- Department of Geography, Records of Environment and Disturbance (RED) Lab, University of Utah, Salt Lake City, UT, United States
Millennial-scale environmental histories from North American desert southwest (SW) ciénegas were examined with existing time series for the North American Monsoon (NAM) and El Niño, in concert with published long-term records of desert vegetation and climate. The goal was to better understand the relationships among fire, the seasonality of precipitation, effective moisture levels, and vegetation type. It was determined that without sufficient winter precipitation fires are rare in desert SW ecosystems. However, it was also determined that in addition to winter moisture, summer ignitions are critical for fire in southwestern deserts. A relationship between the abundance of woody fuels and charcoal abundance was identified, although further calibration on charcoal production in woody vs. grassy desert settings in necessary to fully understand this interplay. Finally, the impacts of climate change and invasive species were considered, with both likely increasing the frequency of fire in desert ecosystems.
Introduction
In the southwestern United States, the role of fire in the ecology and maintenance of many upland ecosystems is well understood due to the availability of dendroecological records. Much less is known about the frequency, role and drivers of fire in low elevation desert environments as a result of the paucity and unsuitability of trees for dendrochronological study. There is no consensus on the role of fire in desert settings; some argue that fires are uncommon in desert ecosystems (Brown and Minnich, 1986; Mack et al., 1996; Felger, 2007), while others suggest that prior to the arrival of Euro-Americans fires were frequent and played a significant role in the maintenance of desert systems (McPherson, 1995; Davis et al., 2002; Turner et al., 2003). This disagreement largely stems from limitations of historical observations and prehistoric fire history data from these ecosystems. The Fire Effects Information System (FEIS) produced by the United States Forest Service (USFS) now provides fire regime estimates using Landfire succession modeling.1 The input for these models is based on Biophysical Settings (BpS) “which represent the vegetation that may have been dominant on the landscape prior to Euro-American settlement and are based on both the current biophysical environment and an approximation of the historical disturbance regime” (Fire Effects Information System, 2021). While the FEIS products give ranges of pre-European fire return intervals for many specific arid-land settings (e.g., Southwest desert grasslands 10–883 years; Sonoran desert shrublands 103–1428 years), the fundamental inputs to the model are not truly known and there is large uncertainty associated with the ranges. If the pre-European fire regimes are not known, we cannot fully understand the current and future drivers. Given that the fire is currently being used as a management tool in desert ecosystems, truly knowing the interplay among the key climatic and ecological variables is critical (e.g., Schussman and Gori, 2004).
With the introduction of invasive plants (e.g., Bromus tectorum, cheatgrass) and their modification of fuel structure, the fire regime in desert ecosystems is increasing from historic return intervals (Archer and Predick, 2008). Non-native species are expected to thrive in our current and future CO2 enriched atmosphere, leaving the native plants at a disadvantage. Having a pre-European baseline for fire history and vegetation composition and density would help assess the impact of these invasive species on the vegetation composition, fuel loads, and fire occurrence.
Bestelmeyer et al. (2018) posit that the transition from grassland to shrubland (hereinafter “woody plant encroachment,” WPE) is a significant terrestrial regime shift that is not well understood and will likely accelerate with climate change. They argue that these systems are restorable, but potentially to novel compositions. In both the case of invasive plants changing fuel structure and WPE, having knowledge of the pre-European ecosystem would be valuable for assessments of composition changes and for management and restoration efforts.
Sedimentary records are a well-accepted source of prehistoric ecological data including vegetation change and fire regimes. In the desert, ciénega (wetland) sediments can provide information about pre-European vegetation and fire history. For example, Davis et al. (2002) used ∼2000 year old microscopic charcoal record to show that regional fire decreased in the Sonoran Desert ca. 200 years ago with the displacement of native agriculture by Euro-American settlement. In arid settings, sedimentary records from ciénegas may be the only way to get direct evidence of past fire and vegetation regimes.
The objectives of this manuscript include:
• To review and synthesize the available long-term records of desert fire ecology and climate from the North American desert southwest (SW) to assess the climate drivers of fire in this ecosystem.
• To examine new data regarding the history of controls on woody plant encroachment (WPE) as it relates to fuel changes in desert environments.
• To examine a North American Monsoon (NAM) time series in concert with El Niño data, as well as charcoal and pollen time series from southwestern desert sites (Brunelle et al., 2018) to assess the role of the seasonal distribution of moisture on desert fire histories.
Site Descriptions and Methods
Climate
The desert SW features scattered spring-fed wetlands known throughout the region as ciénegas (Hendrickson and Minckley, 1985; Minckley et al., 2013; Cole and Cole, 2015). Ciénega form and function is largely driven by groundwater levels, which are in turn driven by recharge from winter precipitation (Flint et al., 2004; Scanlon et al., 2006).
The modern precipitation regime of southwest North America is bimodal (Mock, 1996; Shinker, 2010). Winter precipitation is delivered by midlatitude frontal systems (Mock, 1996; Comrie and Glenn, 1998; Shinker, 2010). Summer precipitation is spatially variable, and arrives as convective thunderstorms from the NAM (Mock, 1996; Adams and Comrie, 1997; Sheppard et al., 2002; Diem and Brown, 2006; Shinker, 2010). The winter component of this bimodal regime includes precipitation anomalies resulting from the status of the El Niño/Southern Oscillation (ENSO) (Ropelewski and Halpert, 1986; Cole and Cook, 1998; Gershunov and Barnett, 1998; Cayan et al., 1999; Dettinger et al., 2000). El Niño is the anomalous warming of the near-surface water off the western coast of South America that initiated about 5000 years ago (Enfield, 1992; Liu et al., 2014). Its atmospheric counterpart is the Southern Oscillation, which is measured by changes in barometric pressure (Wallace and Gutzler, 1981; Enfield, 1992). El Niño years in the instrumental record typically result in higher-than-average winter precipitation in the SW; while strong La Niña years cause lower-than-average winter precipitation and are associated with regional drought (Shinker and Bartlein, 2009).
The NAM is a classic monsoon with a seasonal reversal of wind direction that brings moisture into the SW and Mexico in the summer phase. Higgins et al. (1999) propose that Arizona receives 35% of its annual rainfall from the NAM while New Mexico receives 45%. Barron et al. (2012) summarize a Holocene history of the NAM from several proxy records and suggest that before 8000 cal year BP the extent of the NAM was larger but the strength weaker and at about 6000 cal year BP the spatial extent was more focused and stronger. Stahle et al. (2009) and Jones et al. (2015) present NAM time series that are used here to compare with the existing ciénega time series records (Brunelle et al., 2018).
Site Descriptions
San Bernardino Ciénega (31.3°N; 109.3°W, 1160 m asl; Figure 1) (SBNWR) is located near Douglas, Arizona and crosses the United States/Mexico border into northeastern Sonora, Mexico. This site is located in the Sonoran Desert, but near the ecotone of the Chihuahuan Desert grassland (Minckley and Brunelle, 2007; Minckley et al., 2009; Brunelle et al., 2010). Currently the ciénega surface is dry except for a few artificial impoundments and small perennial springs managed by the San Bernardino National Wildlife Refuge in the United States, and private landowners on the Mexican side of the border. The ciénega sediments were sampled stratigraphically from an arroyo (ephemeral stream) cut into a desiccated ciénega deposit profile and an active ciénega surface.
Cloverdale Ciénega is located in the Peloncillo Mountains, Coronado National Forest, New Mexico (31°26.141′N, 108°58.517 W, 1640 m asl; Figure 1) (Cloverdale). Cloverdale is in the Chihuahuan desert grassland ecosystem and is situated at the woodland-grassland ecotone (Brunelle et al., 2014). Being near the boundary of woodland and grassland makes this location sensitive to changes in community composition over time. Standing water on Cloverdale is ephemeral, however, the sediments currently stay wetted year-round. The site was cored using a vibracorer on the existing active ciénega surface.
Canelo Hills Ciénega (31°33.833′N, 110°31.711′W, 1,506 m asl; Figure 1) (Canelo) is a Nature Conservancy preserve located 17 km southeast of Sonoita, Arizona on the east side of the Canelo Hills. The area is in the footprint of the Sonoran Desert ecosystem. This ciénega is located along a stream channel and the wetland sediments were collected using a vibracorer from the surface of the active ciénega.
Babocamari Ciénega (31°37.901′N, 110°27.178′W, 1,391 m asl; Figure 1) (Babocamari) is 20 km southeast of Sonoita, Arizona and located between the Whetstone and Huachuca Mountains. The site is also located in the Sonoran Desert ecosystem. Babocamari is part of an area with active ciénega restoration by local landowners. The site was sampled with the vibracorer near the stream channel in an undisturbed area.
Site information for Laguna Pallcacocha (Ecuador, Moy et al., 2002), El Malpais National Monument, New Mexico (Stahle et al., 2009) and Laguna de Janacatlan (Mexico, Jones et al., 2015) can be found in the respective publications.
Methods
As described above, all ciénegas except SBNWR were cored using the Vibracore system (e.g., Lanesky et al., 1979). As all four sites are previously published, details of the coring, sampling and lab methods can be found in Brunelle et al. (2010, 2014, 2018). The existing literature presents pollen percentage and influx data, charcoal/fire histories, WPE analyses, and pollen preservation indices. Pollen preservation in arid settings is often described as problematic (Hall, 1985). However, previous work (e.g., Brunelle et al., 2018) demonstrates that the changes in pollen preservation over the course of a record actually provide information about moisture conditions on the ciénega as pollen will not preserve well in dry sediments due to exposure to oxygen and subsequent deterioration.
For this manuscript several new data sets were considered including. Additional pollen samples to increase the resolution of the recent portion of the Cloverdale record. These new samples allowed the refinement of the WPE interpretations originally presented in Brunelle et al. (2014). Examination of the influx of encroacher taxa (Brunelle et al., 2014) was also conducted on the three additional ciénega sites to determine if the record from Cloverdale was unique or if the pattern of WPE was consistent across the region. These new data were compared with all existing data to assess the fire-vegetation dynamics on millennial time scales. Second, two datasets examining the NAM were compared with the existing ciénega data including Stahle et al. (2009) and Jones et al. (2015). For the Stahle et al. (2009) July precipitation events >3 SD from the average were plotted to indicate strong NAM events, while with the Jones et al. (2015) the 100 year periodicity was plotted (Figures 2, 3).
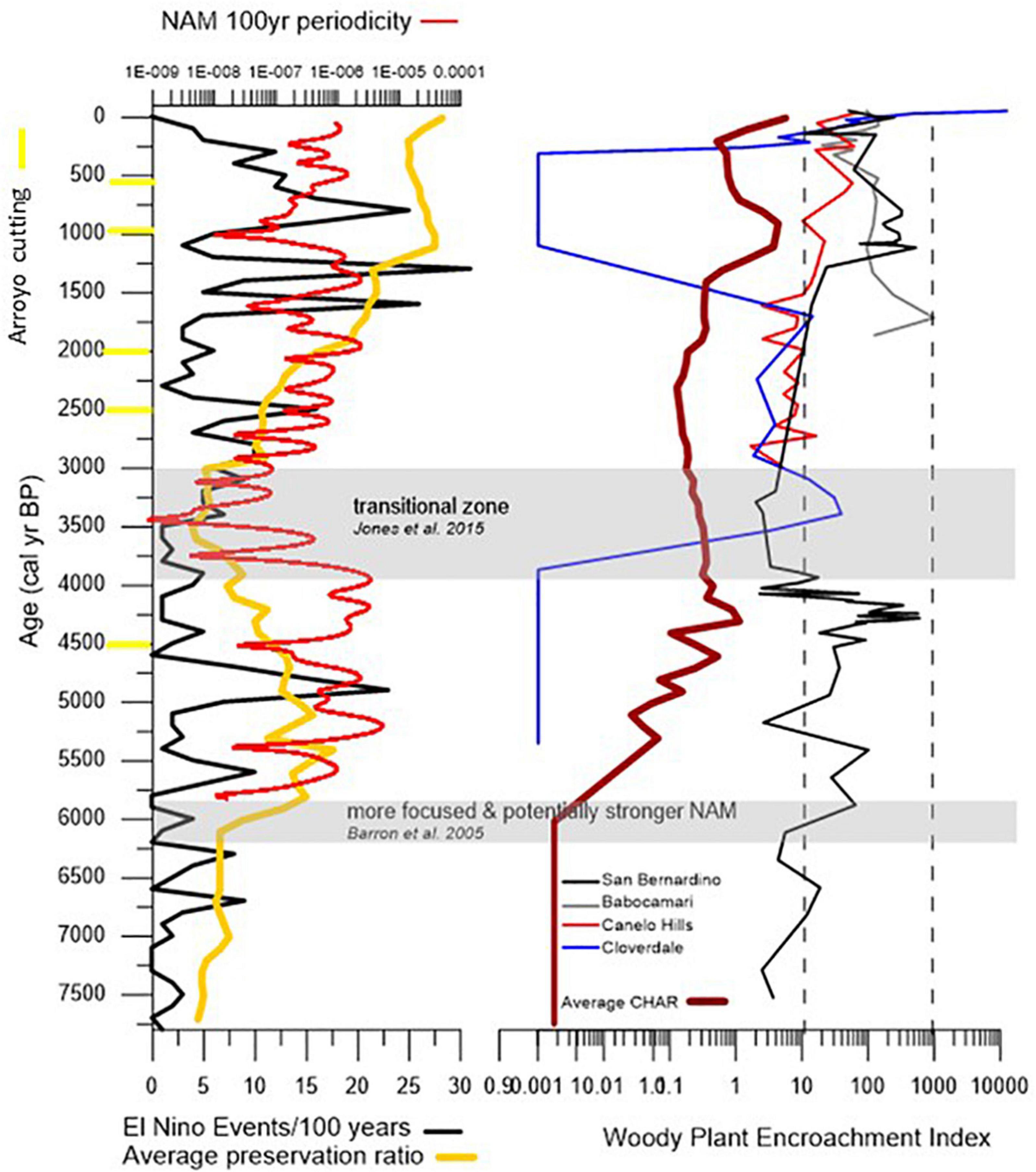
Figure 2. Left panel: Long-term records of El Niño/Southern Oscillation (ENSO) events (black line, Moy et al., 2002), North American Monsoon (NAM) frequency (red line, Jones et al., 2015), average pollen preservation (gold line). Arroyo cutting events are indicated on the y-axis as yellow bars. Right panel: average charcoal accumulation rates (CHAR) (dark red line, Brunelle et al., 2018), and woody plant encroachment (WPE) (see color assignments on figure) indices for the four ciénega sites described in the text.
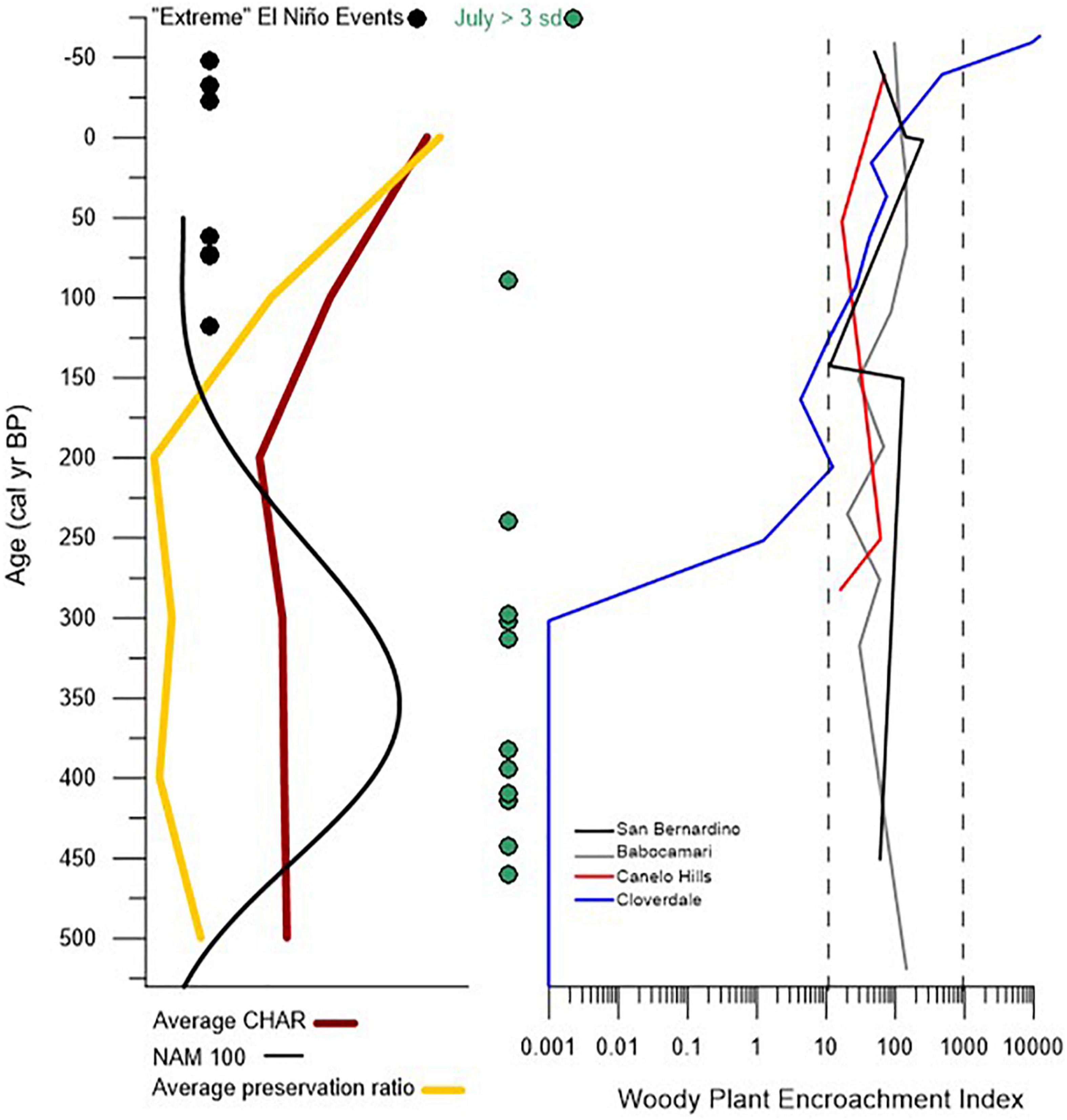
Figure 3. The last ∼500 years of the record. Left panel: “extreme” El Niño events (black dots) as reported by Brönnimann et al. (2007), and extreme NAM events (green dots, Stahle et al., 2009). Also included are average pollen preservation (gold line) and average CHAR (dark red line, Brunelle et al., 2018). Right panel: woody plant encroachment indices (see color assignments on figure) for the four ciénega sites described in the text.
A Pearson’s correlation analysis was conducted among the ciénegas, ENSO (Moy et al., 2002) and NAM (Jones et al., 2015) time series to extract relationships among the climate forcings and ecological responses (Table 1).
Results and Discussion
Long-Term Records
In order to understand fire regimes in desert ecosystems, it is critical to have a baseline understanding of how all the components of this ecosystem have responded to past climate variations. In this manuscript, the “Results and Discussion” sections are merged since much of the ciénega results have been published previously. Fire frequency is inferred from the sedimentary charcoal records of the ciénegas. The records are divided into five periods based on that overall reconstructed fire frequency. The broad-scale assessment of overall climate conditions for the study area are based on the average pollen preservation ratio calculated from the ciénegas (Figure 2). These climate interpretations were then compared with a time series for ENSO (Moy et al., 2002), NAM (Stahle et al., 2009; Jones et al., 2015) and the reconstructed fire history (CHAR). There are some “moderate” (Ratner, 2009) correlations noted (yellow shading, Table 1) among the variables. The strongest is 0.67 between preservation and the 530 year periodicity of the NAM and all p-values for the highlighted moderate correlations were <0.05. Both ENSO and fire also correlate well with preservation. The NAM and ENSO likely correlate well with preservation because conditions of high effective moisture are favorable for pollen preservation (needs saturated, anoxic environments with low pH). Other interesting moderate correlations exist among the fire history and three periodicities of the NAM, confirming the proposition that NAM is required as an ignition source in desert fire regimes.
Table 1 does not indicate a correlation between NAM and ENSO, however Jones et al. (2015) suggest a relationship between phases of ENSO and the strength of the NAM after a transition period that occurred ∼4000–3000 cal year BP. This suggestion is consistent with a comparison to the Moy et al. (2002) ENSO data (Figure 2) which shows general correspondence between the NAM and ENSO.
∼8000–6000 cal Year BP – No Fire
El Niño/Southern Oscillation Quiescent, No North American Monsoon Time Series Data
From 8000 to 6000 cal year BP ciénega sediment deposition is only active at SBNWR. There is essentially no evidence of fire at SBNWR between 8000 and 6000 cal year BP given that average charcoal accumulation rate (CHAR) was zero during most of this interval. During this time period, the preservation ratio is very low (Figure 2), indicating that the ciénega was not continuously wetted. This aridity corresponds to a low occurrence of El Niño-like events (i.e., quiescent El Niño) (Moy et al., 2002). Desert wetlands rely on elevated water tables recharged by winter precipitation (Flint et al., 2004; Scanlon et al., 2006). The infrequent El Niño events from 8000 to 6000 cal year BP would have resulted in dry surface conditions at SBNWR.
The Jones et al. (2015) time series does not include this period so a direct comparison could not be made for the Moy et al. (2002) time series. However, Barron et al. (2012) propose the early Holocene NAM would have been widespread but potentially weaker than later in the Holocene. In desert ecosystems the occurrence of fire depends on the presence and continuity of fine fuels to transmit fire (e.g., Brooks and Pyke, 2002). Currently fine fuel production in the desert is associated with winter storms [e.g., desert superblooms (Winkler and Brooks, 2020)] which then provide a continuous fuel source that is prone to drying, which can be readily ignited by lightning during the late-summer NAM season. Tagestad et al. (2016) indicate that most large fires in the Mojave occur when there is a significant summer moisture component (NAM), but without the fine fuels of winter precipitation the occurrence of fire decreases. This early Holocene period of low winter/moderate-high summer precipitation appears to be a non-analog for the modern setting as there is currently no such described Mojave precipitation regime (Tagestad et al., 2016). The early Holocene precipitation and fire regime data from SBNWR suggests that an active NAM is not enough for large desert fires to occur; large fires also require winter moisture to generate fuels.
The influx of taxa linked to WPE were low in the early Holocene and never exceeded 19 grains/cm2/year (Figure 2), while the abundance of dry adapted Amaranthaceae dominated the pollen assemblage (Figure 4). These data in concert with the low preservation ratio also suggest overall dry conditions for this period of low fire activity.
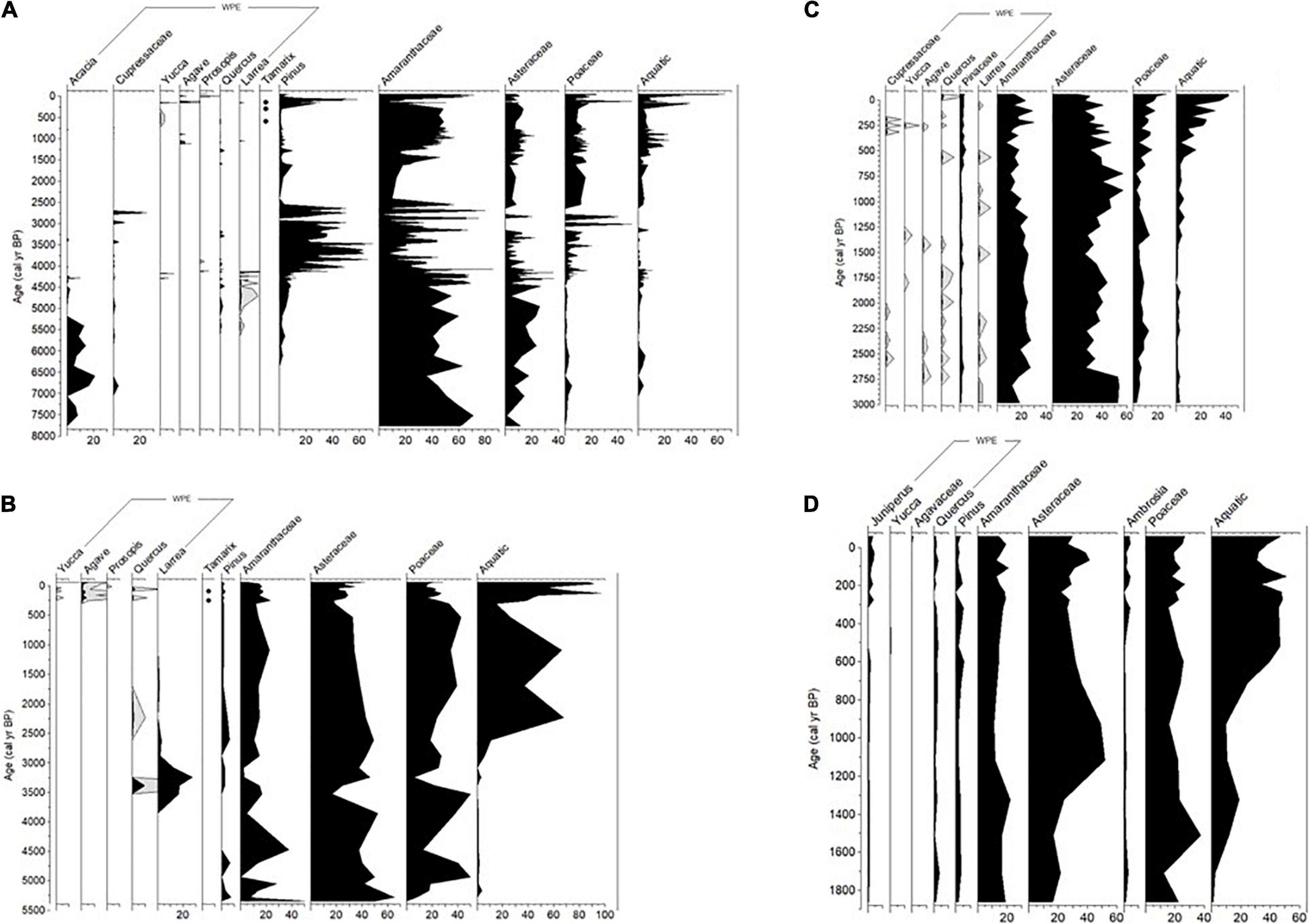
Figure 4. Pollen diagrams from (A) SBNWR, (B) Cloverdale, (C) Canelo Hills, and (D) Babocamari. Shaded curves are 10X exaggeration. Full diagrams available in Brunelle et al. (2018).
Monger (2003) presents a record of climate change for the desert SW in the context of research conducted at the Jornada Long Term Ecological Research (LTER) in the Jornada Basin, New Mexico as well as other long-term records collected from elsewhere around the region. Monger (2003) analyzed δ13C from paleosols to interpret past vegetation regimes. The δ13C values were consistent with their recorded increase of Amaranthaceae pollen and erosion that they interpret as a change from a C4 grassland to a C3 shrubland between 8000 and 5700 cal year BP. The Monger (2003) record of Amaranthaceae is in agreement with the record from SBNWR, which recorded high Amaranthaceae values during this period. The grass pollen is low at SBNWR but C4/C3 specificity cannot be determined from palynological data.
∼6000–4000 cal Year BP – Increasing Fire Frequency
El Niño/Southern Oscillation Active, North American Monsoon Moderate
During the middle Holocene, average CHAR steadily increases, likely due to continuous fuel production. It is also notable that the beginning of the deposition of wetland sediments at Cloverdale is coincident with a peak in El Niño events and the NAM at ∼4750 cal year BP (Figure 2). It is likely that enhanced winter and summer precipitation charged the aquifer to the point where standing surface water became common at that site.
The high pollen preservation index (Figure 2) also suggests that this time interval was wetter-than-previous. The timing of the preservation index increase is roughly synchronous with the onset of modern ENSO variability (Figure 2) and coincides with a stepwise change in charcoal influx in the SBNWR core (Brunelle et al., 2010). Brunelle et al. (2010) propose that the onset of ENSO active (i.e., more frequent El Niño events) led to an increase in fine fuel production (annuals) during spring, which allowed the ignitions by summer convective thunderstorms to carry across the usually sparse desert landscape. The Jones et al. (2015) Ti record of NAM begins at ∼6000 cal year BP and shows a moderate level of NAM activity. Barron et al. (2012) identify ∼6000 cal year BP as a time of spatially reduced but more intense NAM.
Woody plant encroachment reached the highest of the record at SBNWR with influx values reaching 606 grains/cm2/year at ∼4300 cal year BP. The plant assemblage at SBNWR was dominated by Amaranthaceae but an increase in Asteraceae is evident, which may reflect increased moisture and an increase in fine fuels (Figure 4A). Poaceae pollen remains low at SBNWR during the middle Holocene. The WPE at Cloverdale is near zero for this entire zone. The dominant taxa at Cloverdale during this time were Asteraceae, Amaranthaceae, and Poaceae (Figure 4B).
Monger’s (2003) Jornada Basin record also suggests that a wet period occurred between 5700 and 3200 cal year BP. The original pollen record from the desert SW was produced by Freeman (1972) from alluvial sediments in the Chihuahuan desert grassland of southeastern New Mexico. Most pollen records from the desert SW are generated by analyzing either alluvial deposits or packrat middens, which have both provided important information about environmental change over thousands of years. One challenge to interpreting alluvial and packrat midden pollen records is that these records are not continuous. Alluvial deposits are interrupted by cut and fill events, so while pollen information from a stratigraphic layer is valuable and representative for that specific period of time, it is only a discrete snapshot and not a record of continuous change. Packrat midden pollen data have the same issue. In some cases, packrat middens do have stratigraphy, but the depositional environment is still not continuous and uninterrupted sequences not always clearly evident. Dated packrat midden pollen samples again provide a representation of the vegetation at the time the deposit accumulated, but do not provide insight about the drivers of vegetation change through time.
Freeman’s (1972) record includes four sampling locations from the Gardner Spring profile. Each profile has one radiocarbon date that constrains the maximum age of the sedimentary section. The oldest sample indicates a transition from scrub to grassland at ∼5700–4500 cal year BP. The two ciénega records that capture that time period do not record a similar compositional shift. Cloverdale, which is the closest site to Gardner Springs, shows an increase in grass after ∼3500 cal year BP (Figure 4B). SBNWR does show a distinct shrubland/grassland transition, but not until ∼4000 cal year BP (Figure 4A). The Gardner Springs record does not include charcoal data.
Van Devender (1990) indicates, based on packrat midden records, that the Chihuahuan desert scrub developed at ∼4500 cal year BP. The SBNWR and Cloverdale pollen records do not show any distinct changes in vegetation at this time, but there are distinct shifts in fire occurrence that align with the onset of ENSO active conditions and an increase in NAM activity. It is likely that the changes Van Devender (1990) identified are also linked to changes in ENSO and the NAM.
Van Devender (1995) also suggests that the middle Holocene desert grassland was likely mesic, not dry as suggested by Antevs (1955). Van Devender’s assertion is well supported by a wet period in both ciénega reconstructions that capture the middle Holocene (SBNWR and Cloverdale). Both SBNWR and Cloverdale pollen preservation was elevated from ∼6000 to 4000 cal year BP, indicating wet conditions on both ciénegas.
∼4000 to 2250 cal Year BP – Stable Fire Activity
El Niño/Southern Oscillation Low, North American Monsoon Variable
The ciénega records indicate that fire activity (CHAR) remains stable in this zone despite a transition back to drier-than-previous conditions from ∼4000 to 2250 cal year BP. The interpretation of dryness is based on the dramatic decrease in the pollen preservation index (Figure 2). Starting around 4500 cal year BP the number of El Niño events decreased until about 3500 cal year BP. Part of this period is also identified as a “transition zone” by Jones et al. (2015) with respect to the record of the NAM due to the distinctly lower activity (Figure 2). It does start high at the beginning of this zone (∼4000 cal year BP) but declines quickly to its lowest of this period by about 3500 cal year BP, after which it steadily increases. It is likely that the decrease in El Niño events, which deliver winter precipitation to the desert SW, and the NAM which provides a summer moisture source, were drivers in shifting the hydroclimate regime to an overall drier state during the ∼4000–2250 cal year BP time period.
Although already low, the WPE at both SBNWR and Cloverdale decreased slightly during this time period. The Canelo Hills ciénega initiates at about 3000 cal year BP during the increase in El Niño events (Figure 2) likely due to enhanced water table recharge and the persistent surpluses of surface water at that site. Grasses become more abundant during this time which is most evident from SBNWR (Figure 4A).
There seems to be regional agreement among the ciénega reconstructions and other longer-term records from nearby sites. Monger’s (2003) Jornada Basin record suggests that a dry period occurred from 3200 to 1500 cal year BP. While this interval does not perfectly align with the ciénega records, given the discontinuous nature of the Jornada Basin record it is likely that the sites mirror the prevailing climate history for the desert SW region. Van Devender (1995) identifies the oldest creosote (Larrea spp.) from a packrat midden deposit dating to 3650 cal year BP. Based on the pollen records presented here, the oldest creosote pollen dates are 3524 cal year BP (Cloverdale Ciénega) and 4171 cal year BP (SBNWR), which is consistent with Van Devender’s estimation.
Studying periods of arroyo cutting is another way to get prehistoric desert SW climate information (Waters and Haynes, 2001). Waters and Haynes (2001) state that the cause for arroyo cutting is still debated, however, their data suggest that the arroyo cutting prior to Euro-American land modification largely occurred during dry-to-wet transitions. The decreased precipitation associated with dry climate intervals caused water tables to lower and vegetative cover to decrease. The loss of vegetation to anchor soils enhances vulnerability to erosion. As a dry climate interval transitions to a wet period, intense precipitation events, such as those typical of a monsoonal thunderstorm, will readily mobilize the unbound soil causing rapid erosion and arroyo entrenchment. Comparing the arroyo cutting record with the Moy et al. (2002) time series of El Niño events and the Jones et al. (2015) NAM time series supports the argument that arroyo formation is triggered by shifts from dry to wet conditions. Figure 2 shows five of the six synchronous arroyo cutting events identified by Waters and Haynes (2001) (yellow bars). In nearly every case both the ENSO and NAM time series show a shift from dry to wet conditions.
∼2250 cal Year BP to ∼1500 AD (500 cal Year BP) – Increasing Fire Activity
El Niño/Southern Oscillation and North American Monsoon Increasing
Average CHAR remains high with an increase starting at about 1250 cal year BP. Rising CHAR is probably the result of more frequent El Niño episodes and subsequent periods of fine fuel production. Based on the return to a high preservation ratio (Figure 2), the period ∼2250–500 cal year BP is identified as generally wet. Enhanced moisture corresponds well with periods of very frequent El Niño events as recorded by Moy et al. (2002) and an overall increase in NAM activity reported by Jones et al. (2015; Figure 2).
Like Cloverdale and Canelo, the Babocamari ciénega initiates during a peak in the number of El Niño events, again likely due to the elevated water table that allows for the existence of surface water. At SBNWR WPE increases slightly, Cloverdale records no WPE, Canelo’s WPE is stable, and WPE at Babocamari starts relatively high (∼1,000 grains/cm2/year) but decreases toward present. The high level of WPE at the onset of the Babocamari record might suggest landcover conversion from a shrub dominated- to a ciénega-dominated system. This conversion can be seen in Figure 4D with the marked increase in aquatic taxa. Poaceae pollen remains high or increases during this zone. Monger’s (2003) Jornada Basin record indicates a return to more mesic conditions from ∼1500 cal year BP to 1850 AD, however, Freeman (1972) proposes a dry period at ∼2300 cal year BP, from Gardner Springs. Again, while not perfectly aligned with the sediment core records, these overall shifts are fairly consistent across the SW.
500 cal Year BP (1500 AD) to Modern – Increasing Fire Activity
El Niño/Southern Oscillation Decreasing, North American Monsoon Stable
This zone is treated separately because of the influence of Euro-Americans on the desert ecosystem that began around 1500 AD when they arrived in the SW (Hill et al., 2004). The CHAR is relatively low at the start of this zone, but increases toward present, with an inflection at ∼200 cal year BP (1750 AD) (Figure 3). Like CHAR, the preservation is relatively low at the start of this zone, but increases toward present, also with an inflection at ∼200 cal year BP (1750 AD). The 1750 AD inflection (Figure 3) also coincides with the only El Niño events identified as “extreme” for the entire 500-year period (Brönnimann et al., 2007). Increases in snowpack would facilitate both the saturation of the ciénega systems from a higher water table and the production of fine fuels following wet winters.
To add additional data, the 2200 year long July precipitation reconstruction from Stahle et al. (2009) was used identify extreme July precipitation events as a reconstruction of the NAM. The strong correspondence of extreme July precipitation events (Stahle et al., 2009) and high NAM activity (Jones et al., 2015) serves to validate both records. It is also interesting that over the last 500 years the majority of extreme July precipitation events occur between ∼500 and ∼250 years ago; while the extreme El Niño events are all occur in the last 125 years (Brönnimann et al., 2007). CHAR is stable during the period of large July precipitation events, but increases when there are more extreme El Niño events supporting the assertion that fine fuel production from winter precipitation is a critical driver of desert fire.
Average CHAR and pollen preservation trends essentially mirror the WPE from the Cloverdale site, exhibiting a relationship between desert fire and woody fuels at this site. WPE has also increased since 1750 AD, representing a change from fine (grasses) to coarse (woody plants) fuels. The WPE at Babocamari also increases slightly, but at SBNWR and Canelo it remains fairly constant. The more distinct change in WPE at Cloverdale may be the artifact of its location within the Chihuahuan desert grassland bioregion, as opposed to the Sonoran Desert classification of the other sites. The difference in these desert systems may shape their sensitivity to environmental changes, or a sensitivity to recording them, or both.
The pollen data of aquatic taxa from the Canelo and Babocamari pollen records from 1500 AD to modern increase distinctly beginning around 1250 AD (700 cal year BP) (Figures 4C,D). This upward trend likely reflects the formation and expansion of the ciénega systems. Functional ciénegas are known to support continued ciénega development by the wetland plants slowing flood pulses and causing the flood water to drop sediment. Over time this process will cause an increase in the spatial footprint of the ciénega system. SBNWR and Cloverdale pollen data indicate an increase in aquatic taxa starting about 1700 AD (250 cal year BP). WPE also increases at this time at Cloverdale, indicating that despite the encroachment of woody plants around the ciénega, the wetted ciénega surface was stable enough to deter woody plants from establishing on the wetland itself.
The WPE was closely examined in Brunelle et al. (2014) to try and isolate the triggers for the encroachment. Brunelle et al. (2014) examined six different possible mechanisms for WPE including (1) natural variability, (2) drought, (3) ENSO, (4) changes in CO2 concentrations, (5) changes in fire regime and (6) introduction of grazing. They were able to rule out all but increases in CO2 concentrations and grazing, which is consistent with other assessments. For example, Monger (2003) puts forward grazing and climate as possible drivers of WPE. Monger (2003) subsequently eliminated climate based on Conley et al. (1992). A higher-resolution analysis of the Cloverdale WPE data now demonstrates that CO2 can likely be eliminated as the main cause of WPE at this site because the increase in CO2 follows the increase in WPE. Grazing stands out as the main driver of WPE at Cloverdale. Although not the direct cause of WPE, increased CO2 can create a multiplier effect.
Drivers of Fire and Conclusion
Paleoenvironmental records are well suited to contribute on the topic of this special volume, “Fire Regimes in Desert Ecosystems: Drivers, Impacts and Changes.” Ciénega sediment records of past environments are long and continuous, containing information on fire regimes, changes in vegetation type and abundance, and climate dynamics including the seasonality and relative abundance of precipitation on millennial time scales. Drivers of fire regime in the desert SW include prevailing climate conditions, vegetation type, and vegetation density. In the desert, WPE into areas that were only sparsely vegetated or dominated by grasses will also alter fire regime – which is also extractable from a sedimentary pollen record.
The first continuous record of fire from the desert SW (SBNWR) demonstrated that if there are no El Niño events (e.g., before ∼4500 cal year BP) then there is essentially no fire (Figure 2; Figure 4 in Brunelle et al., 2010). This relationship between fire occurrence and ENSO conditions indicates that a fundamental control on desert fire regimes is the abundance of winter precipitation, which over the long-term, has controlled the production of the fine, continuous fuels needed to carry fire in the desert. It is compelling that not only the baseline of fire occurrence is driven by ENSO being active, variations in fire regime reflect more quiescent or active periods of ENSO as well.
There is not a single time series that compares the activity or strength of NAM with fire history over long time periods. However, it is known that currently most desert fires occur when there is a significant amount of summer precipitation (Tagestad et al., 2016). It is also well known that lightning generated from monsoonal storms provides the ignition source needed for desert fire. This manuscript presents the ciénega reconstructions of fire and vegetation in concert with two millennial scale reconstructions of NAM. The main conclusions that can be drawn from these data is that the NAM and El Niño events appear to follow similar trends in highs and lows (Figure 2) and both are needed to support desert fire. Given the availability of the ciénega cores presented here, a next step in understanding more about past NAM variability would be to extract the Ti record from these other desert sites for more, local time series. It would also be valuable to explore the possible additive effect of ENSO + NAM on charcoal abundance (fire) over time.
Each ciénega record also provides a record of vegetation changes (Figure 4). Overall, the ciénega records were consistent with other records of vegetation from the desert SW, e.g., the correspondence among the ciénega records and those from the Jornada Basin site (Monger, 2003). From the pollen time series, an index of WPE was also extracted to specifically examine the history of changes in the abundance of woody plants at each ciénega site with the fire history data (Figure 2). Based on the WPE and average CHAR time series, there is a relationship between woody fuels and charcoal abundance (Figure 2). This is likely a result of woody fuels leaving greater charcoal evidence (less complete combustion than grasses). If we look at the Fire Effects Information System (2021) data for SW desert grasslands vs. Sonoran shrublands, grasslands have a shorter fire return interval. The influence of fuel type on the average CHAR may be minimized by the fact that shrublands burn less frequently but produce more charcoal, where grassland burn more often with less charcoal produced. It would be a valuable calibration study to assess this quantitatively.
Finally, there is the modern issue of invasive plants in the desert SW. Paleoecological records can detect the arrival of some invasive plants (e.g., Tamarix, see Figure 4), but one invasive that cannot be detected using currently available palynological methods are non-native grasses. Grass pollen (family Poaceae) is only identifiable to the family taxonomic level, with the notable exception of maize (Zea mays). Higher taxonomic specificity in the grass family can be attained with phytolith analyses. It may be valuable to develop some phytolith records from the desert SW to get information on grass diversity beyond the historical record. There are several invasive grasses established in the desert SW, including both annuals and perennials. For example, some annual invasive species include cheatgrass (Bromus tectorum), red brome (Bromus rubens), and medusahead (Taeniatherum caput-medusae), and some perennials such as buffelgrass (Pennisetum ciliare), natal grass (Melinus repens) and Lehmann lovegrass (Eragrostis lehmanniana) (Brooks and Pyke, 2002). These non-native, invasive grasses are changing the fuel situation in desert SW ecosystems by creating an annual, connected fuel source. Many of these grasses were deliberately introduced. For example, buffelgrass was intentionally and widely broadcast in the 1930s as potential forage for grazing cattle. Buffelgrass is drought tolerant and not reliant on El Niño events to germinate and produce fine fuels. It is likely that invasive species, like buffelgrass and others, will mimic El Niño-driven fuel conditions and lead to increased fire in desert ecosystems. This novel component in desert fire ecology certainly leads to new challenges in land and fire management, and will change the fundamental fire regime of the desert unless the spread of buffelgrass and other invasive species is brought under control.
El Niño/Southern Oscillation is a critical driver of desert SW fire regimes and it is important to consider its behavior in the future. The model forecasts are not completely consistent but seem to favor an increase in El Niño events. Capotondi et al. (2020) indicate that the last two decades have seen more El Niño events than any other time on record. Capotondi et al. (2020) is consistent with predictions by Cai et al. (2015) that ENSO events (both El Niño and La Niña) would increase in strength and frequency with intensified surface warming in the southern Pacific Ocean. Importantly, Capotondi et al. (2020) also state that current research is still investigating the relationship between this increased frequency and climate change. Stronger and/or more frequent El Niño events would certainly facilitate the occurrence of desert fire.
The ignition source provided by the NAM is also critical to the occurrence of desert fire so the NAM under climate change conditions also needs to be considered. van der Wiel et al. (2016) suggested that in a 2 X CO2 environment that there would be no changes in extreme precipitation events associated with the NAM. However, later work by Pascale et al. (2017) indicates a significant decrease in NAM driven summer precipitation. They indicate that this decrease is a result of uniform sea surface warming causing increased atmospheric stability and thus less convection. Pascale et al. (2018) also suggest the amounts of mean summer time rainfall will decrease in a 2 X CO2 setting, but the possibility of extreme events will increase. Adaptation measures are suggested to deal with the decrease in monsoonal rainfall and the possibility of more severe rainfall events (Pascale et al., 2017, 2018).
Millennial-scale records of fire, vegetation and climate regimes provide a perspective on desert SW fire regimes prior to the influence of Euro-Americans. While this information is certainly helpful in understanding the fundamental drivers of desert SW fire, the basic components of the desert fire regime are changing. With the modern changes in climate, including warming, drying and changes in ENSO and NAM, along with the spread of invasive grasses like buffelgrass we will need to continue to study past, present and future drivers of fire in the desert SW.
Data Availability Statement
The original contributions presented in the study are publicly available. These data can be found here: https://doi.org/10.21233/JMWD-YJ04, 067C-HQ21, 1Z1S-GX38, 3A17-2216, and RKTY-2H81.
Author Contributions
The author solely conducted the new analyses and wrote the manuscript. The previously published work includes efforts by many other colleagues but are cited as such throughout the text.
Funding
This work was supported by the Environmental Protection Agency program: Southwestern Consortium for Environmental Research and Policy.
Conflict of Interest
The author declares that the research was conducted in the absence of any commercial or financial relationships that could be construed as a potential conflict of interest.
Publisher’s Note
All claims expressed in this article are solely those of the authors and do not necessarily represent those of their affiliated organizations, or those of the publisher, the editors and the reviewers. Any product that may be evaluated in this article, or claim that may be made by its manufacturer, is not guaranteed or endorsed by the publisher.
Acknowledgments
The author would like to dedicate this manuscript to George Hepner, Emeritus Professor of Geography, University of Utah. Hepner’s early encouragement and support (through the Southwestern Consortium for Environmental Research and Policy) of this borderland paleoecology research launched nearly two decades (and counting) of work in an ecosystem with few long-term records. The author is particularly grateful to Jennifer Watt and Jesse Morris for their thoughtful and thorough reviews of an early draft of this manuscript, their suggestions resulted in significant improvement. Thank you to MB and reviewers for constructive feedback on the first draft of the manuscript. I also thank the team that conducted this work over the years, specifically my desert research partner, Thomas Minckley. For field, lab, and analytical contributions I’d also like to thank Shawn Blissett, Stephanie Cobabe, Jose Delgadillo, Corinne Springer, Salek Shafiqullah, Trevor Hare, Sky Island Alliance, The Nature Conservancy, Van Clothier, Bill Radke, Brenda Guzman, Joe and Valer Austin, Shizuo Nishizawa, Todd Daines, Jessica Spencer, The Brophy Family, and Dale Turner.
Footnotes
References
Adams, D. K., and Comrie, A. C. (1997). The North American monsoon. Bull. Am. Meteorol. Soc. 78, 2197–2213.
Antevs, E. (1955). Geologic-climatic dating in the West. Am. Antiq. 20, 317–335. doi: 10.2307/277066
Archer, S. R., and Predick, K. I. (2008). Climate change and ecosystems of the southwestern United States. Rangelands 30, 23–28. doi: 10.2111/1551-501x(2008)30[23:ccaeot]2.0.co;2
Barron, J. A., Metcalfe, S. E., and Addison, J. A. (2012). Response of the North American monsoon to regional changes in ocean surface temperature. Paleoceanography 27:A3206. doi: 10.1098/rstb.2007.0037
Bestelmeyer, B. T., Peters, D. P. S., Archer, S. R., Browning, D. M., Okin, G. S., Schooley, R. L., et al. (2018). The grassland–shrubland regime shift in the southwestern United States: misconceptions and their implications for management. Bioscience 68, 678–690.
Brönnimann, S., Xoplaki, E., Casty, C., Pauling, A., and Luterbacher, J. (2007). ENSO influence on Europe during the last centuries. Clim. Dyn. 28, 181–197.
Brooks, M.L., and Pyke, D.A. (2002). “Invasive plants and fire in the deserts of North America”, in Proceedings of the Invasive Plant Workshop: The Role of Fire in the Control and Spread of Invasive Species, eds K. E. M. Galley, T. P. Wilson (Tallahassee: Tall Timbers Research Station). 1–14.
Brown, D. E., and Minnich, R. A. (1986). Fire and changes in creosote bush scrub of the western Sonoran Desert, California. Am. Midl. Nat. 116, 411–422. doi: 10.2307/2425750
Brunelle, A., Minckley, T. A., Blissett, S., Cobabe, S. K., and Guzman, B. L. (2010). A∼ 8000 year fire history from an Arizona/Sonora borderland ciénega. J. Arid Environ. 74, 475–481. doi: 10.1016/j.jaridenv.2009.10.006
Brunelle, A., Minckley, T. A., Delgadillo, J., and Blissett, S. (2014). A long-term perspective on woody plant encroachment in the desert southwest, New M exico, USA. J. Veg. Sci. 25, 829–838. doi: 10.1111/jvs.12125
Brunelle, A., Minckley, T. A., Shinker, J. J., and Heyer, J. (2018). Filling a geographical gap: new paleoecological reconstructions from the desert Southwest, USA. Front. Earth Sci. 6:106. doi: 10.3389/feart.2018.00106
Cai, W., Santoso, A., Wang, G., Yeh, S. W., An, S. I., Cobb, K. M., et al. (2015). ENSO and greenhouse warming. Nat. Clim. Chang. 5, 849–859. doi: 10.1126/science.1104666
Capotondi, A., Deser, C., Phillips, A. S., Okumura, Y., and Larson, S. M. (2020). ENSO and Pacific Decadal Variability in the Community Earth System Model Version 2. J. Adv. Model. Earth Syst. 12:e2019MS002022.
Cayan, D. R., Redmond, K. T., and Riddle, L. G. (1999). ENSO and Hydrologic Extremes in the Western United States. J. Clim. 12, 2881–2893. doi: 10.1175/1520-0442(1999)012<2881:eaheit>2.0.co;2
Cole, A., and Cole, C. (2015). An overview of aridland ciénagas, with proposals for their classification, restoration and preservation. New Mexico Bot. 28–56.
Cole, J. E., and Cook, E. R. (1998). The changing relationship between ENSO variability and moisture balance in the continental United States. Geophy. Res. Lett. 25, 4529–4532.
Comrie, A. C., and Glenn, E. C. (1998). Principal components-based regionalization of precipitation regimes across the southwest United States and northern Mexico, with an application to monsoon precipitation variability. Clim. Res. 10, 201–215. doi: 10.3354/cr010201
Conley, W., Conley, M. R., and Karl, T. R. (1992). A computational study of episodic events and historical context in long-term ecological processes: climate and grazing in the northern Chihuahuan desert. Coenoses 7, 55–60.
Davis, O. K., Minckley, T., Moutoux, T., Jull, T., and Kalin, B. (2002). The transformation of Sonoran Desert wetlands following the historic decrease of burning. J. Arid Environ. 50, 393–412. doi: 10.1006/jare.2001.0914
Dettinger, M. D., Cayan, D. R., Mccabe, G. M., and Marengo, J. A. (2000). “Multiscale streamflow variability associated with El Niño/Southern Oscillation,” in El Niño and the Southern Oscillation-Multiscale Variability and Global and Regional Impacts, eds H. F. Diaz and V. Markgraf (Cambridge: Cambridge University Press), 113–146. doi: 10.1017/cbo9780511573125.005
Diem, J. E., and Brown, D. P. (2006). Tropospheric moisture and monsoonal rainfall over the southwestern United States. J. Geophys. Res. 111:D16112. doi: 10.1029/2005JD006836
Enfield, D. B. (1992). “El Nino Historical and Paleoclimatic Aspects of the Southern Oscillation,” in El Nino: Historical and Paleoclimatic Aspects of the Southern Oscillation, eds H. F. Diaz and V. Markgraf (Cambridge: Cambridge University Press), 95–117.
Felger, R. S. (2007). “Living resources at the center of the Sonoran Desert: Native American plant and animal utilization,” in Dry Borders: Great Natural Reserves of the Sonoran Desert, eds Felger and B. Broyles (Salt Lake City: University of Utah Press), 147–192.
Fire Effects Information System [FEIS] (2021). Fire Effects Information System. Available online at: https://www.feis-crs.org/feis/ (Accessed June 28, 2021).
Flint, A. L., Flint, L. E., and Hevesi, J. A. (2004). “Fundamental concepts of recharge in the desert southwest: a regional modeling perspective,” in Groundwater Recharge in a Desert Environment: The Southwestern United States, eds J. F. Hogan, F. M. Phillips, and B. R. Scanlon (Washington: American Geophysical Union), 9. doi: 10.1142/9789811213380_0002
Freeman, C. E. (1972). Pollen study of some Holocene alluvial deposits in Dona Ana County, southern New Mexico. Tex. J. Sci. 24, 203–220.
Gershunov, A., and Barnett, T. P. (1998). ENSO Influence on Intraseasonal Extreme Rainfall and Temperature Frequencies in the Contiguous United States: observations and Model Results. J. Clim. 11, 1575–1586. doi: 10.1175/1520-0442(1998)011<1575:eioier>2.0.co;2
Hall, S. A. (1985). “Quaternary Pollen Analysis and Vegetational History of the Southwest,” in Pollen Records of Late-Quaternary North American Sediments, eds V. M. Bryant Jr. and R. G. Holloway (Texas: American Association of Stratigraphic Palynologists Foundation). doi: 10.1111/j.1469-8137.1995.tb04325.x
Hendrickson, D. A., and Minckley, W. L. (1985). Cienegas-vanishing climax communities of the American Southwest. Desert Plants 6, 131–175.
Higgins, R. W., Chen, Y., and Douglas, A. V. (1999). Interannual variability of the North American warm season precipitation regime. J. Clim. 12, 653–680. doi: 10.1175/1520-0442(1999)012<0653:ivotna>2.0.co;2
Hill, J. B., Clark, J. J., Doelle, W. H., and Lyons, P. D. (2004). Prehistoric demography in the Southwest: migration, coalescence, and Hohokam population decline. Am. Antiq. 69, 689–716. doi: 10.2307/4128444
Jones, M. D., Metcalfe, S. E., Davies, S. J., and Noren, A. (2015). Late Holocene climate reorganisation and the north American monsoon. Quat. Sci. Rev. 124, 290–295.
Lanesky, D., Logan, B., Brown, R., and Hine, A. (1979). A new approach to portable vibracoring underwater and on land. J. Sediment. Res. 49, 654–657.
Liu, Z., Lu, Z., Wen, X., Otto-Bliesner, B. L., Timmermann, A., and Cobb, K. M. (2014). Evolution and forcing mechanisms of El Niño over the past 21,000 years. Nature 515, 550–553. doi: 10.1038/ncomms4701
Mack, F., Hoffstadt, J., Esser, G., and Goldammer, J. G. (1996). “Modeling the influence of vegetation fires on the global carbon cycle,” in Biomass Burning and Global Change, ed. J. S. Levine (Cambridge: The MIT Press), 149–159.
McPherson, G. R. (1995). “The role of fire in the desert grasslands,” in The desert grassland, eds M. P. McClaran and T. R. van Devender (Tucson: University of Arizona Press), 130–151.
Minckley, T. A., and Brunelle, A. (2007). Paleohydrology and growth of a desert cienega. J. Arid Environ. 69, 420–431. doi: 10.1016/j.jaridenv.2006.10.014
Minckley, T. A., Clementz, M., Brunelle, A., and Klopfenstein, G. A. (2009). Isotopic analysis of wetland development in the american southwest. Holocene 19, 737–744. doi: 10.1177/0959683609105297
Minckley, T. A., Turner, D. S., and Weinstein, S. R. (2013). The relevance of wetland conservation in arid regions: a re-examination of vanishing communities in the American Southwest. J. Arid Environ. 88, 213–221. doi: 10.1016/j.jaridenv.2012.09.001
Mock, C. J. (1996). Climatic controls and spatial variations of precipitation in the western United States. J. Clim. 9, 1111–1125. doi: 10.1175/1520-0442(1996)009<1111:ccasvo>2.0.co;2
Monger, H. C. (2003). “Millennial-scale climate variability and ecosystem resonse at the Jornada LTER site,” in Climate Variability and Ecosystem Respoinse at Long-Term Ecological Research Sites, eds D. Greenland, D. G. Goodin, and R. C. Smith (Oxford: Oxford University Press), 341–369.
Moy, C. M., Seltzer, G. O., Rodbell, D. T., and Anderson, D. M. (2002). Variability of el nino/southern oscillation activity at millennial timescales during the holocene epoch. Nature 420, 162–165. doi: 10.1038/nature01194
Pascale, S., Boos, W. R., Bordoni, S., Delworth, T. L., Kapnick, S. B., Murakami, H., et al. (2017). Weakening of the North American monsoon with global warming. Nat. Clim. Chang. 7, 806–812. doi: 10.1038/nclimate3412
Pascale, S., Kapnick, S. B., Bordoni, S., and Delworth, T. L. (2018). The influence of CO2 forcing on North American monsoon moisture surges. J. Clim. 31, 7949–7968.
Ratner, B. (2009). The correlation coefficient: its values range between+ 1/- 1, or do they? J. Target. Meas. Anal. Market. 17, 139–142. doi: 10.1057/jt.2009.5
Ropelewski, C. F., and Halpert, M. S. (1986). North American precipitation and temperature patterns associated with the El Niño/Southern Oscillation (ENSO). Mon. Weather Rev. 114, 2352–2362. doi: 10.1175/1520-0493(1986)114<2352:napatp>2.0.co;2
Scanlon, B. R., Keese, K. E., Flint, A. L., Flint, L. E., Gaye, C. B., Edmunds, M., et al. (2006). Global synthesis of groundwater recharge in semiarid and arid regions. Hydrol. Process. 20, 3335–3370. doi: 10.1002/hyp.6335
Schussman, H., and Gori, D. F. (2004). An ecological assessment of the Bureau of Land Management’s Current Fire Management Plans: Materials and Recommendations for future fire planning. Arizona: The Nature Conservancy.
Sheppard, P. R., Comrie, A. C., Packin, G. D., Angersbach, K., and Hughes, M. K. (2002). The climate of the US Southwest. Clim. Res. 21, 219–238.
Shinker, J. J. (2010). Visualizing spatial heterogeneity of western U.S. climate variability. Earth Interact. 14, 1–15.
Shinker, J. J., and Bartlein, P. J. (2009). Visualizing the large-scale patterns of ENSO-related climate anomalies in North America. Earth Interact. 13, 1–50. doi: 10.1175/2008ei244.1
Stahle, D. W., Cleaveland, M. K., Grissino-Mayer, H. D., Griffin, R. D., Fye, F. K., Therrell, M. D., et al. (2009). Cool-and warm-season precipitation reconstructions over western New Mexico. J. Clim. 22, 3729–3750. doi: 10.1175/2008jcli2752.1
Tagestad, J., Brooks, M., Cullinan, V., Downs, J., and McKinley, R. (2016). Precipitation regime classification for the Mojave Desert: implications for fire occurrence. J. Arid Environ. 124, 388–397. doi: 10.1016/j.jaridenv.2015.09.002
Turner, R. M., Webb, R. H., Bowers, J. E., and Hastings, J. R. (2003). The Changing Mile Revisited: An Ecological Study of Vegetation Change With Time in the Lower Mile of an Arid and Semiarid Region. Tucson: University of Arizona Press, 300.
van der Wiel, K., Kapnick, S. B., Vecchi, G. A., Cooke, W. F., Delworth, T. L., Jia, L., et al. (2016). The resolution dependence of contiguous US precipitation extremes in response to CO2 forcing. J. Clim. 29, 7991–8012. doi: 10.1175/jcli-d-16-0307.1
Van Devender, T. R. (1990). Late quaternary vegetation and climate of the Sonoran Desert, United States and Mexico. Packrat Middens 40, 134–165. doi: 10.2307/j.ctv21wj578.10
Van Devender, T. R. (1995). “Desert grassland history,” in The Desert Grassland, eds T. R. Van Devender and M. P. McClaran (Tucson: The University of Arizona Press), 68–99.
Wallace, J. M., and Gutzler, D. S. (1981). Teleconnections in the Geopotential Height Field during the Northern Hemisphere Winter. Mon. Weather Rev. 109, 784–812. doi: 10.1175/1520-0493(1981)109<0784:titghf>2.0.co;2
Waters, M. R., and Haynes, C. V. (2001). Late Quaternary arroyo formation and climate change in the American Southwest. Geology 29, 399–402. doi: 10.1130/0091-7613(2001)029<0399:lqafac>2.0.co;2
Keywords: ciénega, Mexico, pollen, charcoal, woody plant encroachment
Citation: Brunelle A (2022) Interactions Among the Fire, Vegetation, the North American Monsoon and the El Niño-Southern Oscillation in the North American Desert Southwest. Front. Ecol. Evol. 10:656462. doi: 10.3389/fevo.2022.656462
Received: 20 January 2021; Accepted: 04 February 2022;
Published: 27 April 2022.
Edited by:
Matthew Brooks, Western Ecological Research Center, United States Geological Survey (USGS), United StatesReviewed by:
Gianalberto Losapio, University of Lausanne, SwitzerlandEddie John Van Etten, Edith Cowan University, Australia
Copyright © 2022 Brunelle. This is an open-access article distributed under the terms of the Creative Commons Attribution License (CC BY). The use, distribution or reproduction in other forums is permitted, provided the original author(s) and the copyright owner(s) are credited and that the original publication in this journal is cited, in accordance with accepted academic practice. No use, distribution or reproduction is permitted which does not comply with these terms.
*Correspondence: Andrea Brunelle, YW5kcmVhLmJydW5lbGxlQGdlb2cudXRhaC5lZHU=