- 1Department of Earth and Planetary Sciences, Johns Hopkins University, Baltimore, MD, United States
- 2Northern Research Station, USDA Forest Service, Baltimore, MD, United States
- 3NYC Department of Environmental Protection, New York, NY, United States
- 4State Key Laboratory of Urban and Regional Ecology, Research Center for Eco-Environmental Sciences, Chinese Academy of Sciences, Beijing, China
- 5Department of Plant and Soil Sciences, University of Delaware, Newark, DE, United States
- 6Northern Research Station, USDA Forest Service, Newark, DE, United States
Cycling of carbon (C), nitrogen (N), calcium (Ca), phosphorus (P), and sulfur (S) is an important ecosystem service that forest soils provide. Humans influence these biogeochemical processes through the deposition of atmospheric pollutants and site disturbances. One way to study these potential anthropogenic trajectories is through long-term monitoring in association with human-caused environmental gradients such as urban-rural gradients. The objective of this study was to characterize changes in surface soil chemistry of urban, suburban and rural forest patches in the Baltimore Metropolitan area. Soil composite samples (0–10 cm) were analyzed for macro- and micronutrients, pH, and C. A total of 12 sites in forest patches dominated by white oak (Quercus alba) and tulip poplar (Liriodendron tulipifera) were established in 2001, and resampled in 2018. We hypothesized that after almost two decades (1) concentrations of N, Ca, and P, as well as soil pH would be higher, especially in urban forest patches due to local deposition; (2) S levels would be lower due to decreased regional atmospheric deposition and; (3) total soil C would increase overall, but the rate of increase would be higher in the urban end of the gradient due to increased NPP. Overall, means of Ca concentration, pH, and C:N ratios significantly changed from 2001 to 2018. Calcium increased by 35% from 622 to 844 mg kg–1, pH increased from 4.1 to 4.5, and C:N ratios decreased from 17.8 to 16.7. Along the gradient, Ca, N, P, and S were statistically significant with Ca concentration higher in the urban sites; S and N higher in the suburban sites; and P lower in the urban sites. Confounding factors, such as different geologic parent material may have affected these results. However, despite the unique site conditions, patterns of surface soil chemistry in space and time implies that local and regional factors jointly affect soil development in these forest patches. The increase in pH and Ca is especially notable because other long-term studies demonstrated changes in the opposite direction.
Introduction
Soils are considered relatively stable, barring a major disturbance or environmental change; however, in urban environments there are regional and local pressures related to air pollution, deposition, invasive species, and socio-economic factors which can affect change within relatively short periods of time. Long term research studies (>10 years) provide details of how soils are changing through time and the response of vegetation. There are few studies that use repeated soil sampling, retrospective studies, to observe temporal changes (Knoepp et al., 2019). In a world-wide inventory of over 200 long-term field studies, forest soils account for only 10%, and none of those were in urban environments (Richter and Yaalon, 2012). Some of these long-term studies have shown that the soil system is dynamic on a decadal time scale (Mobley et al., 2015). Retrospective studies, in which the researchers return to previously sampled sites several years later to resample the same location, have been conducted in Eastern US forests over various time periods (e.g., Bailey et al., 2005; Johnson et al., 2007; Bedison and Johnson, 2010; Lawrence et al., 2015). These studies are important for providing insight into the mechanisms or drivers of change as well as better predictions of future soil conditions and management planning in response to variation in climate, atmospheric inputs, vegetation composition, and microbial communities (Knoepp et al., 2019).
Natural pedogenic processes are disrupted by human influences (Bidwell and Hole, 1965). Human influence through disturbance and environmental change can lead to different trajectories of soil formation. Soil scientists are beginning to understand anthropogenic effects on soil forming processes but have little knowledge on how long it takes for urban environmental influences to elicit a measurable response in soil chemical properties. Urban-rural gradients have been used to investigate the urban context and its effect on ecosystems (McDonnell and Pickett, 1990) and provide useful data to frame additional hypotheses or to test management strategies (Pouyat et al., 2019). To further support management strategies, adding a temporal component to existing urban-rural gradients provides a new dimension to assess the effects of local and regional environmental factors on soil characteristics.
Local and regional environmental factors affect soil chemical properties such as nutrient concentrations, soil organic matter, and pH (Rustad et al., 2020). In atmospheric modeling studies “regional” is defined as 5–50 km and “local” as 1 km and street (Thunis et al., 2016). Regional factors, including natural soil forming factors, atmospheric deposition from distant sources or background deposition would impact soils at the metropolitan scale. At the finer scale, local factors such as parent material, site history, invasive species, polluting source or local deposition due to building construction or traffic, could produce different soil conditions, for example at forest patch scale (Trammell et al., 2021). Due to local and regional environmental effects, soil chemical properties may change over time. Many of these, such as carbon (C), nitrogen (N), sulfur (S), phosphorus (P), calcium (Ca) and pH are important factors for plant growth, microbial and faunal diversity, and overall soil health.
Acid precipitation is one example of a long-term regional effect that influenced soil nutrient concentration in the not-too-distant past and led to forest health decline. Soil pH affects all soil properties: chemical, physical, and biological (Weil and Brady, 2017). Acid deposition in the past led to soil acidification, lowering soil pH and decreasing exchangeable calcium (Kolb and Mccormick, 1993; Huntington et al., 2000; Bailey et al., 2004; Jenkins et al., 2005), magnesium, and potassium, thereby affecting forest productivity (Adams et al., 2000). This depletion of nutrients, especially Ca, has stressed forest trees, such as sugar maple (Kolb and Mccormick, 1993; Horsley et al., 2002; St Clair et al., 2008; Richardson and Friedland, 2016) and increased freezing injury in red spruce in the northeastern United States (Schaberg and DeHayes, 2000). The Clean Air Act Amendment of 1990 has led to reduced S deposition (less so for N) and today, the acidification of soil may be reversing in some areas (Lawrence et al., 2015). In urban environments, this accelerated depletion of cations from acid rain may be counterbalanced by atmospheric deposition of some cations such as Ca, which is derived from concrete and wallboard (Kuang et al., 2004; Nath et al., 2007). Gradient studies have shown higher Ca bulk deposition at the urban end of the gradient compared to their rural counterpart soils (Lovett et al., 2000; Pouyat et al., 2008).
Unlike S, there are no common atmospheric gases containing P, however, atmospheric P in the form of particulate matter is deposited to the tree canopy (Newman, 1995), ultimately ending up in the soil. Urban areas have higher rates of atmospheric deposition of P compared to rural areas (Eisenreich et al., 1977). Soil P is affected by urbanization (Chen et al., 2010b,2014; Metson et al., 2015; Yesilonis et al., 2016) with higher P soil concentrations in urban soils of various cover types and land uses compared to rural soils (Zhang, 2004; Yuan et al., 2007; Chen et al., 2014; Foti et al., 2020). Comparisons of P concentrations in urban and rural forest soils yielded contradictory results with urban forests soils having higher (Zhang, 2004; Chen et al., 2010b) or lower (Baxter et al., 2002) P level that the rural forest patches.
Carbon and nitrogen pools have been shown to vary by annual (Knoepp and Swank, 1997) and decadal (Johnson et al., 2007) time scales. Urbanization adds another level to an already complex soil C and N temporal dynamic. The net effect of urban environmental interactions can either increase or decrease soil C pools; for example, plant growth, or net primary productivity (NPP) (Ziska et al., 2003), which ultimately affects soil C, may increase due to urban environmental factors. Passive recalcitrant pools of C were higher in urban forest soils compared to rural soils (Groffman et al., 1995). Black carbon, a component of the passive pool, from the burning of fossil fuels is enriched in urban soils (Wang, 2010). Even with the complex interaction of the urban environment, gradient studies have not shown differences in soil C concentrations (Pouyat and Carreiro, 2003; Chen et al., 2010a).
In urban areas, forest systems can experience N saturation where there is increased N atmospheric deposition (Agren and Bosatta, 1988; Aber et al., 1989, 1991). However, soil total N does not always reflect this deposition due to increased soil leaching caused by N-induced acidification (Huang et al., 2012), and possible soil compaction and management practices (Chen et al., 2010a). Gradient studies have shown rural sites higher in total N than urban sites (Huang et al., 2012) or no difference in total N (Pouyat et al., 1995).
Urban, suburban, and rural forest soils were examined in Baltimore City and Baltimore County, Maryland, United States to investigate soil changes of total C and N; nutrients P, S, and Ca; and pH over 17 years. Forest patches in Baltimore make up 34% of the City’s tree canopy. These considerable green spaces provide important ecosystem services, such as stormwater runoff reduction, water purification, food and habitat for animals, air temperature reduction, and C sequestration. Surface soil properties of 15 forest patches, arranged along an urban-rural gradient in Baltimore City and Baltimore County were assessed in 2001. In 2018, twelve of these sites were revisited and soils resampled.
The study objectives were to examine changes in forest soil properties over 17 years and to relate these changes to anthropogenic and natural soil forming factors. Regional and local effects should be more easily seen in forests compared to other land uses that have direct management and inputs, e.g., turf-grass parks or residential lawns. In general, we expected greater changes in the urban end of the gradient because local effects can induce more rapid shifts in soil conditions. In contrast, national policies that have influenced regional effects, i.e., reductions in S emissions, would impact all of patches along the urban-rural gradient.
We hypothesized that after almost two decades (1) concentrations of N, Ca, and P, as well as soil pH would be higher, especially in urban forest patches due to local deposition; (2) S levels would be lower due decreased regional atmospheric deposition and; (3) total soil C would increase overall, but the rate of increase would be higher in the urban end of the gradient due to increased NPP. These hypotheses were tested by comparing 2001 soil chemical properties to 2018. Similar concentrations between the years across the gradient imply environmental factors did not change or changed similarly at the local (urban vs. suburban vs. rural contexts) or regional (same across all sites) scale. However, concentration differences between the years along the gradient imply local effects in at least one of the land use contexts or regional effects over all the land use types caused the change.
Materials and Methods
Study Area
Study sites are located in Baltimore City and Baltimore County, Maryland. This area lies within the upland portion of the Piedmont Plateau Physiographic Province (Edwards, 1981). The Piedmont Plateau is composed of hard, crystalline igneous and metamorphic rocks and extends from the inner edge of the Coastal Plain westward to Catoctin Mountain. The upland portion of the Plateau consists of schist, gneiss, gabbro, and other highly metamorphosed sedimentary and igneous rocks. In several places these rocks have been intruded by granitic plutons and pegmatites. Several domal uplifts of Precambrian gneiss mantled with quartzite, marble, and schist are present.
Mean monthly temperatures range from 5 to 31°C with an average rainfall of approximately 109 cm per year (NOAA, 2000). Soils in the area generally belong to the Manor-Glenelg and Urban Land-Legore Associations (Reybold and Matthews, 1976; Levin and Griffin, 1998). Manor-Glenelg soils are deep, well drained to somewhat excessively drained with a subsoil of loam to light silty clay loam. Urban Land-Legore soils are deep, well-drained, loamy soils that overlie semi-basic or mixed basic and acidic rock. Other series present in the study include Jackland Silt Loam and Relay Silt Loam.
Site Selection and Location
The sites used in this study have been selected for other studies to investigate soil nutrients, metals, earthworms, and nitrification rates along the urban-rural gradient. It was found that Pb and Cu decreased in soil concentration from the city core (Pouyat et al., 2008) and that the density and biomass of earthworms, dominated by European Lumbricidae, were higher in urban forests than rural forests along with higher potential N-transformation rates (Szlavecz et al., 2006). Potential study sites were selected using aerial photographs, topographic maps, and prior knowledge of the Baltimore metropolitan area. To reduce sources of variation, the following criteria were used to screen potential sites prior to inclusion in the study: (1) size ≥2 hectares, (2) overstory dominated by white oak (Quercus alba) and tulip poplar (Liriodendron tulipifera), (3) slopes <25%, and (4) an age of 50+ years with no visual signs of recent human disturbance and intensive management. Unlike managed parks with turf grass and planted trees often pruned and fertilized, our sites are patches of contiguous forest. Trees are only cut if they fall on a trail or a pavement at the edge.
A total of 15 sites met the selection criteria and were included in the 2001 study. Once selected for inclusion, each site was classified as urban, suburban, or rural based on distance from the city center (Inner harbor) and type of development in the surrounding area. The land-use types corresponded generally to Anderson Level II classes (Anderson et al., 1976). Urban sites were located within 9 km of the city center and surrounded by high-density residential (multiple-unit structures) and commercial structures; suburban sites were located between 10–19 km from the city center and surrounded by mid- to low-density residential and commercial structures; and rural sites were located >19 km from the city center and largely surrounded by agricultural land and low-density residential structures (houses on lots larger than an acre).
This urban-rural gradient design is confounded by different parent materials underlying the forest patches at either end, due to Baltimore’s complex geologic formations. All the urban plots are located on Baltimore gabbro complex while only one suburban site is located on this complex. The other suburban sites are located on Baltimore Gneiss and the Potomac group which consist primarily of variegated silt clay and mostly fine to medium sands. The rural sites are underlain by Lower peltic schist and Baltimore gneiss.
In 2018, 12 sites were revisited and sampled for soils (Figure 1); Gunpowder Falls and Druid Hill Forest were excluded because of original datasheet location ambiguity and Cross Country was excluded because generally it was a riparian site with noticeable signs of flooding. Soils were sampled in July in both years. Since the first sampling took place before accessible GPS, original hand drawn field maps, field notes, and the recollection of one of the coauthors who conducted the study in 2001, were referenced to find the original plot locations. This ensured that collection location and methods were comparable.
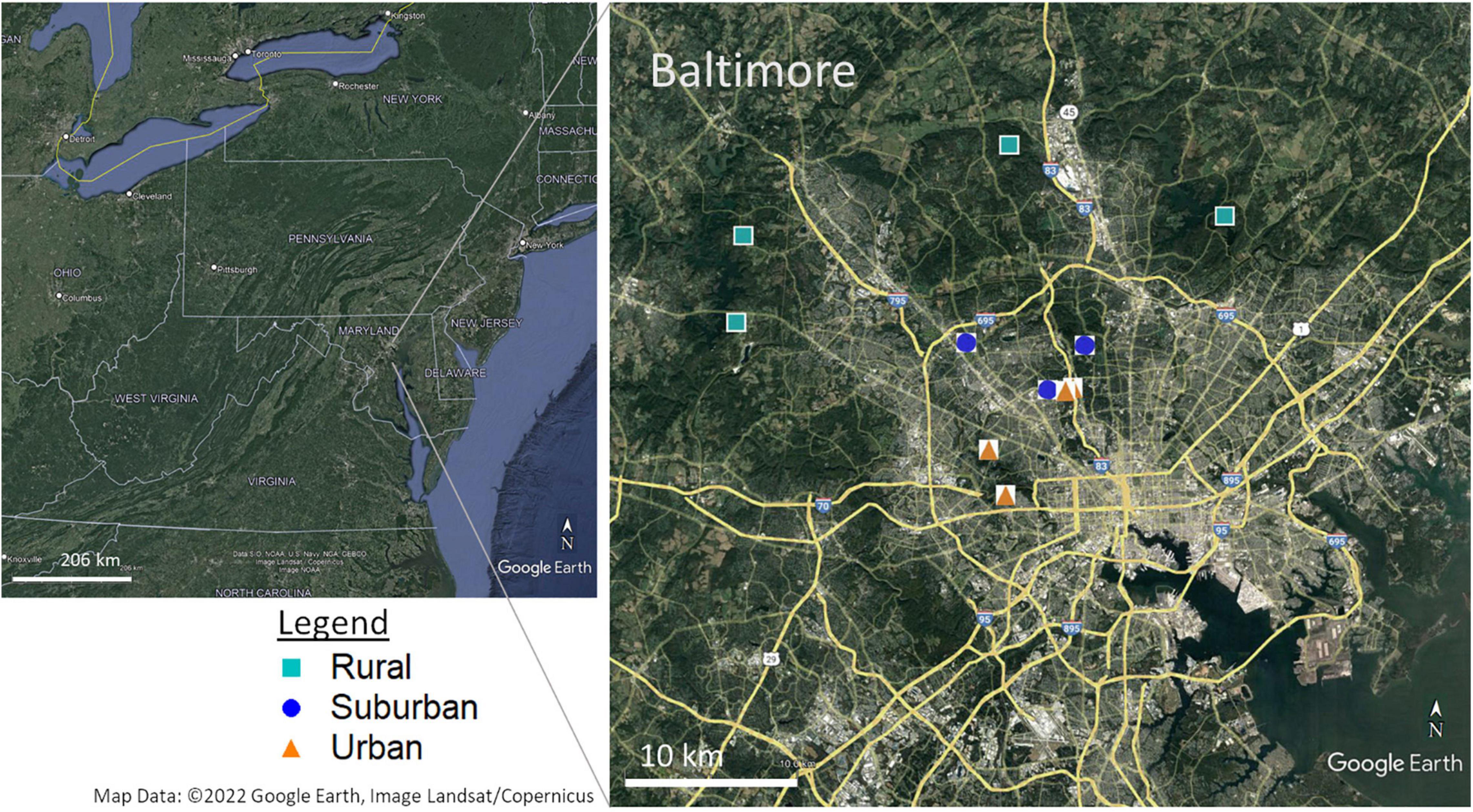
Figure 1. Locations of urban, suburban and rural forest patches in Baltimore City and county. Each symbol indicates a forest patch (n = 12). Within each patch three plots were established.
Soil Sampling
At each site, a series of circular plots were established (11 m radius). Three plots were established for sites <100 ha in size and five plots were established for sites >100 ha. At each plot, one composite soil sample (0–10 cm) containing six subsamples using a 5 cm diameter stainless steel soil corer in 2001 and a 3 cm diameter stainless steel soil corer in 2018. A soil depth of 10 cm roughly corresponded to the division between A and B horizons.
Soil Processing and Analysis
The 2001 soil samples were stored in labeled Whirlpack® bags in banker boxes in a dark cool moisture-free room for 17 years. In 2018, both the archived 2001 samples and 2018 samples were digested using the same method done in 2001. Re-digesting archived samples reduces uncertainty by controlling for analytical bias (Lawrence et al., 2016). All data presented in this paper are from soils analyzed in 2018, i.e., 2001 soil samples were digested in 2018. Mineral soil samples were air-dried and sieved using a 2 mm screen. Prior to analysis, samples were further dried in an oven for 1 week (70°C). Soil pH was determined by glass electrode using an Orion pH meter using 1:2 soil-to-0.01 M CaCl2 solution. Organic matter content was determined by loss on ignition (470°C for 24 h) and calculated as a percent initial weight. Subsamples were ashed for 4 h at 475°C, hot digested with 7.7 N HNO3 for 25 min, filtered through #42 Whatman paper and brought up to 50 ml volume with DI water. Leachates were analyzed in the Cornell Nutrient Analytical Laboratory (3020-Elements in Digest) using an inductively coupled plasma optical emission spectrophotometer (SPECTRO CIROS CCD, Spectro Analytical Instruments, Kleve, Germany). Elemental data are presented as a concentration per dry weight of soil. Mineral soil was analyzed to determine acid soluble Ca, Fe, K, Mg, Mn, Na, P, S, and Ti. Nutrients of Ca, P, and S were used for our analysis because each could be anthropogenically deposited onto the soil surface. For carbon and nitrogen, both archived 2001 and recently sampled 2018 soils were finely ground, homogenized, and dried at 60°C before analysis and analyzed on a Thermo Flash EA 1112 Series NC Soil Analyzer.
Statistical Analysis
The data analysis for this paper was generated using SAS software, Version 9.4 (SAS, 2021). To determine the statistical difference between the years 2001 and 2018, gradient, and the interaction term of year × gradient, PROC GLIMMIX was used. Year was used as the repeated measure or the random factor and the distribution was considered normal. To visualize the differences in soil nutrients and metals among land use, principal component analysis (PCA) using PC-Ord. Correlations and box plots were created at the plot level using R version 3.6.0 software. Since changes could be seen at the plot level, plots were used to create the figures. For all the ANOVA’s, site level data was used to avoid pseudo-replication and artificially increasing statistical power.
Results
To investigate the general patterns of some of the soil nutrient data, principal component analysis (PCA) revealed a separation between urban, suburban, and rural plots using the soil variables Ca, P, S, C, N, SOM, and pH from the 2001 and 2018 soil samples (Figure 2). For 2018, the first factor (PC1) loading on S, C, N, and SOM explained 52% of the variation of the dataset and seemed to separate the suburban from the urban and rural plots. The second factor (PC2) loading on pH and Ca explained 28% of the variation of the dataset and seemed to separate urban from rural plots in 2018. For 2001, the same variables loaded on the same factors (PC1 and PC2), but the variation explained was marginally different at 54% for PC1 and 22% for PC2.
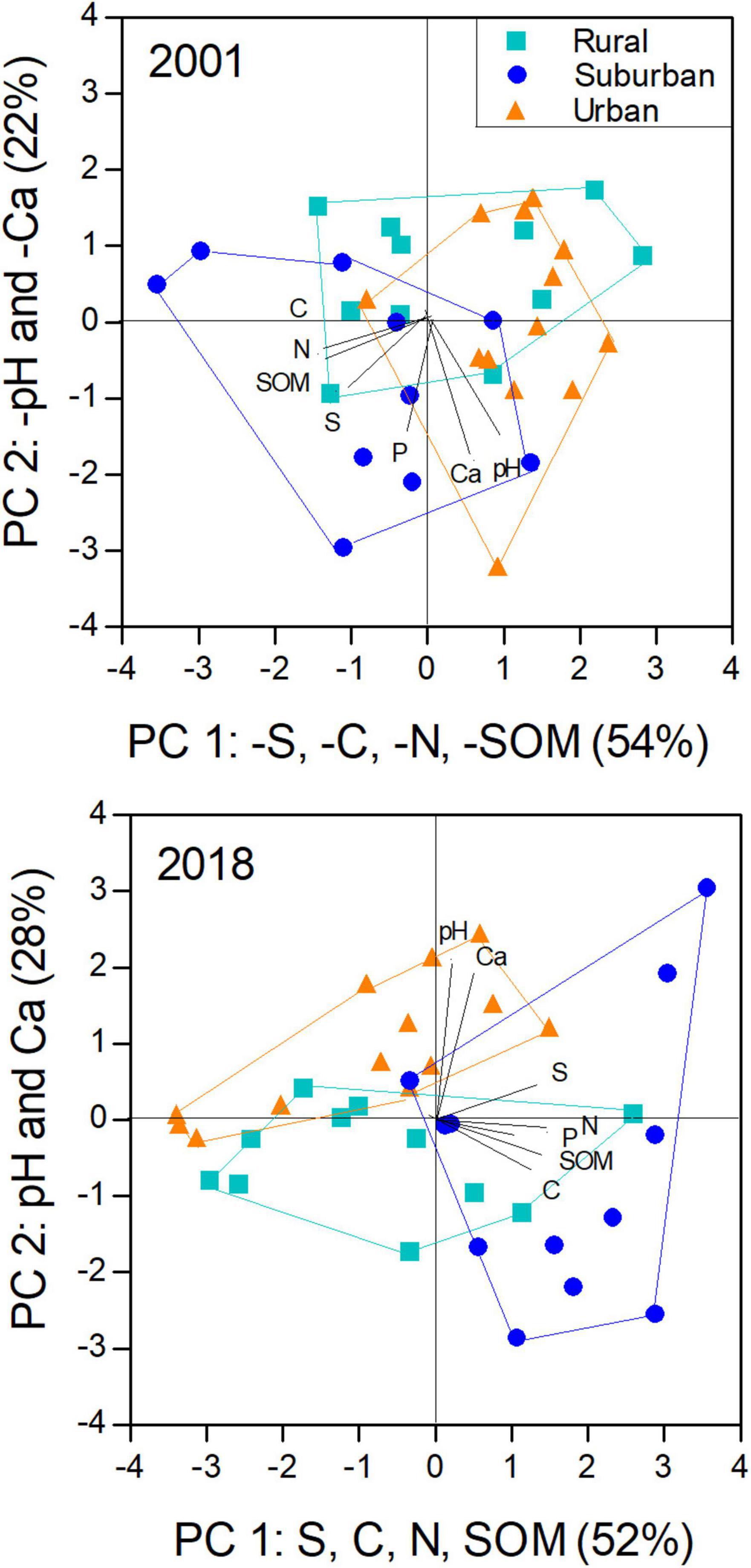
Figure 2. Principal Component Analysis indicates separation of urban, suburban and rural forests plots (n = 36). The soil variables Ca, P, S, C, N, SOM (soil organic matter), and pH were used from 2001 and 2018.
Overall means of Ca concentration, pH, and C:N ratios changed from 2001 to 2018. There were no significant changes for C, N, P, and S over this time period at P < 0.05. Calcium increased 35% (P = 0.01), pH increased 8.7% (P = 0.002), and C:N ratios decreased (P = 0.006) (Table 1). As for the gradient results, Ca, N, P, S, and SOM were significant, meaning that one of the locations along the gradient, i.e., urban, suburban, or rural, was different from the others.
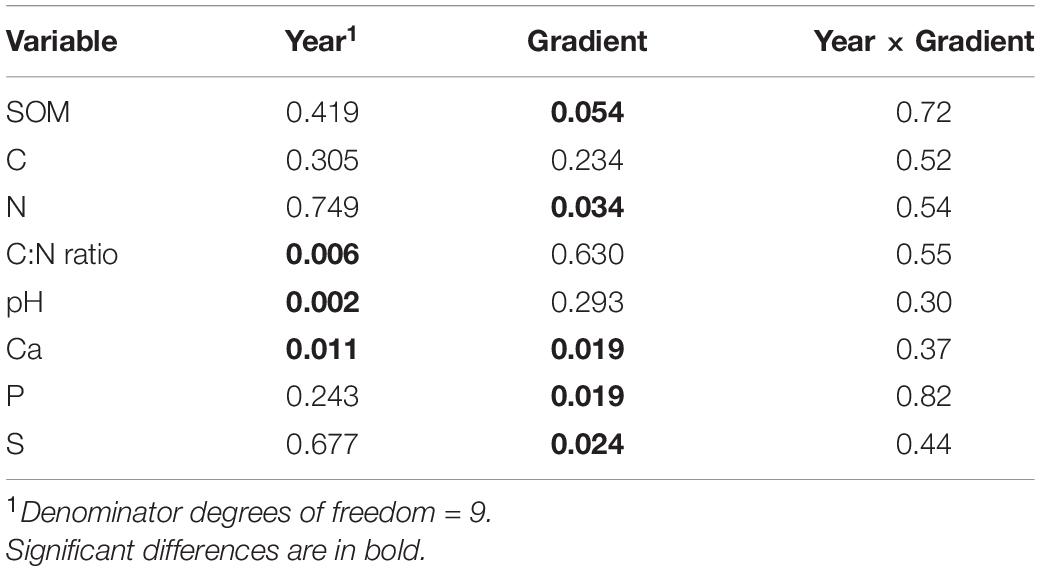
Table 1. P values for repeated GLMM (SAS GLIMMIX) where “year” is for the years 2001 and 2018, “gradient” is the urban-rural gradient, and “year × gradient” is the interaction between year and gradient, i.e., urban 2001 versus urban 2018, at the site level (n = 12 sites).
Even though differences were found related to regional effects (temporal differences of soil chemicals) and to local effects (differences along the gradient), there were no significant interactions for any of the variables, i.e., there were no differences at only one part of the gradient between years. In other words, the local effects did not produce an increase of a nutrient between the years at a rate different from another part of the gradient. Two of the three hypotheses were based on change that was supposed to be different for the urban areas compared to the rural areas, but none of those interactions were significant. Even though the data statistically did not support the hypotheses, there were trends.
Calcium and pH Increased After 17 Years
Of all the nutrients, Ca concentration showed the greatest differences between the years 2001 and 2018, with an overall mean increase from 622 ± 112 mg kg–1 in 2001 to 844 ± 154 (P = 0.011). The soil Ca concentration increased, although not statistically significant, from 2001 to 2018 in the urban sites from 932 ± 111 mg kg–1 to 1,238 ± 228 (Figure 3) which is a 33% increase relative to 2001 while the suburban had the greatest percentage jump at 58% followed by 15% increase in the rural sites. The gradient was also significant, where the urban (1,085 ± 131 mg kg–1) was 65% higher than the rural (381 ± 74) (P = 0.019).
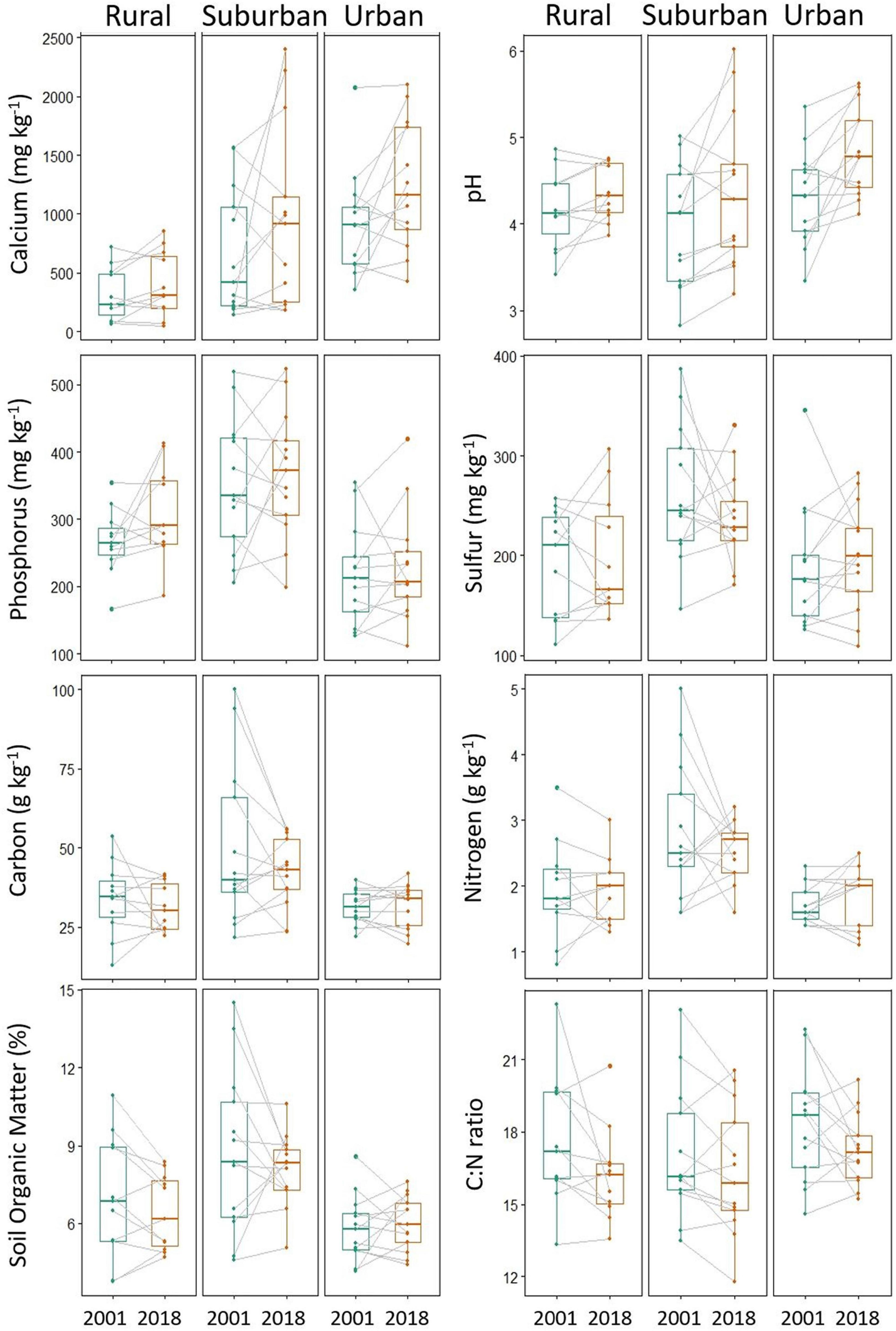
Figure 3. Change in eight soil properties between 2001 and 2018 along the urban-rural gradient. Box plots show changes at the plot level (n = 36) separately for rural (R), suburban (S), and urban (U).
The geology of the sites along the gradient may not be completely responsible for this change in Ca (Supplementary Figure 1). The greatest change in Ca between the years is found on gneiss rock type (rural and suburban sites) which is low in Ca, not the gabbro complex (all the urban sites and one suburban site) which is higher in Ca.
Because Ca is tightly coupled with organic matter (Likens et al., 1998), Ca concentrations were normalized to OM (using loss of ignition) which revealed a 1:1 relationship with the rural sites (r2 = 0.75, P = 0.0005) but less so for urban (r2 = 0.079, P = 0.35) and suburban (r2 = 0.23, P = 0.11) sites which show a greater deviation from the 1:1 line (Figure 4). For the urban sites, there is a higher ratio in 2018 than in 2001. Furthermore, there are low regression values between Ca and SOM (Figure 5).
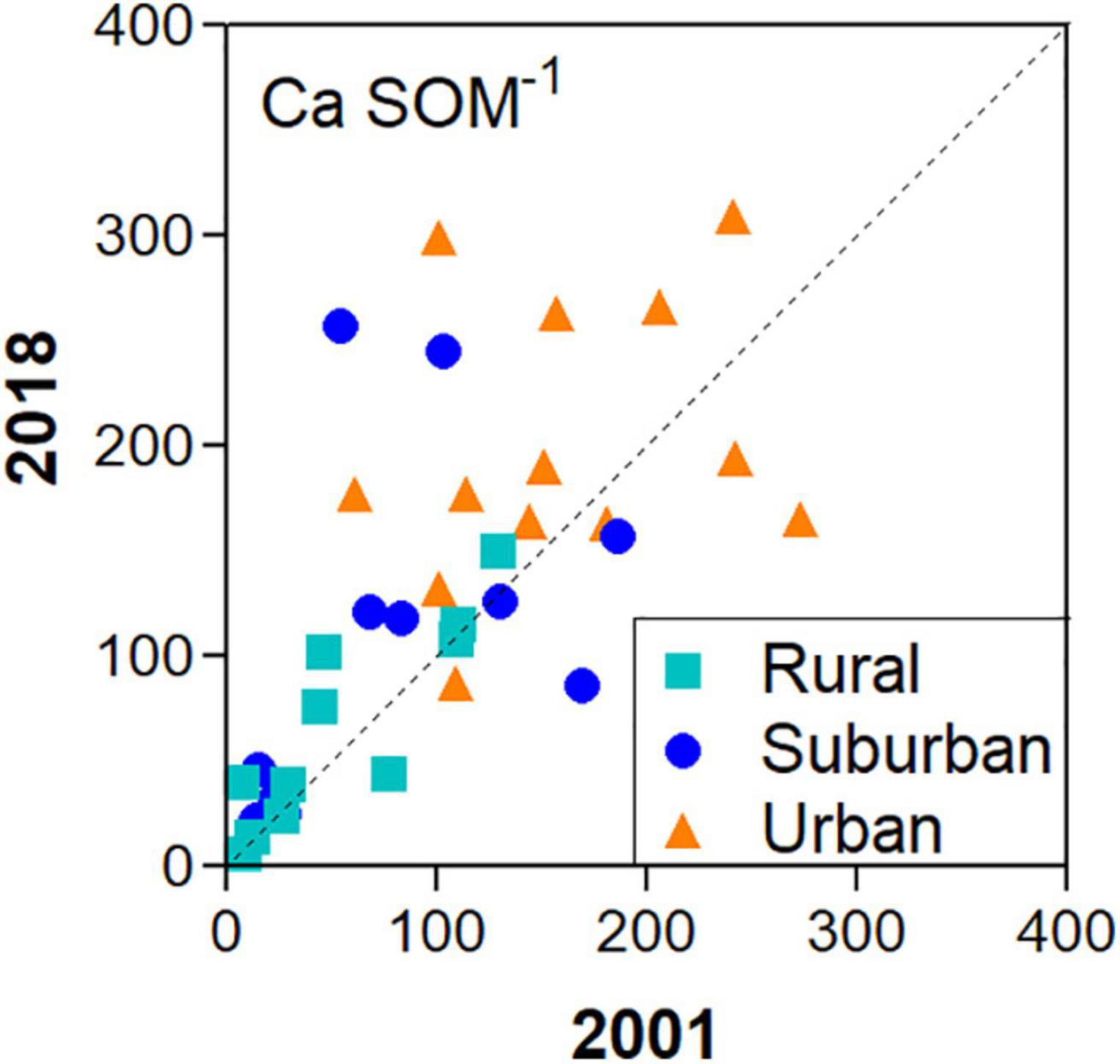
Figure 4. Correlation between surface soil calcium levels in 2001 and 2018. Ca concentrations are normalized by soil organic matter (SOM). Corresponding values are shown at the plot level (n = 36). The relationship is significant in the rural sites (r2 = 0.75, P = 0.0005), but not in the suburban (r2 = 0.23, P = 0.11), and urban (r2 = 0.079, P = 0.35) sites. The dotted line is the 1:1 line.
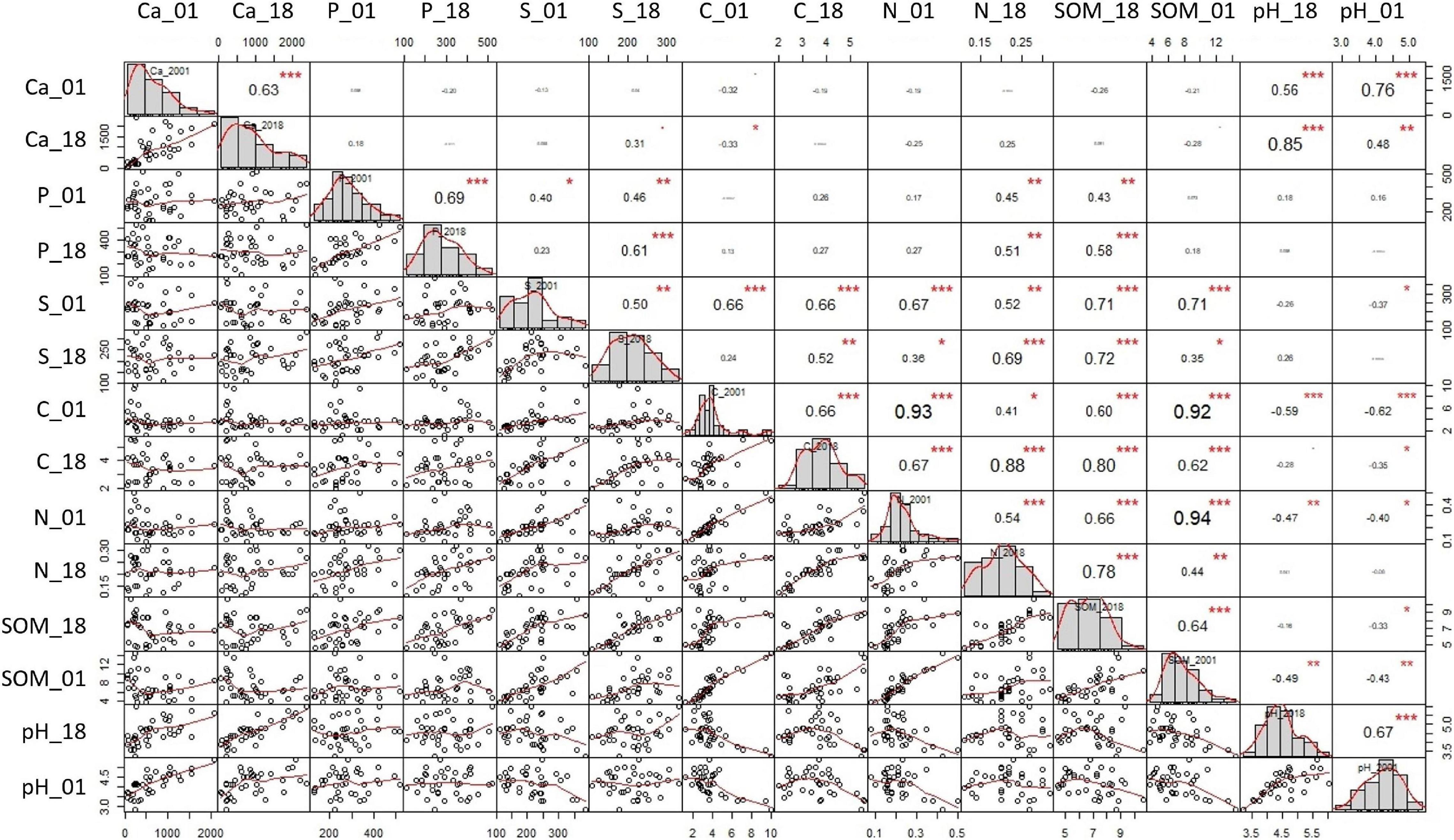
Figure 5. Summary of all surface soil variables collected in 2001 (_01) and 2018 (_18) at the plot level (n = 36). Diagonal: histograms showing variability of each dataset. Lower left: correlation among all variables. Upper right: Pearson’s correlation coefficient. Significant correlations are in bold; *, **, and *** indicate P: < 0.05. < 0.01, and <0.001, respectively.
To further investigate calcium sources, corresponding concentrations of nutrients from possible sources were examined with the idea that strong relationships between certain elements would imply sources. For example, calcium increased in 2018 from 2001 but there was not a corresponding increase in Mg or S (Supplementary Figure 2). Mg shows a strong 1:1 relationship between the years except for two urban plots. A similar relationship is found between Ca and SO42–.
As soil Ca concentrations increase, Ca replaces H ions on the soil particle surfaces. This relationship is most evident in the urban sites, which accumulated Ca ions and lost H ions (Figure 6A). Urban plots accumulated Ca from 0–275% and correspondingly decreased in H ions from 40–98%. Calcium was correlated with pH in both years, e.g., in 2018, r = 0.85 and in 2001, r = 0.76 (Figure 5). The pH increased from 4.14 ± 0.14 in 2001 to 4.5 ± 0.16 in 2018, representing 8.7% increase in pH reflecting a 56% decrease of H ions (Figure 6B).
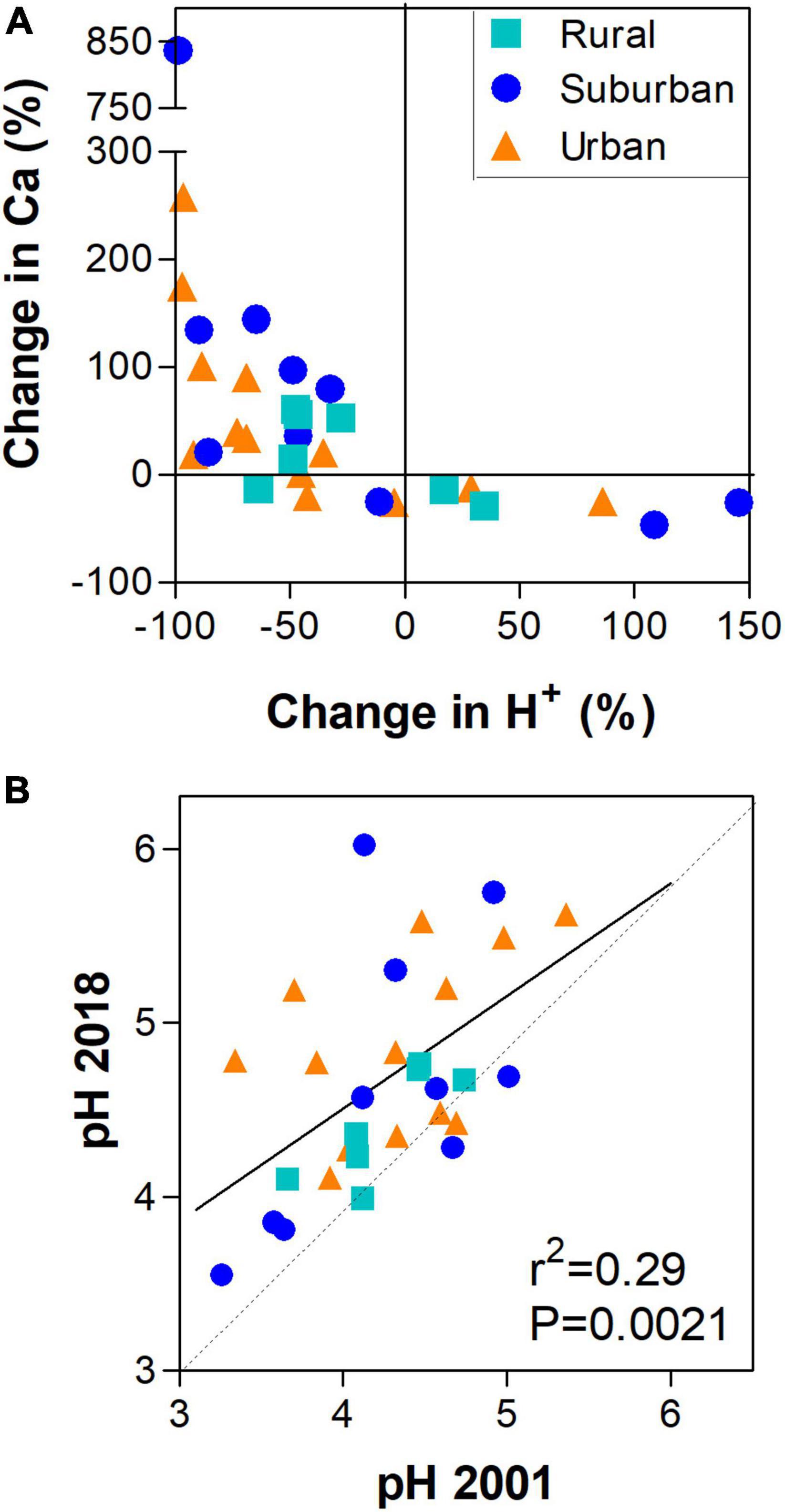
Figure 6. Change in surface soil calcium levels and pH in urban, suburban and rural forest patches. (A) Change in Ca and change in H+ concentrations from 2001 to 2018. Points represent plot level data (n = 36). (B) Correlation between soil pH in 2001 and 2018 at the plot level (n = 36). The dotted line is the 1:1 line and the solid line is the regression line.
Sulfur and Phosphorus Concentrations Were Highest in the Suburban Land Use
Sulfur and phosphorus concentrations did not significantly change after 17 years; however, means along the gradient were significantly different. Sulfur and phosphorus had relatively high concentrations in the suburban sites and both were strongly correlated with soil organic matter. For sulfur, the suburban land use type was significantly different than the U and R land use types (P = 0.024), with the concentration of 258 ± 15 mg kg–1 being 24 and 26% higher, respectively. Similarly, for phosphorus, the suburban and rural land use types were significantly higher than the urban (P = 0.019) type at 363 ± 28 and 287 ± 16 mg kg–1, respectively compared to 220 ± 15 mg kg–1. Both P and S are correlated with SOM (Figure 5) so it is not surprising that the change in S and P over the 17 years and the change of SOM over the 17 years are correlated (Figure 7). The distance from the city showed a polynomial fit for both S, P, and SOM (Figures 8A,B,D). For Ca, a log transformation of the data and distance was the best fit (Figure 8C).
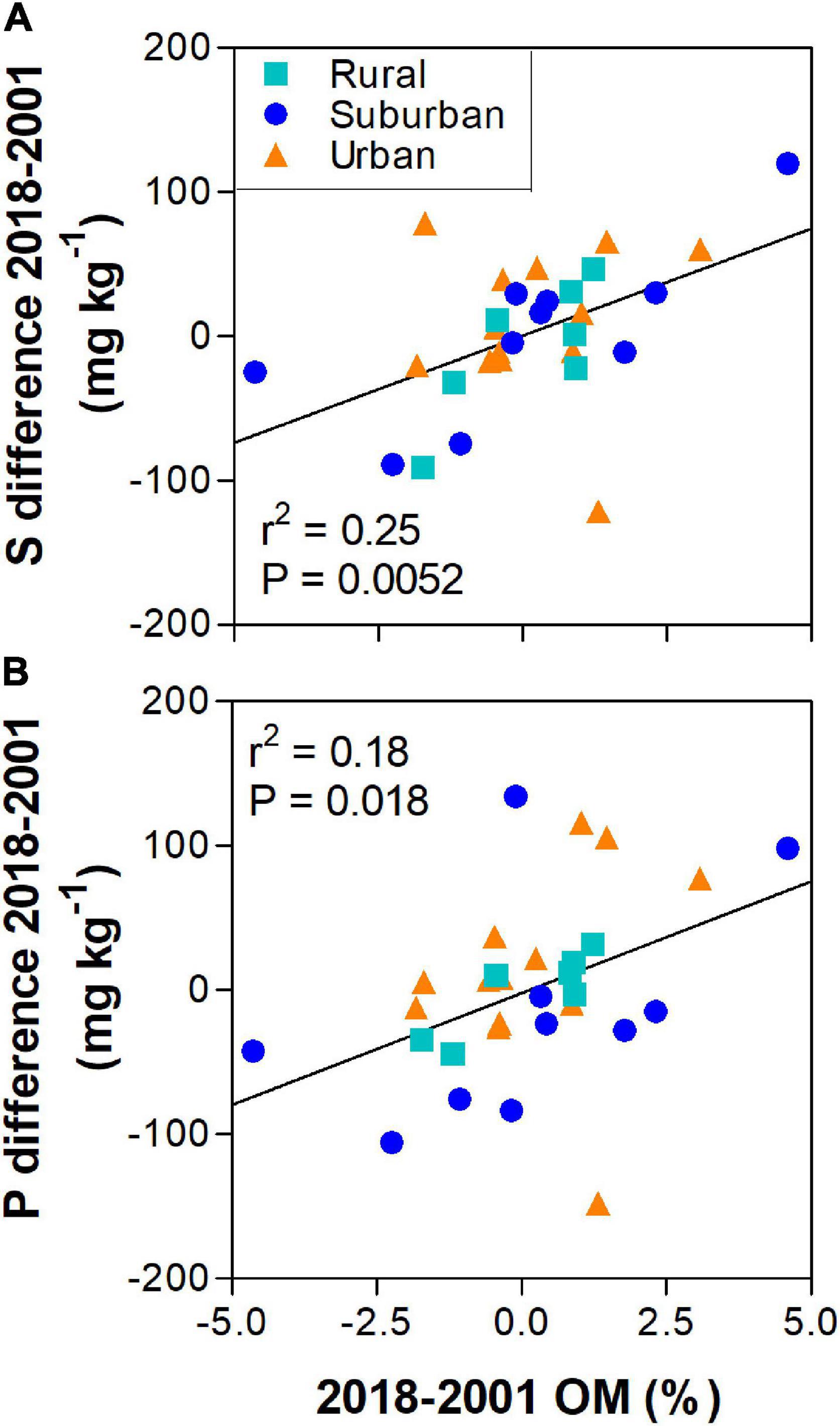
Figure 7. Correlation between the change in sulfur (A), phosphorous (B) and the change is soil organic matter from 2001 to 2018. Points represent plot level data (n = 36).
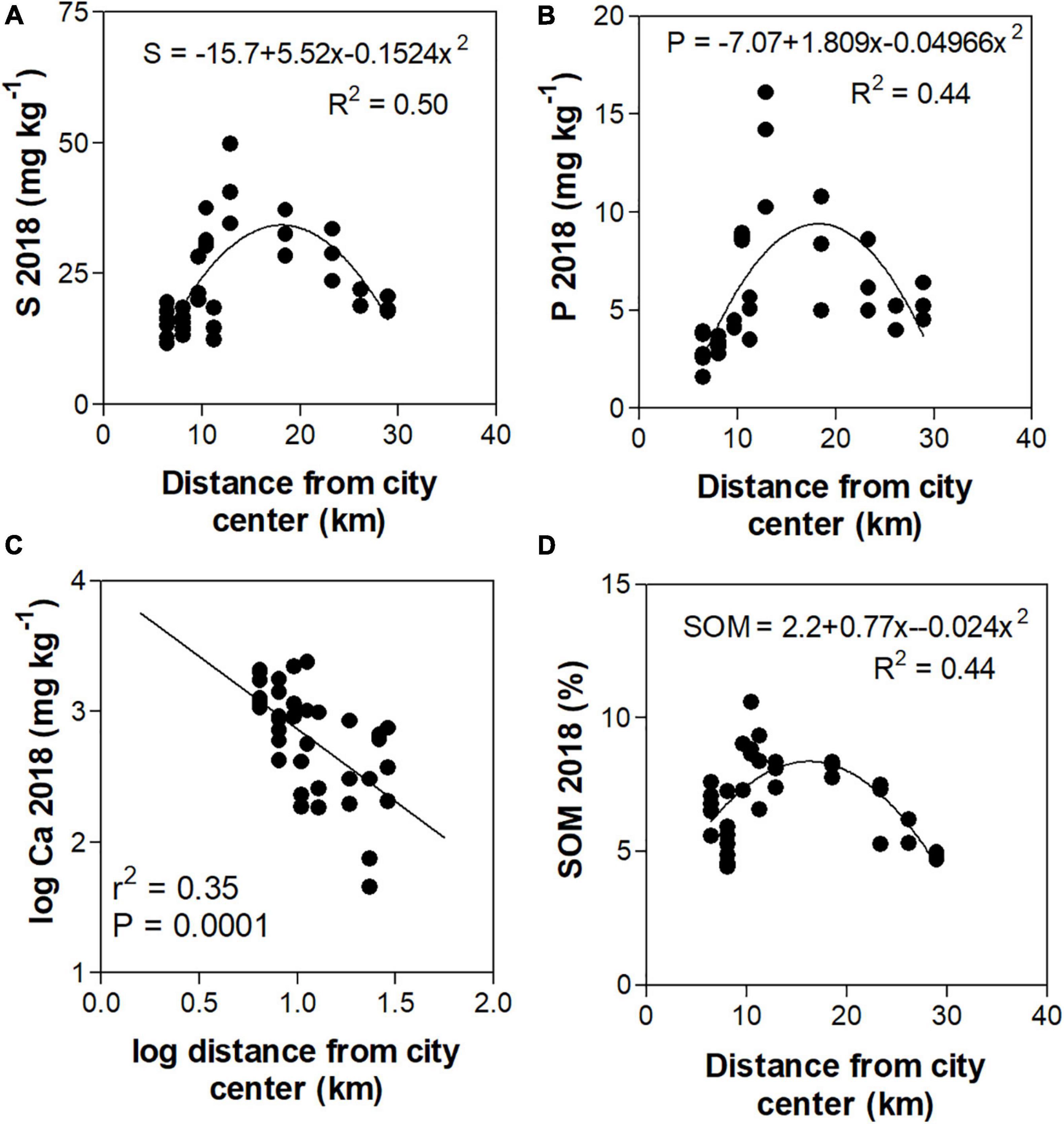
Figure 8. Concentration of some four surface soil characteristics along the urban–rural gradient, expressed as the distance from the city center. Points represent plot-level data (n = 36). Only 2018 data are shown. Equations for S (A), P (B), and SOM (D) represent polynomial best fit, and Ca (C) is represented by linear regression of log-transformed Ca concentration and distance from the city center.
Soil Organic Matter Was Highest in the Suburban Land Use
As with S and P, C and N may be in a steady state, or there is high variability, because concentrations have not changed from 2001 to 2018 (Supplementary Figure 3). There seems to be an overall decrease in carbon, but not statistically significant from 3.9% ± 0.50 to 3.6 ± 0.24, which is also reflected in lower organic matter concentrations from 7.3% ± 0.69 to 6.9 ± 0.41. Nitrogen did not show change at 2.1 g kg–1 for both years. However, even with these stable C and N concentrations between the years, the C:N ratios were statistically significant (P = 0.006) at 17.8 ± 0.56 in 2001 and 16.7 ± 0.47 in 2018 which is a 5.9% decrease in the ratio.
The gradient was statistically significant for N (P = 0.034), where the suburban was approximately 30% higher at 2.7 ± 0.24 g kg–1 than both the urban and rural, 1.8 ± 0.092 and 2.0 ± 0.19 respectively. However, neither C or C:N ratios were significant for the gradient. SOM has lowest concentrations in the urban sites (P = 0.054) compared to suburban, 6.0% ± 0.26 for the urban site which is 45% lower that the suburban sites, 8.7 ± 0.72.
Discussion
Regional and local environmental factors affect soil chemical properties such as nutrient concentrations, soil organic matter, and pH. Over time these factors can create a measurable change in soil conditions along urban-rural environmental gradients (McDonnell and Pickett, 1990). We examined soil properties after two decades along an urban-rural gradient and the results show that surface soil Ca, pH, and C:N ratios are changing over time (Table 1 and Figure 3). The general premise for resampling was the idea that the soils in the urban environment would show measurable change after 17 years via local effects (hypothesis 1 and 3), e.g., urban 2018 would have a higher C, Ca, N, and P concentrations compared to the urban 2001 concentrations, while their rural counterparts would not show any measurable change after only 17 years, and suburban soils fall in the middle. The inference is that these urban environmental pressures would result in relatively rapid changes in soil chemistry. Unfortunately, this was not found to be statistically significant (p < 0.05). The third hypothesis was not confirmed either, meaning that S did not decrease over the years due to decreased regional effects, e.g., atmospheric deposition. While differences between the years were not detected at the urban end of the gradient for these nutrients, it is predicted if current trends continue, clearer patterns and significant differences will emerge. However, along the gradient, P (urban concentration was lower than in suburban and rural sites), S (suburban higher than urban and rural), Ca (urban higher than rural), N (suburban higher than rural) and SOM (suburban higher than urban) were significantly different. The general patterns of the 2001 and 2018 soil concentration data of the PCA analysis showed S, C, N, and SOM separating suburban plots from urban and rural plots; and pH and Ca separating urban and rural plots (Figure 2).
Regional Effect Between the Years
In our study, calcium concentration and pH of surface soils increased and C:N ratio decreased over 17 years. Soil Ca concentrations are a result of native parent materials, acidic deposition, and Ca deposition. A regional effect would be an overall reduction in acidic deposition (mostly from S), while local factors would include Ca deposition (urban dust and construction activities) and S emissions from diesel. Since the pH of the soil is closely linked to the concentration of Ca2+, Mg2+, and other cations, the addition of Ca subsequently raises pH, for example, in Germany, a repeated liming study from 1983 to 2015 increased pH in the mineral soil by 0.3–1.2 pH units (Jansone et al., 2020). We found a pH change at the lower end of that range, an increase of 0.4, suggesting Ca is an important component responsible for this change. The soil pH affects the health and establishment of both flora and fauna, including crop yields, crop suitability, plant nutrient availability, and soil micro-organism activity, which influences key soil processes. For example, soil pH has been shown to be strongly associated with the establishment of invasive vegetation in forest patches (Trammell et al., 2021).
Most previous studies of forest soil in the Atlantic region reveal a decrease of Ca concentrations and pH in the surface horizon (Knoepp and Swank, 1994; Blake et al., 1999; Bailey et al., 2005; Bedison and Johnson, 2010). In the Adirondacks mountains, New York, calcium significantly decreased in surface layer (0–10 cm) from 1932 to 1984 (52 years) and 1932 to 2006 (74 years) (Bedison and Johnson, 2010). Further south in the Allegheny National Forest of Pennsylvania, but still north of the Baltimore sites, from 1967–1997 (30 years), research found significant decreases in exchangeable Ca and Mg concentrations and pH (Bailey et al., 2005). And, finally, sites south of Baltimore, at Coweeta Hydrologic Laboratory in western North Carolina from 1970–1990 (30 years), research showed the A horizon (0–12 cm) decreased in pH, Ca and Mg (Knoepp and Swank, 1994; Table 2). At a higher latitude and different continent, at the Rothamsted Experimental Station, United Kingdom, the pH of the surface soil of Geescroft Wilderness plots (Quercus robur) with a distinct 2–3 cm layer of accumulated litter and little ground vegetation cover had fallen from 6.2 to 3.8 from 1883 to 1991 (108 years) (Blake et al., 1999). A review of soils in North America has shown a general trend of lower pH and Ca values over time (Lawrence et al., 2013). More recent studies, however, have found that the current trend in the Northeastern United States is the increase, not decrease, in pH in the O and B horizons (the surface A horizon was not discussed) suggesting the reversal of forest-soil acidification due to a decrease in sulfate deposition (Lawrence et al., 2015) which supports the recovery of Ca in soils as a regional effect.
We acknowledge that change in soil pH might be an artifact of long-term soil storage. There are documented soil storage transformations related to ionic activity and oxidation of organic matter (Bartlett and James, 1980). Prodromou and Pavlatou-Ve (1998) found that the pH for topsoils (0 ± 30 cm) after 20 years of air-dry storage had decreased 0.3 units in soils that were acid to neutral pH. Another study showed stored samples with an original pH < 5.5, which are similar to our soil pH values, from Rothamsted experiments, changed a maximum of only 0.2 units during storage (Blake et al., 1999). Given the range and direction of change, we are confident that an increase of 0.4 pH units reflect actual change in our forest sites.
Even though there were non-significant results of C and N between the years, there was a significant decrease in forest floor C:N ratios as N concentrations generally trended toward an increase. One study in Germany in Scot pine forests found a similar decrease in C:N ratios between 1974 and 2004 in forest surface soils (0–20 cm) as N was enriched at a greater rate compared to C, coupled with base cation losses in the soils. Both of the forests in the German study showed a decrease of the forest floor C:N ratio from 35.4 to 29.2 for one forest and from 36.5 to 23.0 for the other (Prietzel et al., 2006), which were much higher than our values of 17.8 and 2001 and 16.7 and 2018. Contrary to these results, Johnson et al. (2007) found an increase in C:N ratios in Tennessee forest soils due to higher C values and slightly lower N values between 1972 and 2004. The lower N concentrations were not attributed to changes in vegetation or detritus N storage, atmospheric deposition, or leaching, but instead to high variability.
For the nutrients and properties such as S, P, C, N, and SOM that did not change over time, possible explanations include: (1) the soil has reached a steady-state, i.e., these systems are already saturated with a particular nutrient; 92) changes are occurring at slower rates (different rates for different chemical variables) than what can be detected after 17 years; (3) detectable change occur but at a smaller time period, e.g., seasonally or biannually; or finally, (4) the inherent spatial variability is too high as to mask any detectable temporal change.
Local/Urban Effects
Local factors, e.g., site history, local polluting sources, or anthropogenic environmental effects related to urban land uses, could affect sites differently along the gradient. Local effects influenced Ca, N, P, and S, meaning that one of the locations along the gradient, i.e., urban, suburban, or rural, was different from the others. Calcium concentration were higher at the urban sites; sulfur and N were significantly higher in the suburban soils compared to the urban and rural forest soils; and finally, P was lower in the urban forest soils compared to the suburban and rural forest soils.
Calcium is accumulating on forest soils in higher concentrations found in the urban environments compared to the rural. This was expected as earlier results of the same sites showed Ca was correlated with distance from city core (r2 = 0.44, p = 0.0098) (Pouyat et al., 2008). In Baltimore, to establish a gradient on one rock type is very challenging due to differences in geologic types separated by the piedmont and the coastal plain physiographic regions. The parent material is different in our urban-rural gradient with the urban sites underlain by geology higher in base cations, i.e., gabbro, compared to the rural end of the gradient, i.e., gneiss (Pouyat et al., 2009). One study showed that the weathering rinds of gabbro contained about 10 times more CaO than granite, 8.7% compared to 0.81%, respectively (Fritz, 1988), which may confound the gradient results.
Ca bulk and throughfall deposition (Lovett et al., 2000; Juknys et al., 2007) as well as road dust samples (Apeagyei et al., 2011) have been shown to be higher in urban areas compared to rural. This was supported in a separate study in Baltimore that found riparian samples were higher in Ca closer to the mouth of the watershed/city center compared to the rural end of the watershed (Bain et al., 2012). Also, this was reflected in Baltimore and Philadelphia forest sites where the urban sites had elevated calcium compared to reference forest soils (Sonti et al., 2019). However, there are other explanations for soil Ca concentrations, for example, for forest plots across northern Delaware and southeastern Pennsylvania, plant invasion and site history explained soil Ca trends better than urban metrics and parent material (Trammell et al., 2021).
For those studies that have higher Ca concentrations in the urban sites, atmospheric deposition enriched in Ca-rich dust from concrete and wallboard may be a source (Kuang et al., 2004; Nath et al., 2007). Cement which is composed of 60–65% lime (CaO) and only trace amounts of Mg and S may be a main source because there was no relationship between Ca and Mg or S (Supplementary Figure 3). Similarly, Bain et al. (2012) found that high Ca levels independent of Mg or S concentrations indicated a substantial input of relatively pure Ca to Baltimore riparian sediments associated with urban areas. However, because of this weak relationship with Mg, parent material may not be a major source of Ca.
In Adirondack forest soils, OM was correlated with Ca (Likens et al., 1998) and any change in OM status affected Ca concentrations. In our study, Ca is decoupled from OM in both the urban and suburban forests, which suggest that leaf litter and organic matter accumulation are not a strong driver of this change (Figure 4). While forest age and dominant tree species composition were controlled in the experimental design, the relative abundance of tree species varies by site and by plot. Tree species take up varying concentrations of nutrients in leaf tissue, e.g., Liriodendron tulipifera has high Ca (White et al., 1988; Ma et al., 2014) compared than Quercus spp., and the deposition of these leaves on the soil surface will have an effect on the nutrient concentration of the soil. Along the same line, vegetation change (Templeton et al., 2019), dieback and replacement may have occurred, affecting soil chemical properties locally.
The local effect on sulfur was greatest in the suburban sites. The gradient was significant because the suburban sites had higher concentrations of S relative to urban and rural sites. One possible reason is that the suburban sites had higher organic matter concentrations relative to the urban because the cycles of S are closely linked to organic matter (Scherer, 2009; Kovar and Grant, 2011) mediated by microorganisms (McGill and Cole, 1981). Organic sulfur can make up more than 90% of forest soil sulfur (Bettany et al., 1973; Schindler et al., 1986). Soils can retain and adsorb 48 to 61% of the deposited sulfate (Dail and Fitzgerald, 1999; Cai, 2010), and lower soil pH values have been shown to increase the adsorption sulfates in the soil (Chao et al., 1964). For our soils, pH was not correlated to sulfur which may indicate that, overall, the soil pH for the forest plots were not low enough to have a bearing on the adsorption of sulfate. Even though average traffic counts are slightly lower in the suburban sites compared to the urban sites [46,676 to 49,463 vehicles day–1, respectively (Pouyat et al., 2008)], the higher SOM concentrations may retain more of the S released from diesel fuel in the local area. It wasn’t until after 2010 that the EPA required all highway diesel vehicles to use Ultra Low Sulfur Diesel fuel (EPA, 2022).
Phosphorus concentrations were found to be the lowest in the urban sites. In oak forests along an urban-rural gradient in the New York City metropolitan region, rural sites had four times the amount of extractable phosphorus than urban sites, 100 mg kg–1 compared to 23 mg kg–1, respectively (suburban was not reported) (Baxter et al., 2002). The authors suggest the local effects of earthworm presence in the urban sites and not in the rural sites, and difference in litter quality explain the results. These trends of lower concentrations in urban than in rural agree with our study, although we acknowledge that different forms of P were extracted in the two studies. Another study in China investigated an urban rural gradient that contained two types of forest: (1) conifer and broadleaf mixed forest, and (2) evergreen and broadleaf forest (Chen et al., 2010b). For the conifer and broadleaf mixed forest, the total extracted phosphorus at the urban end of the gradient was 300 mg kg–1, suburban 100 mg kg–1, and rural 110 mg kg–1 with the urban being the highest. For the evergreen and broadleaf forest, the concentrations were 410, 75, and 110 mg kg–1 for urban, suburban, and rural sites, respectively. The authors attribute imported food, and various anthropogenic waste deposits to the high P concentrations at the urban end of the gradient. Contrary to that study, our gradient results show lower concentrations in the urban (220 mg kg–1) and higher for the suburban and rural (363, and 287 mg kg–1, respectively).
The top three 2018 plots (3–5 plots per site) for P content in our dataset were in three suburban sites: two plots in Lake Roland, and one plot in Druid Ridge Cemetery. The Lake Roland plots were downhill of a residential area where there appeared to be some domestic dumping, and Druid Ridge Cemetery showed evidence of dumping of various organic and inorganic materials. Nine of the top 13 plots with the highest P concentrations for 2018 reside on the Baltimore gneiss rock type which, strangely enough, does not contain high levels of P indicating that P is primarily of anthropogenic origin. Plots were selected that were not affected by the forest edge and therefore away from dumping, but it is inherently hard to avoid anthropogenic artifacts and disturbance in urban areas.
Soil organic matter concentrations were significant along the gradient with the highest value in the suburban sites. For SOM, a possible explanation for the higher suburban OM is higher plant productivity due to mild heat island effects while maintaining intermediate OM decay rates relative to urban and rural soils (Trammell et al., 2017). A study investigating the interior forest soils under American beech (Fagus grandifolia) and tulip poplar (Liriodendron tulipifera) along an urban-rural gradient in Newark, DE, found the suburban plots had higher OM percentages of approximately 8.7 ± 1 compared to the urban end of the gradient at approximately 5.0 ± 0.9 for urban (Rosier et al., 2021). These numbers are surprising close to our numbers of 8.7 ± 0.72 for the suburban and 6.0 ± 0.26 for the urban. It would be expected that SOM and C are correlated (r2 = 0.79 for both 2001 and 2018 at the plot level), so it was surprising that C was not statistically significant.
In line with our study results, urban-rural gradient studies have shown no difference in C along the gradient; for example, Pouyat and Carreiro (2003) did not find a significant difference even though there was a possible trend where urban had a higher soil C concentration compared to suburban and rural, 6.5 ± 0.9 g kg–1, 4.7 ± 0.3, and 5.1 ± 0.9, respectively. Baltimore values compared to New York values were much higher at 32 ± 1.3, 47 ± 6.4, and 32 ± 2.8 g kg–1, for urban, suburban, and rural, respectively. Our higher suburban values were also similar to another study that found higher suburban soil C values, even though high variability did not produce significant results for their study (Chen et al., 2010a).
As for total N, higher concentrations of nitrogen were found in the suburban sites (42% higher than both urban and rural) similar to Chen et al. (2010a) which found 58% higher concentrations (than both urban and rural). Our results may be related to high organic matter concentrations. However, other studies have shown that total soil N pools are related to elevated atmospheric N deposition in urban areas, for example, for a gradient in New York, the rural sites trended lower at 0.21 g kg–1 compared to the urban sites of 0.29 g kg–1 (Pouyat et al., 1995). While other results show the opposite, where the total N in rural sites (0.27 g kg–1) were higher than the urban sites (0.21 g kg–1) (Huang et al., 2012). Both of these studies have lower total N concentrations to our sites with a range of 1.9 to 2.7 g kg–1.
Conclusion
We examined patterns of soil properties in multiple dimensions: spatially, i.e., along and urban-rural gradient, and temporally, i.e., the change of these properties over two decades. Local (1 km and street) and regional (5–50 km) effects influenced the change of pH, Ca concentrations, and C:N ratios in Baltimore forest soils after only 17 years. Local effects influenced Ca, N, P, and S, meaning that one of the locations along the gradient, i.e., urban, suburban, or rural, was different from the others. Sulfur and N were significantly higher in the suburban soils and P was lower in the urban forest soils compared to the suburban and rural forest soils. Increased Ca concentrations and soil pH values are especially notable because these properties affect biogeochemical processes, plant community structure and productivity, and soil bacterial, fungal, and faunal assemblages. Some trends in relation to changes may become clearer over time. Future work that includes additional data collection, such as fine scale atmospheric deposition data, as well as detailed assessment of soil carbon forms and fluxes, will provide insight to the mechanisms of these spatio-temporal patterns. Knowing the direction and magnitude of change, these forest systems can be more efficiently managed.
Data Availability Statement
The raw data supporting the conclusions of this article will be made available by the authors, without undue reservation.
Author Contributions
IY and KS conceived of the idea and resampled the plots in 2018. VG and RP established the plots in 2001. VG originally sampled the plots and analyzed the soils in 2001. YH and IY analyzed the data and the soils in 2018 and re-digested the 2001 soils. IY, KS, and RP wrote the manuscript. All authors contributed to the article and approved the submitted version.
Funding
This study was partially funded by NSF-LTER Program to the Baltimore Ecosystem Study (DEB-1637661 and DEB-1855277), JHU-EPS Summer Field Fund, and USDA Forest Service Northern Research Station.
Author Disclaimer
The findings and conclusion in this publication are those of the authors and should not be construed to represent any official USDA or United States Government determination or policy.
Conflict of Interest
The authors declare that the research was conducted in the absence of any commercial or financial relationships that could be construed as a potential conflict of interest.
Publisher’s Note
All claims expressed in this article are solely those of the authors and do not necessarily represent those of their affiliated organizations, or those of the publisher, the editors and the reviewers. Any product that may be evaluated in this article, or claim that may be made by its manufacturer, is not guaranteed or endorsed by the publisher.
Acknowledgments
Erin Baggs, Elliott Strahan, and Keelin Reilly helped in the field and laboratory. Thanks to John Stanovick (USDA Forest Service) for statistical review and Joel Moore (Towson University) for manuscript review.
Supplementary Material
The Supplementary Material for this article can be found online at: https://www.frontiersin.org/articles/10.3389/fevo.2022.786809/full#supplementary-material
References
Aber, J. D., Melillo, J. M., Nadelhoffer, K. J., Pastor, J., and Boone, R. (1991). Factors controlling nitrogen cycling and nitrogen saturation in northern temperate forest ecosystems. Ecol. Appl. 1, 303–315. doi: 10.2307/1941759
Aber, J. D., Nadelhoffer, K. J., Steudler, P., and Melillo, J. M. (1989). Nitrogen Saturation in northern forest ecosystems. Bioscience 39, 378–386. doi: 10.2307/1311067
Adams, M. B., Burger, J. A., Jenkins, A. B., and Zelazny, L. (2000). Impact of harvesting and atmospheric pollution on nutrient depletion of eastern US hardwood forests. For. Ecol. Manag. 138, 301–319. doi: 10.1016/S0378-1127(00)00421-7
Agren, G. I., and Bosatta, E. (1988). Nitrogen saturation of terrestrial ecosystems. Environ. Pollut. 54, 185–197.
Anderson, J. R., Hardy, E. E., Roach, J. T., and Witmer, R. E. (1976). “A land use and land cover classification system for use with remote sensor data,” in Geological Survey Professional Paper 964, (Washington, DC: USGS).
Apeagyei, E., Bank, M. S., and Spengler, J. D. (2011). Distribution of heavy metals in road dust along an urban-rural gradient in Massachusetts. Atmos. Environ. 45, 2310–2323. doi: 10.1016/j.atmosenv.2010.11.015
Bailey, S. W., Horsley, S. B., and Long, R. P. (2005). Thirty years of change in forest soils of the allegheny plateau. Pennsylvania. Soil Sci. Soc. Am. J. 69, 681–690. doi: 10.2136/sssaj2004.0057
Bailey, S. W., Horsley, S. B., Long, R. P., and Hallett, R. A. (2004). Influence of edaphic factors on sugar maple nutrition and health on the allegheny plateau. Soil Sci. Soc. Am. J. 68, 243–252.
Bain, D. J., Yesilonis, I. D., and Pouyat, R. V. (2012). Metal concentrations in urban riparian sediments along an urbanization gradient. Biogeochemistry 107, 67–79. doi: 10.1007/s10533-010-9532-4
Bartlett, R., and James, B. (1980). Studying dried, stored soil samples-some pitfalls. Soil. Sci. Soc. Am. J. 44, 721–724. doi: 10.2136/sssaj1980.03615995004400040011x
Baxter, J. W., Pickett, S. T. A., Dighton, J., and Carreiro, M. M. (2002). Nitrogen and phosphorus availability in oak forest stands exposed to contrasting anthropogenic impacts. Soil Biol, Biochem. 34, 623–633. doi: 10.1016/S0038-0717(01)00224-3
Bedison, J. E., and Johnson, A. H. (2010). Seventy-four years of calcium loss from forest soils of the adirondack Mountains, New York. Soil Sci. Soc. Am. J. 74, 2187–2195. doi: 10.2136/sssaj2009.0367
Bettany, J. R., Stewart, J. W. B., and Halstead, E. H. (1973). Sulfur fractions and carbon, nitrogen and sulfur relationships in grasslands, forest and associated transitional soils. Soil Sci. Soc. Am. J. 37, 915–918. doi: 10.2136/sssaj1973.03615995003700060034x
Bidwell, D. W., and Hole, F. D. (1965). Man as a factor of soil formation. Soil Sci. 99, 65–72. doi: 10.1097/00010694-196501000-00011
Blake, L., Goulding, K. W. T., Mott, C. J. B., and Johnston, A. E. (1999). Changes in soil chemistry accompanying acidication over more than 100 years under woodland and grass at rothamsted experimental station, UK. Eur. J. Soil Sci. 50, 401–412. doi: 10.1046/j.1365-2389.1999.00253.x
Cai, M. (2010). Long-Term Acid Deposition Effects on Soil and Water Chemistry in the Noland Divide Watershed, Great Smoky Mountains National Park, USA. PhD Thesis. Knoxville, TN: University of Tennessee.
Chao, T. T., Harward, M. E., and Fang, S. C. (1964). Iron or aluminum coatings in relation to sulfate adsorption characteristics of soils. Soil Sci. Soc. Am. J. 28, 632–635. doi: 10.2136/sssaj1964.03615995002800050017x
Chen, F. S., Li, X., Greg, N., and Zhan, S.-X. (2010b). Topsoil phosphorus signature in five forest types along an urban-suburban-rural gradient in Nanchang, southern China. J. For. Res. 21, 39–44. doi: 10.1007/s11676-010-0006-2
Chen, F. S., Fahey, T. J., Yu, M. Y., and Gan, L. (2010a). Key nitrogen cycling processes in pine plantations along a short urban-rural gradient in Nanchang, China. For. Ecol. Manag. 259, 477–486. doi: 10.1016/j.foreco.2009.11.003
Chen, F. S., Yavitt, J., and Hu, X.-F. (2014). Phosphorus enrichment helps increase soil carbon mineralization in vegetation along an urban-to-rural gradient, Nanchang, China. Appl. Soil Ecol. 75, 181–188. doi: 10.1016/j.apsoil.2013.11.011
Dail, D. B., and Fitzgerald, J. W. (1999). S Cycling in soil and stream sediment: influence of season and in situ concentrations of carbon, nitrogen and sulfur. Soil Biol. Biochem. 31, 1395–1404. doi: 10.1016/S0038-0717(99)00057-7
Edwards, J. (1981). A Brief Description of the Geology of Maryland. Baltimore MD: Maryland Geological Survey.
Eisenreich, S. J., Emmling, P. J., and Beeton, A. M. (1977). Atmospheric loading of phosphorus and other chemicals to lake michigan. J. Great Lakes Res. 3, 291–304. doi: 10.1016/S0380-1330(77)72261-0
EPA (2022). Diesel Fuel Standards and Rulemakings. Available online at: https://www.epa.gov/diesel-fuel-standards/diesel-fuel-standards-and-rulemakings (accessed January 05, 2022).
Foti, L., Barot, S., Gignoux, J., Grimaldi, M., Lata, J. C., Lerch, T. Z., et al. (2020). Topsoil characteristics of forests and lawns along an urban-rural gradient in the Paris region (France). Soil Use Manag. 37, 749–761. doi: 10.1111/sum.12640
Fritz, S. J. (1988). A comparative study of gabbro and granite weathering. Chem. Geol. 68, 275–290. doi: 10.1016/0009-2541(88)90026-5
Groffman, P. M., Pouyat, R. V., McDonnell, M. J., Pickett, S. T. A., and Zipperer, W. C. (1995). “Carbon pools and trace gas fluxes in urban forest soils,” in Advances in Soil Science: Soil Management and the Greenhouse Effect, eds R. Lal, J. Kimble, E. Levine, and B. A. Steward (Boca Raton, FL: CRC Press, Inc).
Horsley, S. B., Long, R. P., Bailey, S. W., Hallett, R. A., and Wargo, P. M. (2002). Health of eastern North American sugar maple forests and factors affecting decline. N, J. Appl. For. 19, 34–44. doi: 10.1093/njaf/19.1.34
Huang, L., Zhu, W., Ren, H., Chen, H., and Wang, J. (2012). Impact of atmospheric nitrogen deposition on soil properties and herb-layer diversity in remnant forests along an urban-rural gradient in Guangzhou, southern China. Plant Ecol. 213, 1187–1202. doi: 10.1007/s11258-012-0080-y
Huntington, T. G., Hooper, R. P., Johnson, C. E., Aulenbach, B. T., Cappellato, R., and Blum, A. E. (2000). Calcium depletion in a southeastern United States forest ecosystem. Soil Sci. Soc. Am. J. 64, 1845–1858. doi: 10.2136/sssaj2000.6451845x
Jansone, L., Wilpert, K. V., and Hartmann, P. (2020). Natural recovery and liming effects in acidified forest soils in SW-Germany. Soil Syst. 4:38. doi: 10.3390/soilsystems4030038
Jenkins, J., Roy, K., Driscoll, C., and Buerkett, C. (2005). Acid Rain and the Adirondacks: A Research Summary. New York, NY: Ray Brook.
Johnson, D. W., Todd, D. E., Trettin, C. F., and Sedinger, J. S. (2007). Soil carbon and nitrogen changes in forests of walker branch watershed, 1972 to 2004. Soil Sci. Soc. Am. J. 71, 1639–1646. doi: 10.2136/sssaj2006.0365
Juknys, R., Zaltauskaite, J., and Stakenas, V. (2007). Ion fluxes with bulk and throughfall deposition along an Urban-suburban-rural gradient. Water Air Soil Pollut. 178, 363–372.
Knoepp, J. D., Markewitz, D., Mac, A., Callaham, J., Adams, M. B., Laseter, S. H., et al. (2019). “Long-term forest soils research: lessons learned from the US experience,” in Global Change and Forest Soils: Cultivating Stewardship of a Finite Natural Resource, ed. W. R. Horwath (Amsterdam: Elsevier), 473–504. doi: 10.1016/b978-0-444-63998-1.00019-7
Knoepp, J. D., and Swank, W. T. (1994). Long-term soil chemistry changes in aggrading forest ecosystems. Soil Sc. Soc. Am. J. 58, 325–331. doi: 10.2136/sssaj1994.03615995005800020010x
Knoepp, J. D., and Swank, W. T. (1997). Forest management effects on surface soil carbon and nitrogen. Soil Sci. Soc. Am. J. 61, 928–935. doi: 10.2136/sssaj1997.03615995006100030031x
Kolb, T. E., and Mccormick, L. H. (1993). Etiology of sugar maple decline in four pennsylvania stands. Can. J. For. Res. Rev. Can. Rec. For. 23, 2395–2402. doi: 10.1139/x93-296
Kovar, J. L., and Grant, C. A. (2011). Nutrient Cycling in Soils: Sulfur. Soil Management: Building a Stable Base for Agriculture. Hoboken, NJ: John Wiley & Sons, 103–115. doi: 10.2136/2011.soilmanagement.c7
Kuang, C., Neumann, T., Norra, S., and Stuben, D. (2004). Land use related chemical composition of street sediments in Beijing. Environ. Sci. Pollut. Res. 11, 73–83. doi: 10.1007/BF02979706
Lawrence, G. B., Fernandez, I. J., Richter, D. D., Ross, D. S., Hazlett, P. W., Bailey, S. W., et al. (2013). Measuring environmental change in forest ecosystems by repeated soil sampling: a North American perspective. J, Environ, Qual. 42, 623–639. doi: 10.2134/jeq2012.0378
Lawrence, G. B., Hazlett, P. W., Fernandez, I. J., Ouimet, R., Bailey, S. W., Shortle, W. C., et al. (2015). Declining acidic deposition begins reversal of forest-soil acidification in the Northeastern US and Eastern Canada. Environ. Sci. Technol. 49, 13103–13111. doi: 10.1021/acs.est.5b02904
Lawrence, G. B., Fernandez, I. J., Hazlett, P. W., Bailey, S. W., Ross, D. S., Villars, T. R., et al. (2016). Methods of soil resampling to monitor changes in the chemical concentrations of Forest Soils. J. Vis. Exp. 117:54815. doi: 10.3791/54815
Levin, M. J., and Griffin, T. M. (1998). Soil Survey of the City of Baltimore, Maryland. Annapolis, MD: United States Department of Agriculture.
Likens, G. E., Driscoll, C. T., Buso, D. C., Siccama, T. G., Johnson, C. E., Lovett, G. M., et al. (1998). The biogeochemistry of calcium at Hubbard Brook. Biogeochemistry 41, 89–173.
Lovett, G. M., Traynor, M. M., Pouyat, R. V., Carreiro, M. M., Zhu, W. X., and Baxter, J. W. (2000). Atmospheric deposition to oak forests along an urban-rural gradient. Environ. Sci. Technol. 34, 4294–4300. doi: 10.1021/es001077q
Ma, Y. N., Filley, T. R., Szlavecz, K., and McCormick, M. K. (2014). Controls on wood and leaf litter incorporation into soil fractions in forests at different successional stages. Soil Biol. Biochem. 69, 212–222. doi: 10.1016/j.soilbio.2013.10.043
McDonnell, M. J., and Pickett, S. T. A. (1990). Ecosystem structure and function along urban-rural gradients: an unexploited opportunity for ecology. Ecology 71, 1232–1237. doi: 10.2307/1938259
McGill, W. B., and Cole, C. V. (1981). Comparative aspects of cycling of organic C. N, S and P through soil organic matter. Geoderma 26, 267–286. doi: 10.1016/0016-7061(81)90024-0
Metson, G. S., Iwaniec, D. M., Baker, L. A., Bennett, E. M., Childers, D. L., Cordell, D., et al. (2015). Urban phosphorus sustainability: systemically incorporating social, ecological, and technological factors into phosphorus flow analysis. Environ. Sci. Policy 47, 1–11. doi: 10.1016/j.envsci.2014.10.005
Mobley, M. L., Lajtha, K., Kramer, M. G., Bacon, A. R., Heine, P. R., and Richter, D. D. (2015). Surficial gains and subsoil losses of soil carbon and nitrogen during secondary forest development. Glob. Change Biol. 21, 986–996. doi: 10.1111/gcb.12715
Nath, B., Norra, S., Chatterjee, D., and Stuben, D. (2007). Fingerprinting of land use-related chemical patterns in street sediments from Kolkata. India. Environ. For. 8, 313–328.
Newman, E. I. (1995). Phosphorus inputs to terrestrial ecosystems. J. Ecol. 83, 713–726. doi: 10.2307/2261638
NOAA (2000). Comparitive Climatic Data for the United States Through 2000. Washington, DC: National Oceanic and Atmospheric Administration.
Pouyat, R. V., and Carreiro, M. M. (2003). Controls on mass loss and nitrogen dynamics of oak leaf litter along an urban-rural land-use gradient. Oecologia 135, 288–298. doi: 10.1007/s00442-003-1190-y
Pouyat, R. V., Carreiro, M. M., Groffman, P. M., and Zuckerman, M. (2009). “Investigative approaches to urban biogeochemical cycles: New York metropolitan area and Baltimore as case studies,” in Ecology of Cities and Towns: A Comparative Approach, eds M. Mcdonnell, J. Breuste, and A. Hahs (New York, NY: Springer-Verlag).
Pouyat, R. V., McDonnell, M. J., Pickett, S. T. A., Groffman, P. M., Carreiro, M. M., Parmelee, R. W., et al. (1995). “Carbon and nitrogen dynamics in oak stands along an urban-rural gradient,” in Carbon Forms and Functions in Forest Soils, eds J. M. Kelly and W. W. McFee (Madison, WI: Soil Science Society of America), 569–587. doi: 10.2136/1995.carbonforms.c26
Pouyat, R. V., Szlavecz, K., and Yesilonis, I. D. (2019). “Human influences of urban soil development,” in Science for the Sustainable City: Empirical Insights from the Baltimore School of Urban Ecology, eds S. T. A. Pickett, M. L. Cadenasso, and J. M. Grove (New Haven, CT: Yale University Press).
Pouyat, R. V., Yesilonis, I. D., Szlavecz, K., Csuzdi, C., Hornung, E., Korsos, Z., et al. (2008). Response of forest soil properties to urbanization gradients in three metropolitan areas. Landsc. Ecol. 23, 1187–1203.
Prietzel, J., Stetter, U., Klemmt, H. J., and Rehfuess, K. E. (2006). Recent carbon and nitrogen accumulation and acidification in soils of two Scots pine ecosystems in Southern Germany. Plant Soil 289, 153–170. doi: 10.1007/s11104-006-9120-5
Prodromou, K. P., and Pavlatou-Ve, A. S. (1998). Changes in soil pH due to the storage of soils. Soil Use Manag. 14, 182–183. doi: 10.1111/j.1475-2743.1998.tb00146.x
Reybold, W. U., and Matthews, E. D. (1976). Soil survey of Baltimore County, Maryland. Washington, DC: United States Department of Agriculture.
Richardson, J. B., and Friedland, A. J. (2016). Influence of coniferous and deciduous vegetation on major and trace metals in forests of northern New England. USA. Plant Soil 402, 363–378. doi: 10.1007/s11104-016-2805-5
Richter, D. D., and Yaalon, D. H. (2012). “The changing model of soil” revisited. Soil Sci. Soc. Am. J. 76, 766–778. doi: 10.2136/sssaj2011.0407
Rosier, C. L., Polson, S. W., D’Amico, V., Kan, J., and Trammell, T. L. E. (2021). Urbanization pressures alter tree rhizosphere microbiomes. Sci. Rep. 11:9447. doi: 10.1038/s41598-021-88839-8
Rustad, E. R., Knoepp, J., Richter, D. D., and Scott, A. (2020). “Biogeochemical Cycling in Forest and Rangeland Soils of the United States,” in Forest and Rangeland Soils of the United States Under Changing Conditions: A Comprehensive Science Synthesis, eds R. V. Pouyat, D. Dumroese, T. Patel-Weynand, and L. Geiser (Cham: Springer), 51–74.
Schaberg, P. G., and DeHayes, D. H. (2000). “Physiological and environmental causes of freezing injury in red spruce,” in Responses of Northern U.S. Forest to Environmental Change. Ecological Studies 139, eds R. A. Mickler, R. A. Birdsey, and J. Hom (New York, NY: Springer Verlag), 181–227.
Scherer, H. W. (2009). Sulfur in soils. J. Plant Nutr. Soil Sci. 172, 326–335. doi: 10.1002/jpln.200900037
Schindler, S. C., Mitchell, M. J., Scott, T. J., Fuller, R. D., and Driscoll, C. T. (1986). Incorporation of 35S-sulfate into inorganic and organic constituents of two forest soils. Soil Sci. Soc. Am. J. 50, 457–462. doi: 10.2136/sssaj1986.03615995005000020041x
Sonti, N. F., Hallett, R. A., Griffin, K. L., and Sullivan, J. H. (2019). White oak and red maple tree ring analysis reveals enhanced productivity in urban forest patches. For. Ecol. Manag. 453:117626. doi: 10.1016/j.foreco.2019.117626
St Clair, S. B., Sharpe, W. E., and Lynch, J. P. (2008). Key interactions between nutrient limitation and climatic factors in temperate forests: a synthesis of the sugar maple literature. Can. J. For. Res. 38, 401–414. doi: 10.1139/X07-161
Szlavecz, K., Placella, S. A., Pouyat, R. V., Groffman, P. M., Csuzdi, C., and Yesilonis, I. (2006). Invasive earthworm species and nitrogen cycling in remnant forest patches. Appl. Soil Ecol. 32, 54–62. doi: 10.1016/j.apsoil.2005.01.006
Templeton, L. K., Neel, M. C., Groffman, P. M., Cadenasso, M. L., and Sullivan, J. H. (2019). Changes in vegetation structure and composition of urban and rural forest patches in Baltimore from 1998 to 2015. For, Ecol. Manag. 454:117665. doi: 10.1016/j.foreco.2019.117665
Thunis, P., Miranda, A., Baldasano, J. M., Blond, N., Douros, J., Graff, A., et al. (2016). Overview of current regional and local scale air quality modelling practices: assessment and planning tools in the EU. Environ. Sci. Policy 65, 13–21. doi: 10.1016/j.envsci.2016.03.013
Trammell, T. L. E., Day, S., Pouyat, R. V., Rosier, C., Scharenbroch, B., and Yesilonis, I. (2017). “Drivers of Urban soil carbon dynamics,” in Advances in Soil Science: Urban Soils, eds R. Lal and B. A. Stewart (Boca Raton, FL: CRC Press), 406.
Trammell, T. L. E., Pouyat, R. V., and D’Amico, V. (2021). Soil chemical properties in forest patches across multiple spatiotemporal scales in mid-Atlantic U.S. metropolitan areas. Urban Ecosyst. 24, 1085–1100. doi: 10.1007/s11252-021-01096-5
Wang, X. S. (2010). Black carbon in urban topsoils of Xuzhou (China): environmental implication and magnetic proxy. Environ. Monit. Assess. 163, 41–47. doi: 10.1007/s10661-009-0814-z
Weil, R. R., and Brady, N. C. (2017). The Nature and Properties of Soils, 15th Edn. Columbus, OH: Pearson Education, Inc.
White, D., Haines, B., and Boring, L. (1988). Litter decomposition in southern Appalachian black locust and pine hardwood stands— litter quality and nitrogen dynamics. Can. J. For. Res. 18, 54–63. doi: 10.1139/x88-009
Yesilonis, I. D., Pouyat, R. V., Russell-Anelli, J., and Powell, E. (2016). The effects of landscape cover on surface soils in a low density residential neighborhood in Baltimore. Maryland. Urban Ecosyst. 19, 115–129. doi: 10.1007/s11252-015-0502-4
Yuan, D. G., Zhang, G. L., Gong, Z. T., and Burghardt, W. (2007). Variations of soil phosphorus accumulation in Nanjing, China as affected by urban development. J. Plant Nutr. Soil Sci. 170, 244–249. doi: 10.1002/jpln.200622035
Zhang, M. K. (2004). Phosphorus accumulation in soils along an urban-rural land use gradient in Hangzhou, Southeast China. Commun. Soil Sci. Plant Anal. 35, 819–833. doi: 10.1081/css-120030360
Keywords: retrospective, local, regional, anthropogenic, deposition, nutrient, urban-rural gradient, Baltimore
Citation: Yesilonis I, Giorgio V, Hu Y, Pouyat R and Szlavecz K (2022) Changes in Soil Chemistry After 17 Years in Urban and Rural Forest Patches. Front. Ecol. Evol. 10:786809. doi: 10.3389/fevo.2022.786809
Received: 30 September 2021; Accepted: 14 January 2022;
Published: 21 February 2022.
Edited by:
Bryant Scharenbroch, University of Wisconsin–Stevens Point, United StatesReviewed by:
Olawale O. Oladeji, Metropolitan Water Reclamation District of Greater Chicago (MWRDGC), United StatesAnna Paltseva, University of Louisiana at Lafayette, United States
Copyright © 2022 Yesilonis, Giorgio, Hu, Pouyat and Szlavecz. This is an open-access article distributed under the terms of the Creative Commons Attribution License (CC BY). The use, distribution or reproduction in other forums is permitted, provided the original author(s) and the copyright owner(s) are credited and that the original publication in this journal is cited, in accordance with accepted academic practice. No use, distribution or reproduction is permitted which does not comply with these terms.
*Correspondence: Ian Yesilonis, ian.yesilonis@usda.gov