- 1Department of Entomology and MOA Key Laboratory of Pest Monitoring and Green Management, Chinese Medicinal Herbs Research Center and College of Plant Protection, China Agricultural University, Beijing, China
- 2Institute of Plant Protection, Ningxia Academy of Agriculture and Forestry Sciences, Yinchuan, China
Introduction: Perennial Chinese licorice, Glycyrrhiza uralensis, is an important medicinal plant. Diorhabda tarsalis, a leaf beetle, is a serious insect pest on the plant and cause serious yield losses every year and is attracted to healthy and pest-damaged licorice by plant volatiles.
Aim: The biologically active components of the volatiles released from G. uralensis have not been reported; the components of the volatiles that attract D. tarsalis need to be identified. Such compounds could potentially be used for monitoring and mass-trapping pests.
Methods: GC-EAD, GC-MS, EAG, Y-shaped olfactometer behavioral bioassays, and field trials were performed to identify the components and composition of active volatiles.
Results: Male and virgin female adults were generally attracted to volatiles from licorice, and volatiles from pest-infested plants were more attractive. Four compounds from licorice elicited a significant electrophysiological response (EAD) and were confirmed by EAG, including hexanal, (Z)-3-hexenal, (Z)-3-hexen-1-ol, and (E)-2-hexenal. With the exception of the (E)-2-hexenal, these molecules significantly attracted adults in individual behavioral bioassays, and a proportional mixture corresponding to beetle-damaged licorice of hexanal, (Z)-3-hexenal, (Z)-3-hexen-1-ol, and (E)-2-hexenal (8.78:15.26:57.24:18.72) was most effective for attracting D. tarsalis in the field, attracted a mean of 26 ± 7.19 beetles per trap.
Discussion: D. tarsalis was attracted to volatiles from healthy and herbivore-induced G. uralensis under both laboratory and field conditions. The aforementioned compounds show considerable potential for commercial application to monitor and control D. tarsalis populations.
1. Introduction
Plant volatiles function as interspecific communication substances. They regulate insect behavior, including host location, oviposition site selection, self-defense, and aggregation (Beyaert and Hilker, 2014). Most volatile organic compounds (VOCs) contain 6-C esters, terpenes, ketones, aldehydes, and alcohols. Closely-related plant species often release structurally and functionally similar mixtures of volatile secondary metabolites (Ameye et al., 2018). Some phytophagous insects can detect host odors from long distances (Shao et al., 2021), and use them to locate host plants as food sources (Magalhães et al., 2018; Xu and Turlings, 2018). The composition and quantity of volatiles released by host plants can change significantly after insect feeding (Piesik et al., 2014; Mukherjee et al., 2015; Malik et al., 2016; Mitra et al., 2017). Many volatile organic compounds from plants play a crucial role in the plant’s defense system.
Most herbivore-induced plant volatiles (HIPVs) attract natural enemies of pests and present an indirect defense, while some organic compounds from plants deter herbivores directly (Unsicker et al., 2009), thereby priming defense of uninjured organs on the same plant (Rodriguez-Saona et al., 2009). However, some HIPV are also attractive to pests. For example, the volatiles from Solena amplexicaulis (Lam.) Gandhi (Cucurbitaceae) induced by feeding by Aulacophora fovecollis (Lucas) (Coleoptera: Chrysomelidae) are even stronger attractants to A. fovecollis (Sarkar et al., 2015). Changes in plant volatile constituents may depend on the levels of insect damage and feeding time (Mann et al., 2021).
Glycyrrhiza uralensis Fisch. (Chinese licorice, Leguminoseae) is a medicinal perennial herb. It is the most frequently used substance among more than 2,000 materials used in traditional Chinese medicine (Chen et al., 2021). Diorhabda tarsalis Weise (Coleoptera: Chrysomelidae) is a destructive monophagous pest of G. uralensis (Qi et al., 2008) in northwestern China and southeastern Siberia, with larvae and adults that feed solely on this plant Diorhabda tarsalis larvae develop through three instars, and two to three generations occur each year in northwestern China (Supplementary Figure 1). The beetles oviposit and pupate in the top layer of soil. Newly hatched larvae and newly emerged adults search for and migrate to licorice plants for feeding. At room temperature, the durations of the egg, larval, and pupal stages were 9.66 d, 13.50 d and 5.49 d, respectively; adult longevity was 26 d (Zhang et al., 2004).
Volatiles of G. uralensis could modulate the behavior of D. tarsalis. More importantly, these chemicals might help implement comprehensive pest monitoring and control measures based on odor-aided luring of beetles (Camelo et al., 2007). However, the biologically active components of the volatiles released from G. uralensis have not been reported. In order to develop monitoring and eco-friendly control strategies for D. tarsalis management, an analysis of the active components and biological evaluation of the volatiles produced by G. uralensis, and their effects on unmated adult D. tarsalis, urgently needed to be studied. We tested the following hypotheses: (1) that adult D. tarsalis would be attracted by active volatile compounds from G. uralensis; and (2) that a blend of volatile compounds would elicit a stronger attractant response than any of the individual component compounds.
2. Materials and methods
2.1. Insects and plants
Diorhabda tarsalis adults were collected from G. uralensis in Yinchuan City, NingXia Hui Autonomous Region, China, and reared in the laboratory (Ningxia Key Laboratory of Plant Pest Control) at 25 ± 1°C, 60 ± 5% relative humidity, and a 14:10 h (L:D) photoperiod. The beetles were reared in a material cage (30 cm × 30 cm × 40 cm) with a sandy substrate and fed on licorice leaves. Eggs and pupae were placed in Petri dishes containing filter paper and cotton balls moistened with water to maintain high humidity. Hatching and eclosion occurred in darkness [0:24 h (L:D)]. We used virgin adults three days post-eclosion in the experiments. A year-old G. uralensis possessing about 50 leaves were used for the experiments.
2.2. Collection of volatiles from G. uralensis
To assess volatile chemicals produced by G. uralensis, we used plants without feeding damage and plants infested for 24 h and 72 h by 50 virgin adult D. tarsalis (sex ratio 1:1) (damaged plants), and collected volatiles with headspace adsorption. One plant was enclosed in a 20 × 40 cm plastic oven bag. An adsorbent trap consisting of a borosilicate glass tube (13 cm length, 0.7 cm o.d.) containing Porapak-Q (80–100 mesh, 200 mg, Sigma-Aldrich, USA) was connected to one side of the bag, and an activated charcoal filter was attached at the opposite end. Continuous collection over 3 h at a circulated air flow of about 200 mL/min was sustained by an air sampler (QC-1S, China). Eight parallel samples were collected for each treatment. Adsorbents were eluted with chromatographic grade dichloromethane into 1-mL screw-top sample vials, and concentrated to 0.25 mL in a stream of nitrogen gas. The samples were sealed and stored at –20°C for later use. Background volatiles were analyzed in plant volatile collection, EAG, GC-EAD, and behavioral bioassays.
2.3. Identification of active volatile compounds
Antennae of female and male D. tarsalis were excised at the base, positioned between gold wire electrodes, and immersed in saline-filled (0.9% NaCl) wells in a small acrylic plastic holder. The airstream flowing over antennae (500 mL/min) was humidified by bubbling through distilled water before entering an EAD interface. A 4-μL sample of captured volatiles was injected in a GC-2010 gas chromatograph (Shimadzu, Japan) with a DB-WAX capillary GC column (30 m, 0.25 mm, 0.25 μm film thickness; Agilent Technologies, USA). The sample inlet and detector were set at 230°C and 260°C, respectively. The temperature program was: initial temperature of 40°C held for 2 min, followed by 5°C/min increase to 120°C, 15°C/min increase to 240°C, which was held for 4 min. Nitrogen was used as the carrier gas at a flow rate of 2 mL/min. Total detection time was 30 min. Every treatment was performed using at least three biological replicates. Data were recorded with a GC-EAD acquisition system (GC/EAD 32, version 4.4, Syntech, Germany).
Components with GC-EAD activity was identified by GC-MS. A GC-MS-QP2101 (Shimadzu, Japan) was used for gas chromatography-mass spectrometry. A DB-WAX capillary column (30 m, 0.25 mm, 0.25 μm film thickness, Agilent Technologies) was used as the GC column. A 5-μL aliquot was injected in splitless mode. The sample inlet was set at 230°C. The temperature protocol was an initial temperature of 40°C held for 1 min, increased by 5°C/min to 180°C, then increased by 10°C/min to 240°C, which was held for 4 min. Electron ionization was performed at 70 eV ionization energy. The temperature of the ion source was 250°C. The mass range scanned was 40–500 amu. Helium was used as the carrier gas at a flow rate of 1 mL/min. The reference libraries for spectroscopic identification were NIST27 and NIST147. Identified compounds were further validated and characterized by comparison with the retention times and mass spectra of authentic standards analyzed under the same conditions.
Concentrations of active compounds in headspace volatile collections were determined by internal standard method (Yang et al., 2017). A total of 5 ng/μL of methyl benzoate was added in collections as the internal quantification standard. Authentic standards of active compounds were used to calculate the correcting factors. The program of GC-MS same as above.
2.4. Verification of electrophysiological activity
Candidate compounds that showed strong GC-EAD signals were verified by EAG. Electrophysiological responses of adult females and males to candidate compounds were tested at concentrations of 0.01 μg/μL, 0.1 μg/μL, 1 μg/μL, and 10 μg/μL. Dichloromethane was used as the solvent control. Antennae of female and male D. tarsalis were excised at the base, and the base was connected to a reference electrode and the tip to a recording electrode. A silver-silver chloride wire was inserted into the electrodes and connected to a signal amplifier. The conductive solution was a 750 mg/mL NaCl + 35 mg/mL KCl + 29 mg/mL CaCl2⋅2H2O aqueous solution. A clean, moist airstream was continuously blown across the preparation at 400 mL/min. We placed 10 μL of test solution on a 2.5 cm × 0.5 cm filter paper strip and balanced it in a closed Pasteur tube for 20 s before delivering the airstream to the antennae. Each stimulation ran for 0.2 s, separated by a 2 min interval. An EAG2000 acquisition system (Syntech, Netherlands) was used to monitor electrical signals generated by the antennae and collect data (Chen et al., 2019). Three replicates were used for candidate compounds from each treatment, with five antennae measured during each replicate.
2.5. Behavioral bioassays of D. tarsalis in the laboratory
The behavioral responses of virgin female and male D. tarsalis to volatile chemicals from healthy and pest-damaged G. uralensis were tested using a glass Y-shaped olfactometer. For the pest-damaged treatment, a total of 50 adult (25 females and 25 males) D. tarsalis were allowed to feed for 72 h. The olfactometer consisted of a 25 cm stem and two 15 cm arms, each 3 cm in diameter. Arms were separated at a 45° angle. An air flow of 500 mL min–1 was purified by passing it through activated charcoal, moistened with distilled water in a gas-washing bottle, and split into two streams. The arms of the olfactometer were connected to 5-L conical flasks with Teflon tubing, with healthy licorice in one flask and damaged licorice in the other. Other experiments used a blank flask instead of damaged licorice as a blank control. Each insect was observed for 5 min during the bioassay, and a “choice” was recorded when it entered and stayed in an arm for at least 1 min. Fifty adult females and fifty adult males were individually tested per treatment. The position of the arms was exchanged when a repetition was done. All conical flasks and connecting tubes were thoroughly cleaned with alcohol and dried with a hairdryer after individual trial (applicable to each subsequent experiment).
Behavioral responses of female and male D. tarsalis to EAG active compounds were measured using Y-shaped olfactometry. The concentration of the active compounds tested was 10 μg/μL, dichloromethane was used as solvent control.
2.6. Field trials
Active compounds were mixed to replicate the emission ratio of components in undamaged licorice (0 h, OC1), and licorice after 24 h (OC2) and 72 h (OC3) of insect damage. Observed ratios of hexanal, (Z)-3-hexenal, (Z)-3-hexen-1-ol and (E)-2-hexenal were 0:10.04:62.42:27.53 (OC1), 0:10.56:69.44:20.00 (OC2) and 8.78:15.26:57.24:18.72 (OC3), respectively. Field experiments were performed at the licorice base in Gaoshawo Town, Yanchi County in a 0.67 ha G. uralensis field. Active compounds were dissolved in proportion in dichloromethane to prepare 100 μg/μL solutions. Silicon-rubber lures loaded with 100 μg of active compounds were prepared by adsorption, affixed to the center of 20 cm × 10 cm yellow sticky traps (Guangren Biotechnology Co., Ltd.; China), and hung at 2/3 height of G. uralensis plants (about 40 cm) in the field. Individual active components and dichloromethane (DCM) were used as controls. Three replicates were used for each of the eight treatments. The distance between randomly installed traps was 20 m. Numbers of adults caught on each trap was recorded after 5 d.
2.7. Data analysis
Concentrations of volatile compounds were calculated using internal standard quantitation: Cx = f × Cr × Ax/Ar, where Cx is the concentration of the sample, f is the correcting factor, Cr is the concentration of the standard compound, Ax is the peak area of the sample, and Ar is the peak area of the standard compound. Behavioral bioassay data were analyzed by χ2 test for significance of choice preferences. Field trial and EAG response data were assessed for homogeneity of variance and compared using one-way ANOVA followed by Tukey’s multiple range test, the differences between two treatments were analyzed by Student’s t-test. All test data were statistically analyzed and graphed using GraphPad Prism 8 (San Diego, CA, USA).
3. Results
3.1. Behavioral responses of D. tarsalis
Behavioral responses of adult D. tarsalis to healthy and pest-damaged G. uralensis were tested. Both adult females and males of D. tarsalis were significantly attracted to G. uralensis compared to clean air (χ2 = 68.89, P < 0.01; χ2 = 51.18, P < 0.01), and the attraction rates for females and males were 92.00% and 86.27%, respectively. Beetles showed a significant preference for pest-damaged compared to healthy host plants (χ2 = 28.6712, P < 0.01 and χ2 = 29.4449, P < 0.01 for females and males, respectively). The selection of pest-damaged host plants by females and males was 77.27% and 77.63%, respectively (Figure 1).
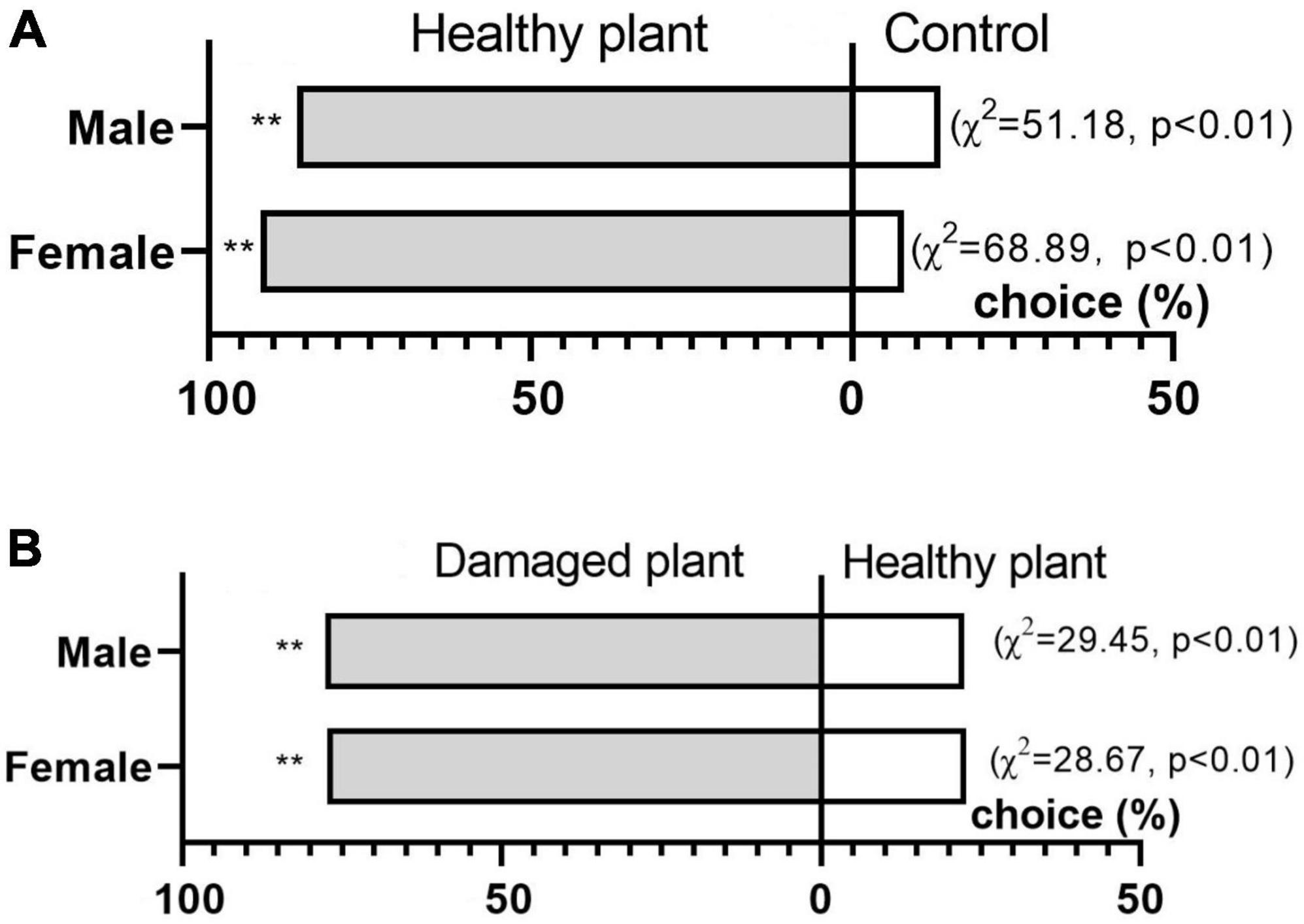
Figure 1. Behavioral responses of female and male Diorhabda tarsalis to (A) healthy licorice and blank control or (B) healthy and damaged licorice. Asterisks indicate a statistically significant difference by chi-squared test (**p = 0.01; n = 50).
3.2. Identification of active volatile compounds
Active volatile compounds were identified and quantified by GC-EAD and GC-MS, after their activity in healthy and pest-damaged G. uralensis was confirmed. Four volatile compounds from licorice showed electrophysiological activity in D. tarsalis adults, as shown in GC-EAD analyses (Figure 2): hexanal (C6H12O), (Z)-3-hexenal (C6H10O), (E)-2-hexenal (C6H10O), and (Z)-3-hexen-1-ol (C6H12O). Their concentrations in the three plant treatments varied, as indicated by mass spectrometry (Supplementary Figure 2). These identified compounds were further validated and characterized by comparison of their retention times and mass spectra with synthetic compounds under the same conditions. Concentrations of these compounds from licorice varied at different times following damage. At 0 h, 24 h, and 72 h post-damage, concentrations of headspace volatile collections were respectively 0 ng/μL hexanal, 0.092 ng/μL (Z)-3-hexenal (10.04%), 0.574 ng/μL (Z)-3-hexen-1-ol (62.42%), 0.253 ng/μL (E)-2-hexenal (27.53%); 0 ng/μL hexanal, 0.045 ng/μL (Z)-3-hexenal (10.56%), 0.298 ng/μL (Z)-3-hexen-1-ol (69.44%), 0.086 ng/μL (E)-2-hexenal (20.00%), and 0.037 ng/μL hexanal (8.78%), 0.063 ng/μL (Z)-3-hexenal (15.26%), 0.239 ng/μL (Z)-3-hexen-1-ol (57.24%), 0.078 ng/μL (E)-2-hexenal (18.72%) (Figure 3).
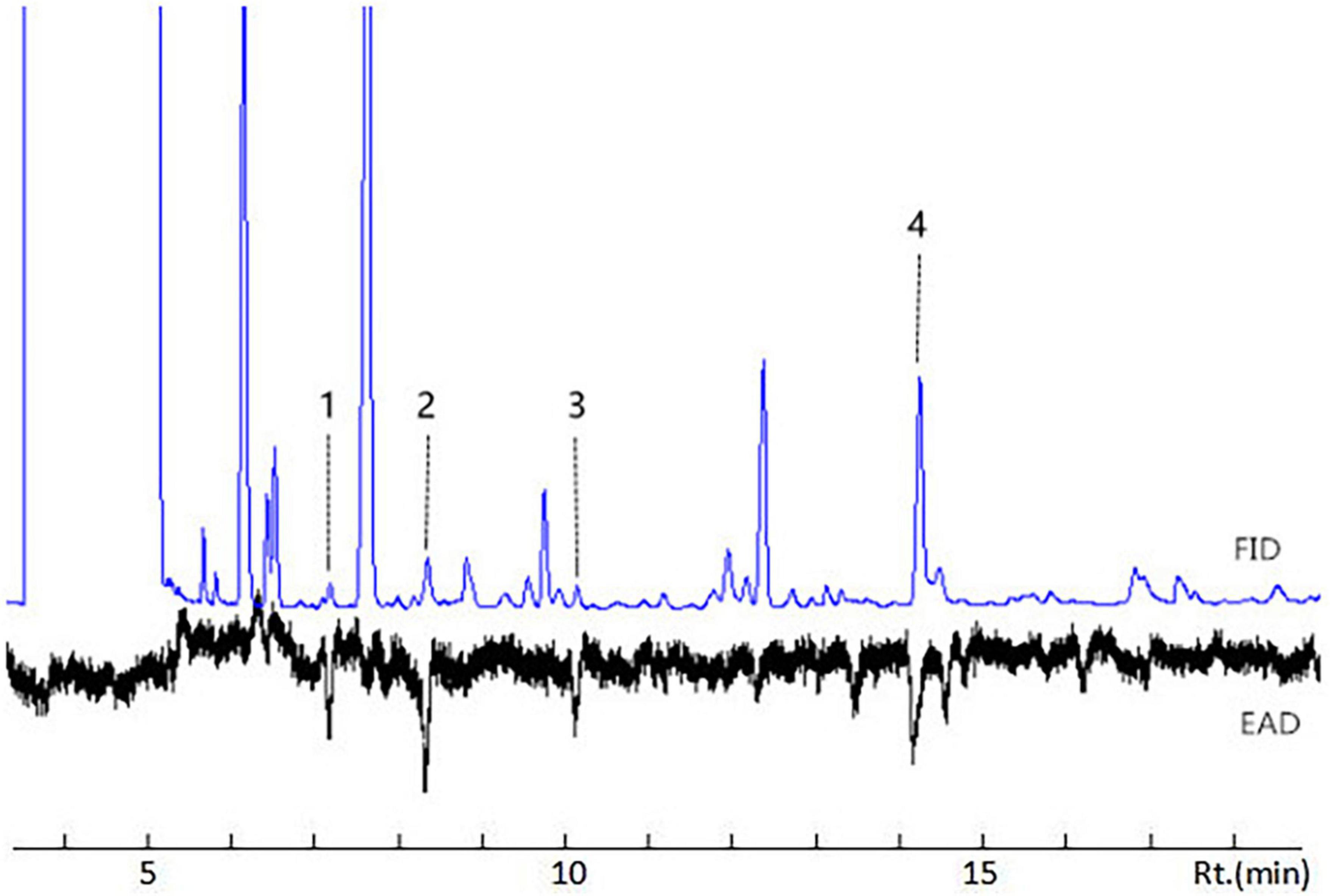
Figure 2. GC-EAD responses of female and male Diorhabda tarsalis to volatiles extracted from licorice. Every treatment was performed using at least three biological replicates. 1: Hexanal; 2: (Z)-3-hexenal; 3: (E)-2-hexenal; 4: (Z)-3-hexen-1-ol. Licorice headspace volatiles 0 h and 24 h after insect damage showed distinct peaks 2, 3, and 4 in the GC profiles (FID). After 72 h, peaks 1, 2, 3, and 4 were observed.
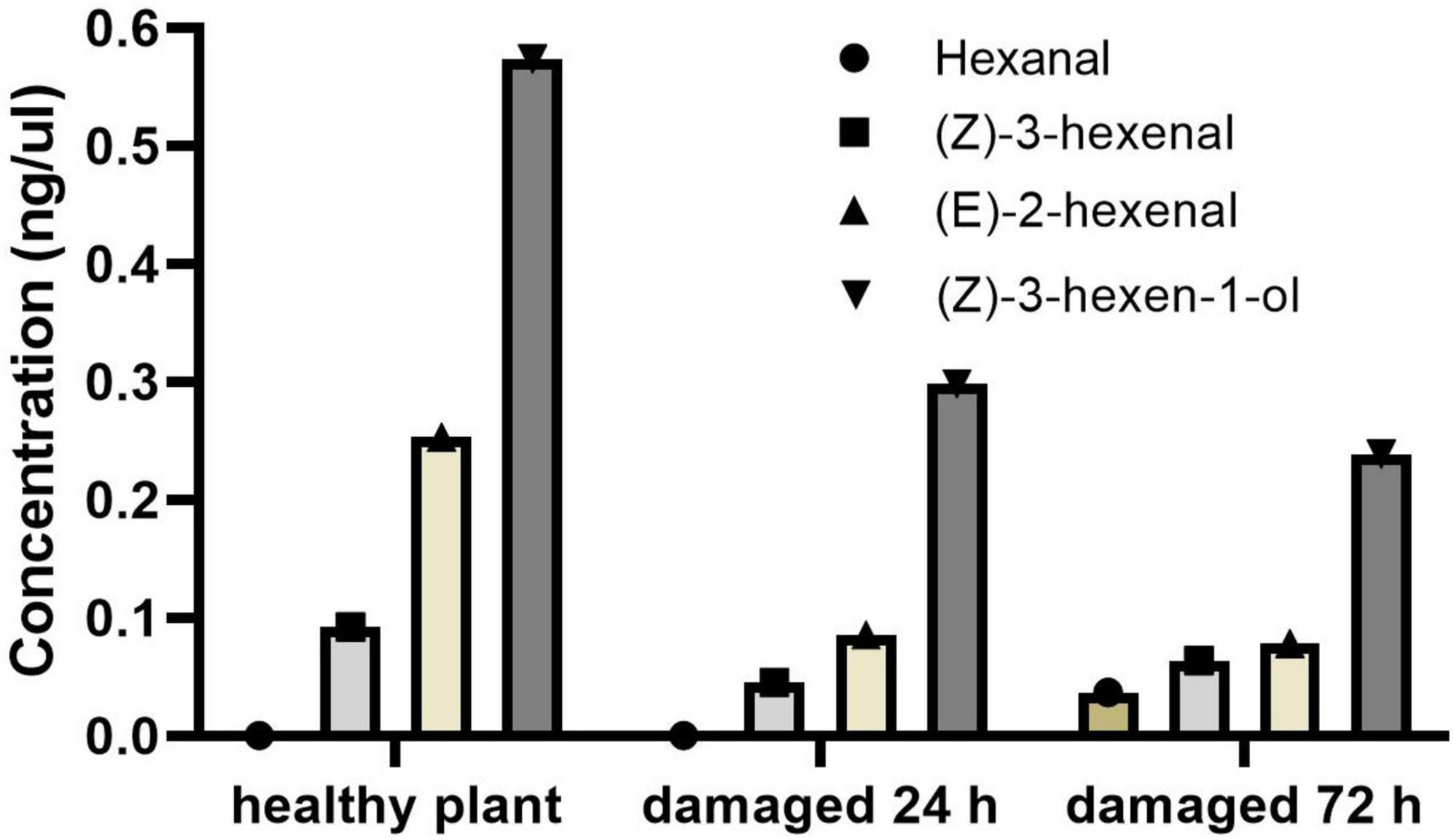
Figure 3. Concentrations of four active compounds in headspace volatile collections released from healthy licorice and plants damaged for 24 h and 72 h.
3.3. EAG responses of active compounds
The four candidate compounds that showed strong GC-EAD signals were diluted into four test concentration solutions to verify their electrophysiological activity by EAG. Contrasting concentrations of the four active compounds all stimulated significantly different EAG responses in both female and male D. tarsalis: hexanal (female: F4,10 = 14.115, P = 0.0004; male: F4,10 = 8.536, P = 0.0029), (Z)-3-hexenal (female: F4,10 = 4.922, P = 0.0187; male: F4,10 = 4.955, P = 0.0183), (E)-2-hexenal (female: F4,10 = 13.934, P = 0.0004; male: F4,10 = 4.055, P = 0.033) and (Z)-3-hexen-1-ol (female: F4,10 = 6.705, P = 0.0069; male: F4,10 = 5.962, P = 0.0102). Post hoc tests showed an increasing EAG response for all compounds with increased concentration.
Responses of the females and males to 10 μg/μL of hexanal and (Z)-3-hexenal were significantly higher than the solvent controls (hexanal: t4 = 8.2056, P = 0.0012 and t4 = 4.9828, P = 0.0076, respectively; (Z)-3-hexenal: t4 = 3.3737, P = 0.0279 and t4 = 4.0552, P = 0.0154, respectively). Responses of the females or males to two concentrations of (Z)-3-hexen-1-ol were significantly higher than the control: at 1 μg/μL, t4 = 10.8341, P = 0.0004 and t4 = 6.2743, P = 0.0033; and at 10 μg/μL, t4 = 4.0189, P = 0.0159 and t4 = 12.6526, P = 0.0002, respectively. Responses of the females and males to 10 μg/μL of (E)-2-hexenal also were significantly higher than the solvent: t4 = 4.9963, P = 0.0075 and t4 = 3.0873, P = 0.0367, respectively. Overall, activity increased as concentration increased. The males also exhibited a stronger response than females to all concentrations of the four compounds (Figure 4).
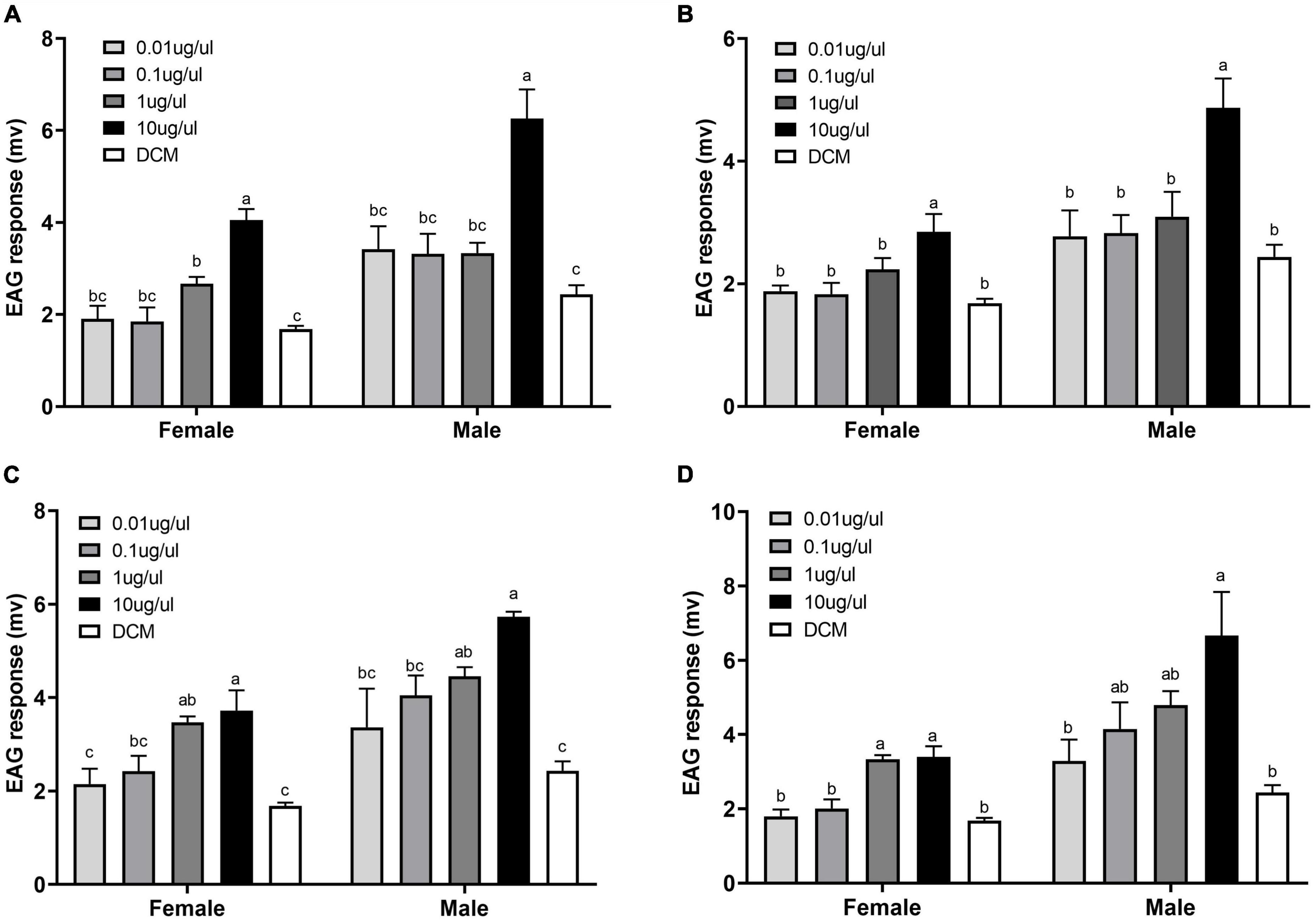
Figure 4. EAG response of female and male Diorhabda tarsalis to concentration gradients of four specific components, (A) Hexanal, (B) (Z)-3-hexenal, (C) (Z)-3-hexen-1-ol, and (D) E-2-hexenal. The experiment used three biological replicates. Values are means ± SD. Letters above bars indicate significant differences by Tukey’s tests (p < 0.05).
3.4. Behavioral bioassays for active compounds
Four volatile compounds that had been verified with electrophysiological activity by EAG were measured with a Y-shaped olfactometer. Both genders of D. tarsalis displayed a significant preference for 10 μg/μL (Z)-3-hexenal, hexanal and (Z)-3-hexen-1-ol (females: χ2 = 35.84, P < 0.01; χ2 = 20.1, P < 0.01; χ2 = 13.53, P = 0.0002 and males: χ2 = 19.08, P < 0.01; χ2 = 22.98, P < 0.01; χ2 = 14.85, P = 0.0001, respectively). The selection by females of (Z)-3-hexenal, hexanal and (Z)-3-hexen-1-ol was 80.43, 72.92 and 68.89%, and that of males was 72.34%, 74.47% and 69.77% respectively. No significant preference was observed for either adult females (χ2 = 2.58, P = 0.10) or males (χ2 = 0.40, P = 0.528) for (E)-2-hexenal (Figure 5).
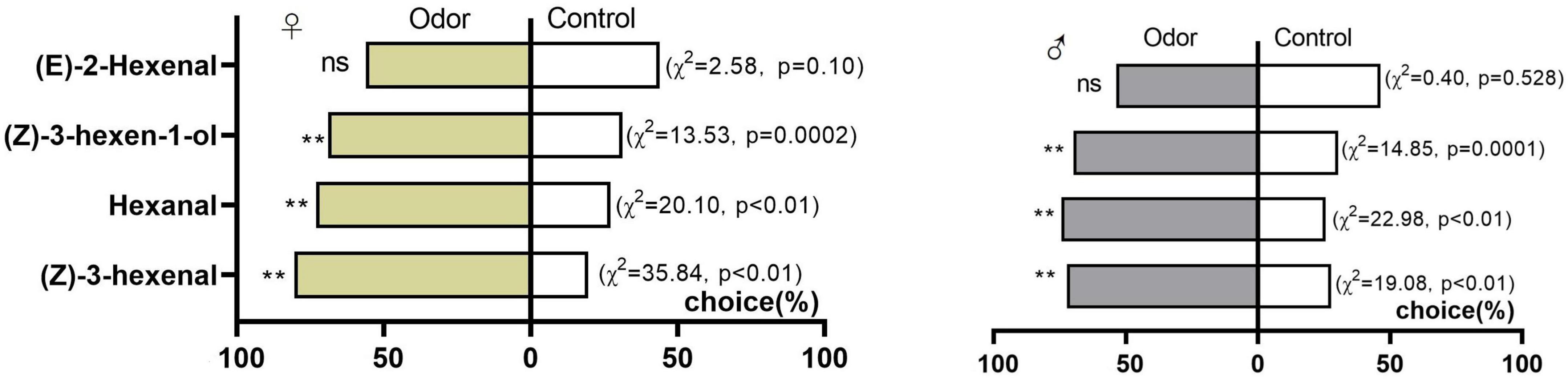
Figure 5. Behavioral responses of female and male D. tarsalis to four volatile compounds. Asterisks indicate a statistically significant difference by chi-squared test (**p = 0.01, ns means not significant; n = 50).
3.5. Attractive effect of combinations of active compounds in the field
Multi-component attractants (OC1, OC2, and OC3) were prepared according to the ratio of the four active compounds from G. uralensis, and their effects were evaluated in the field. Blend OC3 attracted significantly more D. tarsalis adults than OC1, OC2, single-component attractants, or the control solvent (F7,16 = 9.592, P = 0.0002). A statistically significant preference was observed for hexanal (t4 = 4.3811, P = 0.011), (Z)-3-hexenal (t4 = 4.4778, P = 0.011), (Z)-3-hexen-1-ol (t4 = 3.4887, P = 0.039), (E)-2-hexenal (t4 = 4.5626, P = 0.010), OC1 (t4 = 3.2077, P = 0.049) and OC2 (t4 = 3.9176, P = 0.017). OC3 attracted a mean of 26 ± 7.19 beetles per trap (Figure 6).
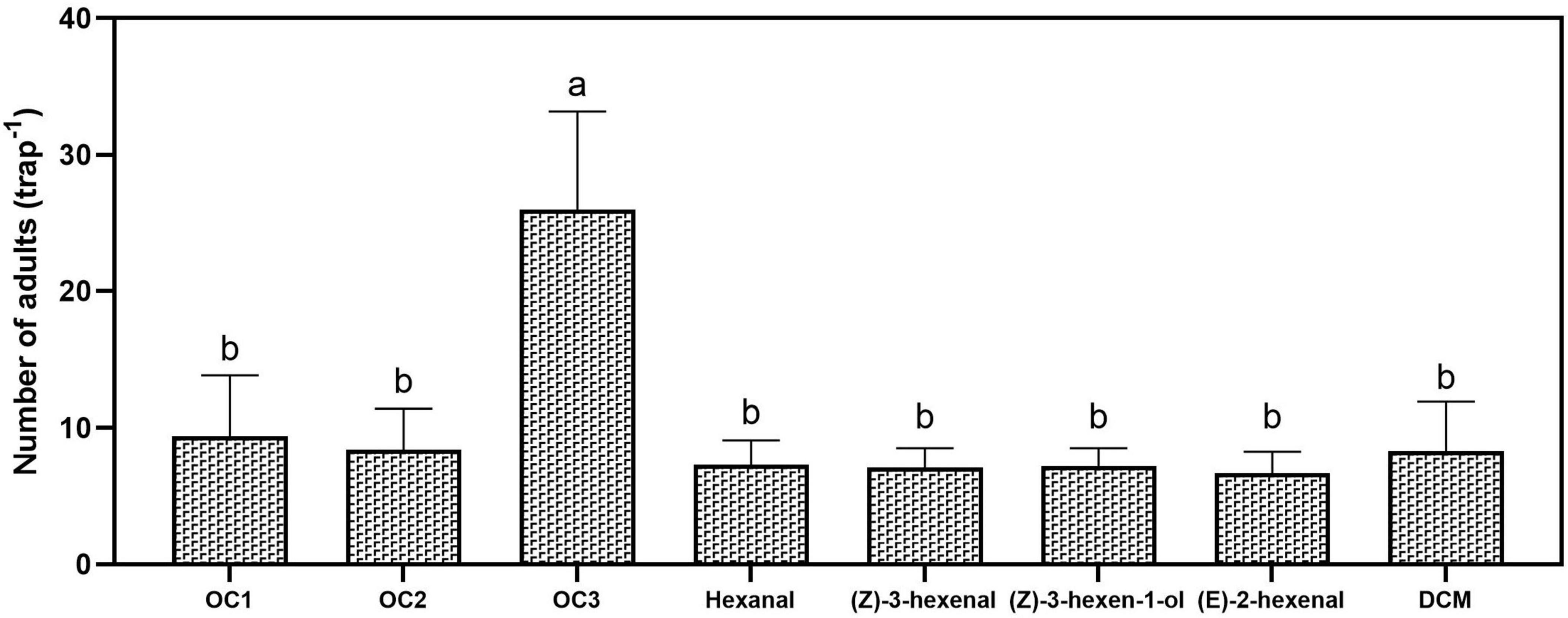
Figure 6. Average numbers of Diorhabda tarsalis adults trapped using different attractants over five days. The experiment used three replicates in field. Values are means ± SD. Different letters indicate significant differences between treatments by Tukey’s tests (p < 0.05).
4. Conclusion
Hexanal, (Z)-3-hexenal, (Z)-3-hexen-1-ol and (E)-2-hexenal from G. uralensis were main active components that appealed to D. tarsalis. The blends of these four compounds mixed in identical proportions to the volatiles emitted from the plant were highly attractive to the pest in the field, particularly the blend corresponding to damage after 72 h of feeding (OC3), and this mixture is a potential chemical agent for monitoring and controlling D. tarsalis in the future.
5. Discussion
The relationship between G. uralensis and its herbivore pest D. tarsalis is odor-mediated. The volatile profile of the plant was previously reported, but the active components that regulated the behaviors of the pest insects were unclear (He et al., 2016). Here we found that hexanal, (Z)-3-hexenal, and (Z)-3-hexen-1-ol are the primary attractants produced by licorice that stimulate beetle behavior. Conversely, hexanal is a strong repellent for Holotrichia parallela Motschulsky (Coleoptera: Scarabaeidae) at low concentrations (Wang et al., 2020). Hexanal is a volatile secondary metabolite of G. uralensis produced after D. tarsalis-induced damage. HIPVs may include more than 200 compounds. Their relative composition can change in response to different phytophagous species, different developmental stages, plant organs that are attacked, and abiotic factors (Poelman et al., 2012; McCormick et al., 2016). Although no significant preference was observed by Y-shaped olfactometry, (E)-2-hexena was used in field trials according to it played a synergistic effect presumably. We found that mixtures of the four active compounds from G. uralensis in natural ratios corresponding to different D. tarsalis damage levels were more strongly attractive to the beetles in the field than the single active compounds. The responses of the beetles to these compounds requires further study.
Host positioning is required for insect feeding and is also important for population maintenance and growth. The ecological significance of this behavior goes beyond simply finding food sources. Aggregation on host plants helps insects overcome host defenses, reduces predation pressure, and increases mating opportunities (Ruxton and Sherratt, 2006). The aggregation behavior of D. carinulata (Desbrochers) and D. elongata (Brullé) were also regulated by volatiles from their host plants, Tamarix spp., and the main electrophysiologically and behaviorally active components were determined to be (E)-2-hexenal, (Z)-3-hexenal, (Z)-3-hexen-1-ol, and (Z)-3-hexenyl acetate (Cossé et al., 2006; Gaffke et al., 2018). The former three components also were included among the volatiles produced by G. uralensis that regulated the aggregation behavior of D. tarsalis, but (Z)-3-hexenyl acetate was not one of the active volatiles isolated from G. uralensis. Higher concentrations of the active components (E)-2-hexenal, (Z)-3-hexenal, (Z)-3-hexen-1-ol, and (Z)-3-hexenyl acetate were emitted from beetle-infested saltcedar, in particular (E)-2-hexena, while lesser amounts of (Z)-3-hexenal, (Z)-3-hexen-1-ol and (E)-2-hexenal were released by beetle-damaged licorice, although the emission of hexanal from the licorice increased significantly after damage.
Therefore, phytophagous insects of the same genus that are closely related to each other utilize similar semiochemical compositions from their host plants, but the ratios of the crucial active ingredients differ. Diorhabda tarsalis can locate G. uralensis by responding to the volatile chemicals released from the plant. Conversely, many plants release defensive substances after insect damage (Tamiru and Khan, 2017) that directly repel pests or attract the pest’s natural enemies (Lucchi et al., 2017; Salamanca et al., 2019; Rim et al., 2020). For example, 4-oxo-(E)-2-hexenal emitted from Tamarix spp. induced by adult D. carinulata was repellent to reproductive adults of both sexes and first instar larvae (Gaffke et al., 2020). However, pests often use such defense substances as new attractants. For example, potato plants attract more potato beetles after initial feeding damage (von Mérey et al., 2013). In this study, hexanal strongly attracted adult D. tarsalis females and males; impacts of this compound on their natural enemies still needs to be addressed.
Diorhabda tarsalis is a monophagous pest, and the four active substances involved in host positioning are common green leaf volatiles (Scala et al., 2013). Other plant species produce specific substances, such as allyl isothiocyanate by crucifers via cleavage and metabolism of mustard oil glycosides, and benzaldehyde by prune trees via prunasin degradation (Visser and Van der Maas, 1978). Plant volatiles are often synergistic/interactive with insect pheromones (Dickens, 2006; Benelli et al., 2014; Ge et al., 2019). For example, male Melolontha hippocastani (Coleoptera: Scarabaeidae) accurately locate host plants with the help of plant volatiles and sex pheromones emitted by conspecific females (Reinecke et al., 2002; Ruther et al., 2002). Papaya extracts can promote male-released aggregation pheromones to attract females in Tephritidae (Landolt et al., 1992). Thus, the production and release of sex pheromones can significantly increase in some insects after stimulation with plant volatiles (Bachmann et al., 2015; Etl et al., 2016). Relationships among active chemicals with pheromones of D. tarsalis or its natural enemies need further research. Exploration of these issues may lead to new technologies and approaches for beetle monitoring and control.
Data availability statement
The original contributions presented in this study are included in this article/Supplementary material, further inquiries can be directed to the corresponding author.
Author contributions
W-PS designed the experiments. H-HC, RZ, S-QT, YW, and X-LL performed the experiments and analyzed the data. H-HC and W-PS wrote the manuscript. All authors contributed to the article and approved the submitted version.
Funding
This research was supported by the Leading Fund of Science and Technology Innovation of Ningxia (Grant No. DWX-2019002).
Acknowledgments
We thank the reviewers for helpful comments and valuable suggestions during the revision of the first version of the manuscript.
Conflict of interest
The authors declare that the research was conducted in the absence of any commercial or financial relationships that could be construed as a potential conflict of interest.
Publisher’s note
All claims expressed in this article are solely those of the authors and do not necessarily represent those of their affiliated organizations, or those of the publisher, the editors and the reviewers. Any product that may be evaluated in this article, or claim that may be made by its manufacturer, is not guaranteed or endorsed by the publisher.
Supplementary material
The Supplementary Material for this article can be found online at: https://www.frontiersin.org/articles/10.3389/fevo.2022.1080208/full#supplementary-material
References
Ameye, M., Allmann, S., Verwaeren, J., Smagghe, G., Haesaert, G., Schuurink, R. C., et al. (2018). Green leaf volatile production by plants: A meta-analysis. New Phytol. 220, 666–683. doi: 10.1111/nph.14671
Bachmann, G. E., Segura, D. F., Francisco, D., Laura, J. M., Josefina, R. M., Teresa, V. M., et al. (2015). Male sexual behavior and pheromone emission is enhanced by exposure to guava fruit volatiles in Anastrepha fraterculus. PLoS One 10:e0129523. doi: 10.1371/journal.pone.0124250
Benelli, G., Daane, K. M., Canale, A., Niu, C. Y., Messing, R. H., and Vargas, R. I (2014). Sexual communication and related behaviours in Tephritidae: Current knowledge and potential applications for integrated pest management. J. Pest Sci. 87, 385–405. doi: 10.1007/s10340-014-0577-3
Beyaert, I., and Hilker, M. (2014). Plant odour plumes as mediators of plant–insect interactions. Biol. Rev. Camb. Philos. Soc. 89, 68–81. doi: 10.1111/brv.12043
Camelo, L. A., Landolt, P. J., and Zack, R. S. (2007). A kairomone based attract-and-kill system effective against alfalfa looper (Lepidoptera: Noctuidae). J. Econ. Entomol. 100, 366–374. doi: 10.1603/0022-0493(2007)100[366:akbase]2.0.co;2
Chen, L., Li, Y. Y., and Shao, K. M. (2019). A practical technique for electrophysiologically recording from lamellated antenna of scarab beetle. J. Chem. Ecol. 45, 392–401. doi: 10.1007/s10886-019-01059-3
Chen, Y. W., Zou, J. Y., Sun, H., Qin, J. H., and Yang, J. Y. (2021). Metals in traditional Chinese medicinal materials (TCMM): A systematic review. Ecotoxicol. Environ. Saf. 207:111311. doi: 10.1016/j.ecoenv.2020.111311
Cossé, A. A., Bartelt, R. J., Zilkowski, B. W., Bean, D. W., and Andress, E. R. (2006). Behaviorally active green leaf volatiles for monitoring the leaf beetle, Diorhabda elongata, a biocontrol agent of saltcedar, Tamarix spp. J. Chem. Ecol. 32, 2695–2708. doi: 10.1007/s10886-006-9193-x
Dickens, J. C. (2006). Plant volatiles moderate response to aggregation pheromone in Colorado potato beetle. J. Appl. Entomol. 130, 26–31. doi: 10.1007/s00114-007-0261-z
Etl, F., Berger, A., Weber, A., Schönenberger, J., and Dötterl, S. (2016). Nocturnal plant bugs use cis-jasmone to locate inflorescences of an Araceae as feeding and mating site. J. Chem. Ecol. 42, 300–304. doi: 10.1007/s10886-016-0688-9
Gaffke, A. M., Sing, S. E., Dudley, T. L., Bean, D. W., Russak, J. A., Mafra-Neto, A., et al. (2018). Semiochemicals to enhance herbivory by Diorhabda carinulata aggregations in saltcedar (Tamarix spp.) infestations. Pest Manag. Sci. 74, 1494–1503. doi: 10.1002/ps.4848
Gaffke, A. M., Sing, S. E., Millar, J. G., Dudley, T. L., Bean, D. W., Peterson, R. K. D., et al. (2020). An herbivore-induced plant volatile from saltcedar (Tamarix spp.) is repellent to Diorhabda carinulata (Coleoptera: Chrysomelidae). Environ. Entomol. 49, 1063–1070. doi: 10.1093/ee/nvaa079
Ge, J., Li, N., Yang, J., Wei, J., and Kang, L. (2019). Female adult puncture-induced plant volatiles promote mating success of the pea leafminer via enhancing vibrational signals. Philos. Trans. R. Soc. Lond. B Biol. Sci. 374:20180318. doi: 10.1098/rstb.2018.0318
He, M., Yang, Z. Y., Guan, W. N., Gonçalves, C. M. V., Nie, J., and Wu, H. (2016). GC–MS analysis and volatile profile comparison for the characteristic smell from Liang-wai Gan Cao (Glycyrrhiza uralensis) and honey-roasting products. J. Chromatogr. Sci. 54, 879–887. doi: 10.1093/chromsci/bmw034
Landolt, P. J., Reed, H. C., and Heath, A. R. (1992). Attraction of female papaya fruit fly (Diptera: Tephritidae) to male pheromone and host fruit. Environ. Entomol. 21, 1154–1159. doi: 10.1093/ee/21.5.1154
Lucchi, A., Loni, A., Gandini, L. M., Scaramozzino, P., Loriatti, C., Ricciardo, R., et al. (2017). Using herbivore-induced plant volatiles to attract lacewing, hoverflies and parasitoid wasps in vineyards: Achievements and constraints. Bull. Insectol. 70, 273–282.
Magalhães, D. M., Borges, M., Laumann, R. A., Woodcock, C., Withall, D., Pickett, J., et al. (2018). Identification of volatile compounds involved in host location by Anthonomus grandis (Coleoptera: Curculionidae). Front. Ecol. Evol. 6:98. doi: 10.3389/fevo.2018.00098
Malik, U., Karmakar, A., and Barik, A. (2016). Attraction of the potential biocontrol agent Galerucella placida (Coleoptera: Chrysomelidae) to the volatiles of Polygonum orientale (Polygonaceae) weed leaves. Chemoecology 26, 45–58. doi: 10.1007/s00049-015-0206-5
Mann, L., Laplanche, D., Turlings, T. C. J., and Desurmont, G. A. (2021). A comparative study of plant volatiles induced by insect and gastropod herbivory. Sci. Rep. 11:23698. doi: 10.1038/s41598-021-02801-2
McCormick, A. C., Reinecke, A., Gershenzon, J., and Unsicker, S. B. (2016). Feeding experience affects the behavioral response of polyphagous gypsy moth caterpillars to herbivore induced poplar volatiles. J. Chem. Ecol. 42, 382–393. doi: 10.1007/s10886-016-0698-7
Mitra, S., Karmakar, A., Mukherjee, A., and Barik, A. (2017). The role of leaf volatiles of Ludwigia octovalvis (Jacq.) Raven in the attraction of Altica cyanea (Weber) (Coleoptera: Chrysomelidae). J. Chem. Ecol. 43, 679–692. doi: 10.1007/s10886-017-0866-4
Mukherjee, A., Sarkar, N., and Barik, A. (2015). Momordica cochinchinensis (Cucurbitaceae) leaf volatiles: Semiochemicals for host location by the insect pest, Aulacophora foveicollis (Coleoptera: Chrysomelidae). Chemoecology 25, 93–104. doi: 10.1007/s00049-014-0179-9
Piesik, D., Kalka, I., Wenda-Piesik, A., and Bocianowski, J. (2014). Apion miniatum Germ. Herbivory on the mossy sorrel, Rumex confertus willd: Induced plant volatiles and weevil orientation responses. Pol. J. Environ. Stud. 23, 2149–2156. doi: 10.15244/pjos/22418
Poelman, E. H., Bruinsma, M., Zhu, F., Weldegergis, B. T., and Boursault, A. E. (2012). Hyperparasitoids use herbivore-induced plant volatiles to locate their parasitoid host. PLoS Biol. 10:e1001435. doi: 10.1371/journal.pbio.1001435
Qi, Q. L., Wang, G. Y., Zang, S. X., and Cheng, F. Z. (2008). Bionomics of the leaf beetle Diorhabda tarsalis. Chin. Bull. Entomol. 45, 975–978.
Reinecke, A., Ruther, J., Tolasch, T., Francke, W., and Hilker, M. (2002). Alcoholism in cockchafers: Orientation of male Melolontha melolontha towards green leaf alcohols. Naturwissenschaften 89, 265–269. doi: 10.1007/s00114-002-0314-2
Rim, H., Hattori, S., and Arimura, G. (2020). Mint companion plants enhance the attraction of the generalist predator Nesidiocoris tenuis according to its experiences of conspecific mint volatiles. Sci. Rep. 10:2078. doi: 10.1038/s41598-020-58907-6
Rodriguez-Saona, C. R., Rodriguez-Saona, L. E., and Frost, C. J. (2009). Herbivore-induced volatiles in the perennial shrub, Vaccinium corymbosum, and their role in inter-branch signaling. J. Chem. Ecol. 35, 163–175. doi: 10.1007/s10886-008-9579-z
Ruther, J., Reinecke, A., and Hilker, M. (2002). Plant volatiles in the sexual communication of Melolontha hippocastani: Response towards time-dependent bouquets and novel function of (Z)-3-hexen-1-ol as a sexual kairomone. Ecol. Entomol. 27, 76–83. doi: 10.1046/j.1365-3032.2000.00183.x
Ruxton, G. D., and Sherratt, T. N. (2006). Aggregation, defence and warning signals: The evolutionary relationship. Proc. Biol. Sci. 273, 2417–2424. doi: 10.1098/rspb.2006.3570
Salamanca, J., Souza, B., Kyryczenko-Roth, V., and Rodriguez-Saona, C. (2019). Methyl salicylate increases attraction and function of beneficial arthropods in cranberries. Insects 10:423. doi: 10.3390/insects10120423
Sarkar, N., Mukherjee, A., and Barik, A. (2015). Attraction of Epilachna dodecastigma (Coleoptera: Coccinellidae) to Momordica charantia (Cucurbitaceae) leaf volatiles. Can. Entomol. 147, 169–180. doi: 10.4039/tce.2014.37
Scala, A., Allmann, S., Mirabella, R., Haring, M. A., and Schuurink, R. C. (2013). Green leaf volatiles: A plant’s multifunctional weapon against herbivores and pathogens. Int. J. Mol. Sci. 14, 17781–17811. doi: 10.3390/ijms140917781
Shao, X. L., Cheng, K., Wang, Z. W., Zhang, Q., and Yang, X. T. (2021). Use of odor by host finding insects: The role of real time odor environment and odor mixing degree. Chemoecology 3, 1–10. doi: 10.1007/s00049-021-00342-8
Tamiru, A., and Khan, Z. R. (2017). Volatile semiochemical mediated plant defense in cereals: A novel strategy for crop protection. Agronomy 7:58. doi: 10.3390/agronomy7030058
Unsicker, S. B., Kunert, G., and Gershenzon, J. (2009). Protective perfumes: The role of vegetative volatiles in plant defense against herbivores. Curr. Opin. Plant Biol. 12, 479–485. doi: 10.1016/j.pbi.2009.04.001
Visser, T., and Van der Maas, J. H. (1978). Systematic interpretation of Raman spectra of organic compounds. II-Ethers. J. Raman Spectrosc. 6, 114–116. doi: 10.1002/jrs.1250060303
von Mérey, G. M., Veyrat, N., D’Alessandro, M., and Turlings, T. C. J. (2013). Herbivore-induced maize leaf volatiles affect attraction and feeding behavior of Spodoptera littoralis caterpillars. Front. Plant Sci. 4:209. doi: 10.3389/fpls.2013.00209
Wang, X., Wang, S., Yi, J. K., Li, Y. S., Liu, J. N., Wang, J., et al. (2020). Three host plant volatiles, hexanal, lauric acid, and tetradecane, are detected by an antenna-biased expressed odorant receptor 27 in the dark black chafer Holotrichia parallela. J. Agric. Food Chem. 68, 7316–7323. doi: 10.1021/acs.jafc.0c00333
Xu, H., and Turlings, T. C. J. (2018). Plant volatiles as mate-finding cures for insects. Trends Plant Sci. 3, 100–111. doi: 10.1016/j.tplants.2017.11.004
Yang, Q., Wang, S. H., and Chen, H. Q. (2017). Evaluation of methylations and external/internal standard quantification of lipids using gas chromatography-mass spectrometry. Anal. Methods 9, 419–426. doi: 10.1039/C6AY02701F
Keywords: Chinese licorice, leaf beetle, semiochemicals, HIPVs, VOCs
Citation: Chen H-h, Zhang R, Tan S-q, Wang Y, Liu X-l and Shi W-p (2023) Components and composition of active volatiles attract on Diorhabda tarsalis (Coleoptera: Chrysomelidae) from Glycyrrhiza uralensis (Rosales: Leguminoseae). Front. Ecol. Evol. 10:1080208. doi: 10.3389/fevo.2022.1080208
Received: 26 October 2022; Accepted: 28 December 2022;
Published: 12 January 2023.
Edited by:
Michael Wink, Heidelberg University, GermanyReviewed by:
Habib Ali, Khwaja Fareed University of Engineering and Information Technology (KFUEIT), PakistanXiaoling Sun, Tea Research Institute (CAAS), China
Copyright © 2023 Chen, Zhang, Tan, Wang, Liu and Shi. This is an open-access article distributed under the terms of the Creative Commons Attribution License (CC BY). The use, distribution or reproduction in other forums is permitted, provided the original author(s) and the copyright owner(s) are credited and that the original publication in this journal is cited, in accordance with accepted academic practice. No use, distribution or reproduction is permitted which does not comply with these terms.
*Correspondence: Wang-peng Shi, d3BzaGlAY2F1LmVkdS5jbg==