larger gastropods in the modern compared to the Pleistocene held (Table 2). Time (geologic age) still had a significant effect on whether a gastropod had at least one scar in the mixed models (Table 2). When backing out the effects of time, the presence and number of scars showed a weak, but significant correlation with size (partial R = 0.134 and 0.141, respectively, p < 0.0001). Region (Palos Verdes vs. San Diego) did not have a significant effect on scars or model performance (Table 2 and Supplementary Table 1). Random effects (lot/locality) also did not have any significant impact on model performance (Table 2). As the fossil lots from the Palos Verdes Hills area are likely attributed to the older 120,000 ya highstand (Figure 6 in Muhs et al., 2006) compared to more mixing of the 80,000, 100,000, and 120,000 ya highstands from the San Diego area (Kern, 1977; Kennedy et al., 1982; Muhs et al., 1994, 2012), this indicates that differences in time averaging between fossil localities and regions are not substantial enough to impact the results. Therefore, changes observed between the Pleistocene and modern are not the result of differences in time averaging of samples.
Figure 4. Results of Pleistocene (A) vs. modern (B) body size and size at attack (SAA) for T. funebralis. Boxplots show differences in gastropod body size and size at attack (SAA) for both the Pleistocene and modern samples (see Table 1 for Wilcoxon rank sum test results). Boxes = upper and lower quartiles, central lines = medians, whiskers = min/max data, circles = outliers. Histograms show distribution of SAA (red) and gastropod body size (blue). Relative positions of the distributions of SAA and body size between Pleistocene and modern samples can be compared based on Figure 3, with results described in Table 3. The y-axes (counts or number of observations in that size class) are not scaled, as Pleistocene and modern sample sizes were different. Dotted horizontal lines indicate the maximum frequency of repair scars in any size bin, with fewer repairs on modern gastropods. Maximum SAA, as well as relative position of the distributions of SAA vs. body size are similar between both the Pleistocene and modern (see Table 3), suggesting that any differences in repair frequency represent changes in the number of crab attacks, rather than a difference in crab relative success. If anything, there are more repairs on smaller gastropods in the modern (Table 3), which should increase the expected frequency of repairs, yet there are still fewer repairs observed in the modern. While the increased frequency of comparatively small gastropods between 11 and 13 mm in the Pleistocene may be the result of time averaging, it is important to note that this does not influence the relative position of the distributions of SAA compared to body size (see also Table 3), nor the observation of fewer repairs in the modern.
Changes in the frequency of repairs between the Pleistocene and modern is indicative of a change in the number of attacks, rather than a change in crab success. The number of repairs expected should be similar between the two samples because even though the average SAA is larger in the modern (p < 0.0001) (Figure 4 and Table 1), as modern gastropod body size also increases, the distribution of repair size (SAA) relative to body size are not significantly different between the two samples (Table 3 and Figure 4). Increases in modern SAA should therefore be influenced by the greater number of larger gastropods available to attack. Hypothetically, even if gastropod size did not overlap between samples, if the observed repairs show the same size distribution relative to the gastropods, as they do for our dataset (Figure 4), the relative success of crabs against those two gastropod populations would be the same, and so would the repair frequencies (e.g., Figures 3B vs. 3D). Thus, observed changes to repair frequencies should be the result of a change in attack rates.
Table 3. Metrics used to compare the relative position of body size (width) and SAA between Pleistocene and modern samples.
If anything, Pleistocene crabs were relatively more successful against the gastropods available to them, as there were fewer repairs at relatively small sizes in the Pleistocene compared to today (4.3 vs. 12.7% of repairs occurred below the minimum gastropod size, [2] in Figure 3A, in Pleistocene vs. modern assemblages) (Figure 4), and the distance between the minimum SAA and the minimum gastropod size ([1] in Figure 3A) was almost twice as large in the modern compared to the Pleistocene (Table 3 and Figure 4). In addition, the maximum size of repairs is similar between the two time intervals (Table 3), indicating that absolute crab strength has not changed substantially since the Pleistocene (Figure 4). Therefore, it is unlikely that the decrease in repairs observed in the modern is a function of a change in success. Furthermore, while size had a significant effect on whether a gastropod had one or more scars, this was true regardless of time (Table 1), indicating that crabs are simply more likely to fail against larger gastropods in each assemblage/location. Fewer repairs observed in the modern therefore conservatively indicates fewer attacks, rather than a change in success between Pleistocene and modern crabs (Figure 4).
Our results demonstrate a decrease in repair frequencies in modern compared to Pleistocene assemblages, conservatively indicating fewer crab attacks today compared to the Pleistocene. Given that differences in time averaging between Pleistocene assemblage lots and modern samples did not impact the results, the signal of reduced predation in the modern can be considered a robust biological signal, as is often an advantage of time averaged assemblages (Kowalewski et al., 1998; Kidwell, 2013; Kidwell and Tomasovych, 2013). There are therefore several alternative explanations to explain the possible reductions in modern crab attacks, all of which suggest that crab fisheries in southern California require increased attention and protections to ensure sustainability of these economically important species and their ecosystems. The results of our study also exemplify the value of including paleontological data and techniques in assessments of important modern coastal resources.
The simplest, and most troubling reason for the decline in crab predation on modern T. funebralis in southern California would be a reduction in the abundance of crabs. Worldwide, crustacean fisheries in general have become increasingly socioeconomically valuable and important in recent decades (Boenish et al., 2021). Severe declines in Dungeness crab populations have been documented locally in recent decades by Indigenous fishers in British Columbia, Canada who attribute these declines to commercial and recreational fishing (Ban et al., 2017). In southern California, recent data modeling studies indicate early warning signs of crab overfishing, along with concerns from fishermen and researchers about observed changes to crab body size and abundance in southern California (Fitzgerald et al., 2018, 2019). Declines in Dungeness crabs populations have been potentially associated with increases in sea otter populations in California (only at a small, localized level) (Grimes et al., 2020), but these are reported declines in crab populations, nonetheless. Our results support a decrease in crab abundances in southern California, suggesting immediate action (e.g., closure of fisheries) is needed to protect commercially exploited crabs in southern California.
Besides overexploitation, reductions in crab predation might also be due to widespread ecosystem changes caused by human activity and climate change. For example, ocean acidification affects crab larval development along the west coast of North America (Hodgson et al., 2018; Bednaršek et al., 2020). All life stages of Dungeness crab are also predicted to be vulnerable to year-round ocean conditions (low pH, low oxygen, and increased temperatures) by the end of the century (Berger et al., 2021). Mollusks, including T. funebralis, are also expected to experience direct consequences of ocean acidification, including impaired behavior, and reduced shell growth and strength (Jellison et al., 2016; Barclay et al., 2019, 2020a). While it does appear that absolute shell strength of T. funebralis may be reduced in the modern [a shift in the minimum absolute SAA to larger sizes indicates that shells may have been easier to crush at those same sizes in the modern (Figure 4)], it does not appear to have impacted the success rate of crabs as of yet. Modern crabs may have also become more restricted vertically, either due to stress or habitat loss, and may have fewer opportunities to feed on T. funebralis in its given tidal range. Rockweeds, kelp forests, and other habitat forming macroalgae have declined in southern California recently (Whitaker et al., 2010; Jurgens and Gaylord, 2018; Beas-Luna et al., 2020). At most of our sites, rockweeds and other large macroalgae were lacking, with T. funebralis found most commonly on bare rock covered in biofilm. The loss of habitat forming macroalgae may restrict crabs to foraging mostly at lower tidal heights where there is still abundant macroalgae, as habitat forming species are critical buffers against temperature stress, moreso than latitude or tidal elevation (Jurgens and Gaylord, 2018), and provide crabs refuge from their own predators, particularly when they are younger (Robles et al., 1989; Behrens Yamada and Groth, 2016).
Crabs may have also switched to other prey items, such as mussels, and attack T. funebralis less often. Indeed, mussels are a more common prey item of crabs (Robles et al., 1989). However, we did not observe any large mussel beds at any of the modern sites, and there have also been notable declines in mussel populations in southern California in recent decades (Smith et al., 2006a,b). Reductions in mussel populations in present-day southern California might increase crab predation on T. funebralis, yet we observe the opposite. While there are other prey items that might be preferred, such as owl limpets and other species of Tegula (e.g., T. brunnea and T. eiseni), these species were also present during the Late Pleistocene. Given that mussel and macroalgal beds are important habitat formers, and there are reported declines in these and associated benthic intertidal taxa in southern California (Smith et al., 2006a,2008; Whitaker et al., 2010; Beas-Luna et al., 2020), if anything, these reductions in biodiversity should reduce the amount and/or number of alternative prey, suggesting that the probability of T. funebralis being included in crab diets, or even becoming a more preferred prey item, should increase. That we still observe a decline in predation on modern T. funebralis is therefore likely conservative with respect to decreased crab abundances.
A difference in temperature, particularly since the cooler 80,000 ya highstand, could have affected predation rates, and/or caused a change in both the size of T. funebralis and their crab predators. However, as the Pleistocene samples were a mix of both the 80,000 and 120,000 ya highstands, the latter of which was similar in temperature to today, temperature alone is an insufficient explanation for changes in body size and repairs. Furthermore, as increases in temperature also can increase predation rates (Bélair and Miron, 2009; Leighton and Tyler, 2021), we would have also expected more repair scars at modern sites, yet the opposite is true. This conservatively implies that decreases in crab attacks are driven by reduced crab abundances in the modern compared to the Pleistocene. A release from predation pressure due to a reduction in crabs might have also driven the increases in body size for T. funebralis, as T. funebralis body size is known to decrease in response to human predation intensity (Erlandson et al., 2015). Additionally, sea stars are another major predator of T. funebralis (Paine, 1969; Fawcett, 1984; Gravem and Morgan, 2017), and their populations have been severely affected by an outbreak of sea star wasting disease in 2013 (Hewson et al., 2014; Menge et al., 2016). Both crabs and sea stars are known to have disproportionately large effects on their ecosystems (Paine, 1966, 1969; Hull and Bourdeau, 2017; Leighton and Tyler, 2021). Reduced populations of both crabs and sea stars might also influence coastal ecosystems due to changes in the body size of their prey.
Regardless of the potential cause, reduced crab predation rates in southern California warrant further attention both from a fisheries/resource management and ecosystem health perspective. To avoid further depletion of current crab stocks, increased management practices may need to be enacted. Past studies show that Dungeness crab populations are quick to recover after spatial closures (Taggart et al., 2004; Frid et al., 2016). However, if the cause of declines in crab populations is also related to ecosystem changes and degradation on southern California’s coast due to anthropogenic impacts, such as changes to macroalgal and seagrass communities (Waycott et al., 2009; Whitaker et al., 2010; Beas-Luna et al., 2020) that are often nurseries for young crabs (Robles et al., 1989; Beck et al., 2003; Holsman et al., 2006; Behrens Yamada and Groth, 2016), or to seawater conditions (Bednaršek et al., 2020; Berger et al., 2021), changes to crab fishery management policies will be insufficient to sustain these resources long-term.
To improve the management and long-term sustainability of crab fisheries in southern California and beyond, we recommend: (1) the enactment of increased management practices in southern California, such as partial or spatial closures of crab fisheries that would allow for potentially rapid recovery of crab stocks, and (2) the use of repairs scar surveys in both modern and fossil assemblages as a fast, cost-effective, and easily implemented tool for initial assessments of crab population health. More broadly, there are several other areas for improvement and continued investigation. First, addressing the ever-increasing urgency of climate change and anthropogenic impacts on coastal ecosystems and resources (Beas-Luna et al., 2020; Bednaršek et al., 2020; Berger et al., 2021) is paramount to ensuring long-term sustainability of far more than just crab fisheries. For example, as macroalgal and seagrass communities are important habitat for young crabs (Robles et al., 1989; Beck et al., 2003; Holsman et al., 2006; Behrens Yamada and Groth, 2016), any restoration of these communities will also greatly benefit crabs (Anderson, 1989). Addressing anthropogenic carbon dioxide emissions is also key to addressing future ocean conditions, including warming, hypoxia, and ocean acidification that will impact crabs (Bednaršek et al., 2020; Berger et al., 2021). Second, conservation paleobiology and historical ecology offer unique perspectives, tools, and datasets that provide much needed and often missing context to ensure the sustainability and protection of ecosystems and their services (Hadlock Seeley, 1986; Erlandson and Rick, 2010; Dietl and Flessa, 2011; Mcclenachan et al., 2012; Rick and Lockwood, 2013; Dietl et al., 2015; Kidwell, 2015; Barnosky et al., 2017; Fordham et al., 2020). Related to crabs, other studies have used historical records of prey (gastropod) morphology as additional evidence of the spread of the invasive European green crab, Carcinus maenas, on the northeast coast of the United States (Hadlock Seeley, 1986). Specifically for fisheries, the regular inclusion of alternative sources of information, including repair scars and paleontological data, as well as historical and traditional knowledge/data can provide important insights to understand the status of data-limited fisheries (Lotze et al., 2011; Ban et al., 2017; Toniello et al., 2019) and should be implemented more widely in fisheries management. For example, Indigenous knowledge and first-hand accounts from fishers have proven valuable to crab fisheries modeling and stock assessments (Ban et al., 2017; Fitzgerald et al., 2018, 2019). Indigenous and first-hand knowledge provides additional context that could be used in conjunction with repair scars to assess the severity and timing of stock declines, as well as to identify areas of greatest concern where repair scar surveys could be implemented.
Our results demonstrate that crab predation traces are a novel, inexpensive initial assessment tool to determine the current health of important coastal species, particularly if current or historical records are sparse or lacking. We also demonstrate that fossil data are valuable for determining pre-human baselines that provide context for the status of current fisheries and long-term responses of both crabs and their prey amidst human-induced environmental changes. Repair scars are therefore a robust indicator that can be used to determine whether further action is needed to provide greater protections for vulnerable crab populations worldwide. We suggest that reduced crab predation in modern gastropods is most likely caused by human-induced changes (e.g., overexploitation of predators or climate-induced ecosystem disturbances), but that regardless of the cause(s), there is immediate need for a detailed investigation of current crab fishery stocks in southern California to ensure the sustainability of these critical coastal socioeconomic resources. An important avenue for continued future research would be to expand upon the current data framework to include historical and archaeological collections (Rick and Lockwood, 2013; Toniello et al., 2019) to understand the timing of crab population declines and improve sampling efforts, and to apply the methods used in this study to other locations of concern for shell-crushing crab resources. We conclude that incorporating paleontological techniques and data (i.e., conservation paleobiology) to resource management provides critical new insights that can aid in the management and sustainability of exploited biological resources.
The original contributions presented in the study are included in the article/Supplementary Material, further inquiries can be directed to the corresponding author.
KB and LL conceived the study, provided funding for the study, revised, and edited the original drafts. KB collected and analyzed all the data and wrote the original drafts. LL provided guidance with analyses and acted in a supervisory role. Both authors contributed to the article and approved the submitted version.
This work was supported by a NHM of LA County Student Collections Grant (KB), and an NSERC Vanier CGS (KB), MSFSS (KB), and NSERC Discovery Grant (LL).
The authors declare that the research was conducted in the absence of any commercial or financial relationships that could be construed as a potential conflict of interest.
All claims expressed in this article are solely those of the authors and do not necessarily represent those of their affiliated organizations, or those of the publisher, the editors and the reviewers. Any product that may be evaluated in this article, or claim that may be made by its manufacturer, is not guaranteed or endorsed by the publisher.
This work was conducted on the traditional lands of the Tongva, Kich, and Kumeyaay peoples. We thank A. Hendy, S. Weidrick, and staff and volunteers of the NHM of LA County invertebrate paleontology collections (LACMIP) for assistance with the collections and identifying material. We also thank R. Dievert for assistance in collecting data for modern gastropod populations. Thank you to GV and JS for their thoughtful feedback which greatly improved the manuscript.
The Supplementary Material for this article can be found online at: https://www.frontiersin.org/articles/10.3389/fevo.2022.810069/full#supplementary-material
Alexander, R. R., and Dietl, G. P. (2001). Shell repair frequencies in New Jersey bivalves: A recent baseline for tests of escalation with Tertiary, mid-Atlantic congeners. Palaios 16, 354–371. doi: 10.1669/0883-13512001016<0354:SRFINJ<2.0.CO;2
Alexander, R. R., and Dietl, G. P. (2003). “The Fossil Record of Shell-Breaking Predation on Marine Bivalves and Gastropods,” in Predator-Prey Interactions in the Fossil Record, eds P. H. Kelley, M. Kowalewski, and T. A. Hansen (New York, NY: Kluwer Academic/Plenum Publishers), 141–176. doi: 10.1007/978-1-4615-0161-9_7
Alf, A. (2019). Tegulidae and Turbinidae of the northeast Pacific. Zoosymposia 13, 70–82. doi: 10.11646/zoosymposia.13.1.8
Allison, E. H., Perry, A. L., Badjeck, M. C., Neil Adger, W., Brown, K., Conway, D., et al. (2009). Vulnerability of national economies to the impacts of climate change on fisheries. Fish Fish. 10, 173–196. doi: 10.1111/j.1467-2979.2008.00310.x
Anderson, E. E. (1989). Economic Benefits of Habitat Restoration: Seagrass and the Virginia Hard-Shell Blue Crab Fishery. North Am. J. Fish. Manag. 9, 140–149. doi: 10.1577/1548-86751989009<0140:ebohrs<2.3.co;2
Ban, N. C., Eckert, L., McGreer, M., and Frid, A. (2017). Indigenous knowledge as data for modern fishery management: a case study of Dungeness crab in Pacific Canada. Ecosyst. Heal. Sustain. 3:1379887. doi: 10.1080/20964129.2017.1379887
Barclay, K. M., Gaylord, B., Jellison, B. M., Shukla, P., Sanford, E., and Leighton, L. R. (2019). Variation in the effects of ocean acidification on shell growth and strength in two intertidal gastropods. Mar. Ecol. Prog. Ser. 626:13056. doi: 10.3354/meps13056
Barclay, K. M., Sinclair, C. R., and Leighton, L. R. (2020b). Patterns of prey selection by the crab Cancer productus among three similar gastropod species (Nucella spp.). J. Exp. Mar. Bio. Ecol. 53:151443. doi: 10.1016/j.jembe.2020.151443
Barclay, K. M., Gingras, M. K., Packer, S. T., and Leighton, L. R. (2020a). The role of gastropod shell composition and microstructure in resisting dissolution caused by ocean acidification. Mar. Environ. Res. 162:105105. doi: 10.1016/j.marenvres.2020.105105
Barnosky, A. D., Hadly, E. A., Gonzalez, P., Head, J., Polly, P. D., Lawing, A. M., et al. (2017). Merging paleobiology with conservation biology to guide the future of terrestrial ecosystems. Science 2017:355. doi: 10.1126/science.aah4787
Baum, J. K., and Fuller, S. D. (2016). Canada’s marine fisheries: Status, recovery potential, and pathways to success. Ottawa, ON: Oceana Canada.
Beas-Luna, R., Micheli, F., Woodson, C. B., Carr, M., Malone, D., Torre, J., et al. (2020). Geographic variation in responses of kelp forest communities of the California Current to recent climatic changes. Glob. Chang. Biol. 26, 1–19. doi: 10.1111/gcb.15273
Beck, M. W., Heck, K. L. J., Able, K. W., Childers, D. L., Eggleston, D. B., Gillanders, B. M., et al. (2003). The Role of Nearshore Ecosystems as Fish and Shellfish Nurseries. Issues Ecol. 11, 1–12.
Bednaršek, N., Feely, R. A., Beck, M. W., Alin, S. R., Siedlecki, S. A., Calosi, P., et al. (2020). Exoskeleton dissolution with mechanoreceptor damage in larval Dungeness crab related to severity of present-day ocean acidification vertical gradients. Sci. Total Environ. 716:136610. doi: 10.1016/j.scitotenv.2020.136610
Behrens Yamada, S., and Boulding, E. G. (1998). Claw morphology, prey size selection and foraging efficiency in generalist and specialist shell-breaking crabs. J. Exp. Mar. Bio. Ecol. 220, 191–211. doi: 10.1016/S0022-0981(97)00122-6
Behrens Yamada, S., and Groth, S. D. (2016). Growth and longevity of the red rock crab Cancer productus (Randall, 1840). J. Shellfish Res. 35, 1045–1051. doi: 10.2983/035.035.0427
Bélair, M.-C., and Miron, G. (2009). Time budget of Cancer irroratus (Say) and Carcinus maenas (L.) under various temperature and prey density conditions during conspecific and heterospecific challenges. J. Shellfish Res. 28, 923–930. doi: 10.2983/035.028.0423
Berger, H. M., Siedlecki, S. A., Matassa, C. M., Alin, S. R., Kaplan, I. C., Hodgson, E. E., et al. (2021). Seasonality and Life History Complexity Determine Vulnerability of Dungeness Crab to Multiple Climate Stressors. AGU Adv. 2, 1–19. doi: 10.1029/2021AV000456
Boenish, R., Kritzer, J. P., Kleisner, K., Steneck, R. S., Werner, K. M., Zhu, W., et al. (2021). The global rise of crustacean fisheries. Front. Ecol. Environ. 2021,1–9. doi: 10.1002/fee.2431
Cadée, G. C., Walker, S. E., and Flessa, K. W. (1997). Gastropod shell repair in the intertidal of Bahia la Choya (N. Gulf of California). Palaeogeogr. Palaeoclimatol. Palaeoecol. 136, 67–78. doi: 10.1016/S0031-0182(97)00041-2
Cinner, J. E., McClanahan, T. R., Graham, N. A. J., Daw, T. M., Maina, J., Stead, S. M., et al. (2012). Vulnerability of coastal communities to key impacts of climate change on coral reef fisheries. Glob. Environ. Chang. 22, 12–20. doi: 10.1016/j.gloenvcha.2011.09.018
Culver, C. S., Schroeter, S. C., Page, H. M., and Dugan, J. E. (2010). Essential fishery information for trap-based fisheries: Development of a framework for collaborative data collection. Mar. Coast. Fish. 2, 98–114. doi: 10.1577/c09-007.1
Dietl, G. P., and Alexander, R. R. (2009). Patterns of unsuccessful shell-crushing predation along a tidal gradient in two geographically separated salt marshes. Mar. Ecol. 30, 116–124. doi: 10.1111/j.1439-0485.2008.00261.x
Dietl, G. P., Durham, S. R., and Kelley, P. H. (2010). Shell repair as a reliable indicator of bivalve predation by shell-wedging gastropods in the fossil record. Palaeogeogr. Palaeoclimatol. Palaeoecol. 296, 174–184. doi: 10.1016/j.palaeo.2010.07.013
Dietl, G. P., and Flessa, K. W. (2011). Conservation paleobiology: putting the dead to work. Trends Ecol. Evol. 26, 30–37. doi: 10.1016/j.tree.2010.09.010
Dietl, G. P., Kidwell, S. M., Brenner, M., Burney, D. A., Flessa, K. W., Jackson, S. T., et al. (2015). Conservation paleobiology: Leveraging knowledge of the past to inform conservation and restoration. Annu. Rev. Earth Planet. Sci. 43, 79–103. doi: 10.1146/annurev-earth-040610-133349
Dolan, A. H., and Walker, I. J. (2006). Understanding Vulnerability of Coastal Communities to Climate Change Related Risks. J. Coast. Res. 3, 1316–1323.
Ekstrom, J. A., Suatoni, L., Cooley, S. R., Pendleton, L. H., Waldbusser, G. G., Cinner, J. E., et al. (2015). Vulnerability and adaptation of US shellfisheries to ocean acidification. Nat. Clim. Chang. 5, 207–214. doi: 10.1038/nclimate2508
Erlandson, J. M., Ainis, A. F., Braje, T. J., Jew, N. P., McVey, M., Rick, T. C., et al. (2015). 12,000 years of human predation on black turban snails (Chlorostoma funebralis) on Alta California’s northern Channel Islands. Calif. Archaeol. 7, 59–91. doi: 10.1179/1947461X15Z.00000000056
Erlandson, J. M., and Rick, T. C. (2010). Archaeology meets marine ecology: The antiquity of maritime cultures and human impacts on marine fisheries and ecosystems. Ann. Rev. Mar. Sci. 2, 231–251. doi: 10.1146/annurev.marine.010908.163749
Fawcett, M. H. (1984). Local and latitudinal variation in predation on an herbivorous marine snail. Ecology 65, 1214–1230. doi: 10.2307/1938329
Fitzgerald, S. P., Lenihan, H. S., Wilson, J. R., Culver, C. S., and Potoski, M. (2019). Collaborative research reveals cryptic declines within the multispecies California rock crab fishery. Fish. Res. 220:105340. doi: 10.1016/j.fishres.2019.105340
Fitzgerald, S. P., Wilson, J. R., and Lenihan, H. S. (2018). Detecting a need for improved management in a data-limited crab fishery. Fish. Res. 208, 133–144. doi: 10.1016/j.fishres.2018.07.012
Fordham, D. A., Jackson, S. T., Brown, S. C., Huntley, B., Brook, B. W., Dahl-Jensen, D., et al. (2020). Using paleo-archives to safeguard biodiversity under climate change. Science 369, 1–10. doi: 10.1126/science.abc5654
Frank, P. W. (1975). Latitudinal variation in the life history features of the black turban snail Tegula funebralis (Prosobranchia: Trochidae). Mar. Biol. 31, 181–192. doi: 10.1007/BF00391630
Frid, A., McGreer, M., and Stevenson, A. (2016). Rapid recovery of Dungeness crab within spatial fishery closures declared under indigenous law in British Columbia. Glob. Ecol. Conserv. 6, 48–57. doi: 10.1016/j.gecco.2016.01.002
Geller, J. B. (1982). Microstructure of shell repair materials in Tegula funebralis (A. Adams, 1855). Veliger 25, 155–159.
Geller, J. B. (1983). Shell repair frequencies of two intertidal gastropods from northern California: Microhabitat differences. Veliger 26, 113–115.
Gravem, S. A., and Morgan, S. G. (2017). Shifts in intertidal zonation and refuge use by prey after mass mortalities of two predators. Ecology 98, 1006–1015. doi: 10.1002/ecy.1672
Grimes, T. M., Tim Tinker, M., Hughes, B. B., Boyer, K. E., Needles, L., Beheshti, K., et al. (2020). Characterizing the impact of recovering sea otters on commercially important crabs in California estuaries. Mar. Ecol. Prog. Ser. 655, 123–137. doi: 10.3354/meps13530
Hadlock Seeley, R. (1986). Intense natural selection caused a rapid morphological transition in a living marine snail. Proc. Natl. Acad. Sci. 83, 6897–6901. doi: 10.1073/pnas.83.18.6897
Harley, C. D. G., Hughes, A. R., Hultgren, K. M., Miner, B. G., Sorte, C. J. B., Thornber, C. S., et al. (2006). The impacts of climate change in coastal marine systems. Ecol. Lett. 9, 228–241. doi: 10.1111/j.1461-0248.2005.00871.x
Hewson, I., Button, J. B., Gudenkauf, B. M., Miner, B., Newton, A. L., Gaydos, J. K., et al. (2014). Densovirus associated with sea-star wasting disease and mass mortality. Proc. Natl. Acad. Sci. 111, 17278–17283. doi: 10.1073/pnas.1416625111
Hodgson, E. E., Kaplan, I. C., Marshall, K. N., Leonard, J., Essington, T. E., Busch, D. S., et al. (2018). Consequences of spatially variable ocean acidification in the California Current: Lower pH drives strongest declines in benthic species in southern regions while greatest economic impacts occur in northern regions. Ecol. Modell. 383, 106–117. doi: 10.1016/j.ecolmodel.2018.05.018
Holsman, K. K., McDonald, P. S., and Armstrong, D. A. (2006). Intertidal migration and habitat use by subadult Dungeness crab Cancer magister in a NE Pacific estuary. Mar. Ecol. Prog. Ser. 308, 183–195. doi: 10.3354/meps308183
Hughes, R. N., and Elner, R. W. (1979). Tactics of a Predator, Carcinus maenas, and Morphological Responses of the Prey, Nucella lapillus. J. Anim. Ecol. 48, 65–78. doi: 10.2307/4100
Hull, W. W., and Bourdeau, P. E. (2017). Can crabs kill like a keystone predator? A field-test of the effects of crab predation on mussel mortality on a northeast Pacific rocky shore. PLoS One 12:e0183064. doi: 10.1371/journal.pone.0183064
Jackson, J. B. C., and Sala, E. (2001). Unnatural Oceans. Sci. Mar. 65, 273–281. doi: 10.3989/scimar.2001.65s2273
Jellison, B. M., Ninokawa, A. T., Hill, T. M., Sanford, E., and Gaylord, B. (2016). Ocean acidification alters the response of intertidal snails to a key sea star predator. Proc. R. Soc. B 283:20160890. doi: 10.1098/rspb.2016.0890
Jurgens, L. J., and Gaylord, B. (2018). Physical effects of habitat-forming species override latitudinal trends in temperature. Ecol. Lett. 21, 190–196. doi: 10.1111/ele.12881
Kennedy, G. L., Lajoie, K. R., and Wehmiller, J. F. (1982). Aminostratigraphy and faunal correlations of late Quaternary marine terraces, Pacific Coast, USA. Nature 299, 545–547. doi: 10.1038/299545a0
Kern, J. P. (1977). Origin and history of upper Pleistocene marine terraces, San Diego, California. Bull. Geol. Soc. Am. 88, 1553–1566. doi: 10.1130/0016-7606197788<1553:OAHOUP<2.0.CO;2
Kidwell, S. M. (2013). Time-averaging and fidelity of modern death assemblages: Building a taphonomic foundation for conservation palaeobiology. Palaeontology 56, 487–522. doi: 10.1111/pala.12042
Kidwell, S. M. (2015). Biology in the Anthropocene: Challenges and insights from young fossil records. Proc. Natl. Acad. Sci. U. S. A. 112, 4922–4929. doi: 10.1073/pnas.1403660112
Kidwell, S. M., and Tomasovych, A. (2013). Implications of Time - Averaged Death Assemblages for Ecology and Conservation Biology. Annu. Rev. Ecol. Evol. Syst 44, 539–563. doi: 10.1146/annurev-ecolsys-110512-135838
Kowalewski, M., Goodfriend, G. A., and Flessa, K. W. (1998). High-Resolution Estimates of Temporal Mixing within Shell Beds: The Evils and Virtues of Time-Averaging. Paleobiology 24, 287–304.
Lake, N. C. H., Jones, M. B., and Paul, J. D. (1987). Crab predation on scallop (Pecten maximus) and its implications for scallop cultivation. J. Mar. Biol. Assoc. U.K. 67, 55–64. doi: 10.1017/S0025315400026357
Leighton, L. R. (2002). Inferring predation intensity in the marine fossil record. Paleobiology 28, 328–342. doi: 10.1666/0094-83732002028<0328:IPIITM<2.0.CO;2
Leighton, L. R., and Tyler, C. L. (2021). Emergent effects of two rocky intertidal predators on prey: Interaction of crabs and sea-stars may reduce mussel mortality. J. Exp. Mar. Bio. Ecol. 539:151542. doi: 10.1016/j.jembe.2021.151542
Lotze, H. K., Erlandson, J. M., Hardt, M. J., Norris, R. D., Roy, K., Smith, T. D., et al. (2011). “Uncovering the Ocean’s Past,” in Shifting Baselines: The Past and Future of Ocean Fisheries, eds J. B. C. Jackson, K. E. Alexander, and E. Sala (Washington: Island Press), 296.
Mcclenachan, L., Ferretti, F., and Baum, J. K. (2012). From archives to conservation: Why historical data are needed to set baselines for marine animals and ecosystems. Conserv. Lett. 5, 349–359. doi: 10.1111/j.1755-263X.2012.00253.x
Mendonca, S. E. (2020). Measurement and Interpretation of Rates of Shell Repair Due to Predation M.Sc. Thesis. Edmonton, AB: University of Alberta, doi: 10.7939/r3-bjy9-pv40
Menge, B. A., Cerny-Chipman, E. B., Johnson, A., Sullivan, J., Gravem, S., and Chan, F. (2016). Sea star wasting disease in the keystone predator Pisaster ochraceus in Oregon: Insights into differential population impacts, recovery, predation rate, and temperature effects from long-term research. PLoS One 11:e0153994. doi: 10.1371/journal.pone.0153994
Menzies, R. J. (1951). Pleistocene Brachyura from the Los Angeles area: Cancridae. J. Paleontol. 25, 165–170.
Molinaro, D. J., Stafford, E. S., Collins, B. M. J., Barclay, K. M., Tyler, C. L., and Leighton, L. R. (2014). Peeling out predation intensity in the fossil record: A test of repair scar frequency as a suitable proxy for predation pressure along a modern predation gradient. Palaeogeogr. Palaeoclimatol. Palaeoecol. 412, 141–147. doi: 10.1016/j.palaeo.2014.07.033
Mondal, S., Bardhan, S., Mallick, S., and Roy, A. (2014). Repair scars on Mactra violacea from the eastern coast of India: A new classification and a model for describing shell breakage on bivalves. Palaeontol. Electron. 17, 1–13. doi: 10.26879/439
Mondal, S., and Harries, P. J. (2015). Temporal patterns in successful and unsuccessful shell-breaking predatory attack strategies on Varicorbula in the Plio-Pleistocene of Florida. Palaeogeogr. Palaeoclim. Palaeoecol. 428, 31–38. doi: 10.1016/j.palaeo.2015.03.031
Moore, S. K., Cline, M. R., Blair, K., Klinger, T., Varney, A., and Norman, K. (2019). An index of fisheries closures due to harmful algal blooms and a framework for identifying vulnerable fishing communities on the U.S. West Coast. Mar. Policy 110:103543. doi: 10.1016/j.marpol.2019.103543
Muhs, D. R., Groves, L. T., and Randall Schumann, R. (2014). Interpreting the paleozoogeography and sea level history of thermally anomalous marine terrace faunas: a case study from the Last Interglacial complex of San Clemente Island, California. Monogr. West. North Am. Nat. 7, 82–108. doi: 10.3398/042.007.0110
Muhs, D. R., Kennedy, G. L., and Rockwell, T. K. (1994). Uranium-series ages of marine terrace corals from the Pacific coast of North America and mplications for Last-Interglacial sea level history. Quat. Res. 42, 72–87. doi: 10.1006/qres.1994.1055
Muhs, D. R., Simmons, K. R., Kennedy, G. L., Ludwig, K. R., and Groves, L. T. (2006). A cool eastern Pacific Ocean at the close of the Last Interglacial complex. Quat. Sci. Rev. 25, 235–262. doi: 10.1016/j.quascirev.2005.03.014
Muhs, D. R., Simmons, K. R., Schumann, R. R., Groves, L. T., Mitrovica, J. X., and Laurel, D. A. (2012). Sea-level history during the Last Interglacial complex on San Nicolas Island, California: Implications for glacial isostatic adjustment processes, paleozoogeography and tectonics. Quat. Sci. Rev. 37, 1–25. doi: 10.1016/j.quascirev.2012.01.010
Nations, J. D. (1975). The genus Cancer (Crustacea: Brachyura): Systematics, biogeography, and fossil record. Bull. Nat. Hist. Museum Los Angeles Cty. 23, 1–104. doi: 10.1080/14772000.2011.554457
Paine, R. T. (1969). The Pisaster-Tegula interaction: Prey patches, predator food preference, and intertidal community structure. Ecology 50, 950–961.
Pilling, G. M., Apostolaki, P., Failler, P., Floros, C., Large, P. A., Morales-Nin, B., et al. (2008). “Assessment and management of data-poor fisheries,” in Advances in Fisheries Science: 50 Years on from Beverton and Holt, eds A. Payne, J. Cotter, and T. Potter (Hoboken, NJ: Blackwell Publishing Ltd), 280–305.
Powell, E. N., Ewing, A. M., and Kuykendall, K. M. (2020). Ocean quahogs (Arctica islandica) and Atlantic surfclams (Spisula solidissima) on the Mid-Atlantic Bight continental shelf and Georges Bank: The death assemblage as a recorder of climate change and the reorganization of the continental shelf. Palaeogeogr. Palaeoclimatol. Palaeoecol. 537:109205. doi: 10.1016/j.palaeo.2019.05.027
Pruden, M. J., Mendonca, S. E., and Leighton, L. R. (2018). The effects of predation on the preservation of ontogenetically young individuals. Palaeogeogr. Palaeoclimatol. Palaeoecol. 490, 404–414. doi: 10.1016/j.palaeo.2017.11.019
Richards, E. J., and Leighton, L. R. (2012). Size refugia from predation through time: A case-study of two Middle Devonian brachiopod genera. Palaeogeogr. Palaeoclimatol. Palaeoecol. 363–364, 163–171. doi: 10.1016/j.palaeo.2012.09.012
Rick, T. C., and Lockwood, R. (2013). Integrating Paleobiology, Archeology, and History to Inform Biological Conservation. Conserv. Biol. 27, 45–54. doi: 10.1111/j.1523-1739.2012.01920.x
Rick, T. C., Sillett, T. S., Ghalambor, C. K., Hofman, C. A., Ralls, K., Anderson, R. S., et al. (2014). Ecological change on California’s Channel Islands from the Pleistocene to the Anthropocene. Bioscience 64, 680–692. doi: 10.2307/90006892
Robles, C., Sweetnam, D. A., and Dittman, D. (1989). Diel variation of intertidal foraging by Cancer productus L. in British Columbia. J. Nat. Hist. 23, 1041–1049. doi: 10.1080/00222938900770951org/10.1080/00222938900770951
Roy, K., Jablonski, D., and Valentine, J. W. (1995). Thermally anomalous assemblages revisited: Patterns in the extraprovincial latitudinal range shifts of Pleistocene marine mollusks. Geology 23, 1071–1074. doi: 10.1130/0091-76131995023<1071:TAARPI<2.3.CO;2
Schindel, D. E., Vermeij, G. J., and Zipser, E. (1982). Frequencies of repaired shell fractures among the Pennsylvanian gastropods of north-central Texas. J. Paleontol. 56, 729–740.
Schindler, D. E., Johnson, B. M., Mackay, N. A., Bouwes, N., and Kitchell, J. F. (1994). Crab: snail size-structured interactions and salt marsh predation gradients. Oecologia 97, 49–61. doi: 10.1007/BF00317908
Schmidt, N. (1989). Paleobiological implications of shell repair in recent marine gastropods from the northern Gulf of California. Hist. Biol. 3, 127–139. doi: 10.1080/08912968909386517
Smith, J. R., Fong, P., and Ambrose, R. F. (2006a). Dramatic Declines in Mussel Bed Community Diversity: Response to Climate Change? Ecology 87, 1153–1161. doi: 10.1890/0012-9658(2006)87[1153:ddimbc]2.0.co;2
Smith, J. R., Fong, P., and Ambrose, R. F. (2006b). Long-term change in mussel (Mytilus californianus Conrad) populations along the wave-exposed coast of southern California. Mar. Biol. 149, 537–545. doi: 10.1007/s00227-005-0223-y
Smith, J. R., Fong, P., and Ambrose, R. F. (2008). The impacts of human visitation on mussel bed communities along the California coast: Are regulatory marine reserves effective in protecting these communities? Environ. Manage. 41, 599–612. doi: 10.1007/s00267-007-9066-2
Stafford, E. S., Tyler, C. L., and Leighton, L. R. (2015b). Gastropod shell repair tracks predator abundance. Mar. Ecol. 36, 1176–1184. doi: 10.1111/maec.12219
Stafford, E. S., Dietl, G. P., Gingras, M. P., and Leighton, L. R. (2015a). Caedichnus, a new ichnogenus representing predatory attack on the gastropod shell aperture. Ichnos 22, 87–102. doi: 10.1080/10420940.2015.1031899
Taggart, S. J., Shirley, T. C., O’Clair, C. E., and Mondragon, J. (2004). Dramatic Increase in the Relative Abundance of Large Male Dungeness Crabs Cancer magister following Closure of Commercial Fishing in Glacier Bay, Alaska. Am. Fish. Soc. Symp. 42, 243–253.
Tomasovych, A., and Kidwell, S. M. (2010). Predicting the effects of increasing temporal scale on species composition, diversity, and rank-abundance distributions. Paleobiology 36, 672–695. doi: 10.1666/08092.1
Toniello, G., Lepofsky, D., Lertzman-Lepofsky, G., Salomon, A. K., and Rowell, K. (2019). 11,500 y of human-clam relationships provide longterm context for intertidal management in the Salish Sea, British Columbia. Proc. Natl. Acad. Sci. U. S. A. 116, 22106–22114. doi: 10.1073/pnas.1905921116
Tyler, C. L., Molinaro, D. J., Mendonca, S. E., Schneider, C. L., Barclay, K., and Leighton, L. R. (2019). Repair scars preserve decadal-scale patterns of predation intensity despite short-term ecological disturbances. Aquat. Conserv. Mar. Freshw. Ecosyst. 29:3202. doi: 10.1002/aqc.3202
Valentine, J. W. (1962). Pleistocene molluscan notes. 4. Older terrace faunas from Palos Verdes Hills, California. J. Geol. 70, 92–101. doi: 10.1086/626796
Vermeij, G. J. (1982c). Unsuccessful predation and evolution. Am. Nat. 120, 701–720. doi: 10.1086/284025
Vermeij, G. J. (1982b). Gastropod shell form, breakage, and repair in relation to predation by the crab Calappa. Malacologica 23, 1–12.
Vermeij, G. J. (1982a). Environmental change and the evolutionary history of the periwinkle (Littorina littorea) in North America. Evolution 36, 561–580. doi: 10.1111/j.1558-5646.1982.tb05077.x
Vermeij, G. J. (1987). Evolution and escalation: an ecological history of life. Princeton, N. J: Princeton University Press, doi: 10.1016/s0016-6995(88)80050-0
Waycott, M., Duarte, C. M., Carruthers, T. J. B., Orth, R. J., Dennison, W. C., Olyarnik, S., et al. (2009). Accelerating loss of seagrasses across the globe threatens coastal ecosystems. Proc. Natl. Acad. Sci. U. S. A. 106, 12377–12381. doi: 10.1073/pnas.0905620106
Whetstone, J. M., and Eversole, A. G. (1981). Effects of size and temperature on mud crab, Panopeus herbstii, predation on hard clams, Mercenaria mercenaria. Estuaries 4, 153–156. doi: 10.2307/1351680
Whitaker, S. G., Smith, J. R., and Murray, S. N. (2010). Reestablishment of the southern California rocky intertidal brown alga, Silvetia compressa: An experimental investigation of techniques and abiotic and biotic factors that affect restoration success. Restor. Ecol. 28, 18–26. doi: 10.1111/j.1526-100X.2010.00717.x
Whitenack, L. B., and Herbert, G. S. (2015). Did shell-crushing crabs trigger an escalatory arms race in the aftermath of a Late Neogene regional mass extinction event? An experimental test. Palaeogeogr. Palaeoclimatol. Palaeoecol. 417, 57–65. doi: 10.1016/j.palaeo.2014.09.026
Whitney, C. K., and Ban, N. C. (2019). Barriers and opportunities for social-ecological adaptation to climate change in coastal British Columbia. Ocean Coast. Manag. 179:104808. doi: 10.1016/j.ocecoaman.2019.05.010
Wiedenmann, J., Wilberg, M. J., and Miller, T. J. (2013). An Evaluation of Harvest Control Rules for Data-Poor Fisheries. North Am. J. Fish. Manag. 33, 845–860. doi: 10.1080/02755947.2013.811128
Keywords: repair scars, crab fisheries, conservation paleobiology, historical ecology, predation traces, Caedichnus, Cancridae, durophagy
Citation: Barclay KM and Leighton LR (2022) Predation Scars Reveal Declines in Crab Populations Since the Pleistocene. Front. Ecol. Evol. 10:810069. doi: 10.3389/fevo.2022.810069
Received: 05 November 2021; Accepted: 19 January 2022;
Published: 10 February 2022.
Edited by:
G. Lynn Wingard, United States Geological Survey (USGS), United StatesReviewed by:
Geerat Vermeij, University of California, Davis, United StatesCopyright © 2022 Barclay and Leighton. This is an open-access article distributed under the terms of the Creative Commons Attribution License (CC BY). The use, distribution or reproduction in other forums is permitted, provided the original author(s) and the copyright owner(s) are credited and that the original publication in this journal is cited, in accordance with accepted academic practice. No use, distribution or reproduction is permitted which does not comply with these terms.
*Correspondence: Kristina M. Barclay, a2JhcmNsYXlAdWFsYmVydGEuY2E=
Disclaimer: All claims expressed in this article are solely those of the authors and do not necessarily represent those of their affiliated organizations, or those of the publisher, the editors and the reviewers. Any product that may be evaluated in this article or claim that may be made by its manufacturer is not guaranteed or endorsed by the publisher.
Research integrity at Frontiers
Learn more about the work of our research integrity team to safeguard the quality of each article we publish.