- Jiangsu Key Laboratory for Biodiversity and Biotechnology, School of Biological Sciences, Nanjing Normal University, Nanjing, China
Herbicide pollution is persistent, which not only has a negative impact on individual organisms, but also may alter population dynamics and stability of interspecific relationships. Cladocerans, an important part of zooplankton, are often simultaneously exposed to environmental pollutants and predation risk in the aquatic environment. To evaluate the combined effects of atrazine and fish predation risk on the population traits of cladocerans, we exposed Daphnia pulex to different concentrations of atrazine (0, 0.05, 0.10, and 1.0 mg L−1) with or without fish (Rhodeus ocellatus) kairomone, recorded the key population traits, and fitted Gaussian model to population dynamics. Results showed that fish kairomone increased the population density at the end of the experiment and resting eggs production, and tended to decrease the total biomass and the average dry weight per individual of D. pulex. Atrazine reduced the total biomass, the average dry weight per individual, and resting eggs production of D. pulex populations. Atrazine also decreased the population density at the end of the experiment of D. pulex in fish kairomone treatment, and attenuated the promoting effect of fish kairomone on resting eggs production and the reduction of the total biomass. The findings highlighted the importance of considering the combined impact of environmental pollutants and predation risks on zooplankton populations.
1. Introduction
Atrazine has been widely used around the world as an herbicide to eliminate field weeds. As atrazine is soluble in water and can persist in the environment (Pathak and Dikshit, 2011), the residual atrazine in farmland soil will enter the water body through surface runoff (Jablonowski et al., 2011; de Albuquerque et al., 2020), which causes atrazine concentration in the watersheds all over the world ranging from 5.0 × 10−6 to 0.227 mg L−1 (Graymore et al., 2001; Benotti et al., 2009). Atrazine is a common endocrine disrupting chemical (Benotti et al., 2009; Sanchez et al., 2020), and can adversely affect the development, behavior, and reproduction of a variety of freshwater organisms by disrupting the physiological activities and endocrine systems (Russo and Lagadic, 2000; Hanazato, 2001; Greulich and Pflugmacher, 2003; Hayes et al., 2003).
Atrazine also interferes with the signaling of intraspecific and interspecific relationships in aquatic ecosystem. For example, atrazine makes tadpoles (Osteopilus septentrionalis) unable to sense the chemical signals of their predators, dragonfly larvae, thereby increasing the probability of encounters with predators (Ehrsam et al., 2016). After exposure to atrazine for 96 h, the ability of male rusty crayfish (Orconectes rusticus) to distinguish the source of the odor of female was weakened or even completely lost (Belanger et al., 2017). For freshwater organisms, chemical signals play a crucial role in maintaining a variety of intra-and inter-species relationships (Chivers and Smith, 1998; Kats and Dill, 1998; Rohr et al., 2005). As one of the important interspecific relationships in nature, the predation relationship plays an important role in maintaining the energy flow in the ecosystem (Dawkins and Krebs, 1979). In the long-term “arms race” coevolution process, some prey species have evolved the ability to resist predation by altering their phenotypes or life history strategies (inducible defenses) after detecting predator kairomones (predator-released chemicals; Harvell, 1990; Boersma et al., 1998; Agrawal, 2001; Kessler and Baldwin, 2001; Carter et al., 2017), which is important for prey to maintain population stability (Wissinger et al., 2010; Peacor et al., 2012).
Cladocerans are an important component of zooplankton as they can consume algae and are also an important food source for some fish and invertebrates (Dettmers and Stein, 1992; Lyu et al., 2019). Cladocerans are highly sensitive to changes in the water environment (Dodson and Hanazato, 1995; Yang et al., 2011), so they have been widely used as model organisms to study the effects of pollutants (Lyu et al., 2013, 2016). Previous studies have reported that atrazine increases the proportion of male offspring, reduces fecundity, and affects the expression of genes related to reproduction and energy metabolism in cladocerans (Dodson et al., 2000; Klementova et al., 2019). Atrazine, as an endocrine disrupting chemical (Mizota and Ueda, 2006; Benotti et al., 2009), may interfere with responses of organisms to predation risk and further affect prey populations. Therefore, it is necessary to study the impact of atrazine on prey population dynamics under predation risk to comprehensively assess the impact of pollutants on the stability of interspecies relationships in ecosystems (Beckerman et al., 2010).
Based on the above analysis, it is necessary to comprehensively evaluate the effects of atrazine on cladocerans under presence or absence of predation risk at the population level. In this study, we hypothesized that: (1) atrazine will reduce the population density and total biomass of cladocerans; (2) atrazine will reduce the production of resting eggs; (3) atrazine will interfere with the population dynamics under predation risk. To test these hypotheses, we exposed Daphnia pulex to different field-relevant atrazine concentrations (0, 0.05, 0.10, and 1.0 mg L−1) in the presence and absence of fish kairomone, and recorded the number of individuals, the number of resting eggs, and the total biomass.
2. Materials and methods
2.1. Daphnia and algae culture
Before the experiment, D. pulex was cultured in the COMBO media (Kilham et al., 1998) under a light intensity of 10 μmol photons m−2 s−1 with a light–dark period of 14: 10 h at 25°C. The COMBO medium was changed every day, and 1.50 mg C L−1 of green alga Chlorella pyrenoidosa (FACHB11) as food for D. pulex was provided daily. The green alga C. pyrenoidosa was cultured in BG-11 medium, with a condition at 25°C, 20 μmol photons m−2 s−1 light intensity, and 14: 10 light–dark period. When cell abundance reached exponential phase, algae were collected by centrifugation for 15 min at 4200 g and resuspended in deionized water. The concentrated algae were stored at 4°C as food for D. pulex.
2.2. Fish kairomone preparation and atrazine dissolution
The kairomone of the predator a cyprinid fish Rhodeus ocellatus was prepared according to the method described in the previous study (Gu et al., 2020a). In brief, two fish (3–5 cm in length) were fed with 200 individuals of D. pulex for 6 h in a tank, and then transferred to 1 L of COMBO medium for 18 h without any food supply for them to release kairomone. The COMBO medium containing kairomone was collected and filtered through a 0.22 μm glass fiber filter (Millipore). This stock concentration was diluted 20 times (i.e., 1 fish per 10 L) when used in the experiment (Gu et al., 2020a).
Atrazine (CAS No. 1912-24-9, analytical grade, purity ≥99.5%) was purchased from Aladdin Industrial Corporation (Shanghai, China). Atrazine stock solution with a concentration of 1.0 mg mL−1 (with dimethyl sulfoxide as solvent) was prepared 1 day before the experiment. Specifically, 50 mg of atrazine were weighed and added to 50 ml of DMSO solution. The solution was stirred with a magnetic stirrer for 1 h to fully dissolve atrazine and mixed thoroughly before each experiment. Previous studies have found that DMSO at a concentration of 1‰ does not have a negative effect on cladocerans (David et al., 2012). Especially, our experiment also proved that adding DMSO at a concentration of 1‰ had no effect on the population dynamics and resting eggs production under presence of fish kairomone of D. pulex (for detail see Table 1).
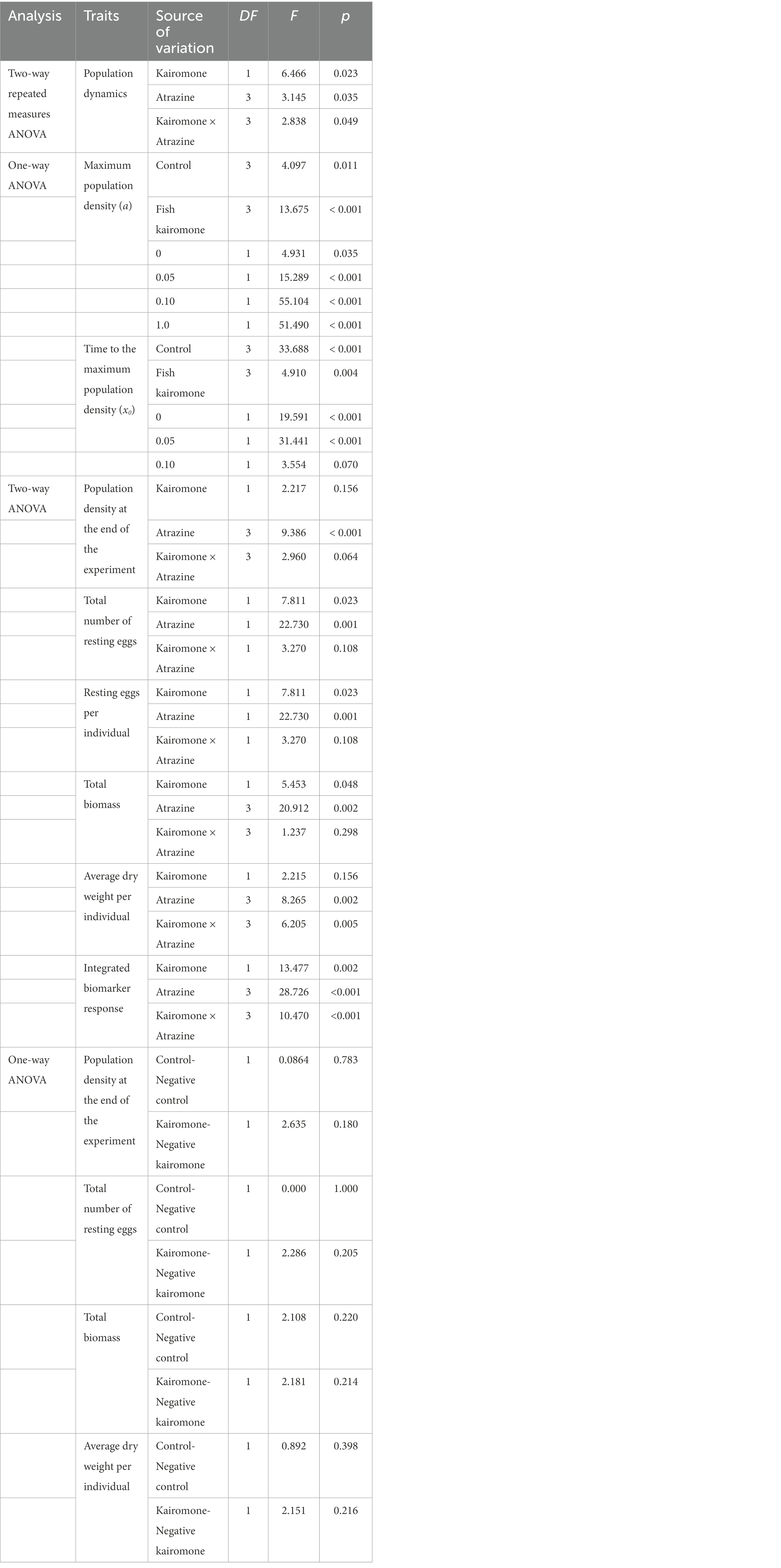
Table 1. The results of two-way repeated measures ANOVA on population size, the two-way ANOVA on population traits, the one-way ANOVA on parameters of the three-parameter Gaussian model on the different conditions, and the one-way ANOVA on different DMSO treatments.
2.3. Experimental design
The experimental individuals were neonates (< 12 h) collected from the third brood of 10 maternal D. pulex, which synchronously developed. These neonates were exposed to eight test conditions, that is, presence or absence of fish kairomone (Control and Fish kairomone treatment) × four atrazine concentrations (0, 0.05, 0.10, and 1.0 mg L−1). Each treatment had three replicates, and five neonates were cultured in a beaker containing 250 ml test medium as a replicate. The experiment was performed under the same conditions as described in “Daphnia and algae culture.” The experimental medium of different treatments was refreshed and C. pyrenoidosa suspension was added to obtain a concentration of 1.50 mg C L−1 daily.
After 4 days, all individuals in all treatments were sexually mature. During the population growth period (from the fifth day to the end of the experiment), we counted the number of individuals and resting eggs in each beaker while transferring the individuals to fresh culture medium daily. The experiment ended at 15 days, when the population density of the control treatments reached a relatively stable level, and the D. pulex in each treatment were collected and then washed with deionized water. These D. pulex were dried in an oven (50°C) for 36 h, and then the total dry weights (i.e., total biomass) were weighed using an electronic balance (AUW120D, Shimadzu, Japan; Accuracy: 0.01 mg). The average dry weight per individual was calculated based on total biomass/number of individuals.
2.4. Statistical analysis
The three-parameter Gaussian model was used for fitting the population dynamics during the experiment, where y is the population size, x is experiment time, x0 represents the time to the maximum population density, a indicates the maximum population density, b is the form parameter determining shape of curve. At the end of the experiment, the total biomass of the population was fitted using a three-parameter exponential decay function , where r is one of the total biomass, A is atrazine concentration, r0 is the predicted asymptotes of the exponential decline (minimum value) function when A approaches infinity, k describes the initial rate of change in the response for the decline function, and m is a constant, respectively. The correlation between resting eggs production and population density at the end of the experiment were calculated using the Pearson correlation coefficient. The response score (S) and integrated biomarker response (IBR) were calculated based on four population traits (i.e., population density at the end of the experiment, total number of resting eggs, total biomass, and average dry weight per individual) at eight different treatments according to the formula in the study of Beliaeff and Burgeot (2002).
Two-way repeated measures ANOVA followed by the Tukey test was applied to evaluate the effects of different atrazine concentrations and fish kairomone treatment on the population size on different days. Two-way ANOVA followed by the Tukey test was applied to evaluate the effects of different atrazine concentrations and fish kairomone treatment on the population density at the end of the experiment, total number of resting eggs, total biomass, and average dry weight per individual. One-way ANOVA followed by the Tukey test was applied to evaluate the effects of different treatments on the maximum population density (a) and the time to the maximum population density (x0). Differences were deemed significant with p < 0.05. All statistical analysis and figures were performed using SigmaPlot 14.0.
3. Results
3.1. Population dynamics
The three-parameter Gaussian model could well fit the early population dynamics (Figures 1A,B). The two-way repeated measures ANOVA showed that population dynamics during the experiment were significantly affected by fish kairomone and atrazine concentrations, and there was a significant interaction between the two factors (Table 1). Specifically, two high concentrations of atrazine (0.10 and 1.0 mg L−1) had a significantly synergistic effect on population dynamics (maximum population density and the time to the maximum population density) in the presence of fish kairomone, but did not have a significant effect on population dynamics in the control (all p > 0.506). Additionally, there were significant differences in population dynamics between the control and fish kairomone at high concentrations of atrazine (0.10 mg L−1: p = 0.005; 1.0 mg L−1: p = 0.005), while it showed no significant differences at low concentrations of atrazine (0 mg L−1: p = 0.555; 0.05 mg L−1: p = 0.243).
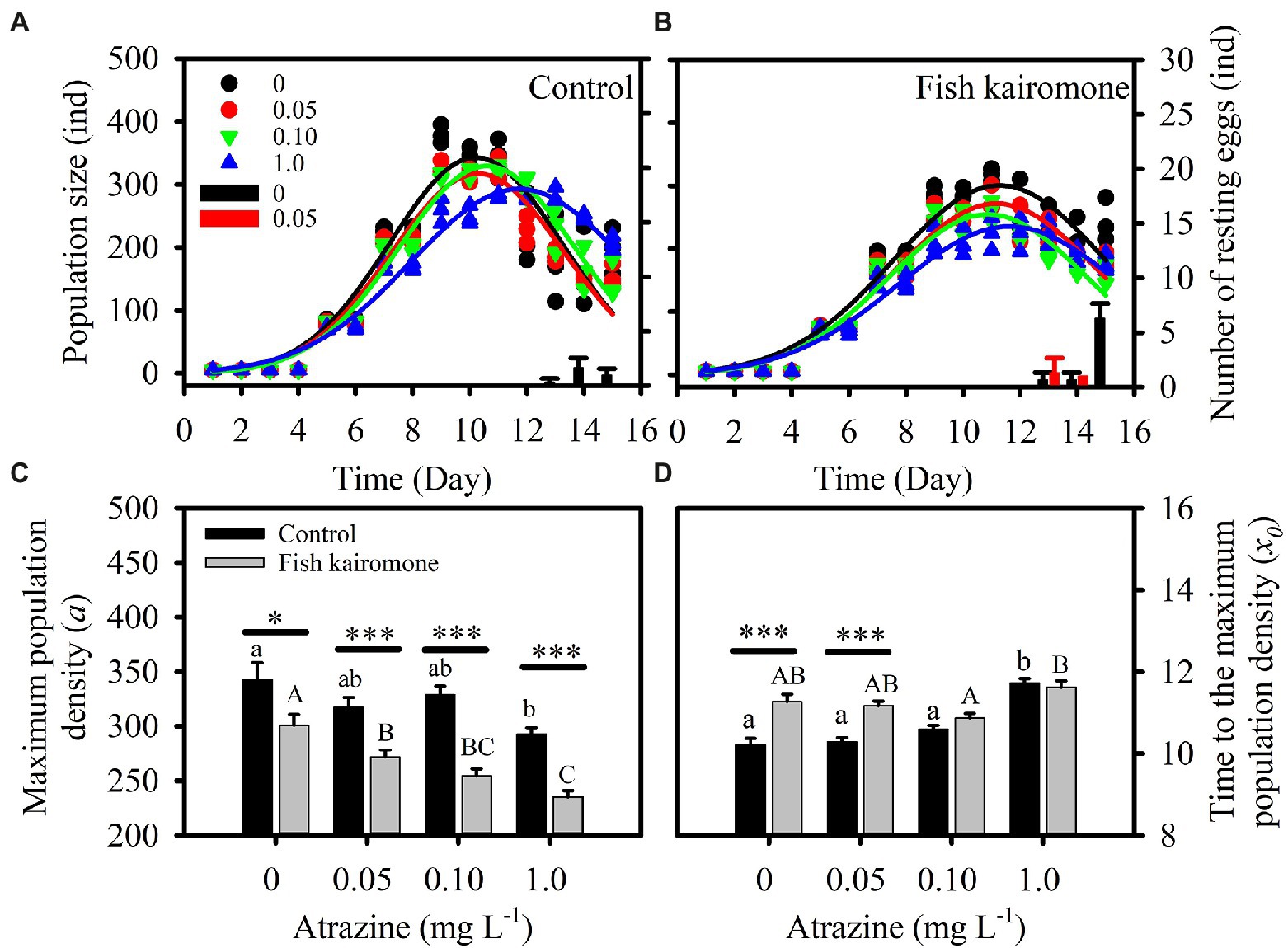
Figure 1. Population dynamics and resting eggs production of D. pulex (A,B). The solid curves in the figure are the population dynamics fitted by a three-parameter Gaussian model, i.e., , and the columns represent the daily production of resting eggs. D. pulex began to produce resting eggs from day 13. Resting eggs are produced only in the two lowest atrazine concentrations, and the black and red bars represent daily resting eggs production at 0 and 0.05 mg L−1 atrazine concentrations, respectively. (C,D) The population parameters of D. pulex in different treatments. Asterisks indicate significant differences between the control and kairomone treatment (*p < 0.05, ***p < 0.001), and lowercase and uppercase letters indicate differences among the control and fish kairomone treatment, respectively. The error bars indicate standard error.
Both atrazine and fish kairomone decreased the maximum population density (a) and increased the time to the maximum population density (x0; Figures 1C,D; Table 1). In addition, atrazine adversely affected population traits under fish kairomone treatment, resulting in a reduction in the maximum population density (a; Figure 1C; Table 1). Specially, fish kairomone increased population density at the end of the experiment, but the high concentration of atrazine tended to reverse this effect (Figure 2A; Table 1). Compared with the control, the maximum population density of fish kairomone treatment significantly decreased by 12.3%, while the population density at the end of the experiment significantly increased by 24.0%. Compared with 0 mg L−1 atrazine concentrations, the maximum population density of 1.0 mg L−1 atrazine concentrations significantly decreased by 14.5%, and the time to the maximum population density significantly increased by 14.8%.
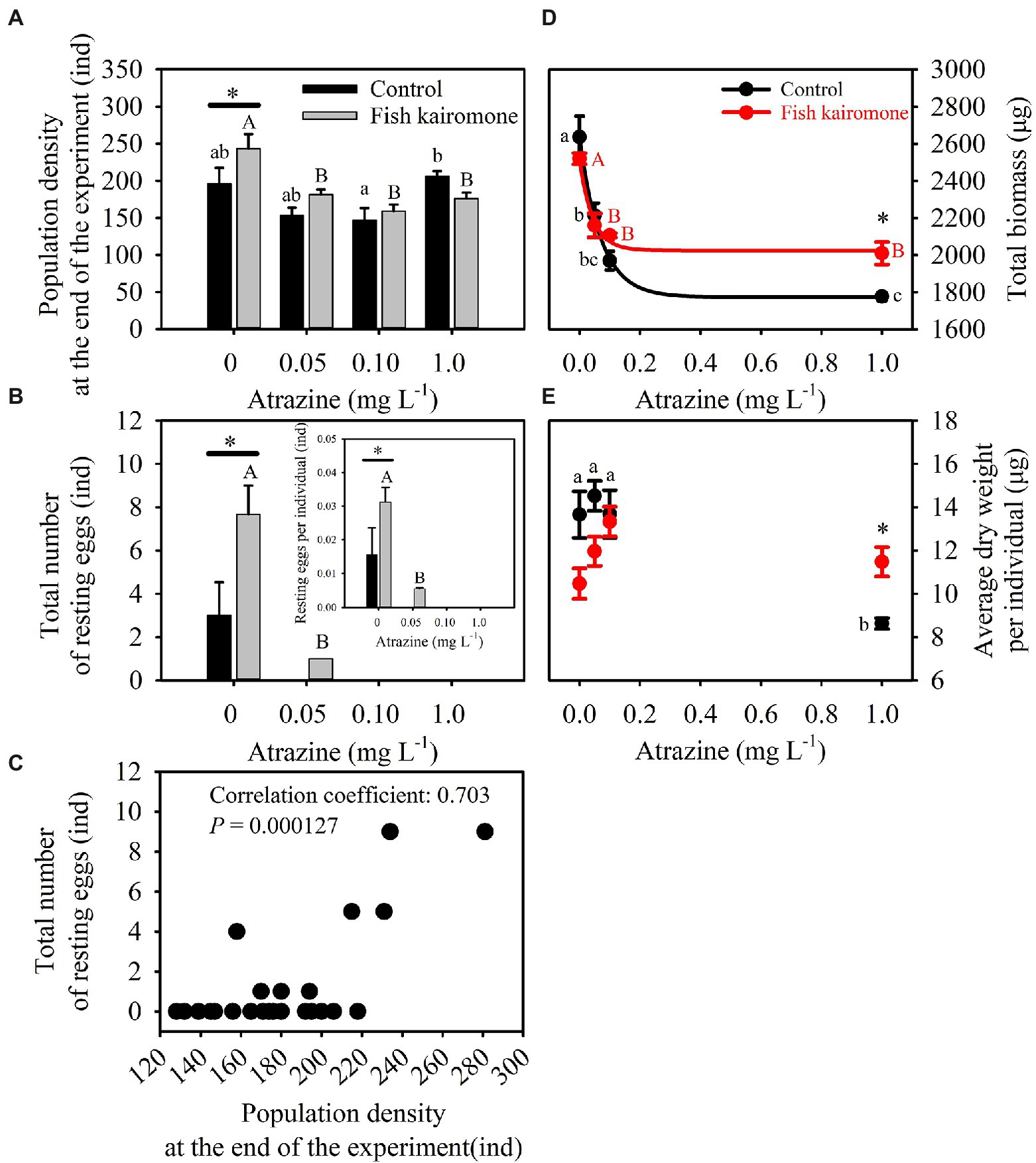
Figure 2. Population traits of D. pulex under different treatments. The correlation between resting eggs production and population density at the end of the experiment were calculated using the Pearson correlation coefficient. The total biomass at different treatments was fitted using the three-parameter exponential decay equation . Asterisks indicate significant differences between control and kairomone treatments (*p < 0.05), and lowercase and uppercase letters indicate differences among the control and fish kairomone treatment, respectively. The error bars indicate standard error. The results of two-way ANOVA are shown in Table 1.
3.2. Resting eggs production
From the 13th day of the experiment, populations of D. pulex began to produce resting eggs only under the low concentrations of atrazine treatment (0 and 0.05 mg L−1; Figures 1A,B, 2B). The total number of resting eggs and the resting eggs per individual was significantly affected by atrazine concentrations and fish kairomone treatments (Table 1). Fish kairomone significantly promoted the production of resting eggs and the resting eggs per individual (Figures 1A,B, 2B; Table 1; p = 0.023), whereas the presence of atrazine significantly inhibited the production of resting eggs and the resting eggs per individual (Figures 1A,B, 2B; Table 1; p = 0.001). In the control, resting eggs were produced only with no atrazine, but in the fish kairomone treatment, resting eggs were produced at 0 and 0.05 mg L−1. The results showed that resting eggs production and population density at the end of the experiment tend to increase together (Figure 2C; Correlation coefficient: 0.703; p = 0.0001).
3.3. Total biomass and dry weight
Fish kairomone and atrazine had a significant interaction on the total biomass (total dry weight) and the average dry weight per individual of the population (Table 1; Total biomass: p = 0.036; Average dry weight per individual: p = 0.005). The total biomass of D. pulex in both the control and fish kairomone treatment decreased exponentially with increasing atrazine concentration (Figure 2D). Specifically, the total biomass of D. pulex in the fish kairomone treatment tended to be lower than that of the control at low concentrations of atrazine (0 mg L−1: −4.42%; 0.05 mg L−1: −2.41%), whereas the results were reversed at high concentrations (0.10 mg L−1: 6.97%; 1.0 mg L−1: 13.13%). Meanwhile, in the presence of fish kairomone, the asymptotic total biomass (r0) increased significantly compared to that in the absence of fish kairomone (p < 0.001), and the exponential decline rate (k) in total biomass did not change significantly (p = 0.188). The results showed that fish kairomone could attenuate the reduction effect of atrazine on the total biomass of the population. In the absence of fish kairomone, the average dry weight per individual significantly decreased at high concentrations of atrazine, but atrazine did not cause a significant difference in the fish kairomone treatments (Figure 2D), which indicated that fish kairomone could attenuate the reduction effect of high concentrations of atrazine on the average dry weight per individual. Furthermore, fish kairomone tended to reduce mean individual dry weight of D. pulex (p = 0.068), but atrazine caused this effect to be reversed (Figure 2E).
3.4. Integrated biomarker response analysis
On the star plots, the variable sizes and geometric forms of the area polygons represented the response score, which reflected the different reaction intensities of the indicators at different treatments (Figures 3A,B). The presence of atrazine significantly reduced the integrated biomarker response (IBR) of population traits under fish kairomone treatment (Figure 3C). At low concentrations of atrazine (0 and 0.05 mg L−1), the IBR value of D. pulex in the fish kairomone treatment was higher than that in the control, whereas the result was reversed at high concentrations (1.0 mg L−1; Figure 3C).
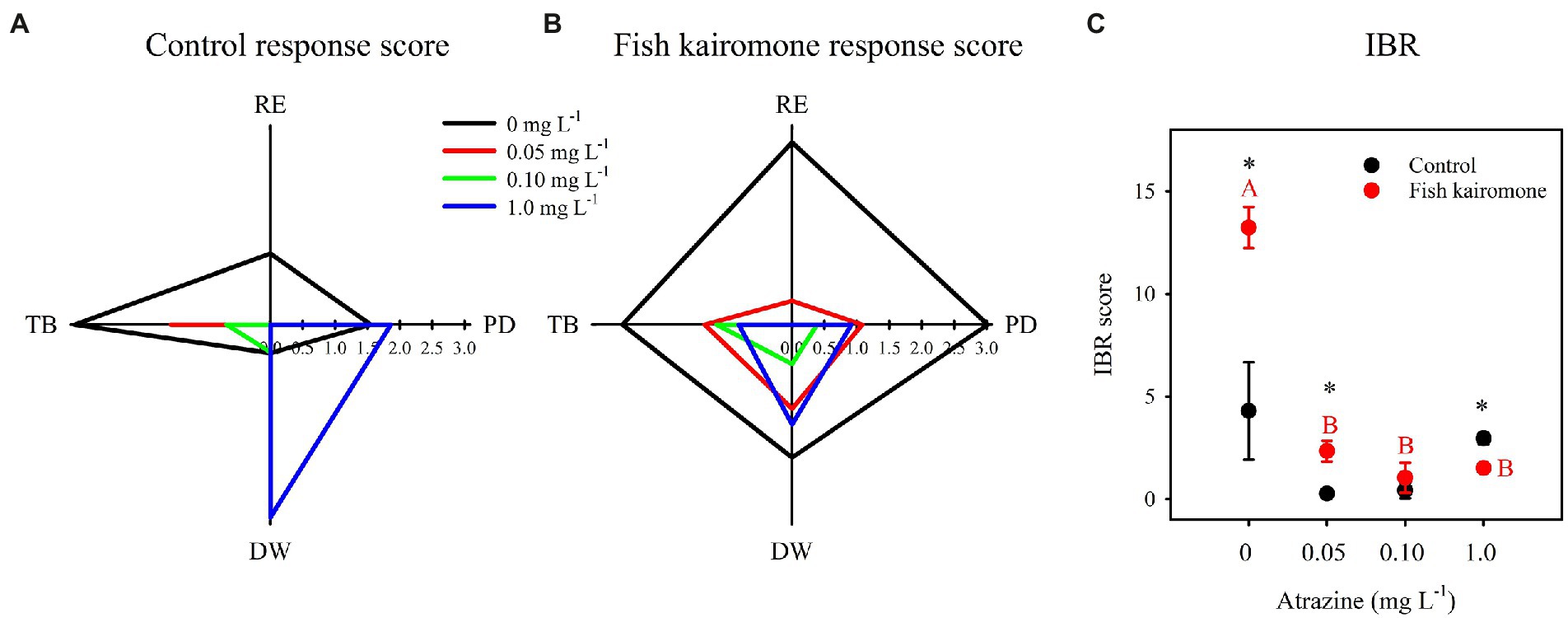
Figure 3. The response score (S) and the integrated biomarker response (IBR) of population traits in different treatments. The IBR consists of four indicators: population density at the end of the experiment (PD), total number of resting eggs (RE), total biomass (TB), and average dry weight per individual (DW). Asterisks indicate significant differences between control and kairomone treatments (*p < 0.05), and uppercase letters indicate differences among the fish kairomone treatment. The error bars indicate standard error. The results of two-way ANOVA are shown in Table 1.
4. Discussion
Predator-specific infochemicals causing individual defensive responses in prey can directly affect the population stability of prey (Stibor, 1992; Benard, 2004; Lind and Cresswell, 2005; Zhu et al., 2021). The impact of environmental changes on the individual traits of organisms can be transmitted to the population level, and have a comprehensive impact on zooplankton (Ieromina et al., 2014; Adamczuk, 2020; Lyu et al., 2021; Huang et al., 2022b). Our study showed that increasing atrazine concentration had negative impacts on the population traits and resting eggs production of D. pulex. Specifically, atrazine reduced the total biomass, the average dry weight per individual, and resting eggs production of D. pulex population. Fish kairomone increased the population density at the end of the experiment and resting eggs production of D. pulex population and tend to decrease the total biomass and the average dry weight per individual. Importantly, the total biomass and the average dry weight per individual of D. pulex in fish kairomone treatment were higher than those in the control at high concentrations of atrazine. At low concentrations of atrazine, the IBR value of the fish kairomone was higher than that of the control, whereas the opposite was true at high concentration, which indicated that atrazine reversed the direction of action of fish kairomone on population traits. These core findings confirmed our initial hypothesis.
Confronting predation risk and pollutant exposure simultaneously, organisms face a physiological decision that may involve a trade-off between resource allocation, to avoid being killed by predators and being damaged by toxicants. For instance, the low concentrations of chromium and endosulfan have negative effects on the behavioral responses of microcrustaceans (the copepod Notodiaptomus conifer and the cladoceran Ceriodaphnia dubia) to fish chemical cues (Florencia Gutierrez et al., 2012). Our results suggested that fish kairomone caused a decrease in the maximum population density and an increase in the time to the maximum population density, which may be related to the response in the individual defense traits of D. pulex to the risk of fish predation, that is, a decrease in the offspring number (Lu et al., 2021). However, fish kairomone increased population density of D. pulex at the end of the experiment, which may be associated with reducing body size under fish kairomone treatment (Lu et al., 2021; Qin et al., 2022). Smaller body size also means that individuals need less food to maintain normal life activities of the body (Gu et al., 2020a). Therefore, under the condition of limited space and resources, the population of D. pulex can maintain a higher density due to enhanced fecundity under fish kairomone treatment (Gliwicz et al., 2012; Langer et al., 2019). Previous studies suggested that atrazine affects the reproduction of aquatic animals and plants, interfering with the structure of aquatic community (Graymore et al., 2001). Atrazine adversely affected populations in the presence of predation risk, resulting in the decreased maximum population density and the population density at the end of the experiment, which was caused by the harm of atrazine to the reproduction of cladocerans (Schober and Lampert, 1977; Dodson et al., 2000; Palma et al., 2009; Religia et al., 2019). Previous studies have reported that environmental stress causes decreased population size of cladocerans, mainly due to the reduction in their reproduction (Huang et al., 2022a,b). Although the experiment may have a certain limitation as it was ended when the population of the control was relatively stable, the potential influence of fish kairomone and atrazine on population density could also be detected from the 15 days population dynamics. The impact of atrazine on cladocerans population will inevitably interfere with the biomass of other trophic organisms, especially the adjacent trophic level organisms of cladocerans, such as their prey algae and their predator fish.
Cladocerans are able to adjust their reproductive strategy based on current environmental signals, shifting from current reproduction (parthenogenesis) to future reproduction (sexual reproduction), and retain resting eggs to wait for the arrival of suitable environmental conditions (Haltiner et al., 2020). Also, resting eggs can disperse over time, so more resting eggs can help cladocerans have a higher chance to disperse to new habitats (Van de Meutter et al., 2008; Smith et al., 2009). Many signals can trigger the production of resting eggs, such as food restriction, photoperiod changes, temperature changes, predator signals, nutrient depletion, or crowding signals (Folt and Goldman, 1981; Booksmythe et al., 2018; Haltiner et al., 2020). In this study, there was a trade-off between the production of resting eggs and population growth. The emergence of resting eggs in the population is the response of cladocerans to changes in their living environment (Leblanc and Medlock, 2015; Zhou et al., 2020). Predator kairomone promotes the production of resting eggs, which is thought to be a positive response of cladocerans to the predation risk (Pijanowska and Stolpe, 1996); however, the latest research showed that predator kairomone triggers sexual reproduction of Daphnia population essentially via crowding caused by increasing population density (Zhou et al., 2022). Atrazine inhibited the resting eggs production, which may be the result of low crowding effect (population density reduction) or the direct effect of atrazine itself, but this needs further research. Our study found that the presence of atrazine inhibited the production of resting eggs even under fish kairomone treatments, which obviously affected the anti-predation defenses of cladocerans and had a negative impact on the future of the population, as lower number of resting eggs increases the probability of extinction of cladocerans populations in specific waters, and also reduces the dispersal of cladocerans populations.
Smaller individuals are an adaptive response of cladocerans to predation risk, which means they contain less energy. This way not only reduce fish selection rates but also makes them not be easily detected by fish (Stibor, 1992; Huelsmann et al., 2011). However, the presence of atrazine caused a significant increase in the average dry weight per individual under kairomone treatment compared with the without kairomone treatment, which means that the individual is larger and/or has more energy. Larger individuals are more likely to be spotted by predators (Barnhisel, 1991; Caramujo and Boavida, 2000), which means that atrazine can adversely affect individual survival and population stability under predation risk. Our results showed that the high concentration of atrazine caused the decrease of individual dry weight of D. pulex, but as the concentration selected in this experiment was limited, thus the critical concentration of atrazine that causes the reduction of individual dry weight cannot be obtained. Previous studies concluded that fish predation kairomone attenuates the adverse effects of environmental stressors on the growth and reproduction of cladocerans (Robison et al., 2018; Gu et al., 2020b; Huang et al., 2021), and the results of our experiment demonstrated for the first time that the fish predation kairomone attenuated the negative effects of atrazine on several population traits. These results indicated that fish kairomone can alleviate the stress ability of cladocerans. As the components of fish kairomone are complex and unclear, its alleviative effect on atrazine stress needs further study. In addition, the decrease in the total biomass and the population density at the end of the experiment of cladocerans caused by pesticide pollution may also lead to the increase of harmful phytoplankton in the water, and thus may further lead to the deterioration of water quality (Urrutia-Cordero et al., 2016; Ger et al., 2019).
Therefore, from the perspective of the relationships between predator and prey populations, the changes in population dynamics caused by changes in individual traits are the adaptive responses of prey to predation risks (Petrusek et al., 2009; Reger et al., 2018). Pollutants can change the direction and intensity of the response of population dynamics to predation risk signals, which will inevitably have adverse effects on the adaptive response strategies of organisms.
5. Conclusion
Our study demonstrated that fish kairomone increased the population density at the end of the experiment and resting eggs production and tended to decrease the total biomass and the average dry weight per individual of D. pulex. Atrazine reduced the total biomass, the average dry weight per individual, and resting eggs production of D. pulex population. Atrazine reduced the population density at the end of the experiment of D. pulex in fish kairomone treatment, and attenuated the promoting effect of fish kairomone on resting eggs production and the reduction of the total biomass. This study facilitates the understanding of the impact of environmental pollutants in aquatic ecosystems on the population dynamics of organisms under predation risk.
Data availability statement
The raw data supporting the conclusions of this article will be made available by the authors, without undue reservation.
Ethics statement
The animal study was reviewed and approved by the Institutional Animal Care and Use Committees (IACUC) of the Nanjing Normal University, Nanjing, China (Research permit number: SYXK2015-0028).
Author contributions
SQ: conceptualization, data curation, formal analysis, writing – original draft, and writing – review and editing. TX: validation and formal analysis. GL: visualization and writing – review and editing. LG: conceptualization, formal analysis, and writing – review and editing. YS: formal analysis and writing – review and editing. ZY: conceptualization, formal analysis, funding acquisition, supervision, and writing – review and editing. All authors contributed to the article and approved the submitted version.
Funding
The study was supported by National Natural Science Foundation of China (31730105) and the Priority Academic Program Development of Jiangsu Higher Education Institutions of China.
Conflict of interest
The authors declare that they have no known competing financial interests or personal relationships that could have appeared to influence the work reported in this paper.
Publisher’s note
All claims expressed in this article are solely those of the authors and do not necessarily represent those of their affiliated organizations, or those of the publisher, the editors and the reviewers. Any product that may be evaluated in this article, or claim that may be made by its manufacturer, is not guaranteed or endorsed by the publisher.
References
Adamczuk, M. (2020). Population dynamics and life history traits of Daphnia magna across thermal regimes of environments. Sci. Total Environ. 723:137963. doi: 10.1016/j.scitotenv.2020.137963
Agrawal, A. A. (2001). Phenotypic plasticity in the interactions and evolution of species. Science 294, 321–326. doi: 10.1126/science.1060701
Barnhisel, D. R. (1991). Zooplankton spine induces aversion in small fish predators. Oecologia 88, 444–450. doi: 10.1007/BF00317591
Beckerman, A. P., Petchey, O. L., and Morin, P. J. (2010). Adaptive foragers and community ecology: linking individuals to communities and ecosystems. Funct. Ecol. 24, 1–6. doi: 10.1111/j.1365-2435.2009.01673.x
Belanger, R. M., Evans, K. R., Abraham, N. K., and Barawi, K. M. (2017). Diminished conspecific odor recognition in the rusty crayfish (Orconectes rusticus) following a 96-h exposure to atrazine. B. Environ. Contam. Tox. 99, 555–560. doi: 10.1007/s00128-017-2178-3
Beliaeff, B., and Burgeot, T. (2002). Integrated biomarker response: a useful tool for ecological risk assessment. Environ. Toxicol. Chem. 21, 1316–1322. doi: 10.1002/etc.5620210629
Benard, M. F. (2004). Predator-induced phenotypic plasticity in organisms with complex life histories. Annu. Rev. Ecol. Evol. Sci. 35, 651–673. doi: 10.1146/annurev.ecolsys.35.021004.112426
Benotti, M. J., Trenholm, R. A., Vanderford, B. J., Holady, J. C., Stanford, B. D., and Snyder, S. A. (2009). Pharmaceuticals and endocrine disrupting compounds in US drinking water. Environ. Sci. Technol. 43, 597–603. doi: 10.1021/es801845a
Boersma, M., Spaak, P., and De Meester, L. (1998). Predator-mediated plasticity in morphology, life history, and behavior of Daphnia: the uncoupling of responses. Am. Nat. 152, 237–248. doi: 10.1086/286164
Booksmythe, I., Gerber, N., Ebert, D., and Kokko, H. (2018). Daphnia females adjust sex allocation in response to current sex ratio and density. Ecol. Lett. 21, 629–637. doi: 10.1111/ele.12929
Caramujo, M. J., and Boavida, M. J. (2000). Induction and costs of tail spine elongation in Daphnia hyalina × galeata: reduction of susceptibility to copepod predation. Freshw. Biol. 45, 413–423. doi: 10.1046/j.1365-2427.2000.00642.x
Carter, M. J., Lind, M. I., Dennis, S. R., Hentley, W., and Beckerman, A. P. (2017). Evolution of a predator-induced, nonlinear reaction norm. Proc. Roy. Soc. B-Biol. Sci. 284:20170859. doi: 10.1098/rspb.2017.0859
Chivers, D. P., and Smith, R. J. F. (1998). Chemical alarm signalling in aquatic predator-prey systems: a review and prospectus. Écoscience 5, 338–352. doi: 10.1080/11956860.1998.11682471
David, R. M., Jones, H. S., Panter, G. H., Winter, M. J., Hutchinson, T. H., and Chipman, J. K. (2012). Interference with xenobiotic metabolic activity by the commonly used vehicle solvents dimethylsulfoxide and methanol in zebrafish (Danio rerio) larvae but not Daphnia magna. Chemosphere 88, 912–917. doi: 10.1016/j.chemosphere.2012.03.018
Dawkins, R., and Krebs, J. R. (1979). Arms races between and within species. Proc. R. Soc. Ser. B-Bio. 205, 489–511. doi: 10.1098/rspb.1979.0081
de Albuquerque, F. P., de Oliveira, J. L., Moschini-Carlos, V., and Fraceto, L. F. (2020). An overview of the potential impacts of atrazine in aquatic environments: perspectives for tailored solutions based on nanotechnology. Sci. Total Environ. 700:134868. doi: 10.1016/j.scitotenv.2019.134868
Dettmers, J. M., and Stein, R. A. (1992). Food consumption by larval Gizzard Shad: zooplankton effects and implications for reservoir communities. T. Am. Fish. Soc. 121, 494–507. doi: 10.1577/1548-8659(1992)121<0494:FCBLGS>2.3.CO;2
Dodson, S. I., and Hanazato, T. (1995). Commentary on effects of anthropogenic and natural organic chemicals on development, swimming behavior, and reproduction of Daphnia, a key member of aquatic ecosystems. Environ. Health Perspect. 103, 7–11. doi: 10.2307/3432405
Dodson, S. I., Merritt, C. M., Shannahan, J. P., and Shults, C. M. (2000). Low exposure concentrations of atrazine increase male production in Daphnia pulicaria. Environ. Toxicol. Chem. 18, 1568–1573. doi: 10.1002/etc.5620180732
Ehrsam, M., Knutie, S. A., and Rohr, J. R. (2016). The herbicide atrazine induces hyperactivity and compromises tadpole detection of predator chemical cues. Environ. Toxicol. Chem. 35, 2239–2244. doi: 10.1002/etc.3377
Florencia Gutierrez, M., Cesar Paggi, J., and Maria Gagneten, A. (2012). Infodisruptions in predator-prey interactions: xenobiotics alter microcrustaceans responses to fish infochemicals. Ecotox. Environl. Safe. 81, 11–16. doi: 10.1016/j.biocontrol.2019.02.008
Folt, C., and Goldman, C. R. (1981). Allelopathy between zooplankton: a mechanism for interference competition. Science 213, 1133–1135. doi: 10.1126/science.213.4512.1133
Ger, K. A., Naus-Wiezer, S., De Meester, L., and Lurling, M. (2019). Zooplankton grazing selectivity regulates herbivory and dominance of toxic phytoplankton over multiple prey generations. Limnol. Oceanogr. 64, 1214–1227. doi: 10.1002/lno.11108
Gliwicz, Z. M., Maszczyk, P., and Uszko, W. (2012). Enhanced growth at low population density in Daphnia: the absence of crowding effects or relief from visual predation? Freshw. Biol. 57, 1166–1179. doi: 10.1111/j.1365-2427.2012.02783.x
Graymore, M., Stagnitti, F., and Allinson, G. (2001). Impacts of atrazine in aquatic ecosystems. Environ. Int. 26, 483–495. doi: 10.1016/S0160-4120(01)00031-9
Greulich, K., and Pflugmacher, S. (2003). Differences in susceptibility of various life stages of amphibians to pesticide exposure. Aquat. Toxicol. 65, 329–336. doi: 10.1016/S0166-445X(03)00153-X
Gu, L., Qin, S., Lu, N., Zhao, Y., Zhou, Q., Zhang, L., et al. (2020a). Daphnia mitsukuri traits responding to predation cues alter its population dynamics. Ecol. Indic. 117:106587. doi: 10.1016/j.ecolind.2020.106587
Gu, L., Qin, S., Zhu, S., Lu, N., Sun, Y., Zhang, L., et al. (2020b). Microcystis aeruginosa affects the inducible anti-predator responses of Ceriodaphnia cornuta. Environ. Pollut. 259:113952. doi: 10.1016/j.envpol.2020.113952
Haltiner, L., Hanggi, C., Spaak, P., and Dennis, S. R. (2020). Sex in crowded places: population density regulates reproductive strategy. Hydrobiologia 847, 1727–1738. doi: 10.1007/s10750-019-04143-7
Hanazato, T. (2001). Pesticide effects on freshwater zooplankton: an ecological perspective. Environ. Pollut. 112, 1–10. doi: 10.1016/S0269-7491(00)00110-X
Harvell, C. D. (1990). The ecology and evolution of inducible defenses. Q. Rev. Biol. 65, 323–340. doi: 10.1086/416841
Hayes, T., Haston, K., Tsui, M., Hoang, A., Haeffele, C., and Vonk, A. (2003). Atrazine-induced hermaphroditism at 0.1 ppb in American leopard frogs (Rana pipiens): laboratory and field evidence. Environ. Health Perspect. 111, 568–575. doi: 10.1289/ehp.5932
Huang, J., Li, Y., Sun, Y., Zhang, L., Lyu, K., and Yang, Z. (2022a). Size-specific sensitivity of cladocerans to freshwater salinization: evidences from the changes in life history and population dynamics. Environ. Pollut. 296:118770. doi: 10.1016/j.envpol.2021.118770
Huang, J., Wang, Z., Yu, B., Sun, Y., Gu, L., Zhang, L., et al. (2022b). Population changes of Daphnia caused by declined calcium concentration: evidences from population dynamics and sexual reproduction. Ecotox. Environl. Safe. 233:113352. doi: 10.1016/j.ecoenv.2022.113352
Huang, J., Xu, X., Li, D., Sun, Y., Gu, L., Zhang, L., et al. (2021). Decreased calcium concentration interferes with life history defense strategies of Ceriodaphnia cornuta in response to fish kairomone. Limnol. Oceanogr. 66, 3237–3252. doi: 10.1002/lno.11876
Huelsmann, S., Rinke, K., and Mooij, W. M. (2011). Size-selective predation and predator-induced life-history shifts alter the outcome of competition between planktonic grazers. Funct. Ecol. 25, 199–208. doi: 10.1111/j.1365-2435.2010.01768.x
Ieromina, O., Peijnenburg, W. J. G. M., de Snoo, G., Mueller, J., Knepper, T. P., and Vijver, M. G. (2014). Impact of imidacloprid on Daphnia magna under different food quality regimes. Environ. Toxicol. Chem. 33, 621–631. doi: 10.1002/etc.2472
Jablonowski, N. D., Schaeffer, A., and Burauel, P. (2011). Still present after all these years: persistence plus potential toxicity raise questions about the use of atrazine. Environ. Sci. Pollut. Res. 18, 328–331. doi: 10.1007/s11356-010-0431-y
Kats, L. B., and Dill, L. M. (1998). The scent of death: chemosensory assessment of predation risk by prey animals. Écoscience 5, 361–394. doi: 10.1080/11956860.1998.11682468
Kessler, A., and Baldwin, I. T. (2001). Defensive function of herbivore-induced plant volatile emissions in nature. Science 291, 2141–2144. doi: 10.1126/science.291.5511.2141
Kilham, S. S., Kreeger, D. A., Lynn, S. G., Goulden, C. E., and Herrera, L. (1998). COMBO: a defined freshwater culture medium for algae and zooplankton. Hydrobiologia 377, 147–159. doi: 10.1023/A:1003231628456
Klementova, S., Hornychova, L., Sorf, M., Zemanova, J., and Kahoun, D. (2019). Toxicity of atrazine and the products of its homogeneous photocatalytic degradation on the aquatic organisms Lemna minor and Daphnia magna. Environ. Sci. Pollut. R. 26, 27259–27267. doi: 10.1007/s11356-019-05710-0
Langer, S. M., Weiss, L. C., Ekvall, M. T., Bianco, G., Hansson, L.-A., and Tollrian, R. (2019). A three-dimensional perspective of Daphnia's swimming behavior with and without predator cues. Limnol. Oceanogr. 64, 1515–1525. doi: 10.1002/lno.11132
Leblanc, G. A., and Medlock, E. K. (2015). Males on demand: the environmental-neuro-endocrine control of male sex determination in daphnids. FEBS J. 282, 4080–4093. doi: 10.1111/febs.13393
Lind, J., and Cresswell, W. (2005). Determining the fitness consequences of antipredation behavior. Behav. Ecol. 16, 945–956. doi: 10.1093/beheco/ari075
Lu, N., Sun, Y., Wei, J., Gu, L., Zhang, L., Yang, Z., et al. (2021). Toxic Microcystis aeruginosa alters the resource allocation in Daphnia mitsukuri responding to fish predation cues. Environ. Pollut. 278:116918. doi: 10.1016/j.envpol.2021.116918
Lyu, K., Cao, C., Li, D., Akbar, S., and Yang, Z. (2021). The thermal regime modifies the response of aquatic keystone species Daphnia to microplastics: evidence from population fitness, accumulation, histopathological analysis and candidate gene expression. Sci. Total Environ. 783:147154. doi: 10.1016/j.scitotenv.2021.147154
Lyu, K., Gu, L., Wang, H., Zhu, X., Zhang, L., Sun, Y., et al. (2019). Transcriptomic analysis dissects the mechanistic insight into the Daphnia clonal variation in tolerance to toxic Microcystis. Limnol. Oceanogr. 64, 272–283. doi: 10.1002/lno.11038
Lyu, K., Meng, Q., Zhu, X., Dai, D., Zhang, L., Huang, Y., et al. (2016). Changes in iTRAQ-based proteomic profiling of the cladoceran Daphnia magna exposed to microcystin-producing and microcystin-free Microcystis aeruginosa. Environ. Sci. Technol. 50, 4798–4807. doi: 10.1021/acs.est.6b00101
Lyu, K., Zhu, X., Wang, Q., Chen, Y., and Yang, Z. (2013). Copper/zinc superoxide dismutase from the cladoceran Daphnia magna: molecular cloning and expression in response to different acute environmental stressors. Environ. Sci. Technol. 47, 8887–8893. doi: 10.1021/es4015212
Mizota, K., and Ueda, H. (2006). Endocrine disrupting chemical atrazine causes degranulation through G(q/11) protein-coupled neurosteroid receptor in mast cells. Toxicol. Sci. 90, 362–368. doi: 10.1093/toxsci/kfj087
Palma, P., Palma, V. L., Matos, C., Fernandes, R. M., Bohn, A., Soares, A. M. V. M., et al. (2009). Assessment of the pesticides atrazine, endosulfan sulphate and chlorpyrifos for juvenoid-related endocrine activity using Daphnia magna. Chemosphere 76, 335–340. doi: 10.1016/j.chemosphere.2009.03.059
Pathak, R. K., and Dikshit, A. K. (2011). Atrazine and human health. Int. J. Ecosyst. 1, 14–23. doi: 10.5923/j.ije.20110101.03
Peacor, S. D., Pangle, K. L., Schiesari, L., and Werner, E. E. (2012). Scaling-up anti-predator phenotypic responses of prey: impacts over multiple generations in a complex aquatic community. P. Roy. Soc. B-Biol. Sci. 279, 122–128. doi: 10.1098/rspb.2011.0606
Petrusek, A., Tollrian, R., Schwenk, K., Haas, A., and Laforsch, C. (2009). A crown of thorns is an inducible defense that protects Daphnia against an ancient predator. Proc. Natl. Acad. Sci. USA 106, 2248–2252. doi: 10.1073/pnas.0808075106
Pijanowska, J., and Stolpe, G. (1996). Summer diapause in Daphnia as a reaction to the presence of fish. J. Plankton Res. 18, 1407–1412. doi: 10.1093/plankt/18.8.1407
Qin, S., Yang, T., Yu, B., Zhang, L., Gu, L., Sun, Y., et al. (2022). The stress effect of atrazine on the inducible defense traits of Daphnia pulex in response to fish predation risk: evidences from morphology, life history traits, and expression of the defense-related genes. Environ. Pollut. 311:119965. doi: 10.1016/j.envpol.2022.119965
Reger, J., Lind, M. I., Robinson, M. R., and Beckerman, A. P. (2018). Predation drives local adaptation of phenotypic plasticity. Nat. Ecol. Evol. 2, 100–107. doi: 10.1038/s41559-017-0373-6
Religia, P., Kato, Y., Fukushima, E. O., Matsuura, T., Muranaka, T., and Watanabe, H. (2019). Atrazine exposed phytoplankton causes the production of non-viable offspring on Daphnia magna. Mar. Environ. Res. 145, 177–183. doi: 10.1016/j.marenvres.2019.02.007
Robison, A. L., Chapman, T., and Bidwell, J. R. (2018). Predation cues influence metabolic rate and sensitivity to other chemical stressors in fathead minnows (Pimephales promelas) and Daphnia pulex. Ecotoxicology 27, 55–68. doi: 10.1007/s10646-017-1870-8
Rohr, J. R., Park, D., Sullivan, A. M., McKenna, M., Propper, C. R., and Madison, D. M. (2005). Operational sex ratio in newts: field responses and characterization of a constituent chemical cue. Behav. Ecol. 16, 286–293. doi: 10.1093/beheco/arh164
Russo, J., and Lagadic, L. (2000). Effects of parasitism and pesticide exposure on characteristics and functions of hemocyte populations in the freshwater snail Lymnaea palustris (Gastropoda, Pulmonata). Cell Biol.Toxicol. 16, 15–30. doi: 10.1023/A:1007640519746
Sanchez, O. F., Lin, L., Bryan, C. J., Xie, J., Freeman, J. L., and Yuan, C. (2020). Profiling epigenetic changes in human cell line induced by atrazine exposure. Environ. Pollut. 258:113712. doi: 10.1016/j.envpol.2019.113712
Schober, U., and Lampert, W. (1977). Effects of sublethal concentrations of the herbicide atrazin on growth and reproduction of Daphnia pulex. Bull. Environ. Contam. Toxicol. 17, 269–277. doi: 10.1007/BF01686079
Smith, A. S., Acharya, K., and Jack, J. (2009). Overcrowding, food and phosphorus limitation effects on ephipphia production and population dynamics in the invasive species Daphnia lumholtzi. Hydrobiologia 618, 47–56. doi: 10.1007/s10750-008-9546-2
Stibor, H. (1992). Predator induced life-history shifts in a freshwater cladoceran. Oecologia 92, 162–165. doi: 10.1007/BF00317358
Urrutia-Cordero, P., Ekvall, M. K., and Hansson, L. A. (2016). Controlling harmful cyanobacteria: taxa-specific responses of cyanobacteria to grazing by large-bodied Daphnia in a biomanipulation scenario. PLoS One 11:e0153032. doi: 10.1371/journal.pone.0153032
Van de Meutter, F., Stoks, R., and de Meester, L. (2008). Size-selective dispersal of Daphnia resting eggs by backswimmers (Notonecta maculata). Biol. Lett. 4, 494–496. doi: 10.1098/rsbl.2008.0323
Wissinger, S. A., Whiteman, H. H., Denoel, M., Mumford, M. L., and Aubee, C. B. (2010). Consumptive and nonconsumptive effects of cannibalism in fluctuating age-structured populations. Ecology 91, 549–559. doi: 10.1890/08-1366.1
Yang, Z., Xiang, F., Minter, E. J. A., Lue, K., Chen, Y., and Montagnes, D. J. S. (2011). The interactive effects of microcystin and nitrite on life-history parameters of the cladoceran Daphnia obtusa. J. Hazard. Mater. 190, 113–118. doi: 10.1016/j.jhazmat.2011.03.002
Zhou, Q., Huang, J., Gu, L., Lyu, K., Huang, Y., and Yang, Z. (2022). Predator kairomone triggers sexual reproduction of Daphnia population via increasing population density. Freshw. Biol. 67, 1644–1655. doi: 10.1111/fwb.13969
Zhou, Q. M., Lu, N., Gu, L., Sun, Y. F., Zhang, L., Huang, Y., et al. (2020). Daphnia enhances relative reproductive allocation in response to toxic Microcystis: changes in the performance of parthenogenetic and sexual reproduction. Environ. Pollut. 259:113890. doi: 10.1016/j.envpol.2019.113890
Keywords: Daphnia pulex, fish kairomone, population dynamics, resting eggs, atrazine
Citation: Qin S, Xia T, Li G, Gu L, Sun Y and Yang Z (2023) Impact of atrazine on the dynamic response of Daphnia pulex populations to fish predation risk. Front. Ecol. Evol. 10:1068077. doi: 10.3389/fevo.2022.1068077
Edited by:
Pablo Cortes, NEOM, Saudi ArabiaCopyright © 2023 Qin, Xia, Li, Gu, Sun and Yang. This is an open-access article distributed under the terms of the Creative Commons Attribution License (CC BY). The use, distribution or reproduction in other forums is permitted, provided the original author(s) and the copyright owner(s) are credited and that the original publication in this journal is cited, in accordance with accepted academic practice. No use, distribution or reproduction is permitted which does not comply with these terms.
*Correspondence: Lei Gu, ✉ lei.gu@njnu.edu.cn; Zhou Yang, ✉ yangzhou@njnu.edu.cn